- 1The Roslin Institute and Royal (Dick) School of Veterinary Studies, The University of Edinburgh, Edinburgh, United Kingdom
- 2Facultad de Ciencias Veterinarias y Pecuarias, Universidad de Chile, Santiago, Chile
- 3Aquainnovo S.A., Puerto Montt, Chile
Sea lice are parasitic copepods that cause large economic losses to salmon aquaculture worldwide. Frequent chemotherapeutic treatments are typically required to control this parasite, and alternative measures such as breeding for improved host resistance are desirable. Insight into the host–parasite interaction and mechanisms of host resistance can lead to improvements in selective breeding, and potentially novel treatment targets. In this study, RNA sequencing was used to study the skin transcriptome of Atlantic salmon (Salmo salar) parasitized with sea lice (Caligus rogercresseyi). The overall aims were to compare the transcriptomic profile of skin at louse attachment sites and “healthy” skin, and to assess differences in gene expression response between animals with varying levels of resistance to the parasite. Atlantic salmon pre-smolts were challenged with C. rogercresseyi, growth and lice count measurements were taken for each fish. 21 animals were selected and RNA-Seq was performed on skin from a louse attachment site, and skin distal to attachment sites for each animal. These animals were classified into family-balanced groups according to the traits of resistance (high vs. low lice count), and growth during infestation. Overall comparison of skin from louse attachment sites vs. healthy skin showed that 4,355 genes were differentially expressed, indicating local up-regulation of several immune pathways and activation of tissue repair mechanisms. Comparison between resistant and susceptible animals highlighted expression differences in several immune response and pattern recognition genes, and also myogenic and iron availability factors. Components of the pathways involved in differential response to sea lice may be targets for studies aimed at improved or novel treatment strategies, or to prioritize candidate functional polymorphisms to enhance genomic selection for host resistance in commercial salmon breeding programs.
Introduction
Aquaculture is currently the fastest growing food industry (Food and Agriculture Organization of the United Nations, 2016) and is essential to meet increasing global demands for fish. However, the sustainability and prolonged success of any farming industry depends on effective disease prevention and control, and this tends to be particularly challenging for aquaculture. The aquatic environment and high stock density can expedite pathogen spread, which has historically resulted in periodic mass mortality events (Lafferty et al., 2015; Food and Agriculture Organization of the United Nations, 2017) and ongoing challenges in disease prevention and control. While biosecurity measures, vaccination, nutrition, and medicines all play vital roles for several diseases, selective breeding to produce more resistant and tolerant aquaculture stocks is rapidly becoming a key component of the battle to prevent these outbreaks (Yáñez et al., 2014a; Palaiokostas et al., 2016).
Sea lice, ectoparasites of the family Caligidae, are one of the major disease problems that the aquaculture industry is facing, and specifically for salmon farming. Atlantic salmon (Salmo salar) is the most important species in aquaculture with a production value of 14.7 billion US dollars in 2014 (Food and Agriculture Organization of the United Nations, 2016), therefore control of sea lice is a primary goal for the industry. Sea lice-related economic losses to worldwide salmonid aquaculture were estimated at ∼430 million USD per annum (Costello, 2009). Two lice species present the primary concerns for salmon farming: primarily Lepeophtheirus salmonis in the Northern Hemisphere and Caligus rogercresseyi in the Southern Hemisphere (Johnson et al., 2004). These copepods parasitize salmon during the marine phase of the lifecycle by attaching to their skin or fins, and feeding on the blood and tissue. This leads to open wounds which can facilitate the entry of other pathogens. The impaired growth and secondary infections cause significant negative animal welfare and economic impact (Frazer et al., 2012). Despite extensive use of both chemical (i.e., hydrogen peroxide, emamectin benzoate, organophosphates, pyrethroids, or benzoyl ureas) and non-chemical treatments (i.e., fresh water bath) to control sea lice, their negative impact on salmon aquaculture has increased in the past years (Torrisen et al., 2013), and various sea lice populations have been reported to be resistant to the most common chemicals available for therapeutic control, such as emamectin benzoate, azamethiphos (organophosphate), deltamethrin (pyrethroid), and even hydrogen peroxide (Aaen et al., 2015). Therefore, alternative methods to control sea lice are currently being studied, including the use of probiotics to reduce salmon attractiveness for sea lice (Jodaa et al., 2016) or cohabitation with lice-eating species (Imsland et al., 2014; Leclercq et al., 2014).
Knowledge of the interaction between salmon and sea lice can help devise more effective prevention and treatment strategies. Therefore, a lot of effort has been put in characterizing the host response to sea lice infestation (reviewed in Fast, 2014). Interestingly, the outcome of infestation varies for different salmonid species (Johnson and Albright, 1992), with coho salmon (Oncorhynchus kisutch) showing rapid inflammatory response and epithelial hyperplasia, leading to parasite encapsulation and more than 90% reduction in lice loads (Fast, 2014). In comparison, Atlantic salmon (S. salar) is highly susceptible to sea lice infestation and seemingly cannot mount a fully effective immune response (Fast, 2014). Comparative transcriptomics has shown that iron sequestration, increased expression of pattern recognition receptors such as c-type lectins and up-regulation of pro-inflammatory cytokines such as interleukin-1β are observed in salmon species resistant to sea lice (Sutherland et al., 2014). Interleukin-1β has also been implicated in successful responses to sea lice in other salmonid species such as pink salmon (Oncorhynchus gorbuscha) and coho salmon (Braden et al., 2012, 2015; Sutherland et al., 2015), and recently immunostimulant feeds up-regulating interleukin-1β in skin and spleen have shown some promising results to boost Atlantic salmon resistance to sea lice (Sutherland et al., 2017). While these studies have mainly focused on L. salmonis, similar findings have been observed in C. rogercresseyi infestation. For instance, comparative analyses of Atlantic and coho salmon parasitized with C. rogercresseyi showed that despite both showing up-regulation of pro-inflammatory genes, the response was highly specific, characterized in coho by an activation of the TH1 response (Valenzuela-Muñoz et al., 2016). Another study linked iron sequestration and depletion mechanisms to the Atlantic salmon immune response to C. rogercresseyi (Valenzuela-Muñoz et al., 2017).
A promising and potentially complementary approach to existing control measures is to exploit natural genetic variation in farmed salmon populations to breed stocks with enhanced resistance to the parasite. The presence of significant genetic variation for resistance to C. rogercresseyi, with heritability values ranging between 0.1 and 0.34, demonstrates the feasibility of improving this trait by selective breeding in Atlantic salmon (Lhorente et al., 2014; Yáñez et al., 2014b). Current evidence indicates that host resistance to sea lice in Atlantic salmon has a highly polygenic genetic basis, with little evidence for major QTL (Ødegård et al., 2014; Gharbi et al., 2015; Correa et al., 2016, 2017; Tsai et al., 2016). Therefore, genomic selection using genome-wide markers to predict lice resistant breeding values has been widely applied in commercial Atlantic salmon breeding programs, with a relative advantage compared to pedigree selection of 10–27% (Tsai et al., 2016; Correa et al., 2017). Understanding the underlying functional basis of genetic resistance to sea lice can lead to improved methods of selective breeding. For example, incorporating functional variants into genomic prediction models could help improve prediction accuracy, in particular for cross-population prediction (MacLeod et al., 2016). Functional annotation of reference genomes is pertinent to this process, and the emerging Functional Annotation of All Salmonid Genomes (FAASG) project (Macqueen et al., 2017) is aiming to improve genome annotation for Atlantic salmon (among other species). Further, the discovery of putatively causative genes and variants could, in the near future, lead to their introduction into populations or species where it has never been present through the use of genome editing, for example using CRISPR-Cas9 technology, which has been successfully applied in salmon to knockout two genes related to pigmentation (tyrosinase and solute carrier family 45 member 2) and the dnd (dead end) gene, producing albino (Edvardsen et al., 2014), and germ cell-free salmon (Wargelius et al., 2016), respectively.
