Corrigendum: Gene and Blood Analysis Reveal That Transfer from Brackish Water to Freshwater Is More Stressful to the Silverside Odontesthes humensis
- 1Laboratory of Structural Genomics, Technological Development Center, Federal University of Pelotas, Pelotas, Brazil
- 2Laboratory of Physiology, Institute of Biology, Federal University of Pelotas, Pelotas, Brazil
- 3Laboratory of Cancer Biotechnology, Technological Development Center, Federal University of Pelotas, Pelotas, Brazil
- 4Genomics and Molecular Evolution Laboratory, Department of Genetics, Institute of Biosciences of Botucatu, São Paulo State University, Botucatu, Brazil
- 5Laboratory of Vaccinology, Technological Development Center, Federal University of Pelotas, Pelotas, Brazil
Silversides are fish that inhabit marine coastal waters, coastal lagoons, and estuarine regions in southern South America. The freshwater (FW) silversides have the ability to tolerate salinity variations. Odontesthes humensis have similar habitats and biological characteristics of congeneric O. bonariensis, the most studied silverside species and with great economic importance. Studies revealed that O. bonariensis is not fully adapted to FW, despite inhabiting hyposmotic environments in nature. However, there is little information about stressful environments for cultivation of silverside O. humensis. Thus, the aim of this study was to evaluate the stress and osmoregulation responses triggered by the osmotic transfers on silverside O. humensis. Silversides were acclimated to FW (0 ppt) and to brackish water (BW, 10 ppt) and then they were exposed to opposite salinity treatment. Silverside gills and blood were sampled on pre-transfer (D0) and 1, 7, and 15 days (D1, D7, and D15) after changes in environmental salinity, the expression levels of genes atp1a3a, slc12a2b, kcnh1, and hspa1a were determined by quantitative reverse transcription-PCR for evaluation of osmoregulatory and stress responses. Furthermore, glycemia, hematocrit, and osmolality were also evaluated. The expression of atp1a3a was up- and down-regulated at D1 after the FW–BW and BW–FW transfers, respectively. Slc12a2b was up-regulated after FW–BW transfer. Similarly, kcnh1 and hspa1a were up-regulated at D1 after the BW–FW transfer. O. humensis blood osmolality decreased after the exposure to FW. It remained stable after exposure to BW, indicating an efficient hyposmoregulation. The glycemia had a peak at D1 after BW–FW transfer. No changes were observed in hematocrit. The return to the pre-transfer levels at D7 after the significant increases in responses of almost all evaluated molecular and blood parameters indicated that this period is enough for acclimation to the experimental conditions. In conclusion, our results suggest that BW–FW transfer is more stressful to O. humensis than FW–BW transfer and the physiology of O. humensis is only partially adapted to FW.
Introduction
Odontesthes spp., popularly known as pejerreyes or silversides, is a fish genus naturally endemic from South America waters (Bemvenuti, 2006). This genus comprises the biggest number of species of Atherinopsidae and the broader area of distribution, with species inhabiting marine coastal waters, coastal lagoons, and estuarine regions in southern South America (Dyer, 2006). Currently, some fishes from these species occupy FW environments, despite all Odontesthes spp. have a recent common marine origin (Heras and Roldán, 2011; Campanella et al., 2015). Thus, the FW silversides have an interesting ability to tolerate salinity variations. This euryhaline characteristic has drawn attention of researchers to its application in aquaculture in estuarine regions, where there are continuous alterations in water salinity (Piedras et al., 2009).
The most studied species of silverside, with economic importance and favorable projections for production, is Odontesthes bonariensis. In Argentina, it has an importance for flesh commercialization and sport fishing (Menone et al., 2000; Somoza et al., 2008). In Japan, O. bonariensis cultivation has been deployed, due to the efforts to farming conditions improvements in the last few years (Tsuzuki et al., 2000b, 2001, 2007). This species has also been introduced in Chile, Bolivia, and Italy (Dyer, 2000, 2006). The species is also exotic and has economic importance in Peru, where O. bonariensis was introduced in the Titicaca Lake in 1946 and until nowadays continues to be caught (Loubens and Osorio, 1991).
On the other hand, the congeneric Odontesthes humensis receives little attention from researchers and fish farmers. Only studies involving fry growth (Pouey et al., 2012), embryos salinity tolerance (Piedras et al., 2009), growth in intensive system (Tavares et al., 2014), and toxicology (Zebral et al., 2017) are developed in captivity specimens. Both O. bonariensis and O. humensis species have similar habitats, biological characteristics (including morphology), and are capable of interbreeding. Due to this, both species have similar potential for captivity cultivation (Dyer, 2006; Piedras et al., 2009). Main differences between both species are: O. bonariensis is the largest species in Atheriniforms and it has 32–38 gill rakers on lower branch, vomerine teeth present, and the prognathism can be present. Furthermore, this species is native to Brazil and Argentina, but not to Uruguay. On the other hand, O. humensis is smaller, has 13–19 gill rakers on lower branch, vomerine teeth is absent, prognathism is never present, and the species is native to Brazil, Uruguay, and Argentina (Dyer, 2006). Although the commercial production of O. humensis has not increased in recent years, this species has potential to be a bioindicator in toxicological studies (Zebral et al., 2017) due to its demands for water quality, surviving only in a narrow range of water parameters.
Thus, information about basic biology of O. humensis is necessary to the adequate maintenance of this silverside species in farm and laboratory conditions. Some studies revealed that the silverside O. bonariensis is not fully adapted to FW yet, despite inhabiting hyposmotic environments in nature (Tsuzuki et al., 2000b, 2001). However, there is no information about the less stressful environment, if with or without salt, to the cultivation of silverside O. humensis. We hypothesized that O. humensis shared, besides the habitats and reproductive physiology, the same pattern of response of O. bonariensis to environmental salinity. The corroboration of this hypothesis will help in maintenance of laboratorial or commercial cultivation, in addition to reinforcing the theory of invasion of FW habitats by silversides marine ancestors. Thus, the aim of this study was to evaluate, using cellular, biochemical, and molecular analysis, the stress and osmoregulation responses triggered by the osmotic transfers in silverside O. humensis.
Materials and Methods
Animals and Conditions
The silversides O. humensis used in this study came from eggs collected in nature (Arroio Grande, Brazil – 32°14′15′′S/53°05′13′′W) and hatched in tanks. The eggs were collected from wild breeders and incubated at laboratory conditions in FW (0) and pH values near 7.5, the same conditions applied to larviculture. The fish were 1.5 years old and have mean weight of 27.3 ± 9.5 g at experimental time. The silversides were maintained in 1,000 L cylindrical plastic tanks within an experimental room, fed three times a day with commercial feed (Supra, 38% crude protein) until satiety, under natural photoperiod of autumn (11 h light/13 h dark), with water pH 7.5 ± 0.3, and 19 ± 0.3°C for acclimation. The tank sides were opaque to reduce visual stress and incidence of luminosity through the top according to the daily variance. The acclimation period was 4 weeks for silversides in FW (salinity of 0 ppt) and in BW (salinity of 10 ppt), under the same previous conditions. The salinity levels were achieved through the dissolution of non-iodized sea salt in the water.
