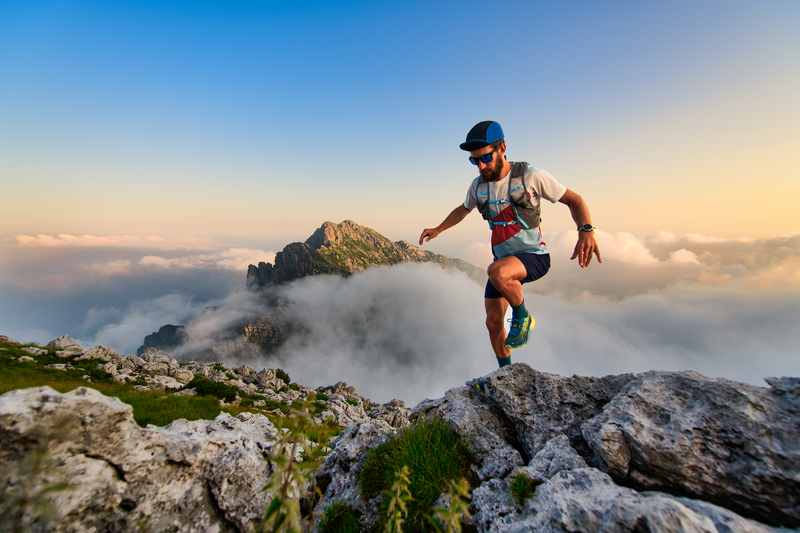
94% of researchers rate our articles as excellent or good
Learn more about the work of our research integrity team to safeguard the quality of each article we publish.
Find out more
MINI REVIEW article
Front. Genet. , 25 September 2018
Sec. Stem Cell Research
Volume 9 - 2018 | https://doi.org/10.3389/fgene.2018.00418
This article is part of the Research Topic FGF10 in Development, Homeostasis, Disease and Repair After Injury View all 19 articles
The lung is morphologically structured into a complex tree-like network with branched airways ending distally in a large number of alveoli for efficient oxygen exchange. At the cellular level, the adult lung consists of at least 40–60 different cell types which can be broadly classified into epithelial, endothelial, mesenchymal, and immune cells. Fibroblast growth factor 10 (Fgf10) located in the lung mesenchyme is essential to regulate epithelial proliferation and lineage commitment during embryonic development and post-natal life, and to drive epithelial regeneration after injury. The cells that express Fgf10 in the mesenchyme are progenitors for mesenchymal cell lineages during embryonic development. During adult lung homeostasis, Fgf10 is expressed in mesenchymal stromal niches, between cartilage rings in the upper conducting airways where basal cells normally reside, and in the lipofibroblasts adjacent to alveolar type 2 cells. Fgf10 protects and promotes lung epithelial regeneration after different types of lung injuries. An Fgf10-Hippo epithelial-mesenchymal crosstalk ensures maintenance of stemness and quiescence during homeostasis and basal stem cell (BSC) recruitment to further promote regeneration in response to injury. Fgf10 signaling is dysregulated in different human lung diseases including bronchopulmonary dysplasia (BPD), idiopathic pulmonary fibrosis (IPF), and chronic obstructive pulmonary disease (COPD), suggesting that dysregulation of the FGF10 pathway is critical to the pathogenesis of several human lung diseases.
Fibroblast growth factor 10 (Fgf10) was first detected using whole-mount in situ hybridization 20 years ago in the splanchnic mesoderm surrounding the foregut around E9.5 when the primary lung buds start to emerge. Lung primordial mesoderm-specific transcription factor Tbx4 defines the Fgf10 expression domain, at both the anterior and posterior boundaries (Sakiyama et al., 2003). The importance of Fgf10 in lung development is well illustrated by the total failure of lung formation and perinatal lethality of Fgf10 deficient mice (Min et al., 1998; Xu et al., 1998; Sekine et al., 1999). Even though Fgf10 binds with high affinity to Fgfr2b, it has a weaker affinity for Fgfr1b (Ohuchi et al., 2000). The Fgf10 knockout phenotype is phenocopied in mice lacking Fgfr2b (Arman et al., 1999; De Moerlooze et al., 2000), which is highly expressed in respiratory epithelium from the early embryonic lung bud stages through late fetal lung development (Peters et al., 1992). Intriguingly, Fgfr2b has also been detected in the lung mesenchyme (Al Alam et al., 2015), but its mesenchymal function requires further investigation. Although Fgfr2b is a receptor for both Fgf7 and Fgf10 during lung development, Fgf7 knockout mice do not exhibit an obvious lung defect (Guo et al., 1996), even though Fgf7 is expressed in the developing lung mesenchyme starting at E14.5 (Mason et al., 1994). However, overexpression of Fgf7 in mice using the human Sftpc promoter results in severe pulmonary malformations, including bronchial airway enlargement, cystic lung lesions and impaired branching morphogenesis leading to embryonic lethality (Simonet et al., 1995).
From E10.5 to E12.5, Fgf10 expression is restricted to the distal lung mesenchyme at sites where branching occurs (Bellusci et al., 1997) and the ventral mesenchyme of the trachea (Sala et al., 2011; Figure 1A). For a long time, the localized pattern of Fgf10 expression in the distal lung was thought to determine where new lung buds sprout. However, proper epithelial branching still occurs in developing Fgf10-/- lungs in which Fgf10 is overexpressed in every cell. This indicates that the precise spatial organization of Fgf10 expression is not required for the highly preserved and stereotypic branching morphogenesis. Hence, other mechanical and/or signaling pathways systems must be in place to control bud outgrowth. Instead, localized Fgf10 expression in the distal mesenchyme is required to regulate epithelial lineage commitment (Volckaert et al., 2013) by maintaining the undifferentiated status of the distal Sox9-expressing epithelial progenitors and preventing them from differentiating into Sox2pos bronchial epithelium (Figure 1A). Fgf10 achieves this, in part, by activating epithelial β-catenin signaling through activation of Akt, which negatively regulates Sox2 expression (Volckaert et al., 2013). Indeed, Wnt/β-catenin signaling is important for the regulation of proximal-distal differentiation in the developing airway epithelium (De Langhe et al., 2005; Hashimoto et al., 2012; Ostrin et al., 2018). As the epithelium grows out, cells which become further and further displaced from the source of Fgf10 start to differentiate into Sox2pos bronchial epithelium (Volckaert et al., 2013; Volckaert and De Langhe, 2014; Figure 1A). As a corollary, Fgf10 hypomorphs and conditional Fgf10 (Dermo1-cre;Fgf10) and Fgfr2 (Sftpc-cre;Fgfr2) mutants fail to maintain distal progenitors, resulting in a proximalized lung with impaired alveolar epithelial lineage formation and reduced capacity to produce surfactant proteins (Mailleux et al., 2005; Ramasamy et al., 2007; Abler et al., 2009). In addition, in lungs overexpressing Fgf10 early on, distal epithelial progenitors fail to differentiate into bronchial epithelium (Volckaert et al., 2013). Taken together, these findings indicate that epithelial-mesenchymal interactions between Fgfr2b and its ligand Fgf10 is required for lung epithelial lineage commitment (Xu et al., 1998; Sekine et al., 1999; Ohuchi et al., 2000).