Expression differences between Atlantic salmon resistant and susceptible families in response to L. salmonis for 32 immune genes suggested that resistant fish are better at avoiding immunosuppression (Holm et al., 2015). The same study found suggestive evidence that physical tissue barrier such as enhanced mucus production does not contribute to resistance (Holm et al., 2015). However, to our knowledge, the functional basis of genomic resistance to sea lice in Atlantic salmon has not been studied on a genome-wide scale, nor it has been explored in response to C. rogercresseyi. RNA sequencing can provide a first layer toward a holistic view of the host response to parasite infection, which in turn can highlight specific genes, pathways, and networks involved in the host–parasite interaction. RNA sequencing can also be used to identify single nucleotide polymorphisms in transcribed regions, and to assess the putative impact of those genetic markers on transcript and protein function. The effect of these markers on gene expression (and ultimately host resistance) can be assessed by allelic-specific expression or expression QTL studies, leading to a shortlist of candidate functional variants.
The overall aims of the current study were to compare the transcriptome profile of salmon skin at louse attachment sites and “healthy” skin (from the same fish), and to evaluate differences in these profiles between animals with varying levels of resistance to the parasite. To achieve this, challenged animals were classified into family-balanced groups according to resistance (based on high vs. low lice count) and growth during infestation, and RNA sequencing was performed on individual samples. By comparing resistant vs. susceptible samples, genes and pathways related to local immune response and host resistance were identified, and their potential role discussed.
Materials and Methods
Experimental Design
2,668 Atlantic salmon (S. salar) pre-smolts (average weight: 136 g) from 104 families from the breeding population of AquaInnovo (Salmones Chaicas, Xth Region, Chile), were experimentally challenged with C. rogercresseyi (chalimus II–III). This population will be used for a future study on sea lice resistance genetic architecture and genomic selection. Briefly, infestation with the parasite was carried out by using 13–24 copepodids per fish and stopping the water flow for 6 h after infestation. Eight days after the infestation fish were euthanized and fins from each fish were collected and fixed for processing and lice counting. 42 samples from 21 fish from 6 different families (2–5 fish per family) were selected for RNA sequencing (Supplementary File S1) based on the traits of interest (number of sea lice attached to their fins and growth during challenge). Skin samples (both from attachment sites and health skin) were obtained from each animal and stored in RNAlater at 4°C for 24 h, and then at -20°C until RNA extraction for sequencing.
RNA Extraction and Sequencing
For all the 42 samples a standard TRI Reagent RNA extraction protocol was followed. Briefly, approximately 50 mg of skin was homogenized in 1 ml of TRI Reagent (Sigma, St. Louis, MO, United States) by shaking using 1.4 mm silica beads, then 100 μl of 1-bromo-3-chloropropane (BCP) was added for phase separation. This was followed by precipitation with 500 μl of isopropanol and posterior washes with 65–75% ethanol. The RNA was then resuspended in RNAse-free water and treated with Turbo DNAse (Ambion). Samples were then cleaned up using Qiagen RNeasy Mini kit columns and their integrity was checked on Agilent 2200 Bioanalyzer (Agilent Technologies, United States). Thereafter, the Illumina Truseq mRNA stranded RNA-Seq Library Prep Kit protocol was followed directly. Libraries were checked for quality and quantified using the Bioanalyzer 2100 (Agilent), before being sequenced on three lanes of the Illumina Hiseq 4000 instrument using 75 base paired-end sequencing at Edinburgh Genomics, United Kingdom. Raw reads have been deposited in NCBI’s Sequence Read Archive (SRA) under Accession No. SRP100978.
Read Mapping
The quality of the sequencing output was assessed using FastQC v.0.11.5.1 Quality filtering and removal of residual adaptor sequences was conducted on read pairs using Trimmomatic v.0.32 (Bolger et al., 2004). Specifically, Illumina specific adaptors were clipped from the reads, leading, and trailing bases with a Phred score less than 20 were removed and the read trimmed if the sliding window average Phred score over four bases was less than 20. Only reads where both pairs were longer than 36 bp post-filtering were retained. Filtered reads were mapped to the most recent Atlantic salmon genome assembly (ICSASG_v2; GenBank Accession No. GCF_000233375.1; Lien et al., 2016) using STAR v.2.5.2b (Dobin et al., 2013), the maximum number of mismatches for each read pair was set to 10% of trimmed read length, and minimum and maximum intron lengths were set to 20 bases and 1 Mb, respectively. Uniquely mapped paired-reads were counted and assigned to genes (NCBI S. salar Annotation Release 100) using FeatureCounts (Liao et al., 2014), included in the SourceForge Subread package v.1.5.0. Only reads with both ends mapped to the same gene were considered in downstream analyses.
Differential Expression
Differential expression analyses and gene functional and pathway enrichment analyses were performed using R v.3.3.1 (R Core Team, 2014). Gene count data were used to estimate differential gene expression using the Bioconductor package DESeq2 v.3.4 (Love et al., 2014). Briefly, size factors were calculated for each sample using the median of ratios method and count data was normalized to account for differences in library depth, next gene-wise dispersion estimates were fitted to the mean intensity using a parametric model and shrinked toward the expected dispersion values, finally a gegative binomial model was fitted for each gene and the significance of the coefficients was assessed using the Wald test. The Benjamini–Hochberg false discovery rate (FDR) multiple test correction was applied, and transcripts with FDR < 0.05 and absolute log2 fold change values (FC) > 0.5 were considered differentially expressed genes. Hierarchical clustering and principal component analyses were performed to visually identify outlier samples that did not cluster close to other samples in the same category (lice attachment site or healthy skin), which were then removed from the analyses as sampling errors could not be discounted. PCA plots were created using the R package factoextra.2
Pathway Enrichment
Gene Ontology (GO) enrichment analyses were performed using Blast2GO v.4.1 (Conesa et al., 2005). Briefly, genes showing >10 reads in >90% of the samples were annotated against the manually curated protein database Swiss-Prot (Bairoch et al., 2004) and GO terms were assigned to them using Blast2GO. GO enrichment for specific genes lists was tested against the whole set of expressed genes using Fisher’s exact test. GO terms with ≥5 DE genes assigned and showing a Benjamini–Hochberg FDR corrected p-value < 0.05 were considered enriched. Kyoto Encyclopedia of Genes and Genomes (KEGG) enrichment analyses were performed using KOBAS v3.0.3 (Wu et al., 2006). Briefly, genes showing >10 reads in >90% of the samples were annotated against KEGG protein database (Kanehisa and Goto, 2000) to determine KEGG Orthology. KEGG enrichment for specific gene lists was tested by comparison to the whole set of expressed genes using Fisher’s exact test. KEGG pathways with ≥5 differentially expressed (DE) genes assigned and showing a Benjamini–Hochberg FDR corrected p-value < 0.05 were considered enriched.