The fish continued to be fed three times a day during the experimental period. Once a week the water of the tanks was totally renewed with dissolved oxygen maintained in 7.8 ± 0.3 mg L-1; the total ammonia levels lower than 0.6 ± 0.2 mg L-1; temperature 20.01 ± 0.34°C; and pH 7.78 ± 0.03.
Experimental Design and Protocol
The experimental setup consisted in two treatments performed in quadruplicate, totaling eight tanks with 15 fish each. Four tanks contained silversides acclimated to FW and other four tanks contained silversides acclimated to BW. Both FW and BW groups were shortly transferred to opposite salt treatment. Fish were sampled at four different time points getting three fish per tank: a control before the osmotic transfer (D0) and on day 1 (D1), on day 7 (D7), and on day 15 (D15) after the transfer. Hence, 96 silverside fish were analyzed in different times of salt exposure.
Sampling
When captured, at all time points, the silversides were anesthetized with benzocaine (50 mg L-1). Blood samples were collected from caudal puncture using a heparinized syringe for hematocrit, glycemia, and osmolality evaluation. While anesthetized, the fish were euthanized by medullary section and brain excision to perform the post-mortem gills collection. Gill samples from three fish of each tank, at the four time points, were collected using sterilized material. Left second branchial arches were collected and immediately stored in liquid nitrogen (N2) until posterior RNA preparation. All experimental procedures were previously approved by the Ethics Committee on Animal Experimentation of Federal University of Pelotas (Process no. 23110.007018/2015-85).
Analyzed Genes
The Na+/K+-ATPase (NKA) enzyme is responsible to active transports three Na+ out of and two K+ into animal cells. In fishes, NKA is directly involved in the osmoregulation process (Hiroi and McCormick, 2012). Among NKAα1∼α4, we selected NKAα3 (encoded by atp1a3) which has similar responses (Stefansson et al., 2007; Whitehead et al., 2012; Armesto et al., 2014; Li et al., 2014; Taugbøl et al., 2014) and is expressed in similar tissues (Guynn et al., 2002) as NKAα1. NKAα1 is the most studied isoform of NKA (Havird et al., 2013), however, its mRNA expression can be influenced by the stress (Kiilerich et al., 2011) and this could disrupt the interpretation of results.
The Na+/K+/2Cl- cotransporter 1 (NKCC1) mediates the intake of one Na+, one K+, and two Cl- ions into the ionocytes down the electrochemical gradient generated by NKA (Hwang et al., 2011). In teleosts there are two isoforms of NKCC1 and NKCC2: NKCC1a/b and NKCC2a/b (Kakumura et al., 2015). NKCC1 (encoded by slc12a2) is more associated with ion secretion in teleost gills (Tipsmark et al., 2002; Nilsen et al., 2007), whereas NKCC2 mRNA is not present in large amount in fish gills (Hiroi and McCormick, 2012).
The voltage-gated potassium channels (KCNH) are gated by changes in membrane potential and play an important role in the repolarization of cells (Morais-Cabral and Robertson, 2015). KCNH1 has already been correlated to myoblast fusion (Occhiodoro et al., 1998), to embryonic development (Stengel et al., 2012), and has been used as target to cancer researches (Pardo and Stühmer, 2013) but the precise role of KCNH1 in normal tissues is poorly known. The kcnh1 gene has two copies in fishes, kcnh1a/b that are expressed differently in the organism (Stengel et al., 2012). KCNH4 has already been proposed as an osmoregulation marker (Taugbøl et al., 2014).
The heat shock 70kDa proteins (HSP70) are mainly responsible to protein folding, degradation of misfolded proteins, membrane translocation, and activation of immune system. HSP70 are expressed under normal conditions but also in response to stress conditions (Morimoto, 1998; Yamashita et al., 2010; McCallister et al., 2016). The genes encoding HSP70 in fishes are hspa1a and hspa1b (He et al., 2013). Thus, both hspa1 genes and the HSP70 protein are considered stress indicators in fish (Basu et al., 2001; Larsen et al., 2012; Taugbøl et al., 2014).
Molecular Cloning and Sequencing
Total RNA was isolated from gill samples of each fish separately, using RNeasy® Mini Kit (Qiagen, United States) according to the manufacturer’s protocol. DNase treatment of RNA samples was conducted with a DNA-Free® Kit (Ambion, United States) following the manufacturer’s protocol. The RNA concentration and quality were verified by spectrophotometry using NanoVueTM Plus (GE Healthcare Life Sciences, United states), and only samples with absorption A260/A280 ratio ≥2.0 were used in reverse transcription (RT) reactions.
First-strand cDNA was performed with 2 μg of RNA using random primers and SuperScript® III Reverse Transcriptase (Invitrogen, United States) according to the manufacturer’s protocol. Primer sets (Table 1) were designed to clone partial cDNA sequences of atp1a3, slc12a2, kcnh1, and hspa1 genes from gills of silverside fish based on the alignment of sequences of other fish species. The PCR parameters were: an initial denaturation for 1 min at 94°C, followed by 35 cycles of 94°C for 30 s; 57°C (for atp1a3); 62°C (for slc12a2); 64.6°C (for kcnh1); and 61°C (for hspa1) for 30 s; and 72°C for 1 min, with a final extension of 5 min at 72°C. PCR products were inserted into pCRTM4-TOPO® TA cloning vector and transformed in the electrocompetent Escherichia coli strain DH5α. These procedures were performed according to the manufacturer specifications (Invitrogen, United States). The positive clones were sequenced using a Big Dye® V3.1 Terminator Kit and M13 Primers (Applied Biosystems, United States). The cloned fragments were sequenced using an Applied Biosystems 3500 Genetic Analyzer® automatic sequencer (Life Technologies, United States).
In Silico and Phylogenetic Analysis
The identity of all sequenced cDNA was confirmed by Blast tool of the NCBI database1. The translation of sequenced nucleotides to amino acid sequences as well as the open-reading frame (ORF) identification was made using ExPASy bioinformatics resource portal2. Conserved domains and sites were mapped from UniProt database3. Amino acid sequences of NKA, NKCC, KCNH, and HSP70 from various vertebrate species were obtained from GenBank and aligned to the new deduced amino acid sequences of O. humensis using ClustalW. Phylogenetic analyses were carried out using MEGA version 6 (Tamura et al., 2013). Phylogenetic trees were constructed based on alignments of amino acid sequences using Neighbor-Joining method. Bootstrap analyses were done with 10,000 replications to test the tree reliability. The outgroups were Caenorhabditis elegans (for NKA and HSP70 analysis), Strongylocentrotus purpuratus (for NKCC analysis), and Toxocara canis (for KCNH analysis).