FIGURE 1. A Wnt7b-Fgf10 epithelial-mesenchymal crosstalk maintains distal epithelial progenitors during lung development and becomes reactivated in the adult lung to regenerate injured airway epithelium. (A) During the branching stage of lung development, Fgf10 is expressed by mesenchymal progenitor cells, which depends on Wnt/β-catenin signaling, and acts on the distal epithelium to induce Bmp4 and Sox9 expression to keep them in an undifferentiated state. As the epithelial tube grows toward the Fgf10 source, Sox9 + progenitors acquire more proximal positions, switch on Sox2 expression and acquire bronchial epithelial fate. Simultaneously, distal Fgf10-expressing airway smooth muscle (ASMC) progenitors encounter epithelial Bmp4 and Shh (not shown) causing them to stop expressing Fgf10 and differentiate into mature ASMCs as they relocate proximally. (B) In the adult, basal stem cells (BSCs) generate their own Fgf10-expressing niche mediated by Yap-Wnt7b, and their maintenance is critically dependent on Fgf10-Fgfr2b signaling. The non-cartilaginous airway epithelium is kept quiescent during homeostasis, by active Integrin-linked kinase (Ilk)-Hippo signaling, which prevents Fgf10 expression in ASMCs. In response to injury, surviving epithelial cells spread out, leading to a destabilization of Merlin and inactivation (dephosphorylation) of Hippo kinases Mst1/2. This increases nuclear Yap in spreading epithelial cells causing these cells to secrete Wnt7b. Epithelial-derived Wnt7b then acts on ASMCs to induce Fgf10 expression, which is required for epithelial regeneration. Solid cell borders represent lineage labels to follow the fate of epithelial cells in response to injury.
The localized expression of Fgf10 in the trachea, on the other hand, drives submucosal gland (SMG) and basal cell development and their maintenance (Rawlins and Hogan, 2005; Volckaert et al., 2013; Volckaert et al., 2017). At the onset of lung and trachea initiation, Fgf10 is detected in the ventral mesenchyme of the trachea (Sala et al., 2011), and then becomes restricted to the intercartilage mesenchyme at later stages and into adulthood (Sala et al., 2011). Interestingly, although Fgf10-/- and Fgfr2b-/- embryos are born without lungs, they still develop a trachea (Sekine et al., 1999; De Moerlooze et al., 2000; Sala et al., 2011). SMGs are severely reduced in number and size in Fgf10 heterozygotes (Jaskoll et al., 2005; Rawlins and Hogan, 2005). Abnormal function of SMGs of the upper respiratory tract are associated with severe/fatal asthma and cystic fibrosis later in life (Benayoun et al., 2003; Salinas et al., 2005). However, despite the significance of SMGs for human respiratory diseases, little is known about the mechanisms of Fgf10 signaling that controls their growth, differentiation, and homeostasis during early postnatal and adult life.
Overexpression of Fgf10 at later stages of lung development, post-Sox2pos bronchial epithelial specification, directs the differentiation of Sox2pos proximal airway epithelium toward the p63/Krt5pos basal cell lineage while blocking Foxj1pos ciliated cell fate throughout the conducting airway (Volckaert et al., 2013). The cells that express Fgf10 in the mesenchyme are themselves progenitors for airway and vascular smooth muscle cells as well as lipofibroblasts (LIFs) during embryonic development, and a subset of lung resident mesenchymal stem cells during adult life (Mailleux et al., 2005; Taniguchi et al., 2007; El Agha et al., 2014). Interestingly, Fgf10 also directly and indirectly orchestrates differentiation of these mesenchymal progenitors (El Agha and Bellusci, 2014; Chao et al., 2015). Epithelial BMP4, a target of Fgf10, controls the differentiation of cells arising from the distal mesenchymal Fgf10-expression domain into the airway smooth muscle cell (ASMC) lineage (Mailleux et al., 2005). In addition, Fgf10 hypomorphs demonstrate defective formation of alveolar myofibroblasts (aMYFs) at different developmental stages (Mailleux et al., 2005; Ramasamy et al., 2007).