Results and Discussion
Disease Challenge
A total of 2,632 fish belonging to 105 families from a commercial breeding program were challenged with C. rogercresseyi copepods, and euthanized for sampling 8 days post-challenge. Average lice burden per fish was 38 ± 16, and the estimated heritability of sea lice load was 0.28 ± 0.04 (unpublished results), therefore the differences in sea lice counts between fish has a genetic component. Fish were selected for RNA sequencing based on the traits of resistance, measured as number and concentration of lice per fish, and weight and length gain since the start of the challenge, which may reflect the ability of the fish to cope with the infestation. The selected fish allowed for 8 vs. 8 comparisons between family-matched fish showing differential resistance (26.2 ± 5.5 vs. 54.9 ± 13.5 sea lice per fish) and differential growth during infestation (7.0 ± 4.3 vs. 28.8 ± 12.3 weight gain percentage). A total of 42 samples (21 fish, skin from sites of louse attachment and healthy skin) were sequenced, resulting in an average of ∼27.9 ± 2.7 million reads per sample. After trimming, these were aligned against the salmon reference genome (ICSASG_v2; GenBank Accession No. GCF_000233375.1; Lien et al., 2016) and levels of gene expression were estimated according to the official salmon genome annotation (NCBI S. salar Annotation Release 100). An average of 19 M trimmed reads per sample were assigned to genes and used for downstream analyses of gene expression. All raw sequence data is available in NCBI’s SRA under BioProject Accession No. SRP100978, and may be a useful contribution to the functional annotation of all salmonid genomes initiative (FAASG; Macqueen et al., 2017).
Louse Attachment Sites Versus Healthy Skin
Principal component analysis of gene expression (Figure 1) revealed a relatively clear cluster of healthy skin samples, while lice-attachment samples were more scattered, probably reflecting variation in the individual response to sea lice. Differential expression between healthy and louse attachment sites resulted in 4,355 DE genes (Supplementary File S2), with a higher number of up-regulated (more expressed in attachment sites) than down-regulated genes (n = 3,114 vs. n = 1,241). Among these DE genes were well-known components of the innate immune response such as interleukins, interferon response factors and complement components (Figure 2A). GO term and KEGG pathway analyses (Supplementary File S2) revealed a clear enrichment of immune pathways and functions among the up-regulated genes (Figure 2B), highlighting a localized immune response strongly related to cytokine activity. A similar scenario has been observed in other salmonids such as coho salmon where resistance to sea lice has been associated with early inflammation in skin and head kidney, which results in epithelial hyperplasia and often parasite encapsulation and removal of the sea lice within 2 weeks (Johnson and Albright, 1992; Fast et al., 2002). In pink salmon, an early and high expression of pro-inflammatory genes (IL-8, TNFα-1, and IL-1β) has been suggested as a mechanism of rapid louse rejection (Fast et al., 2007). The classical complement pathway has also been linked to resistance of host fish to parasitic copepod infection (Fast, 2014). The results presented here indicate that despite a marked up-regulation of the local inflammatory response and complement pathway in Atlantic salmon, in part resembling the response of coho salmon or pink salmon, this does not seem to be sufficient to successfully respond to the louse attachment and feeding.
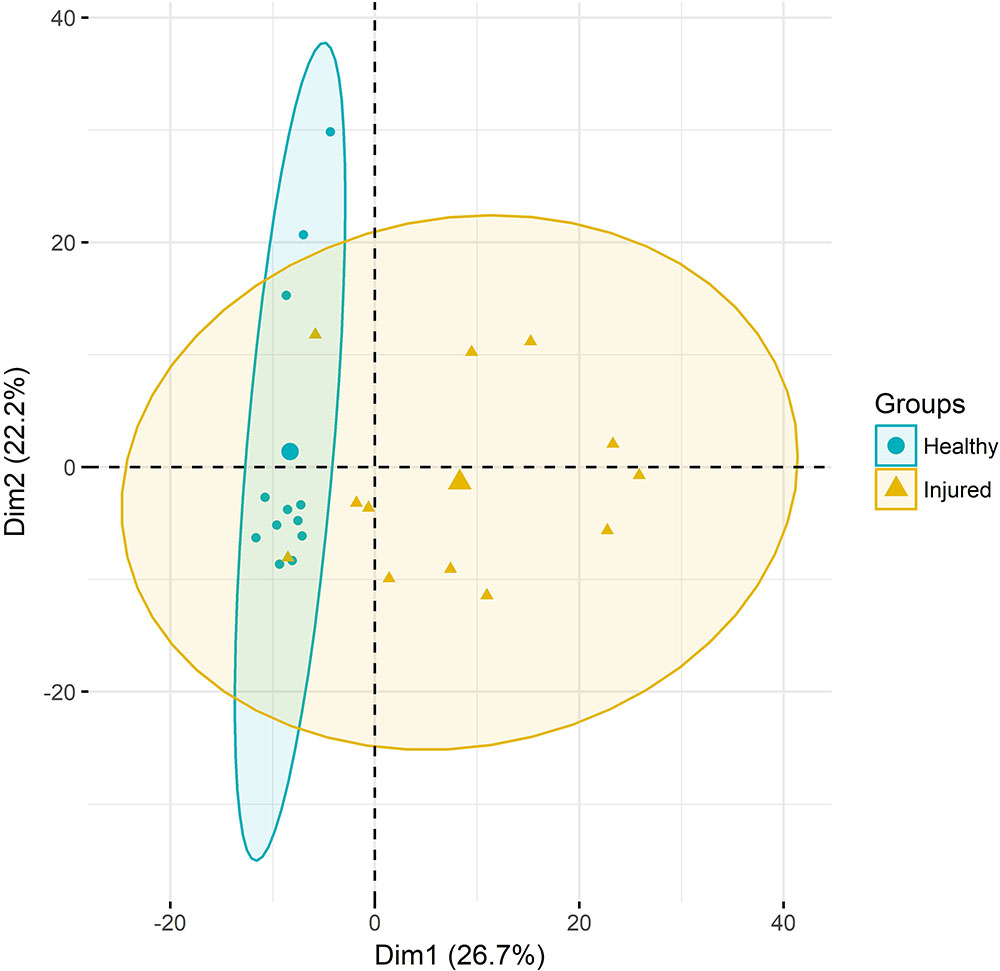
FIGURE 1. Principal component analyses. RNA-Seq samples clustered according to their gene expression. Ellipses represent 95% confidence intervals.