Evaluation of Gene Expression after Salinity Changes
Quantitative RT-PCR (qRT-PCR) was run on CFX96TM Real-Time PCR Detection System (Bio-Rad Laboratories, United States) using SYBR® Green PCR Master Mix (Applied Biosystems, United States). Primers (Table 1) for the identified genes atp1a3, slc12a2, kcnh1, and hspa1 and for the used reference genes β-actin (actb, GenBank Accession No. EF044319) and histone h3a (h3a, GenBank Accession no. KX060037) were designed using Primer3 online software4. Initial validation experiments were conducted to ensure that all primer pairs had equivalent PCR efficiencies calculated by standard dilution curve. Amplification was carried out at the standard cycling conditions of 95°C for 10 min, followed by 40 cycles at 95°C for 15 s, 60°C for 60 s followed by conditions to calculate the melting curve. All PCR runs for each cDNA sample were performed in triplicate. The qRT-PCR data were analyzed using the 2-ΔΔCt method considering primer amplification efficiencies (Livak and Schmittgen, 2001).
Blood Analysis
After blood collection, with fasted animals, the glycemia was measured using Accu Chek Glucometer (Roche Diagnostics, United Kingdom). The samples from each fish were immediately transferred to microcapillaries and centrifuged at 12,000 ×g for 5 min to hematocrit analysis. The blood was transferred to sterilized microtubes and centrifuged at 1,500 ×g at 4°C for 10 min to plasma separation for osmolality measurements in Vapro 5520® Vapor Pressure Osmometer (Wescor, United States).
Statistical Analysis
Quantitative data were expressed as means ± standard error of mean. Significant differences among means were evaluated by two-way ANOVA followed by Tukey’s test, setting the significance level at 95% (P < 0.05). The exception was the expression of reference genes, which was expressed as means of cycle threshold (Ct) values ± standard deviation of mean and the differences were analyzed by one-way ANOVA followed by Tukey’s test.
Results
cDNA Cloning and Characterization
The atp1a3, slc12a2, kcnh1, and hspa1 cloned fragments from O. humensis were, respectively, 975, 423, 560, and 286 bp (base pairs) in length. They were sequenced and deposited under GenBank accession numbers KR920364, KT001464, KX035016, and KU639716, respectively. The cloned fragments of atp1a3 and kcnh1 belong, respectively, to the middle of the ORF +2 and to the initial part of the ORF +3. The fragments of slc12a2 and hspa1 are part of the center of ORF +1. The atp1a3 fragment codes to 324 amino acids belonging to the P-type ATPase family. The slc12a2 codes to 141 amino acid residues belonging to the Na/K/Cl co-transporter 1 family. The cloned fragment of kcnh1 codes to 186 amino acids belonging to the potassium channel, voltage-dependent family. The cloned fragment of hspa1 codes to 95 amino acids belonging to the heat shock protein 70 family.
The percentage identity values between NKAα3, NKCC1, KCNH, and HSP70 putative sequences of silverside and of the other analyzed species were, respectively, 77–98, 55–92, 26–99, and 37–97% (Supplementary Figures S1–S4). The phylogenetic tree constructed based on alignment of NKAα-subunits amino acid sequences (Supplementary Figure S1) reveals that α1, α2, α3, and α4 form different clusters with mammal and fish sequences in each, except in α4 group, which was devoid of NKA sequences of fishes. Furthermore, the silverside NKAα3 was grouped in the monophyletic α3 cluster, showing that it was more closely related to NKAα3a than NKAα3b and NKAα2. Based on these evidences, the sequence found in the gills of O. humensis was identified as atp1a3α. NKCC1 and NKCC2 were grouped in different clusters (Supplementary Figure S2).
The paralogous NKCC1a and NKCC1b were grouped together in NKCC1 group. Furthermore, the tree has shown more evidence that silverside NKCC is closer to fish NKCC1b than to NKCC1a or NKCC2. Thus, the cloned and sequenced cDNA from silverside gills was identified as slc12a2b. The KCNH phylogenetic tree (Supplementary Figure S3) forms two monophyletic main groups: ELK family cluster, composed by vertebrates KCNH3, KCNH4, and KCNH8, and EAG family cluster, composed by vertebrates KCNH5 and KCNH1, in which the new sequence of silverside KCNH1 was grouped. Based on this analysis, the cloned KCNH sequence from silverside gills was confirmed as kcnh1.
The HSP70 phylogenetic analysis (Supplementary Figure S4) separated HSPA1 and HSPA2 sequences in different clusters. HSPA2 was composed only by sequences of mammals while HSPA1 was composed by mammals and teleosts. The new sequence of HSPA1 from silverside was clustered within fish HSPA1a. Based on these results, the HSPA sequence from silverside gills was identified as hspa1a.
Gene Expression after Salinity Changes
Primer sets for atp1a3a, slc12a2b, kcnh1, hspa1a, actb, and h3a had amplification efficiencies in qRT-PCR of 1.14, 1.07, 1.06, 1.02, 0.98, and 1.05, respectively. No difference was observed in the expression of reference genes between four different time points or between the treatments (Supplementary Table S1). The relative expression of atp1a3a (Figure 1A) was not different (P > 0.05) between FW- and BW-acclimated fishes in control D0. However, after the transfer of FW-acclimated fish to BW (FW–BW) and vice versa (BW–FW), a change in atp1a3a expression was observed. In FW–BW-transferred fish, there was an increase (P < 0.05) in atp1a3a expression, while in BW–FW there was a decrease at D1 (P < 0.05). The levels of atp1a3a expression returned close to the initial conditions in both FW–BW and BW–FW groups, without difference between groups (P > 0.05), at D7 and D15.