Starting at E16.5, Id2pos Sox9pos Sftpcpos Pdpnpos alveolar/bipotent epithelial progenitors give rise to alveolar type I and II (AT1/AT2) cells (Desai et al., 2014; Treutlein et al., 2014). Alveolar epithelial differentiation is coordinated by both mechanical forces and growth factors. In this context, it was recently shown that mechanical forces generated by fetal breathing movements stimulate AT1 cell differentiation, whereas Fgf10-mediated ERK1/2 signaling in distal progenitor cells prevents them from differentiating, thereby ensuring their AT2 fate (Li et al., 2018). In the mesenchyme, Glipos Pdgfrapos mesenchymal progenitor cells give rise to aMYFs and LIFs (Li et al., 2015; Chao et al., 2016). Although aMYFs and LIFs are both derived from Gli1pos Pdgfrαpos mesenchymal progenitors, LIFs exhibit lower Pdgfrαpos expression and higher levels of Fgf10 expression in association with its receptors Fgfr1b and Fgfr2b. This suggests that different Fgfr and ligand profiles might mediate the direction of differentiation from Pdgfrαpos mesenchymal progenitors toward LIF or aMYF (McGowan and McCoy, 2015). Interestingly, it has been shown that LIFs consist of both Fgf10pos and Fgf10neg subpopulations (Al Alam et al., 2015). Fgf10 reduction in Fgf10 hypomorphs as well as knockdown of Fgfr2b ligand in vivo led to significantly decreased expression of LIF marker Adrp at E18.5 in global LIF subpopulations (Fgf10pos and Fgf10neg). This suggests that Fgf10 signals promote the formation of LIFs in an autocrine and/or paracrine fashion (Al Alam et al., 2015). Additionally, constitutive Fgfr1b knockouts and conditional partial loss of Fgfr2b in lung mesenchyme revealed that Fgfr1b and Fgfr2b are likely to play redundant roles in LIF formation (Al Alam et al., 2015). Finally, Apert syndrome mice, which exhibit a splicing switch defect resulting in increased mesenchymal Fgfr2b expression, demonstrate increased Fgf10 expression and signaling in the mesenchyme. These mice display reduced epithelial branching, arrested development of terminal airways and an “emphysema like” phenotype in post-natal lungs resulting from decreased canonical Wnt signaling (De Langhe et al., 2006), likely due to sequestering of the Fgf10 ligand by the misexpressed Fgfr2b receptor.
During homeostasis, adult mouse lungs harbor three main stem cell populations that maintain the lung epithelium: basal stem/progenitor cells (BSCs) in the cartilaginous airways, club cells in the conducting airways and subsets of AT2 cells in the alveoli (Rawlins et al., 2009; Rock et al., 2010; Barkauskas et al., 2013). During homeostasis Fgf10 is expressed in mesenchymal stromal niches, between cartilage rings in the upper conducting airway where basal cells normally reside, and in the LIFs adjacent to AT2 cells in the alveoli (El Agha et al., 2014; Figures 1B, 2).
FIGURE 2. Fgf10-expressing lipofibroblasts are a source of activated myofibroblasts, which re-acquire lipogenic fate during fibrosis resolution. During homeostasis, lipofibroblasts (LIFs) express Fgf10 and are near alveolar type II (AT2) cells. In response to bleomycin-mediated alveolar epithelial injury, LIFs undergo a lipogenic to myogenic switch in fibroblastic phenotype and upregulate Fgf10 expression. Vice versa, during fibrosis resolution, myofibroblasts re-acquire lipofibroblast fate yet retain high Fgf10 expression. Solid cell borders represent lineage labels to follow the fate of (lipo)fibroblasts.
BSCs are progenitors for club, Tuft1/2, neuroendocrine and ionocyte cells (Rock et al., 2009, 2010; Montoro et al., 2018). In the developing trachea, Fgf10 secreted by the inter-cartilage stromal tissue is involved in the development and maintenance of BSCs (Figure 1B). Overexpression of Fgf10 in the trachea leads to BSC amplification whereas overexpressing Fgf10 in adult club cells extends the BSC niche and induces club and BSC hyperplasia in conducting airways (Volckaert et al., 2017). Consistently, both Fgfr2b ligands Fgf7 and Fgf10 can promote basal cell colony expansion in vitro (Balasooriya et al., 2017). Furthermore, Fgfr2b signaling in the trachea is required for BSC maintenance during adult lung homeostasis (Volckaert et al., 2013, 2017). Even loss of one copy of Fgfr2 in adult mouse airway BSCs is sufficient to reduce BSC self-renewal with cells quickly becoming senescent (Balasooriya et al., 2017). Interestingly, conditional deletion of Fgfr1 or Spry2 specifically in adult mouse tracheal BSCs using the Krt5 promoter causes increased ERK/AKT signaling and BSC proliferation and a block in ciliated cell differentiation (Balasooriya et al., 2016), possibly due to increased Fgfr2b signaling caused by a lack of Spry2 activation by Fgfr1. This phenotype resembles that of tracheas overexpressing Fgf10, suggesting that this Fgfr1-SPRY2 signaling axis might function to antagonize Fgf10/Fgfr2b/ERK/AKT signaling, which is required for maintaining quiescence and restricting BSC proliferation in the steady-state airway epithelium in vivo.
Recent studies indicate that Fgf10 prevents lung injury and promotes lung epithelial regeneration after various stresses, including bleomycin-induced alveolar epithelial lung injury (Gupte et al., 2009), influenza-induced acute respiratory distress syndrome (Quantius et al., 2016), high altitude pulmonary edema (She et al., 2012), LPS-induced lung injury (Tong et al., 2014), mechanical ventilation induced lung injury (Bi et al., 2014), ischemia-reperfusion lung injury (Fang et al., 2014), hyperoxia-induced neonatal lung injury (Chao et al., 2017), and naphthalene injury (Volckaert et al., 2011). In a post-pneumonectomy model, Fgfr2b ligands were shown to be required for aMYF formation during the regenerative response (Chen et al., 2012).
In the bleomycin model of pulmonary fibrosis, Fgf10 overexpression in the alveolar epithelium of Sftpc-rtTA;Tet-Fgf10 mice attenuates fibrosis through inhibition of TGF-β and improved survival of AT2 cells This indicates that Fgf10 has a protective as well as regenerative effect on epithelial progenitor cells (Gupte et al., 2009). Similarly, Fgf10 via the Grb2-SOS/Ras/Raf-1/MAPK pathway attenuates H2O2-induced alveolar epithelial DNA damage (Upadhyay et al., 2004). Overexpression of a dominant-negative Fgfr2 receptor (dnFgfr), specifically in the lung epithelium, inhibited retinoic acid-induced alveolar regeneration in association with increased PDGFRαpos and reduced expression of SMA in interstitial myofibroblasts (Perl and Gale, 2009). Intra-tracheal administration of Fgf10 attenuates lipopolysaccharide (LPS)-induced acute lung injury with increased AT2 proliferation (Tong et al., 2014). Lung resident mesenchymal stromal cells (MSCs) isolated from Fgf10 pretreated rats are protected against LPS-induced acute lung injury (Tong et al., 2016). However, the mechanism underlying these protective effects of Fgf10 signaling during injury and regeneration in adult lung have not yet been fully elucidated.