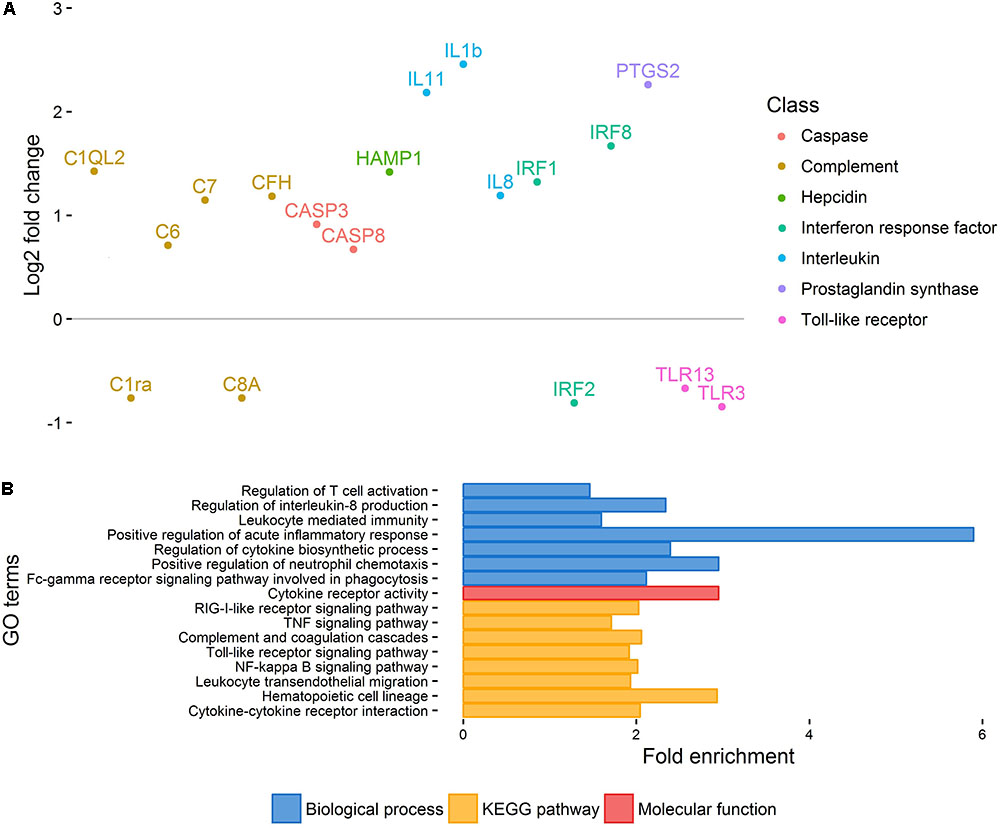
FIGURE 2. Healthy vs. injured skin. (A) Important immune-related genes showing differential expression between healthy and injured skin. Genes have been arbitrarily positioned along the x-axis. (B) Selection of GO terms enriched amongst DE genes between healthy and injured skin.
In addition to the expected innate immune response observed above, cell division related processes were also clearly up-regulated at louse attachment sites, and well-characterized genes involved in tissue repair such as fibroblast growth factor-binding protein 1 and Epigen showed significant differences between lice attachment sites and healthy skin (FC > 3). Several genes related to the cell matrix and cell adhesion also had higher expression at attachment sites (i.e., cadherin-13, integrin alpha-2, desmoplakin, or various keratin and collagen genes). Cell proliferation is the main response to skin wounds in fish (Iger and Abraham, 1990), and these results are consistent with those previously found in the early response to L. salmonis (Skugor et al., 2008). Several mucins were also found to have higher expression at attachment sites, pointing toward increased mucus production and secretion, which can also be a typical response to wounding in fish (Fast, 2014).
Resistance
Resistance, measured as number of sea lice per fish, was evaluated using two different approaches: correlation between gene expression and sea lice loads, and differential expression between family-matched fish showing high and low sea lice loads.
Correlation Between Gene Expression and Sea Lice Loads
We studied the correlation of gene expression and sea lice counts in healthy skin and sea lice attachment sites. Genes showing r > |0.75| with sea lice counts were considered of interest (Supplementary File S3).
The expression levels of five immune receptors in healthy skin were positively correlated with sea lice loads (Table 1). Macrophage mannose receptor 1 (MRC1) shows the highest positive correlation with number of sea lice (r = 0.87), and also the highest expression difference between louse attachment vs. healthy skin (FC = 4.79). MRC1 is a c-type lectin receptor, expressed in macrophages, dendritic cells, and skin in humans. MRC1 plays a role both in innate and adaptive immunity and also acts as a recognition receptor for different pathogens such as bacteria, virus, or fungi (East and Isacke, 2002). C-type lectin receptor A (r = 0.81; FC = -1.34) is another lectin receptor involved in antigen recognition and immune response (Geiktenbeek and Gringhuis, 2009). Lectins such as MRC1 and CLEC4E have been found to be induced by glucosinolate-enriched feeds in Atlantic salmon, which also reduced lice counts between 17 and 25% (Holm et al., 2016), and are also up-regulated in response to sea lice in the more resistant pink salmon species (Sutherland et al., 2014). Lectins have been reported to activate the immune system in response to parasites in several different species (Vázquez-Mendoza et al., 2013; Hoving et al., 2014), therefore modulation of these genes represents a possible route to enhance Atlantic immune responses to sea lice. Two immune receptors were negatively correlated with number of sea lice, CD97 (r = -0.84) and suppressor of cytokine signaling 5 (SOCS5; r = -0.76). CD97 regulates cytokine production and T-cell activation and proliferation (Capasso et al., 2006; Abbott et al., 2007); while SOCS5 is part of the cytokine-mediated signaling pathway, and acts as a negative regulator of inflammatory response and other immune-related pathways (Seki et al., 2002). Since, it was not possible to take skin samples prior to infection, it is difficult to distinguish between cause and effect; i.e., it is plausible that the negative correlation of these genes with number of sea lice is simply indicating that the immune system of the host responds proportionally to the degree of lice infestation. Nonetheless, the data support a major role for these genes in the host response to sea lice, and the differences in lice count between fish has a genetic component, to which these genes may contribute.
Amongst genes without a (well-known) immune function, there was an association between SUMO1 (r = 0.76) and SUMO3 (r = -0.91) expression and sea lice loads. Small ubiquitin-like modifier (SUMO) proteins are small proteins similar to ubiquitins that are covalently attached to other proteins to modify their function. According to the gene expression data, SUMO1 seems to be preferred over SUMO3 in salmon upon sea lice infestation. Although post-translational modifications have been barely explored in fish, in mice SUMOylation has been shown to be involved in modulation of host innate immune response to pathogens (Decque et al., 2016). SUMOylation is also a very active field of research in plants, where SUMO is known to be involved in many important processes such as plant response to environmental stresses, including pathogens (Park et al., 2011). It would be interesting to further study the role of SUMO in modulating Atlantic salmon responses to sea lice.
The results were markedly different in lice-attachment sites (Supplementary File S3), and congruent with differential expression between lice-attachment and healthy skin, with inflammatory genes such as toll-like receptor 12 or caspase 3 showing high correlations with sea lice loads. Similarly, one of the sox9 paralogs (sox9a) was also highly correlated with lice loads. Sox9 has a pro-proliferation function in human epidermal keratinocytes (Shi et al., 2013), and therefore this transcription factor is probably promoting wound healing in sea lice attachment sites. Finally, the gene hepcidin-1 is also correlated with sea lice counts in lice-attachment sites. Hepcidin is a regulator of iron metabolism, which as mentioned in the introduction has been associated with response to C. rogercresseyi (Valenzuela-Muñoz et al., 2017).
Differential Expression Between High and Low Sea Lice Loads
The samples for RNA sequencing were chosen to enable 8 vs. 8 comparison between family-matched fish (three families with two fish per group, two families with one fish) with high and low values for resistance (26.2 ± 5.5 vs. 54.9 ± 13.5 sea lice per fish). There were 43 genes significantly differentially expressed between resistant and susceptible fish (Supplementary File S4). All but one were from comparison of healthy skin samples between the two groups, which seems to suggest that the differences in resistance are systemic rather than local to louse attachment sites. The susceptible group had higher expression levels for genes involved in muscle contraction like troponins and myosins, which was also highlighted by GO enrichment analyses (Figure 3). Myosins and troponins have previously been identified as genes that respond to sea lice attachment in salmon skin (Holm et al., 2015). Further, Caligus infection is known to induce increased enzyme activity in muscle tissue (Vargas-Chacoff et al., 2017), and behavioral changes in the fish such as flashing and jumping are associated with ectoparasite removal (Furevik et al., 1993; Magnhagen et al., 2008). It has been recently reported that inactivity or reduced swimming activity contribute to resistance to sea lice (Bui, 2017), so it is possible that the high lice counts of susceptible fish in this study are due to higher activity levels with associated expression of muscle contraction related genes. In turn, high lice burden can provoke behavioral responses increasing fish activity, which results in the up-regulation of muscle genes, increasing the expression differences between resistant-passive-low lice fish and susceptible-active-high lice fish.