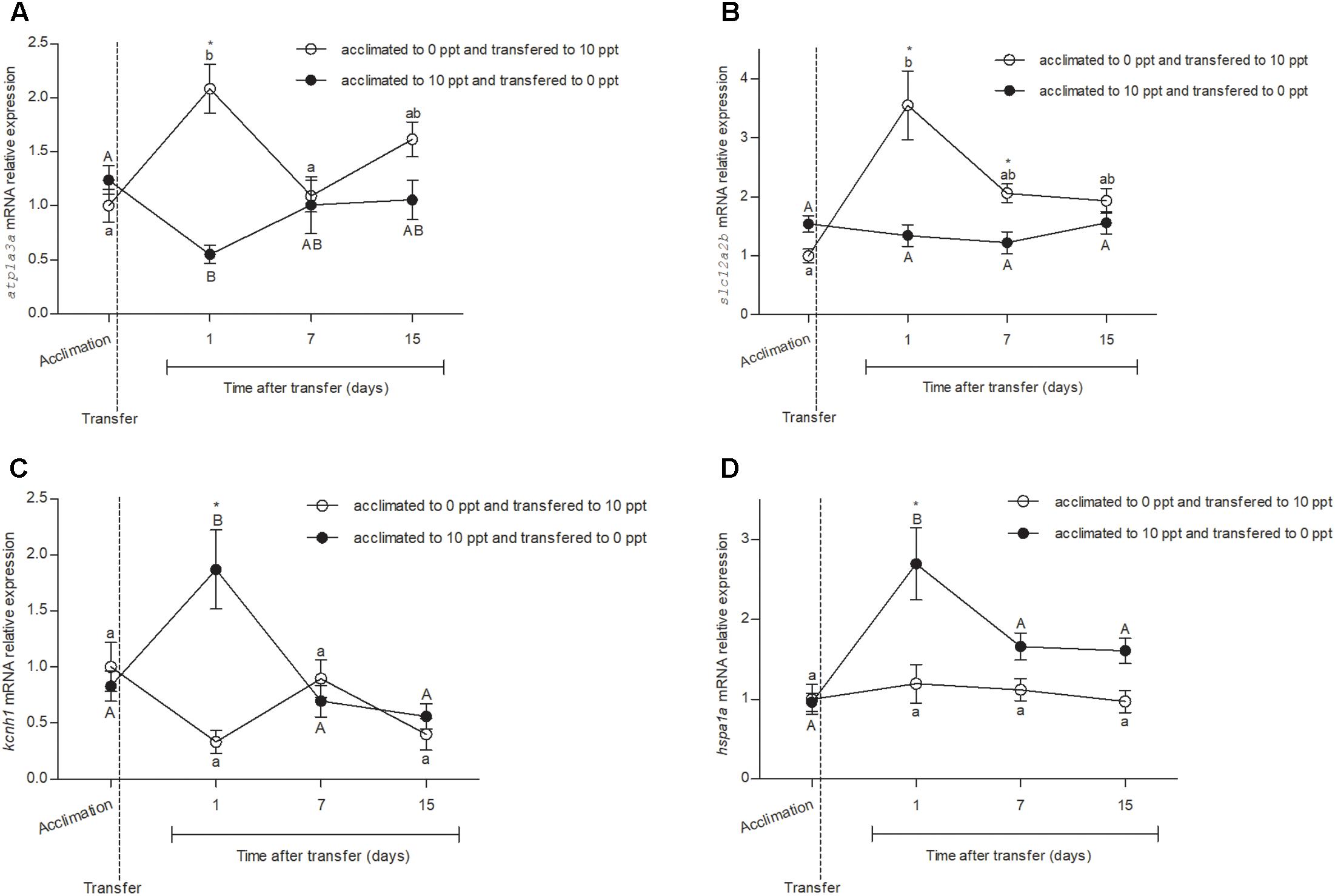
FIGURE 1. Relative expression of cloned genes atp1a3a, slc12a2b, kcnh1, and hspa1a from Odontesthes humensis submitted to salinity transfer. (A) Atp1a3a mRNA relative expression before and after the salinity change. (B) Slc12a2b mRNA relative expression before and after the salinity change. (C) Kcnh1 mRNA relative expression before and after the salinity change. (D) Hspa1a mRNA relative expression before and after the salinity change. Mean ± SEM (n = 8–10). Different letters in each group (lowercase to FW–BW transferred group and uppercase to BW–FW transferred group) represent significant difference between the measurements in a same group. The asterisk represents the significant difference between groups in a same experimental time. The vertical broken line indicates the transfer from BW to FW and from FW to BW moments.
The relative expression of slc12a2b (Figure 1B) was not different between FW- and BW-acclimated fish at D0 (P > 0.05). However, after the FW–BW and BW–FW transfers, a change in slc12a2b mRNA expression was observed. In FW–BW-transferred fish, there was an increase (P < 0.05) in slc12a2b mRNA levels at D1. The gene expression decreased (P < 0.05) to intermediary levels at D7 and remained without difference until D15 (P > 0.05). The slc12a2b expression levels did not change (P > 0.05) after the transfer in the BW–FW group in any experimental time. Difference in slc12a2b relative expression between the BW–FW and FW–BW groups (P < 0.05) was observed at D1 and D7.
None significant difference was observed between the relative expression of kcnh1 (Figure 1C) at D0 from FW- or BW-acclimated silversides (P > 0.05). After the transfers, at D1, it was observed a significant increase (P < 0.05) in the kcnh1 expression of the BW–FW group. The expression levels decreased (P < 0.05), returning to the initial patterns at D7 and D15. The kcnh1 expression levels did not change significantly (P > 0.05) after the transfer in the FW–BW group in any experimental time. Difference in kcnh1 relative expression between the BW–FW and FW–BW groups (P < 0.05) was observed only at D1.
The relative expression of hspa1a (Figure 1D) was not different between FW- and BW-acclimated fish at D0 (P > 0.05). After the transfers, at D1, it was observed a significant increase (P < 0.05) in the hspa1a relative expression of the BW–FW-transferred fish. The expression levels decreased (P < 0.05) to initial pattern at D7 and remained unchanged until D15 (P > 0.05). The hspa1a expression levels did not change (P > 0.05) after the transfer in the FW–BW group in any experimental time. Difference in hspa1a relative expression between the BW–FW and FW–BW groups (P < 0.05) was observed only at D1.
Glycemia
No difference in blood glucose concentration (P > 0.05) was observed between FW- or BW-acclimated fish at D0 (Figure 2A). The BW–FW-transferred fish presented an increase (P < 0.05) in glycemia values at D1, a decrease (P < 0.05) to pre-transfer levels at D7, and remained without difference (P > 0.05) until D15. None difference (P > 0.05) between time-points was observed in FW–BW transfer group. Significant difference between both groups was observed only at D1.
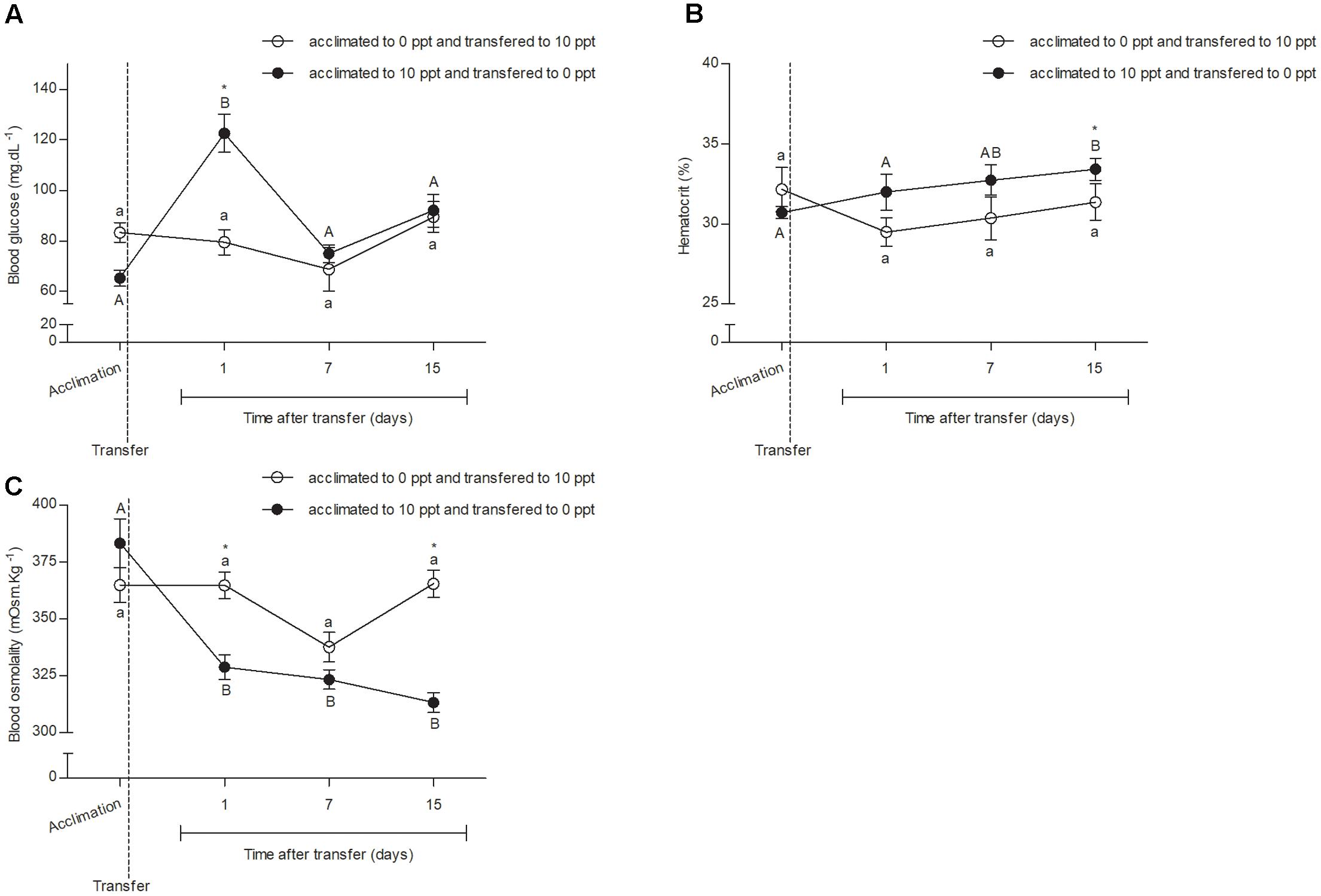
FIGURE 2. Blood responses from O. humensis submitted to salinity transfer. (A) Glycemia before and after the salinity change. (B) Hematocrit before and after the salinity change. (C) Blood osmolality before and after the salinity change. Mean ± SEM (n = 12). Different letters in each group (lowercase to FW–SW transferred group and uppercase to SW–FW transferred group) represent significant difference between the measurements in a same group. The asterisk represents the significant difference between groups in a same experimental time. The vertical broken line indicates the transfer from SW to FW and from FW to SW moments.
Hematocrit
No difference (P > 0.05) between the hematocrit (Figure 2B) in none group of analyzed silverside of experimental time was observed. Only trends of increase in hematocrit soon after BW–FW transfer and decrease after FW–BW were verified.
Osmolality
No difference (P > 0.05) between the blood osmotic concentration (Figure 2C) of FW- and BW-acclimated fishes was observed at D0. After transfer, there was a reduction (P < 0.05) in osmolality of BW–FW-transferred fish that was kept the same until D15. Differences were not observed in blood osmolality of FW–BW-transferred fish. Differences (P < 0.05) between groups were found right after the transfer (D1) and at the final measurements (D15).