Fgf10-expressing cells were identified as a subset of LIF progenitors during embryonic development (El Agha et al., 2014). Fgf10-expressing LIFs have been shown to differentiate into activated MYFs upon bleomycin injury, while simultaneously upregulating their Fgf10 expression levels (El Agha et al., 2017). Fgf10-expressing MYFs dedifferentiate back into LIFs but do not downregulate their Fgf10 expression levels during the resolution phase of lung fibrosis (El Agha et al., 2017) suggesting that they retain a memory of the injury which might protect against further injury. This supports the concept that LIFs serve as a source of activated MYFs during fibrogenesis which revert back to LIFs during fibrosis resolution (El Agha et al., 2017; Figure 2).
Naphthalene injury is a well-established injury model to study conducting airway epithelial regeneration by selectively ablating club cells except for a few naphthalene-resistant club stem cells located at bronchoalveolar duct junctions (BADJs) and adjacent to neuroendocrine bodies (NEBs). In the adult lung, Fgf10 is not expressed in mature ASMCs during homeostasis (Figure 1B). However, upon conducting airway epithelial injury, when surviving differentiated epithelial cells spread in an attempt to maintain barrier function, they downregulate their Hippo pathway to drive Yap into the nucleus, and induce the secretion of Wnt7b. Epithelial-derived Wnt7b, in turn, induces Lgr6pos ASMCs to release Fgf10 (Volckaert et al., 2011, 2017; Volckaert and De Langhe, 2014; Lee et al., 2017), which activates Notch and β-catenin signaling in surviving club cells to drive their amplification to promote regeneration (Volckaert et al., 2011; Lee et al., 2017; Figure 1B). Together, these findings provide strong evidence that ASMCs function as a niche for conducting airway epithelial stem cells. Besides club cell regeneration, the induction of Fgf10 expression by the ASMC niche in non-cartilaginous airways extends the BSC niche, allowing the recruitment of tracheal BSCs and/or driving the differentiation of Sox2posp63posKrt5neg progenitors along the BSC lineage (Volckaert et al., 2017; Yang et al., 2018). In summary, the Fgf10-Hippo epithelial-mesenchymal crosstalk ensures maintenance of stemness and quiescence during homeostasis and recruitment of BSCs to promote regeneration in response to injury (Volckaert et al., 2017; Figure 1B).
A similar tonic Hedgehog signal maintains lung airway epithelial and mesenchymal quiescence in the distal mouse airways (Peng et al., 2015). In this model, loss of Hedgehog signaling drives regeneration in response to naphthalene-induced epithelial injury via a mesenchymal feedback mechanism, and deregulation of hedgehog during naphthalene induced epithelial lung injury leads to aberrant repair and regeneration (Peng et al., 2015). These findings imply that the Wnt-Fgf10 epithelial-mesenchymal cross-talk and Shh pathway may function as an interactive signaling network in airway and alveolar remodeling responses to chronic injury in asthma, chronic obstructive pulmonary disease (COPD) and pulmonary fibrosis.
Several syndromic craniosynostoses have been associated with dominantly acting mutations of FGFR1, FGFR2, and FGFR3 (Hajihosseini et al., 2001). FGFR2B is up-regulated in cultured fibroblasts of some Apert’s and Pfeiffer’s syndrome patients (Oldridge et al., 1999). Gain-of-Fgfr2b function mice Fgfr2c+/Δ show phenotypic resemblance to Apert’s and Pfeiffer’s syndromes, including visceral and growth defects, neonatal growth retardation and death, coronal synostosis, ocular proptosis, precocious sternal fusion, and abnormalities in secondary branching in lung and kidney that undergo branching morphogenesis (Hajihosseini et al., 2001; De Langhe et al., 2006).
In humans, haploinsuffiencies for FGF10 or FGFR2B result in autosomal dominant aplasia of lacrimal and salivary glands and lacrimo auriculo-dentodigital syndrome, respectively (Entesarian et al., 2005; Klar et al., 2011). In the former syndrome, patients exhibit irreversible airway obstruction, indicating that genetic variants affecting the FGF10 signaling pathway are important determinants of lung function which ultimately contribute to COPD (Klar et al., 2011). Notably, an airway branch variant with absence of the right medial-basal airway associated with polymorphisms within the FGF10 gene is associated with COPD among smokers (Smith et al., 2018). Interestingly, increased nuclear YAP levels, along with FGFR2B and WNT7b expression, were observed in squamous metaplastic areas within the airway epithelium of COPD subjects (Volckaert et al., 2017), suggesting that the Hippo pathway is inactivated to induce FGF10 expression and BSC amplification in human COPD.
Bronchopulmonary dysplasia (BPD) is a chronic pulmonary disease of prematurely born infants characterized by arrested alveolar development (Chao et al., 2017). BPD biopsy samples show reduced FGF10 expression (Benjamin et al., 2007), implicating that FGF10 signaling may be involved in BPD. By using hyperoxia-induced neonatal lung injury from post-natal day 0 (P0) to P8 as a mouse model of BPD, Chao et al. (2017) have shown that Fgf10 deficiency causes lethality from P5 in Fgf10+/- pups due to impaired AT2 formation after hyperoxic injury. In this study, overexpression of a secreted dominant negative Fgfr2b, demonstrated that post-natal deficiency of Fgfr2b ligands in the context of hyperoxia-exposure causes decreased Sftpc expression and eventually leads to significant lethality. This indicates that Fgfr2b ligands are important for repair after hyperoxia exposure in neonatal lung.
Idiopathic pulmonary fibrosis (IPF) is a chronic interstitial lung disease characterized by the loss of alveolar epithelial integrity, progressive invasion of the lung parenchyma by myofibroblasts and increased extracellular matrix (ECM) deposition leading to respiratory failure, and death often within 5 years of diagnosis (Thannickal et al., 2004; King et al., 2011; Steele and Schwartz, 2013; Yang et al., 2013). Gene expression profiles of MSCs from IPF patient lungs revealed that FGF10 expression in MSCs is suppressed in IPF subjects with progressive disease, along with upregulation of both TGF-β1 and SHH signaling. This suggests that FGF10 deficiency is a potentially critical factor in disease progression (Chanda et al., 2016). However, recently it has been shown that FGF10 is significantly upregulated at both mRNA and protein level in IPF lungs compared to the donor lungs, especially in dense fibrotic islands where ACTA2pos cells accumulate (El Agha et al., 2017).