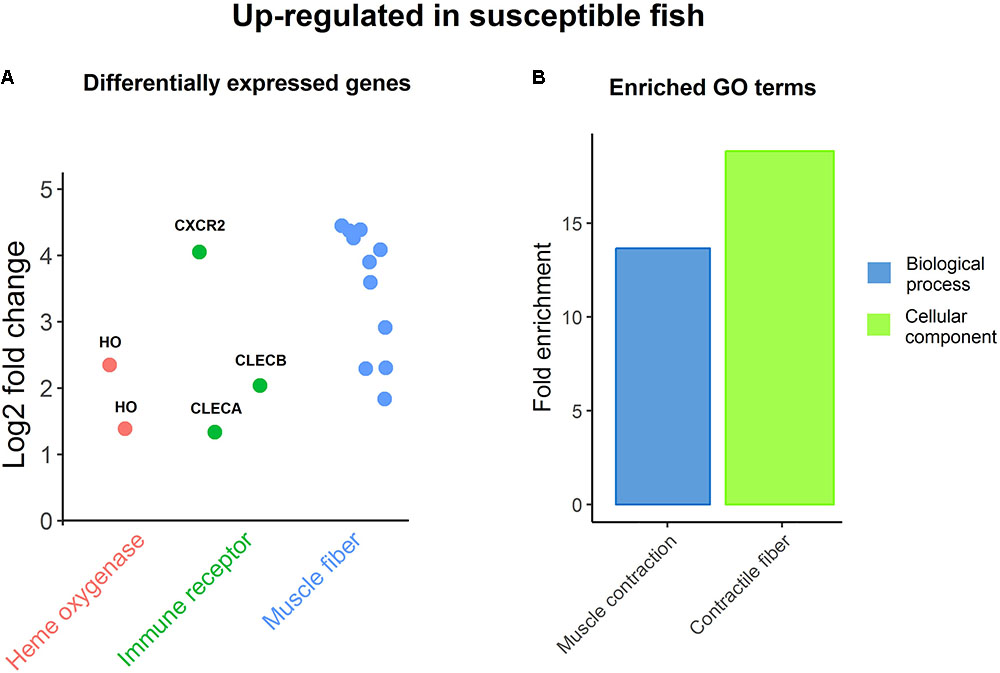
FIGURE 3. Up-regulation in susceptible fish. (A) Genes DE between resistant and susceptible fish, being up-regulated in the latter. (B) Enriched GO terms amongst DE genes up-regulated in susceptible fish.
Two heme oxygenase genes, encoding enzymes, which catalyze the degradation of heme, also had higher expression levels in susceptible samples (Figure 3), which is consistent with the positive correlation with lice loads of the iron-sequestration gene hepcidin. These genes have been previously shown to be up-regulated in response to Caligus infection (Valenzuela-Muñoz and Gallardo-Escárate, 2017). Importantly, iron availability was found to be reduced in the highly resistant species pink salmon infected with L. Salmonis (Sutherland et al., 2014), and hematocrit and anemia were also found to be reduced in chum salmon (Oncorhynchus keta) in response to sea lice (Jones et al., 2007). It is therefore plausible that the more effective reduction of iron availability in Atlantic salmon (perhaps behaving more similarly to the resistant pink salmon) might be related to increased resistance to sea lice.
Finally, three immune receptors showed higher expression in susceptible samples (Figure 3); C-X-C chemokine receptor type 2 is a receptor for IL-8, its binding causes activation of neutrophils; while C type lectin receptors A (also found to be positively correlated with sea lice counts in healthy skin) and B are leptin receptors with an important role in pathogen recognition and immunity (Geiktenbeek and Gringhuis, 2009), as previously discussed. While it is clear that resistance and host response to sea lice is multifactorial in nature, these genes related to muscle contraction, iron availability and immunity may be targets for functional validation in future studies, and for cross-referencing with genome-wide association analyses to identify candidate causative genes and variants.
Growth During Infestation
Differences in weight gain percentage from the start to the end of the trial were also investigated. Weight gain during infestation did not show any significant correlation with initial weight (r = -0.27, p = 0.10), sea lice counts (r = 0.12, p = 0.45) or sea lice density (r = 0.19, p = 0.24) in our dataset, and the means for these three traits are not significantly different between our groups showing differential growth during infestation (t-test p-values > 0.35). Family-matched fish (8 vs. 8; three families with two fish per group, two families with one fish) with differential weight gain during infestation (7.0 ± 4.3 vs. 28.8 ± 12.3 weight gain percentage) were compared. A total of 24 and 1 genes were found differentially expressed between fish showing high and low weight gains in healthy and sea louse attachment site samples, respectively (Supplementary File S5). The gene differentially expressed in injured skin, solute carrier family 15 member 1 (SLC15A1), also showed the lowest p-value and highest FC in healthy skin (FC = 3.38, p = 0.003). The SLC15A1 protein is a membrane transporter that mediates the uptake of dipeptides and tripeptides, in humans this gene is expressed in the intestinal epithelium and plays a major role in protein absorption (Adibi, 1997). Another interesting DE gene is myogenic regulatory factor 6 (MYF6; FC = 0.72, p = 0.04). Myogenic regulatory factors are transcription factors that regulate muscle development (Perry and Rudnick, 2000); in Senegalese sole decreased expression of these factors was observed in fast muscle when fed with a high-lipid content diet, which caused reduced growth (Campos et al., 2010). While skin is unlikely to be a highly suitable tissue to study genes underlying fish growth during sea lice infestation, both myogenic factors and increased nutrient absorption, and specifically MYF6 and SLC15A1, are good candidates to better understand growth impairment differences under sea lice infestation.
Conclusion
The results of this study highlight that the early gene expression response of Atlantic salmon to sea lice involves up-regulation of many different components of the immune system (inflammatory response, cytokine production, TNF and NF-kappa B signaling and complement activation) along with tissue repair activation. The comparison of resistant vs. susceptible animals highlighted enrichment of pathways related to fish activity, iron availability and receptors modulating pathogen recognition and immune response. Overall, this study contributes to an improved understanding of Atlantic salmon early response to sea lice in skin, and into the gene expression profiles underpinning genetic resistance to sea lice in salmon. The identified pathways and genes may be targets for future studies aimed at development of new treatments, vaccines, or prevention strategies. The data can also be cross-referenced with high power genome-wide association studies to help prioritize putative causative genes and variants that have potential to improve genomic selection programs for genetic improvement of resistance to this industry’s most serious disease.
Data Availability
The raw reads generated for this study have been deposited in NCBI’s Sequence Read Archive (SRA) under Accession No. SRP100978.
Ethics Statement
The lice challenge experiments were performed under local and national regulatory systems and were approved by the Animal Bioethics Committee (ABC) of the Faculty of Veterinary and Animal Sciences of the University of Chile (Santiago, Chile), Certificate No. 01-2016, which based its decision on the Council for International Organizations of Medical Sciences (CIOMS) standards, in accordance with the Chilean standard NCh-324-2011.