Discussion
Salinity is a frequent abiotic stressor that restrains fish growth and development by inducing osmotic stress responses. When fishes are subjected to salinity stress, related genes are activated to induce salinity stress tolerance. This study was successful in the identification and characterization of atp1a3a, slc12a2b, kcnh1, and hspa1a mRNA sequences in the silverside O. humensis. The molecular cloning made possible to adequately monitor changes in the expression of these genes after salinity challenges. In addition, it was demonstrated that alterations in blood glucose and osmolality after environmental salinity change.
In FW–BW-transferred fish, atp1a3a expression increased soon upon salinity stress, whereas in BW–FW group it was downregulated. Similar effects in atp1a3a expression were also observed in gills of Mozambique tilapia challenged by salinity stress, which presented higher expression levels in SW and lower in FW (Li et al., 2014). In threespine stickleback, the SW transfer also generated an increase in atp1a3a mRNA expression (Taugbøl et al., 2014). The increase of mRNA expression and protein activity of NKAα-subunit in gills, in response to the SW transfer, occurred in Atlantic salmon (D’Cotta et al., 2000), in brown trout (Tipsmark et al., 2002), and in killifish (Scott and Schulte, 2005; Scott et al., 2008). Yet, the transfer to lower salinity generates a decrease in mRNA expression and protein activity of NKAα-subunit in gills of Mozambique tilapia (Lin et al., 2004). In the present study, the downregulation in atp1a3a expression indicates a reduction in osmoregulatory activity due the lack of salt in water.
In silverside, slc12a2b expression in gills and in response to increase of salinity was well detectable, evidencing the high importance of NKCC1b to hyposmoregulation of O. humensis. While NKA can have activity in ion absorption and secretion, NKCC1 is more associated with ion secretion in teleost gills. Here, the augmented water salt concentration leads O. humensis to increase its slc12a2b mRNA expression when FW–BW transferred. This fact, in association with the blood osmolality stabilization even when transferred to BW, indicates an efficient ion secretion activity. Furthermore, the transfer to lower salinity media did not affect the slc12a2b mRNA expression. Despite the opposite responses of NKCC in hypo- or hyperosmotic environment are the most common reported in fishes, none variations after salinity changes is also possible, especially in transfers for low salinities. In gills of brown trout (Tipsmark et al., 2002) and striped bass (Tipsmark et al., 2004), both gene and protein expression of NKCC1 were positively correlated to environmental salinity, with increases after FW–SW transfer and decreases after SW–FW transfer. In brackish medaka (Kang et al., 2010) and climbing perch (Loong et al., 2012), the slc12a2b mRNA expression and NKCC protein quantity followed the saline concentration of water inhabited by FW- and SW-acclimated fish. However, no response was reported in slc12a2a mRNA expression after the transfer of Mozambique tilapia from BW–FW (Breves et al., 2010).
The branchial tissue of silversides of this study had an increase in kcnh1 mRNA expression soon after transfer to FW. A similar result was obtained in threespine stickleback, in which the kcnh4 mRNA expression was higher in FW- than in SW-acclimated fish (Taugbøl et al., 2014). Furthermore, the return of mRNA expression to pre-transfer levels indicates an acclimation to media. Although kcnh1 functions in organisms are not well known, our results reveal that this gene may play a role in osmoregulation.
The HSP70 overexpression is induced by some environmental stressors, such as temperature changes, UV and γ-irradiation, and chemical exposure (Place and Hofmann, 2005; Yamashita et al., 2010; Rajeshkumar et al., 2013). In O. humensis, the salinity change was able to induce a response of hspa1a gene. The same was observed in threespine stickleback (Taugbøl et al., 2014), in North Sea cod and Baltic Sea cod (Larsen et al., 2012), and in Kaluga (Peng et al., 2016). The up-regulation in hspa1a mRNA after BW–FW transfer indicates an increase in stress. The significant down-regulation observed after 7 days in FW indicates a reduction in stress levels, indicating acclimation. On the other hand, the unchanged hspa1a mRNA levels after the FW–BW transfer reveal that this salinity challenge is less stressful to O. humensis.