Fgf10 signaling is essential for lung development and adult stem cell maintenance. Important questions remain regarding the mechanisms that regulate Fgf10 expression in the niche to unleash the full therapeutic potential of Fgf10. In addition, very little is known about the importance of FGF10 signaling in human lung development and homeostasis. During homeostasis, BSCs are restricted to the cartilaginous airway in mice as they require Fgfr2b signaling for their maintenance, whereas in humans they can be found deep in the lung. However, upon different types of injury BSCs are deployed throughout the mouse lung as ASMCs in the non-cartilaginous airways re-express Fgf10 to regenerate the airway epithelium. It is therefore likely that the apparent restricted BSC pattern in the mouse lung is due to it being housed in a fairly sterile environment rather than constantly being exposed to environmental insults as is the case for humans.
TY, TV, and SDL wrote the manuscript. DC and VT edited the manuscript.
This study was supported by NIH R01 HL126732, HL132156 and March of Dimes Grant No. 1-FY15-463 awarded to SDL.
The authors declare that the research was conducted in the absence of any commercial or financial relationships that could be construed as a potential conflict of interest.
Abler, L. L., Mansour, S. L., and Sun, X. (2009). Conditional gene inactivation reveals roles for Fgf10 and Fgfr2 in establishing a normal pattern of epithelial branching in the mouse lung. Dev. Dyn. 238, 1999–2013. doi: 10.1002/dvdy.22032
Al Alam, D., El Agha, E., Sakurai, R., Kheirollahi, V., Moiseenko, A., Danopoulos, S., et al. (2015). Evidence for the involvement of fibroblast growth factor 10 in lipofibroblast formation during embryonic lung development. Development 142, 4139–4150. doi: 10.1242/dev.109173
Arman, E., Haffner-Krausz, R., Gorivodsky, M., and Lonai, P. (1999). Fgfr2 is required for limb outgrowth and lung-branching morphogenesis. Proc. Natl. Acad. Sci. U.S.A. 96, 11895–11899. doi: 10.1073/pnas.96.21.11895
Balasooriya, G. I., Goschorska, M., Piddini, E., and Rawlins, E. L. (2017). FGFR2 is required for airway basal cell self-renewal and terminal differentiation. Development 144, 1600–1606. doi: 10.1242/dev.135681
Balasooriya, G. I., Johnson, J. A., Basson, M. A., and Rawlins, E. L. (2016). An FGFR1-SPRY2 signaling axis limits basal cell proliferation in the steady-state airway epithelium. Dev. Cell 37, 85–97. doi: 10.1016/j.devcel.2016.03.001
Barkauskas, C. E., Cronce, M. J., Rackley, C. R., Bowie, E. J., Keene, D. R., Stripp, B. R., et al. (2013). Type 2 alveolar cells are stem cells in adult lung. J. Clin. Invest. 123, 3025–3036. doi: 10.1172/JCI68782
Bellusci, S., Grindley, J., Emoto, H., Itoh, N., and Hogan, B. L. (1997). Fibroblast growth factor 10 (FGF10) and branching morphogenesis in the embryonic mouse lung. Development 124, 4867–4878.
Benayoun, L., Druilhe, A., Dombret, M. C., Aubier, M., and Pretolani, M. (2003). Airway structural alterations selectively associated with severe asthma. Am. J. Respir. Crit. Care Med. 167, 1360–1368. doi: 10.1164/rccm.200209-1030OC
Benjamin, J. T., Smith, R. J., Halloran, B. A., Day, T. J., Kelly, D. R., and Prince, L. S. (2007). FGF-10 is decreased in bronchopulmonary dysplasia and suppressed by Toll-like receptor activation. Am. J. Physiol. Lung Cell Mol. Physiol. 292, L550–L558. doi: 10.1152/ajplung.00329.2006
Bi, J., Tong, L., Zhu, X., Yang, D., Bai, C., Song, Y., et al. (2014). Keratinocyte growth factor-2 intratracheal instillation significantly attenuates ventilator-induced lung injury in rats. J. Cell Mol. Med. 18, 1226–1235. doi: 10.1111/jcmm.12269
Chanda, D., Kurundkar, A., Rangarajan, S., Locy, M., Bernard, K., Sharma, N. S., et al. (2016). Developmental reprogramming in mesenchymal stromal cells of human subjects with idiopathic pulmonary fibrosis. Sci. Rep. 6:37445. doi: 10.1038/srep37445
Chao, C. M., El Agha, E., Tiozzo, C., Minoo, P., and Bellusci, S. (2015). A breath of fresh air on the mesenchyme: impact of impaired mesenchymal development on the pathogenesis of bronchopulmonary dysplasia. Front. Med. 2:27. doi: 10.3389/fmed.2015.00027
Chao, C. M., Moiseenko, A., Zimmer, K. P., and Bellusci, S. (2016). Alveologenesis: key cellular players and fibroblast growth factor 10 signaling. Mol. Cell Pediatr. 3:17. doi: 10.1186/s40348-016-0045-7
Chao, C. M., Yahya, F., Moiseenko, A., Tiozzo, C., Shrestha, A., Ahmadvand, N., et al. (2017). Fgf10 deficiency is causative for lethality in a mouse model of bronchopulmonary dysplasia. J. Pathol. 241, 91–103. doi: 10.1002/path.4834
Chen, L., Acciani, T., Le Cras, T., Lutzko, C., and Perl, A. K. (2012). Dynamic regulation of platelet-derived growth factor receptor alpha expression in alveolar fibroblasts during realveolarization. Am. J. Respir. Cell Mol. Biol. 47, 517–527. doi: 10.1165/rcmb.2012-0030OC
De Langhe, S. P., Carraro, G., Warburton, D., Hajihosseini, M. K., and Bellusci, S. (2006). Levels of mesenchymal FGFR2 signaling modulate smooth muscle progenitor cell commitment in the lung. Dev. Biol. 299, 52–62. doi: 10.1016/j.ydbio.2006.07.001
De Langhe, S. P., Sala, F. G., Del Moral, P. M., Fairbanks, T. J., Yamada, K. M., Warburton, D., et al. (2005). Dickkopf-1 (DKK1) reveals that fibronectin is a major target of Wnt signaling in branching morphogenesis of the mouse embryonic lung. Dev. Biol. 277, 316–331. doi: 10.1016/j.ydbio.2004.09.023
De Moerlooze, L., Spencer-Dene, B., Revest, J. M., Hajihosseini, M., Rosewell, I., and Dickson, C. (2000). An important role for the IIIb isoform of fibroblast growth factor receptor 2 (FGFR2) in mesenchymal-epithelial signalling during mouse organogenesis. Development 127, 483–492.