Author Contributions
RH, JY, and DR were responsible for the concept and design of this work and drafted the manuscript. AB managed the collection of the samples. AG performed the molecular biology experiments. DR performed bioinformatic and statistical analyses. All authors read and approved the final manuscript.
Funding
This work was supported by an RCUK-CONICYT (Grant No. BB/N024044/1), Institute Strategic Funding Grants to The Roslin Institute (Grant Nos. BBS/E/D/20002172, BBS/E/D/30002275, and BBS/E/D/10002070), Edinburgh Genomics was partly supported through core grants from NERC (Grant No. R8/H10/56), MRC (Grant No. MR/K001744/1), and BBSRC (Grant No. BB/J004243/1). DR was supported by a Newton International Fellowship of the Royal Society (Grant No. NF160037).
Conflict of Interest Statement
JY was supported by Aquainnovo S.A.
The remaining authors declare that the research was conducted in the absence of any commercial or financial relationships that could be construed as a potential conflict of interest.
Acknowledgments
The authors would like to thank the contribution of Aquainnovo and Salmones Chaicas for providing the biological material and phenotypic records of the experimental challenges.
Supplementary Material
The Supplementary Material for this article can be found online at: https://www.frontiersin.org/articles/10.3389/fgene.2018.00287/full#supplementary-material
Footnotes
- ^http://www.bioinformatics.babraham.ac.uk/projects/fastqc/
- ^http://www.sthda.com/english/rpkgs/factoextra/
References
Aaen, S. M., Helgesen, K. O., Bakke, M. K., Kaur, K., and Horsberg, T. E. (2015). Drug resistance in sea lice: a threat to salmonid aquaculture. Trends Parasitol. 31, 72–81. doi: 10.1016/j.pt.2014.12.006
Abbott, R. J., Spendlove, I., Roversi, P., Fitzgibbon, H., Knott, V., Teriete, P., et al. (2007). Structural and functional characterization of a novel T cell receptor co-regulatory protein complex. CD97-CD55. J. Biol. Chem. 282, 22023–22032. doi: 10.1074/jbc.M702588200
Adibi, S. A. (1997). The oligopeptide transporter (Pept-1) in human intestine: biology and function. Gastroenterology 113, 332–340. doi: 10.1016/S0016-5085(97)70112-4
Bairoch, A., Boeckmann, B., Ferro, S., and Gasteiger, E. (2004). Swiss-Prot: juggling between evolution and stability. Brief. Bioinform. 5, 39–55. doi: 10.1093/bib/5.1.39
Bolger, A. M., Lohse, M., and Usadel, B. (2004). Trimmomatic: a flexible trimmer for Illumina sequence data. Bioinformatics 30, 2114–2120. doi: 10.1093/bioinformatics/btu170
Braden, L. M., Barker, D. E., Koop, B. F., and Jones, S. R. M. (2012). Comparative defense-associated responses in salmon skin elicited by the ectoparasite Lepeophtheirus salmonis. Comp. Biochem. Physiol. Part D Genomics Proteomics 7, 100–109. doi: 10.1016/j.cbd.2011.12.002
Braden, L. M., Koop, B. F., and Jones, S. R. M. (2015). Signatures of resistance to Lepeophtheirus salmonis include a TH2-type response at the louse-salmon interface. Dev. Comp. Immunol. 48, 178–191. doi: 10.1016/j.dci.2014.09.015
Bui, S. (2017). The Behavioural Resistance and Response of Atlantic Salmon to the Ectoparasite Lepeophtheirus salmonis. Ph.D. thesis, University of Melbourne, Parkville VIC.
Campos, C., Valente, L. M. P., Borges, P., Bizuayehu, T., and Fernandes, J. M. O. (2010). Dietary lipid levels have a remarkable impact on the expression of growth-related genes in Senegalese sole (Solea senegalensis Kaup). J. Exp. Biol. 213, 200–209. doi: 10.1242/jeb.033126
Capasso, M., Durrant, L. G., Stacey, M., Gordon, S., Ramage, J., and Spendlove, I. (2006). Costimulation via CD55 on human CD4+ T cells mediated by CD97. J. Immunol. 177, 1070–1077. doi: 10.4049/jimmunol.177.2.1070
Conesa, A., Götz, S., García-Gómez, J. M., Terol, J., Talón, M., and Robles, M. (2005). Blast2GO: a universal tool for annotation, visualization and analysis in functional genomics research. Bioinformatics 21, 3674–3676. doi: 10.1093/bioinformatics/bti610
Correa, K., Bangera, R., Figueroa, R., Lhorente, J. P., and Yáñez, J. M. (2017). The use of genomic information increases the accuracy of breeding value predictions for sea louse (Caligus rogercresseyi) resistance in Atlantic salmon (Salmo salar). Genet. Sel. Evol. 49:15. doi: 10.1186/s12711-017-0291-8
Correa, K., Lhorente, J. P., Bassini, L., López, M. E., Di Genova, A., Maass, A., et al. (2016). Genome wide association study for resistance to Caligus rogercresseyi in Atlantic salmon (Salmo salar L.) using a 50K SNP genotyping array. Aquaculture 472, 61–65. doi: 10.1016/j.aquaculture.2016.04.008
Costello, M. J. (2009). The global economic cost of sea lice to the salmonid farming industry. J. Fish Dis. 32, 115–118. doi: 10.1111/j.1365-2761.2008.01011.x
Decque, A., Joffre, O., Magalhaes, J. G., Cossec, J. C., Blecher-Gonen, R., Lapaguette, P., et al. (2016). Sumoylation coordinates the repression of inflammatory and anti-viral gene-expression programs during innate sensing. Nat. Immunol. 17, 140–149. doi: 10.1038/ni.3342
Dobin, A., Davis, C. A., Schlesinger, F., Drenkow, J., Zaleski, C., Jha, S., et al. (2013). STAR: ultrafast universal RNA-seq aligner. Bioinformatics 29, 15–21. doi: 10.1093/bioinformatics/bts635
East, L., and Isacke, C. M. (2002). The mannose receptor family. Biochim. Biophys. Acta 1572, 364–386. doi: 10.1016/S0304-4165(02)00319-7
Edvardsen, R. B., Leininger, S., Kleppe, L., Skaftnesmo, K. O., and Wargelius, A. (2014). Targeted mutagenesis in Atlantic salmon (Salmo salar L.) using the CRISPR/Cas9 system induces complete knockout individuals in the F0 generation. PLoS One 9:e108622. doi: 10.1371/journal.pone.0108622
Fast, M. D. (2014). Fish immune responses to parasitic copepod (namely sea lice) infection. Dev. Comp. Immunol. 43, 300–312. doi: 10.1016/j.dci.2013.08.019
Fast, M. D., Johnson, S. C., and Jones, S. R. M. (2007). Differential susceptibility and the response of pink (Oncorhynchus gorbuscha) and chum (O. keta) salmon juveniles to infection with Lepeophtheirus salmonis. Dis. Aquat. Organ. 75, 229–238.
Fast, M. D., Ross, N. W., Mustafa, A., Sims, D. E., Johnson, S. C., Conboy, G. A., et al. (2002). Susceptibility of rainbow trout Oncorhynchus mykiss, Atlantic salmon Salmo salar and coho salmon Oncorhynchus kisutch to experimental infection with sea lice Lepeophtheirus salmonis. Dis. Aquat. Org. 52, 57–68. doi: 10.3354/dao052057
Food and Agriculture Organization of the United Nations (2016). The State of World Fisheries and Aquaculture 2016. Rome: Contributing to food security and nutrition for all.