Blood glucose has been used to estimate acute stress conditions in fishes (Cataldi et al., 2005), as reported to silverside O. bonariensis (Tsuzuki et al., 2001). Furthermore, the hematocrit levels also can serve as stress indicator in fishes. The increment in hematocrit may indicate the presence of an active stress factor (Hudson et al., 2008). The quick increase in glycemia and the trend of increase in hematocrit, allied to the increase in hspa1a expression after BW–FW transfer, reveal that this “salt-free” environment is significantly more stressful to O. humensis than the BW medium. In O. bonariensis, the transfer from FW to 20 ppt BW triggered a decrease in stress followed by a decrease in glycemia and hematocrit, indicating that FW is more stressful than BW also in this species (Tsuzuki et al., 2001). Moreover, the unchanged blood glucose and hematocrit corroborates to hspa1a expression results, which indicates that FW–BW transfer leads to lower levels of stress in O. humensis.
Reduction in atp1a3a expression and blood osmolality in BW–FW-transferred silversides indicates a decrease in osmoregulation activity, which is energetically expensive to fishes. Even so, the stress in BW–FW group was higher, suggesting that the physiology of O. humensis is only partially adapted to FW. In the FW–BW transfer group, the blood osmolality maintains its levels. In O. bonariensis, the transfer to 20 ppt BW generates a significant increment in osmolality until 24 h post-transfer (Tsuzuki et al., 2001, 2007). The 5 ppt BW transfer also generated an increase in blood osmolality, but not as significant as 20 ppt (Tsuzuki et al., 2007). Here, O. humensis osmolality remained without significant variation in FW–BW. Maybe the 10 ppt salt concentration medium was insufficient to increase significantly the blood osmolality of O. humensis, despite the half salt concentration is able to increase this parameter of O. bonariensis. However, we hypothesized that FW–BW transference causes increased recruitment of both atp1a3a and slc12a2b, which would work together in the control of ion secretion and stabilization of blood osmolality. This may indicate that the silverside O. humensis is more efficient than O. bonariensis in the hyposmoregulation process.
The return of osmoregulatory genes expression and blood glucose close to pre-transfer levels after significant increase indicates an efficient acclimation (Grutter and Pankhurst, 2000; Havird et al., 2013). The blood glucose and atp1a3a, slc12a2b, kcnh1, and hspa1a mRNA stabilized in 7 days. These markers of osmoregulation and stress corroborated acclimation after 1 week despite the high stress occurred after the saline challenge.
Our results suggest that the BW–FW transfer is more stressful to O. humensis than the FW–BW transfer. Even though O. humensis is not widely cultivated in aquaculture today, the information that this species follows the responses of O. bonariensis and remains less stressed when in brackish environments is very important. Salinity levels were shown to modulate the energy supply available for growth and reproduction in farmed fishes (Altinok and Grizzle, 2001; Chand et al., 2015), and its optimal adjustment can benefit O. humensis production in captivity for aquaculture purposes. The brackish medium has potential for decrease in economic losses by mortality due to handling, transport, crowding, and poor water quality (Strüssmann et al., 1996; Tsuzuki et al., 2000b, 2001) and increase the survival rate of embryos in farm production (Piedras et al., 2009). Keeping O. humensis in this near-isosmotic environment can potentially allow for better growth rates, food conversion ratio, energy absorption efficiency, among other parameters of interest. Furthermore, the cultivation of O. humensis in conditions closer to the ideal decreases the stress and the physiological variations due to it and favors the use of this species as a model in scientific research.
Even regarded as FW, these species are commonly in contact with salt and BW on estuary and coastal lagoons of South America and have better development and survival in saline environments (Tsuzuki et al., 2000a, 2001; Piedras et al., 2009). The coastal plain of southern Brazil system was originated from successive transgressions and regressions moves since the upper Pleistocene (Bemvenuti, 2006). The formation and radiation of Odontesthes spp. is also recent and occurs during Pleistocene–Holocene (Beheregaray et al., 2002; Lovejoy et al., 2006; Heras and Roldán, 2011). Furthermore, there are strong evidences that various genus of Atherinopsidae order, including Odontesthes, have an evolutionary tendency to invade the continental FWs (Bloom et al., 2013; Campanella et al., 2015). Thus, the same events that originated the Southeastern South America continental lakes and lagoons may have triggered the speciation process of Odontesthes spp.
The previous and the present study corroborate to the theory that the FW is not the ideal environment for O. humensis and give more arguments to the theory of repeated invasion of FW habitats by silversides marine ancestors. The conquest of the FW environment is very recent, so there has not been enough time for the selection of adaptive mechanisms for life in this condition without the elimination of some basal stress levels.
Author Contributions
TS, GM, and VC were responsible for experimental design, data analysis, and manuscript writing. TS, GM, MR, and RR were responsible for fish acclimation and maintenance. TS, GM, BB, IL, LS, MR, RR, and WD were responsible for the biological collections. TS, BB, IL, LS, WD, and VC were responsible for the molecular biology, from RNA extraction to sequencing, and qRT-PCR analysis. OD, TC, and FS were also responsible for qRT-PCR analysis. DP and RR were also responsible for data analysis and language review.
Funding
This study was supported by the Ministério da Ciência (422292/2016-8), Tecnologia e Inovação/Conselho Nacional de Desenvolvimento Científico e Tecnológico (Edital Universal No. 472210/2013-0) and Coordenação de Aperfeiçoamento de Pessoal de Nível Superior (AUXPE 2900/2014). TS, GM, WD, and MR are individually supported by Coordenação de Aperfeiçoamento de Pessoal de Nível Superior. DP, OD, TC, FS, RR, and VC are also individually supported by Conselho Nacional de Desenvolvimento Científico e Tecnológico.
Conflict of Interest Statement
The authors declare that the research was conducted in the absence of any commercial or financial relationships that could be construed as a potential conflict of interest.
Acknowledgments
We are greatly thankful to Mr. Nilton Link and Mr. Vítor Colvara for the help in the maintenance of animals and Janaína Pedron for the help in blood osmolality analysis.
Abbreviations
BW, brackish water; FW, freshwater; SW, seawater.
Supplementary Material
The Supplementary Material for this article can be found online at: https://www.frontiersin.org/articles/10.3389/fgene.2018.00028/full#supplementary-material
FIGURE S1 | Phylogenetic analysis of NKAα-subunit amino acid sequences of mammals and teleosts. The tree was generated by MEGA v6 software using the Neighbor-Joining method. The bootstrap values from 10,000 replicates are showed at each node. Values between parentheses represent the identity (in %) of the sequence with NKAα3a of Odontesthes humensis. Scale bar units represent the number of amino acid substitutions per site. NKAα1 GenBank ID: Homo sapiens, CAA27840.1. NKAα1a GenBank ID: Danio rerio, NP_571762.1; Solea senegalensis, BAN17690.1; Fundulus heteroclitus, AAL18002.1; Oncorhynchus masou, BAJ13363.1; Oncorhynchus mykiss, NP_001117933.1. NKAα1b GenBank ID: O. mykiss, NP_001117932.1; O. masou, BAJ13362.1; S. senegalensis, BAN17691.1; D. rerio, NP_571765.1. NKAα2 GenBank ID: Rattus norvegicus, NP_036637.1; O. mykiss, NP_001117930.1; D. rerio, AAF98359.1; S. senegalensis, BAO02373.1; F. heteroclitus, AAL18003.1. NKAα3 GenBank ID: H. sapiens, NP_689509.1; R. norvegicus, NP_036638.1; Oreochromis mossambicus, AAF75108.1; O. mykiss, NP_001118102.1. NKAα3a GenBank ID: D. rerio, NP_571759.2; S. senegalensis, BAN17693.1; Monopterus albus, AGV06213.1. NKAα3b GenBank ID: M. albus, AGV06214.1; S. senegalensis, BAN17692.1. NKAα4 GenBank ID: H. sapiens, Q13733.3; R. norvegicus, NP_074039.1. NKAα1 from Caenorhabditis elegans, NP_506269.1, was used as outgroup.