Desai, T. J., Brownfield, D. G., and Krasnow, M. A. (2014). Alveolar progenitor and stem cells in lung development, renewal and cancer. Nature 507, 190–194. doi: 10.1038/nature12930
El Agha, E., and Bellusci, S. (2014). Walking along the fibroblast growth factor 10 route: a key pathway to understand the control and regulation of epithelial and mesenchymal cell-lineage formation during lung development and repair after injury. Scientifica 2014:538379. doi: 10.1155/2014/538379
El Agha, E., Herold, S., Al Alam, D., Quantius, J., MacKenzie, B., Carraro, G., et al. (2014). Fgf10-positive cells represent a progenitor cell population during lung development and postnatally. Development 141, 296–306. doi: 10.1242/dev.099747
El Agha, E., Moiseenko, A., Kheirollahi, V., De Langhe, S., Crnkovic, S., Kwapiszewska, G., et al. (2017). Two-Way conversion between lipogenic and myogenic fibroblastic phenotypes marks the progression and resolution of lung fibrosis. Cell Stem Cell 20, 261.e3–273.e3. doi: 10.1016/j.stem.2017.03.011
Entesarian, M., Matsson, H., Klar, J., Bergendal, B., Olson, L., Arakaki, R., et al. (2005). Mutations in the gene encoding fibroblast growth factor 10 are associated with aplasia of lacrimal and salivary glands. Nat. Genet. 37, 125–127. doi: 10.1038/ng1507
Fang, X., Wang, L., Shi, L., Chen, C., Wang, Q., Bai, C., et al. (2014). Protective effects of keratinocyte growth factor-2 on ischemia-reperfusion-induced lung injury in rats. Am. J. Respir. Cell Mol. Biol. 50, 1156–1165. doi: 10.1165/rcmb.2013-0268OC
Guo, L., Degenstein, L., and Fuchs, E. (1996). Keratinocyte growth factor is required for hair development but not for wound healing. Genes Dev. 10, 165–175.
Gupte, V. V., Ramasamy, S. K., Reddy, R., Lee, J., Weinreb, P. H., Violette, S. M., et al. (2009). Overexpression of fibroblast growth factor-10 during both inflammatory and fibrotic phases attenuates bleomycin-induced pulmonary fibrosis in mice. Am. J. Respir. Crit. Care Med. 180, 424–436. doi: 10.1164/rccm.200811-1794OC
Hajihosseini, M. K., Wilson, S., De Moerlooze, L., and Dickson, C. (2001). A splicing switch and gain-of-function mutation in FgfR2-IIIc hemizygotes causes Apert/Pfeiffer-syndrome-like phenotypes. Proc. Natl. Acad. Sci. U.S.A. 98, 3855–3860. doi: 10.1073/pnas.071586898
Hashimoto, S., Chen, H., Que, J., Brockway, B. L., Drake, J. A., Snyder, J. C., et al. (2012). beta-Catenin-SOX2 signaling regulates the fate of developing airway epithelium. J. Cell Sci. 125(Pt 4), 932–942. doi: 10.1242/jcs.092734
Jaskoll, T., Abichaker, G., Witcher, D., Sala, F. G., Bellusci, S., Hajihosseini, M. K., et al. (2005). FGF10/FGFR2b signaling plays essential roles during in vivo embryonic submandibular salivary gland morphogenesis. BMC Dev. Biol. 5:11. doi: 10.1186/1471-213X-5-11
King, T. E. Jr., Pardo, A., and Selman, M. (2011). Idiopathic pulmonary fibrosis. Lancet 378, 1949–1961. doi: 10.1016/S0140-6736(11)60052-4
Klar, J., Blomstrand, P., Brunmark, C., Badhai, J., Hakansson, H. F., Brange, C. S., et al. (2011). Fibroblast growth factor 10 haploinsufficiency causes chronic obstructive pulmonary disease. J. Med. Genet. 48, 705–709. doi: 10.1136/jmedgenet-2011-100166
Lee, J. H., Tammela, T., Hofree, M., Choi, J., Marjanovic, N. D., Han, S., et al. (2017). Anatomically and functionally distinct lung mesenchymal populations marked by Lgr5 and Lgr6. Cell 170, 1149.e12–1163.e12. doi: 10.1016/j.cell.2017.07.028
Li, C., Li, M., Li, S., Xing, Y., Yang, C. Y., Li, A., et al. (2015). Progenitors of secondary crest myofibroblasts are developmentally committed in early lung mesoderm. Stem Cells 33, 999–1012. doi: 10.1002/stem.1911
Li, J., Wang, Z., Chu, Q., Jiang, K., Li, J., and Tang, N. (2018). The strength of mechanical forces determines the differentiation of alveolar epithelial cells. Dev. Cell 44, 297.e5–312.e5. doi: 10.1016/j.devcel.2018.01.008
Mailleux, A. A., Kelly, R., Veltmaat, J. M., De Langhe, S. P., Zaffran, S., Thiery, J. P., et al. (2005). Fgf10 expression identifies parabronchial smooth muscle cell progenitors and is required for their entry into the smooth muscle cell lineage. Development 132, 2157–2166. doi: 10.1242/dev.01795
Mason, I. J., Fuller-Pace, F., Smith, R., and Dickson, C. (1994). FGF-7 (keratinocyte growth factor) expression during mouse development suggests roles in myogenesis, forebrain regionalisation and epithelial-mesenchymal interactions. Mech. Dev. 45, 15–30. doi: 10.1016/0925-4773(94)90050-7
McGowan, S. E., and McCoy, D. M. (2015). Fibroblast growth factor signaling in myofibroblasts differs from lipofibroblasts during alveolar septation in mice. Am. J. Physiol. Lung Cell Mol. Physiol. 309, L463–L474. doi: 10.1152/ajplung.00013.2015