Food and Agriculture Organization of the United Nations (2017). GLOBEFISH Highlights – Special Issue 2017. Rome: Food and Agriculture Organization of the United Nations, 24. doi: 10.1098/rspb.2011.2210
Frazer, L. N., Morton, A., and Krkosek, M. (2012). Critical thresholds in sea lice epidemics: evidence, sensitivity and subcritical estimation. Proc. Biol. Sci. 279, 1950–1958. doi: 10.1098/rspb.2011.2210
Furevik, D. M., Bjordal, A., Huse, I., and Fernö, A. (1993). Surface activity of Atlantic salmon (Salmo salar L.) in net pens. Aquaculture 110, 119–128. doi: 10.1038/nri2569
Geiktenbeek, T. B., and Gringhuis, S. I. (2009). Signalling through C-type lectin receptors: shaping immune responses. Nat. Rev. Immunol. 9, 465–479. doi: 10.1038/nri2569
Gharbi, K., Matthews, L., Bron, J., Roberts, R., Tinch, A., and Stear, M. (2015). The control of sea lice in Atlantic salmon by selective breeding. J. R. Soc. Interface 12:0574. doi: 10.1098/rsif.2015.0574
Holm, H., Santi, N., Kjøglum, S., Perisic, N., Skugor, S., and Evensen, Ø. (2015). Difference in skin immune responses to infection with salmon louse (Lepeophtheirus salmonis) in Atlantic salmon (Salmo salar L.) of families selected for resistance and susceptibility. Fish Shellfish Immunol. 42, 384–394. doi: 10.1016/j.fsi.2014.10.038
Holm, H. J., Wadsworth, S., Bjelland, A. K., Krasnov, A., Evensen,Ø., and Skugor, S. (2016). Dietary phytochemicals modulate skin gene expression profiles and result in reduced lice counts after experimental infection in Atlantic salmon. Parasit. Vectors 9:271. doi: 10.1186/s13071-016-1537-y
Hoving, J. C., Wilson, G. J., and Brown, G. D. (2014). Signalling C-type lectin receptors, microbial recognition and immunity. Cell Microbiol. 16, 185–194. doi: 10.1111/cmi.12249
Iger, Y., and Abraham, M. (1990). The process of skin healing in experimentally wounded carp. J. Fish Biol. 36, 421–437. doi: 10.1016/j.aquaculture.2013.12.033
Imsland, A., Reynolds, P., Eliassen, G., Hangstad, T. A., Foss, A., Vikingstad, E., et al. (2014). The use of lumpfish (Cyclopterus lumpus L.) to control sea lice (Lepeophtheirus salmonis Krøyer) infestations in intensively farmed Atlantic salmon (Salmo salar). Aquaculture 42, 19–23. doi: 10.1186/s13071-016-1537-y
Jodaa, H. H., Wadsworth, S., Bjelland, A. K., Krasnov, A., Evensen,Ø., and Skugor, S. (2016). Dietary phytochemicals modulate skin gene expression profiles and result in reduced lice counts after experimental infection in Atlantic salmon. Parasit. Vectors 9:271. doi: 10.1186/s13071-016-1537-y
Johnson, S. C., and Albright, L. J. (1992). Comparative susceptibility and histopathology of the response of naive Atlantic, Chinook and coho salmon to experimental infection with Lepeoptheirus salmonis (Copepoda, Caligidae). Dis. Aquat. Organ. 14, 179–193.
Johnson, S. C., Treasurer, J. W., Bravo, S., Nagasawa, K., and Kabata, Z. (2004). A review of the impact of parasitic copepods on marine aquaculture. Zool. Stud. 43, 229–243. doi: 10.3354/dao075229
Jones, S. R. M., Fast, M. D., Johnson, S. C., and Groman, D. B. (2007). Differential rejection of salmon lice by pink and chum salmon: disease consequences and expression of proinflammatory genes. Dis. Aquat. Org. 75, 229–238. doi: 10.1093/nar/28.1.27
Kanehisa, M., and Goto, S. (2000). KEGG: Kyoto encyclopedia of genes and genomes. Nucleic Acids Res. 28, 27–30. doi: 10.1146/annurev-marine-010814-015646
Lafferty, K. D., Harvell, C. D., Conrad, J. M., Friedman, C. S., Kent, M. L., Kuris, A. M., et al. (2015). Infectious diseases affect marine fisheries and aquaculture economics. Ann. Rev. Mar. Sci. 7, 471–496. doi: 10.1146/annurev-marine-010814-015646
Leclercq, E., Davie, A., and Migaud, H. (2014). Delousing efficiency of farmed ballan wrasse (Labrus bergylta) against Lepeophtheirus salmonis infecting Atlantic salmon (Salmo salar) post-smolts. Pest. Manag. Sci. 70, 1274–1282. doi: 10.1002/ps.3692
Lhorente, J. P., Gallardo, J. A., Villanueva, B., Carabaño, M. J., and Neira, R. (2014). Disease resistance in Atlantic salmon (Salmo salar): coinfection of the intracellular bacterial pathogen Piscirickettsia salmonis and the sea louse Caligus rogercresseyi. PLoS One 9:e95397. doi: 10.1371/journal.pone.0095397
Liao, Y., Smyth, G. K., and Shi, W. (2014). FeatureCounts: an efficient general purpose program for assigning sequence reads to genomic features. Bioinformatics 30, 923–930. doi: 10.1093/bioinformatics/btt656
Lien, S., Koop, B. F., Sandve, S. R., Miller, J. R., Kent, M. P., Nome, T., et al. (2016). The Atlantic salmon genome provides insights into rediploidization. Nature 533, 200–205. doi: 10.1038/nature17164
Love, M. I., Huber, W., and Anders, S. (2014). Moderated estimation of fold change and dispersion for RNA-seq data with DESeq2. Genome Biol. 15:550. doi: 10.1186/s12864-016-2443-6
MacLeod, I. M., Bowman, P. J., Vander Jaqt, C. J., Haile-Mariam, M., Kemper, K. E., Chamberlain, A. J., et al. (2016). Exploiting biological priors and sequence variants enhances QTL discovery and genomic prediction of complex traits. BMC Genomics 17:144. doi: 10.1186/s12864-016-2443-6
Macqueen, D. J., Primmer, C. R., Houston, R. D., Nowak, B. F., Bernatchez, L., Bergseth, S., et al. (2017). Functional annotation of all salmonid genomes (FAASG): an international initiative supporting future salmonid research, conservation and aquaculture. BMC Genomics 18:484.
Magnhagen, C., Braithwaite, V., Forsgren, E., and Kapoor, B. G. (2008). Fish Behaviour. Mount Washington, NH: Enfield Science Publishers.
Ødegård, J., Moen, T., Santi, N., Korsvoll, S. A., Kjøglum, S., and Meuwissen, T. H. E. (2014). Genomic prediction in an admixed population of Atlantic salmon (Salmo salar). Front. Genet. 5:402. doi: 10.3389/fgene.2014.00402
Palaiokostas, C., Ferraresso, S., Franch, R., Houston, R. D., and Bargelloni, L. (2016). Genomic prediction of resistance to pasteurellosis in gilthead sea bream (Sparus aurata) using 2b-RAD sequencing. G3 6, 3693–3700. doi: 10.1534/g3.116.035220
Park, H. J., Kim, W. Y., Park, H. C., Lee, S. Y., Bohnert, H. J., and Yun, D. J. (2011). SUMO and SUMOylation in plants. Mol. Cells 32, 305–316. doi: 10.1007/s10059-011-0122-7
Perry, R., and Rudnick, M. (2000). Molecular mechanisms regulating myogenic determination and differentiation. Front. Biosci. 5:D750–D767.