FIGURE S2 | Phylogenetic analysis of NKCC amino acid sequences of mammals and teleosts. The tree was generated by MEGA v6 software using the Neighbor-Joining method. The bootstrap values from 10,000 replicates are showed at each node. Values between parentheses represent the identity (in %) of the sequence with NKCC1b of O. humensis. Scale bar units represent the number of amino acid substitutions per site. NKCC1 GenBank ID: H. sapiens, P55011.1; Mus musculus, NP_033220.2. NKCC1a GenBank ID: Anguilla Anguilla, CAD31111.1; Salmo salar, NP_001117155.1; Oryzias dancena, ADN18710.1; O. mossambicus, AAR97731.1; Anabas testudineus AFK29496.1. NKCC1b GenBank ID: O. dancena, ADK47392.1; M. albus, AGX01628.1; O. mossambicus, AAR97732.1; A. anguilla, CAD31112.1. NKCC2 GenBank ID: H. sapiens, Q13621.2; M. musculus, P55014.2; O. mossambicus, AAR97733.1; Takifugu obscurus, BAH20440.1. NKCC1 from Strongylocentrotus purpuratus, NP_001106707.1, was used as outgroup.
FIGURE S3 | Phylogenetic analysis of KCNH amino acid sequences from EAG and ELK subfamilies of mammals and teleosts. Third existent ERG subfamily was discard in order to maintain an abbreviated analysis. The tree was generated by MEGA v6 software using the Neighbor-Joining method. The bootstrap values from 10,000 replicates are showed at each node. Values between parentheses represent the identity (in %) of the sequence with KCNH1 of O. humensis. Scale bar units represent the number of amino acid substitutions per site. KCNH1 GenBank ID: H. sapiens, NP_758872.1; M. musculus, NP_034730.1; D. rerio, XP_009291371.1; Oreochromis niloticus, XP_005474450.1. KCNH3 GenBank ID: H. sapiens, NP_001300959.1; D. rerio, XP_001919436.3; Nothobranchius furzeri, SBP57798.1. KCNH4 GenBank ID: H. sapiens, NP_036417.1; P. reticulata, XP_008415281.2; O. niloticus, XP_019213276.1; Maylandia zebra, XP_012774834.1. KCNH5 GenBank ID: H. sapiens, NP_647479.2; M. musculus, NP_766393.2; D. rerio, NP_001263209.1; O. niloticus, XP_003451242.1. KCNH8 GenBank ID: H. sapiens, NP_653234.2; M. musculus, NP_001026981.2; O. niloticus, XP_003448945.1; M. zebra, XP_004547937.1; Poecilia reticulata, XP_008419899.1. KCNH1 from Toxocara canis, KHN74999.1, was used as outgroup.
FIGURE S4 | Phylogenetic analysis of HSP70 amino acid sequences of mammals and teleosts. The tree was generated by MEGA v6 software using the Neighbor-Joining method. The bootstrap values from 10,000 replicates are showed at each node. Values between parentheses represent the identity (in %) of the sequence with HSP70 of Odontesthes humensis. Scale bar units represent the number of amino acid substitutions per site. HSP70-1A GenBank ID: H. sapiens, NP_005336.3; M. musculus, NP_034609.2; Notothenia coriiceps, XP_010769991.1; M. albus, AGO01980.1; Nothobranchius korthausae, SBQ68887.1. HSP70-1B GenBank ID: M. albus, AGO01981.1; D. rerio, NP_001093532.1. HSP70-2 GenBank ID: H. sapiens, AAH36107.1; M. musculus, EDL36460.1. HSP70-1 from C. elegans, NP_503068.1, was used as outgroup.
TABLE S1 | Expression of reference genes of Odontesthes humensis before (D0) and after (D1, D7, and D15) hypo– and hyperosmotic shock. Equal letters represent no difference in a same column. Abbreviations: Ct, cycle threshold values; D0, day zero; D1, day one; D7, day seven; D15, day fifteen; FW–BW, transfer from freshwater to brackish water; BW–FW, transfer from brackish water to freshwater.
Footnotes
- ^ http://blast.ncbi.nlm.nih.gov/
- ^ https://www.expasy.org/
- ^ http://www.uniprot.org/
- ^ http://primer3.ut.ee
References
Altinok, I., and Grizzle, J. M. (2001). Effects of brackish water on growth, feed conversion and energy absorption efficiency by juvenile euryhaline and freshwater stenohaline fishes. J. Fish Biol. 59, 1142–1152. doi: 10.1111/j.1095-8649.2001.tb00181.x
Armesto, P., Campinho, M. A., Rodríguez-Rúa, A., Cousin, X., Power, D. M., Manchado, M., et al. (2014). Molecular characterization and transcriptional regulation of the Na+/K+ ATPase α subunit isoforms during development and salinity challenge in a teleost fish, the Senegalese sole (Solea senegalensis). Comp. Biochem. Physiol. B Biochem. Mol. Biol. 175, 23–38. doi: 10.1016/j.cbpb.2014.06.004
Basu, N., Nakano, T., Grau, E. G., and Iwama, G. K. (2001). The effects of cortisol on Heat Shock Protein 70 levels in two fish species. Gen. Comp. Endocrinol. 124, 97–105. doi: 10.1006/gcen.2001.7688
Beheregaray, L. B., Sunnucks, P., and Briscoe, D. A. (2002). A rapid fish radiation associated with the last sea-level changes in southern Brazil: the silverside Odontesthes perugiae complex. Proc. R. Soc. B Biol. Sci. 269, 65–73. doi: 10.1098/rspb.2001.1838
Bemvenuti, M. A. (2006). Silversides in South Brazil: morphological and ecological aspects. Biocell 30, 111–118.