Min, H., Danilenko, D. M., Scully, S. A., Bolon, B., Ring, B. D., Tarpley, J. E., et al. (1998). Fgf-10 is required for both limb and lung development and exhibits striking functional similarity to Drosophila branchless. Genes Dev. 12, 3156–3161. doi: 10.1101/gad.12.20.3156
Montoro, D. T., Haber, A. L., Biton, M., Vinarsky, V., Lin, B., Birket, S. E., et al. (2018). A revised airway epithelial hierarchy includes CFTR-expressing ionocytes. Nature 560, 319–324. doi: 10.1038/s41586-018-0393-7
Ohuchi, H., Hori, Y., Yamasaki, M., Harada, H., Sekine, K., Kato, S., et al. (2000). FGF10 acts as a major ligand for FGF receptor 2 IIIb in mouse multi-organ development. Biochem. Biophys. Res. Commun. 277, 643–649. doi: 10.1006/bbrc.2000.3721
Oldridge, M., Zackai, E. H., McDonald-McGinn, D. M., Iseki, S., Morriss-Kay, G. M., Twigg, S. R., et al. (1999). De novo alu-element insertions in FGFR2 identify a distinct pathological basis for Apert syndrome. Am. J. Hum. Genet. 64, 446–461. doi: 10.1086/302245
Ostrin, E. J., Little, D. R., Gerner-Mauro, K. N., Sumner, E. A., Rios-Corzo, R., Ambrosio, E., et al. (2018). Beta-Catenin maintains lung epithelial progenitors after lung specification. Development 2018:160788. doi: 10.1242/dev.160788
Peng, T., Frank, D. B., Kadzik, R. S., Morley, M. P., Rathi, K. S., Wang, T., et al. (2015). Hedgehog actively maintains adult lung quiescence and regulates repair and regeneration. Nature 526, 578–582. doi: 10.1038/nature14984
Perl, A. K., and Gale, E. (2009). FGF signaling is required for myofibroblast differentiation during alveolar regeneration. Am. J. Physiol. Lung Cell Mol. Physiol. 297, L299–L308. doi: 10.1152/ajplung.00008.2009
Peters, K. G., Werner, S., Chen, G., and Williams, L. T. (1992). Two FGF receptor genes are differentially expressed in epithelial and mesenchymal tissues during limb formation and organogenesis in the mouse. Development 114, 233–243.
Quantius, J., Schmoldt, C., Vazquez-Armendariz, A. I., Becker, C., El Agha, E., Wilhelm, J., et al. (2016). Influenza virus infects epithelial stem/progenitor cells of the distal lung: impact on Fgfr2b-Driven epithelial repair. PLoS Pathog. 12:e1005544. doi: 10.1371/journal.ppat.1005544
Ramasamy, S. K., Mailleux, A. A., Gupte, V. V., Mata, F., Sala, F. G., Veltmaat, J. M., et al. (2007). Fgf10 dosage is critical for the amplification of epithelial cell progenitors and for the formation of multiple mesenchymal lineages during lung development. Dev. Biol. 307, 237–247. doi: 10.1016/j.ydbio.2007.04.033
Rawlins, E. L., and Hogan, B. L. (2005). Intercellular growth factor signaling and the development of mouse tracheal submucosal glands. Dev. Dyn. 233, 1378–1385. doi: 10.1002/dvdy.20461
Rawlins, E. L., Okubo, T., Xue, Y., Brass, D. M., Auten, R. L., Hasegawa, H., et al. (2009). The role of Scgb1a1 + Clara cells in the long-term maintenance and repair of lung airway, but not alveolar, epithelium. Cell Stem Cell 4, 525–534. doi: 10.1016/j.stem.2009.04.002
Rock, J. R., Onaitis, M. W., Rawlins, E. L., Lu, Y., Clark, C. P., Xue, Y., et al. (2009). Basal cells as stem cells of the mouse trachea and human airway epithelium. Proc. Natl. Acad. Sci. U.S.A. 106, 12771–12775. doi: 10.1073/pnas.0906850106
Rock, J. R., Randell, S. H., and Hogan, B. L. (2010). Airway basal stem cells: a perspective on their roles in epithelial homeostasis and remodeling. Dis. Model. Mech. 3, 545–556. doi: 10.1242/dmm.006031
Sakiyama, J., Yamagishi, A., and Kuroiwa, A. (2003). Tbx4-Fgf10 system controls lung bud formation during chicken embryonic development. Development 130, 1225–1234. doi: 10.1242/dev.00345
Sala, F. G., Del Moral, P. M., Tiozzo, C., Alam, D. A., Warburton, D., Grikscheit, T., et al. (2011). FGF10 controls the patterning of the tracheal cartilage rings via Shh. Development 138, 273–282. doi: 10.1242/dev.051680
Salinas, D., Haggie, P. M., Thiagarajah, J. R., Song, Y., Rosbe, K., Finkbeiner, W. E., et al. (2005). Submucosal gland dysfunction as a primary defect in cystic fibrosis. FASEB J. 19, 431–433. doi: 10.1096/fj.04-2879fje
Sekine, K., Ohuchi, H., Fujiwara, M., Yamasaki, M., Yoshizawa, T., Sato, T., et al. (1999). Fgf10 is essential for limb and lung formation. Nat. Genet. 21, 138–141. doi: 10.1038/5096
She, J., Goolaerts, A., Shen, J., Bi, J., Tong, L., Gao, L., et al. (2012). KGF-2 targets alveolar epithelia and capillary endothelia to reduce high altitude pulmonary oedema in rats. J. Cell Mol. Med. 16, 3074–3084. doi: 10.1111/j.1582-4934.2012.01588.x
Simonet, W. S., DeRose, M. L., Bucay, N., Nguyen, H. Q., Wert, S. E., Zhou, L., et al. (1995). Pulmonary malformation in transgenic mice expressing human keratinocyte growth factor in the lung. Proc. Natl. Acad. Sci. U.S.A. 92, 12461–12465. doi: 10.1073/pnas.92.26.12461
Smith, B. M., Traboulsi, H., Austin, J. H. M., Manichaikul, A., Hoffman, E. A., Bleecker, E. R., et al. (2018). Human airway branch variation and chronic obstructive pulmonary disease. Proc. Natl. Acad. Sci. U.S.A. 115, E974–E981. doi: 10.1073/pnas.1715564115