R Core Team (2014). R: A Language and Environment for Statistical Computing. Vienna: R Foundation for Statistical Computing.
Seki, Y., Hayashi, K., Matsumoto, A., Tsukada, J., Ransom, J., Naka, T., et al. (2002). Expression of the suppressor of cytokine signaling-5 (SOCS5) negatively regulates IL-4-dependent STAT6 activation and Th2 differentiation. Proc. Natl. Acad. Sci. U.S.A. 99, 13003–13008. doi: 10.1073/pnas.202477099
Shi, G., Sohn, K. C., Li, Z., Choi, D. K., Park, Y. M., Kim, J. H., et al. (2013). Expression and functional role of Sox9 in human epidermal keratinocytes. PLoS One 8:e54355. doi: 10.1371/journal.pone.0054355
Skugor, S., Glover, K. A., and Nilsen, F. (2008). Local and systemic gene expression responses of Atlantic salmon (Salmo salar L.) to infection with the salmon louse (Lepeophtheirus salmonis). BMC Genomics 9:498. doi: 10.1186/1471-2164-9-498
Sutherland, B. J. G., Covello, J. M., Friend, S. E., Poley, J. D., Koczka, K. W., Purcell, S. L., et al. (2017). Host–parasite transcriptomics during immunostimulant-enhanced rejection of salmon lice (Lepeophtheirus salmonis) by Atlantic salmon (Salmo salar). FACETS 2, 477–495. doi: 10.1139/facets-2017-0020
Sutherland, B. J. G., Koczka, K. W., Yasuike, M., Jantzen, S. G., Yazawa, R., Koop, B. F., et al. (2014). Comparative transcriptomics of Atlantic Salmo salar, chum Oncorhynchus keta and pink salmon O. gorbuscha during infections with salmon lice Lepeophtheirus salmonis. BMC Genomics 15:200. doi: 10.1186/1471-2164-15-200
Sutherland, B. J. G., Poley, J. D., Igboeli, O. O., Jantzen, J. R., Fast, M. D., Koop, B. F., et al. (2015). Transcriptomic responses to emamectin benzoate in Pacific and Atlantic Canada salmon lice Lepeophtheirus salmonis with differing levels of drug resistance. Evol. Appl. 8, 133–148. doi: 10.1111/eva.12237
Torrisen, O., Jones, S., Asche, F., Guttomsen, A., Skilbrei, O. T., Nilsen, F., et al. (2013). Salmon lice – impact on wild salmonids and salmon aquaculture. J. Fish Dis. 36, 171–194. doi: 10.1111/jfd.12061
Tsai, H. Y., Hamilton, A., Tinch, A. E., Guy, D. R., Bron, J. E., Taggart, J. B., et al. (2016). Genomic prediction of host resistance to sea lice in farmed Atlantic salmon populations. Genet. Sel. Evol. 48:47. doi: 10.1186/s12711-016-0226-9
Valenzuela-Muñoz, V., Boltaña, S., and Gallardo-Escárate, C. (2016). Comparative immunity of Salmo salar and Oncorhynchus kisutch during infestation with the sea louse Caligus rogercresseyi: an enrichment transcriptome analysis. Fish Shellfish Immunol. 59, 276–287. doi: 10.1016/j.fsi.2016.10.046
Valenzuela-Muñoz, V., Boltaña, S., and Gallardo-Escárate, C. (2017). Uncovering iron regulation with species-specific transcriptome patterns in Atlantic and coho salmon during a Caligus rogercresseyi infestation. J. Fish Dis. 40, 1169–1184. doi: 10.1111/jfd.12592
Valenzuela-Muñoz, V., and Gallardo-Escárate, C. (2017). Iron metabolism modulation in Atlantic salmon infested with the sea lice Lepeophtheirus salmonis and Caligus rogercresseyi: a matter of nutritional immunity? Fish Shellfish Immunol. 60, 97–102. doi: 10.1016/j.fsi.2016.11.045
Vargas-Chacoff, L., Muñoz, J. L. P., Hawes, C., Oyarzún, R., Pontigo, J. P., Saravia, J., et al. (2017). Ectoparasite Caligus rogercresseyi modifies the lactate response in Atlantic salmon (Salmo salar) and coho salmon (Oncorhynchus kisutch). Vet. Parasitol. 243, 6–11. doi: 10.1016/j.vetpar.2017.05.031
Vázquez-Mendoza, A., Carrero, J. C., and Rodriguez-Sosa, M. (2013). Parasitic infections: a role for C-type lectins receptors. Biomed. Res. Int. 2013:456352. doi: 10.1155/2013/456352
Wargelius, A., Leininger, S., Skaftnesmo, K. O., Kleppe, L., Andersson, E., Taranger, G. L., et al. (2016). Dnd knockout ablates germ cells and demonstrates germ cell independent sex differentiation in Atlantic salmon. Sci. Rep. 6:21284. doi: 10.1038/srep21284
Wu, J., Mao, X., Cai, T., Luo, J., and Wei, L. (2006). KOBAS server: a web-based platform for automated annotation and pathway identification. Nucleic Acids Res. 34, W720–W724. doi: 10.1093/nar/gkl167
Yáñez, J. M., Houston, R. D., and Newman, S. (2014a). Genetics and genomics of disease resistance in salmonid species. Front. Genet. 5:415. doi: 10.3389/fgene.2014.00415
Yáñez, J. M., Lhorente, J. P., Bassini, L. N., Oyarzún, M., Neira, R., and Newman, S. (2014b). Genetic co-variation between resistance against both Caligus rogercresseyi and Piscirickettsia salmonis, and body weight in Atlantic salmon (Salmo salar). Aquaculture 433, 295–298. doi: 10.1016/j.aquaculture.2014.06.026
Keywords: Caligus rogercresseyi, Salmo salar, aquaculture, disease, parasite, RNA-Seq, host–parasite, differential expression
Citation: Robledo D, Gutiérrez AP, Barría A, Yáñez JM and Houston RD (2018) Gene Expression Response to Sea Lice in Atlantic Salmon Skin: RNA Sequencing Comparison Between Resistant and Susceptible Animals. Front. Genet. 9:287. doi: 10.3389/fgene.2018.00287
Received: 14 May 2018; Accepted: 11 July 2018;
Published: 03 August 2018.
Edited by:
Mark S. Fife, Pirbright Institute (BBSRC), United KingdomReviewed by:
Filippo Biscarini, Consiglio Nazionale delle Ricerche (CNR), ItalyDirk-Jan De Koning, Swedish University of Agricultural Sciences, Sweden
Copyright © 2018 Robledo, Gutiérrez, Barría, Yáñez and Houston. This is an open-access article distributed under the terms of the Creative Commons Attribution License (CC BY). The use, distribution or reproduction in other forums is permitted, provided the original author(s) and the copyright owner(s) are credited and that the original publication in this journal is cited, in accordance with accepted academic practice. No use, distribution or reproduction is permitted which does not comply with these terms.
*Correspondence: Diego Robledo, RGllZ28uUm9ibGVkb0Byb3NsaW4uZWQuYWMudWs= Ross D. Houston, cm9zcy5ob3VzdG9uQHJvc2xpbi5lZC5hYy51aw==
†These authors have contributed equally to this work.