Steele, M. P., and Schwartz, D. A. (2013). Molecular mechanisms in progressive idiopathic pulmonary fibrosis. Annu. Rev. Med. 64, 265–276. doi: 10.1146/annurev-med-042711-142004
Taniguchi, K., Ayada, T., Ichiyama, K., Kohno, R., Yonemitsu, Y., Minami, Y., et al. (2007). Sprouty2 and Sprouty4 are essential for embryonic morphogenesis and regulation of FGF signaling. Biochem. Biophys. Res. Commun. 352, 896–902. doi: 10.1016/j.bbrc.2006.11.107
Thannickal, V. J., Toews, G. B., White, E. S., Lynch, J. P. III, and Martinez, F. J. (2004). Mechanisms of pulmonary fibrosis. Annu. Rev. Med. 55, 395–417. doi: 10.1146/annurev.med.55.091902.103810
Tong, L., Bi, J., Zhu, X., Wang, G., Liu, J., Rong, L., et al. (2014). Keratinocyte growth factor-2 is protective in lipopolysaccharide-induced acute lung injury in rats. Respir. Physiol. Neurobiol. 201, 7–14. doi: 10.1016/j.resp.2014.06.011
Tong, L., Zhou, J., Rong, L., Seeley, E. J., Pan, J., Zhu, X., et al. (2016). Fibroblast growth factor-10 (FGF-10) mobilizes lung-resident mesenchymal stem cells and protects against acute lung injury. Sci. Rep. 6:21642. doi: 10.1038/srep21642
Treutlein, B., Brownfield, D. G., Wu, A. R., Neff, N. F., Mantalas, G. L., Espinoza, F. H., et al. (2014). Reconstructing lineage hierarchies of the distal lung epithelium using single-cell RNA-seq. Nature 509, 371–375. doi: 10.1038/nature13173
Upadhyay, D., Bundesmann, M., Panduri, V., Correa-Meyer, E., and Kamp, D. W. (2004). Fibroblast growth factor-10 attenuates H2O2-induced alveolar epithelial cell DNA damage: role of MAPK activation and DNA repair. Am. J. Respir. Cell Mol. Biol. 31, 107–113. doi: 10.1165/rcmb.2003-0064OC
Volckaert, T., Campbell, A., Dill, E., Li, C., Minoo, P., and De Langhe, S. (2013). Localized Fgf10 expression is not required for lung branching morphogenesis but prevents differentiation of epithelial progenitors. Development 140, 3731–3742. doi: 10.1242/dev.096560
Volckaert, T., and De Langhe, S. (2014). Lung epithelial stem cells and their niches: Fgf10 takes center stage. Fibrogenesis Tissue Repair 7:8. doi: 10.1186/1755-1536-7-8
Volckaert, T., Dill, E., Campbell, A., Tiozzo, C., Majka, S., Bellusci, S., et al. (2011). Parabronchial smooth muscle constitutes an airway epithelial stem cell niche in the mouse lung after injury. J. Clin. Invest. 121, 4409–4419. doi: 10.1172/JCI58097
Volckaert, T., Yuan, T., Chao, C. M., Bell, H., Sitaula, A., Szimmtenings, L., et al. (2017). Fgf10-hippo epithelial-mesenchymal crosstalk maintains and recruits lung basal stem cells. Dev. Cell 43, 48.e5–59.e5. doi: 10.1016/j.devcel.2017.09.003
Xu, X., Weinstein, M., Li, C., Naski, M., Cohen, R. I., Ornitz, D. M., et al. (1998). Fibroblast growth factor receptor 2 (FGFR2)-mediated reciprocal regulation loop between FGF8 and FGF10 is essential for limb induction. Development 125, 753–765.
Yang, J., Wheeler, S. E., Velikoff, M., Kleaveland, K. R., LaFemina, M. J., Frank, J. A., et al. (2013). Activated alveolar epithelial cells initiate fibrosis through secretion of mesenchymal proteins. Am. J. Pathol. 183, 1559–1570. doi: 10.1016/j.ajpath.2013.07.016
Keywords: Fgf10, regeneration, epithelium, fibrosis, injury
Citation: Yuan T, Volckaert T, Chanda D, Thannickal VJ and De Langhe SP (2018) Fgf10 Signaling in Lung Development, Homeostasis, Disease, and Repair After Injury. Front. Genet. 9:418. doi: 10.3389/fgene.2018.00418
Received: 06 August 2018; Accepted: 06 September 2018;
Published: 25 September 2018.
Edited by:
Saverio Bellusci, Justus Liebig Universität Gießen, GermanyReviewed by:
Konstantinos Gkatzis, Max-Planck-Institut für Herz- und Lungenforschung, GermanyCopyright © 2018 Yuan, Volckaert, Chanda, Thannickal and De Langhe. This is an open-access article distributed under the terms of the Creative Commons Attribution License (CC BY). The use, distribution or reproduction in other forums is permitted, provided the original author(s) and the copyright owner(s) are credited and that the original publication in this journal is cited, in accordance with accepted academic practice. No use, distribution or reproduction is permitted which does not comply with these terms.
*Correspondence: Stijn P. De Langhe, c2RlbGFuZ2hlQHVhYm1jLmVkdQ==
Disclaimer: All claims expressed in this article are solely those of the authors and do not necessarily represent those of their affiliated organizations, or those of the publisher, the editors and the reviewers. Any product that may be evaluated in this article or claim that may be made by its manufacturer is not guaranteed or endorsed by the publisher.
Research integrity at Frontiers
Learn more about the work of our research integrity team to safeguard the quality of each article we publish.