Bloom, D. D., Weir, J. T., Piller, K. R., and Lovejoy, N. R. (2013). Do freshwater fishes diversify faster than marine fishes? A test using state-dependent diversification analyses and molecular phylogenetics of New World silversides (Atherinopsidae). Evolution 67, 2040–2057. doi: 10.1111/evo.12074
Breves, J. P., Hasegawa, S., Yoshioka, M., Fox, B. K., Davis, L. K., Lerner, D. T., et al. (2010). Acute salinity challenges in Mozambique and Nile tilapia: differential responses of plasma prolactin, growth hormone and branchial expression of ion transporters. Gen. Comp. Endocrinol. 167, 135–142. doi: 10.1016/j.ygcen.2010.01.022
Campanella, D., Hughes, L. C., Unmack, P. J., Bloom, D. D., Piller, K. R., and Ortí, G. (2015). Multi-locus fossil-calibrated phylogeny of Atheriniformes (Teleostei, Ovalentaria). Mol. Phylogenet. Evol. 86, 8–23. doi: 10.1016/j.ympev.2015.03.001
Cataldi, E., Mandich, A., Ozzimo, A., and Cataudella, S. (2005). The interrelationships between stress and osmoregulation in a euryhaline fish, Oreochromis mossambicus. J. Appl. Ichthyol. 21, 229–231. doi: 10.1111/j.1439-0426.2005.00634.x
Chand, B. K., Trivedi, R. K., Dubey, S. K., Rout, S. K., Beg, M. M., and Das, U. K. (2015). Effect of salinity on survival and growth of giant freshwater prawn Macrobrachium rosenbergii (de Man). Aquacult. Rep. 2, 26–33. doi: 10.1016/j.aqrep.2015.05.002
D’Cotta, H., Valotaire, C., Gac, F., Le, and Prunet, P. (2000). Synthesis of gill Na+-K+-ATPase in Atlantic salmon smolts: differences in α-mRNA and α-protein levels. Am. J. Physiol. Regul. Integr. Comp. Physiol. 278, R101–R110. doi: 10.1152/ajpregu.2000.278.1.R101
Dyer, B. S. (2000). Systematic review and biogeography of the freshwater fishes of Chile. Estud. Oceanol. 19, 77–98. doi: 10.1002/dvdy.24533
Dyer, B. S. (2006). Systematic revision of the South American silversides (Teleostei, Atheriniformes). Biocell 30, 69–88.
Grutter, A. S., and Pankhurst, N. W. (2000). The effects of capture, handling, confinement and ectoparasite load on plasma levels of cortisol, glucose and lactate in the coral reef fish Hemigymnus melapterus. J. Fish Biol. 57, 391–401. doi: 10.1006/jfbi.2000.1312
Guynn, S. R., Scofield, M. A., and Petzel, D. H. (2002). Identification of mRNA and protein expression of the Na/K-ATpase α1-, α2- and α3-subunit isoforms in Antarctic and New Zealand nototheniid fishes. J. Exp. Mar. Biol. Ecol. 273, 15–32. doi: 10.1016/S0022-0981(02)00136-3
Havird, J. C., Henry, R. P., and Wilson, A. E. (2013). Altered expression of Na+/K+–ATPase and other osmoregulatory genes in the gills of euryhaline animals in response to salinity transfer: a meta-analysis of 59 quantitative PCR studies over 10 years. Comp. Biochem. Physiol. D Genomics Proteomics 8, 131–140. doi: 10.1016/j.cbd.2013.01.003
He, Y., Luo, M., Yi, M., Sheng, Y., Cheng, Y., Zhou, R., et al. (2013). Identification of a testis-enriched Heat Shock Protein and fourteen members of Hsp70 family in the swamp eel. PLOS ONE 8:e65269. doi: 10.1371/journal.pone.0065269
Heras, S., and Roldán, M. I. (2011). Phylogenetic inference in Odontesthes and Atherina (Teleostei: Atheriniformes) with insights into ecological adaptation. C. R. Biol. 334, 273–281. doi: 10.1016/j.crvi.2011.03.004
Hiroi, J., and McCormick, S. D. (2012). New insights into gill ionocyte and ion transporter function in euryhaline and diadromous fish. Respir. Physiol. Neurobiol. 184, 257–268. doi: 10.1016/j.resp.2012.07.019
Hudson, H. A., Brauer, P. R., Scofield, M. A., and Petzel, D. H. (2008). Effects of warm acclimation on serum osmolality, cortisol and hematocrit levels in the Antarctic fish, Trematomus bernacchii. Polar Biol. 31, 991–997. doi: 10.1007/s00300-008-0438-8
Hwang, P., Lee, T., and Lin, L. (2011). Ion regulation in fish gills: recent progress in the cellular and molecular mechanisms. Am. J. Physiol. Regul. Integr. Comp. Physiol. 301, R28–R47. doi: 10.1152/ajpregu.00047.2011
Kakumura, K., Takabe, S., Takagi, W., Hasegawa, K., Konno, N., Bell, J. D., et al. (2015). Morphological and molecular investigations of the holocephalan elephant fish nephron: the existence of a countercurrent-like configuration and two separate diluting segments in the distal tubule. Cell Tissue Res. 362, 677–688. doi: 10.1007/s00441-015-2234-4
Kang, C. K., Tsai, H. J., Liu, C. C., Lee, T. H., and Hwang, P. P. (2010). Salinity-dependent expression of a Na+, K+, 2Cl- cotransporter in gills of the brackish medaka Oryzias dancena: a molecular correlate for hyposmoregulatory endurance. Comp. Biochem. Physiol. A Mol. Integr. Physiol. 157, 7–18. doi: 10.1016/j.cbpa.2010.05.013
Kiilerich, P., Tipsmark, C. K., Borski, R. J., and Madsen, S. S. (2011). Differential effects of cortisol and 11-deoxycorticosterone on ion transport protein mRNA levels in gills of two euryhaline teleosts, Mozambique tilapia (Oreochromis mossambicus) and striped bass (Morone saxatilis). J. Endocrinol. 209, 115–126. doi: 10.1530/JOE-10-0326
Larsen, P. F., Nielsen, E. E., Meier, K., Olsvik, P. A., Hansen, M. M., and Loeschcke, V. (2012). Differences in salinity tolerance and gene expression between two populations of Atlantic cod (Gadus morhua) in response to salinity stress. Biochem. Genet. 50, 454–466. doi: 10.1007/s10528-011-9490-0
Li, Z., Lui, E. Y., Wilson, J. M., Ip, Y. K., Lin, Q., and Lam, T. J. (2014). Expression of key ion transporters in the gill and esophageal-gastrointestinal tract of euryhaline Mozambique tilapia Oreochromis mossambicus acclimated to fresh water, seawater and hypersaline water. PLOS ONE 9:e87591. doi: 10.1371/journal.pone.0087591
Lin, C.-H., Huang, C.-L., Yang, C.-H., Lee, T.-H., and Hwang, P.-P. (2004). Time-course changes in the expression of Na, K-ATPase and the morphometry of mitochondrion-rich cells in gills of euryhaline tilapia (Oreochromis mossambicus) during freshwater acclimation. J. Exp. Zool. A Comp. Exp. Biol. 301A, 85–96. doi: 10.1002/jez.a.20007
Livak, K. J., and Schmittgen, T. D. (2001). Analysis of relative gene expression data using real-time quantitative PCR and the 2-ΔΔCT method. Methods 25, 402–408. doi: 10.1006/meth.2001.1262
Loong, A. M., Chew, S. F., Wong, W. P., Lam, S. H., and Ip, Y. K. (2012). Both seawater acclimation and environmental ammonia exposure lead to increases in mRNA expression and protein abundance of Na+:K+:2Cl- cotransporter in the gills of the climbing perch, Anabas testudineus. J. Comp. Physiol. B Biochem. Syst. Environ. Physiol. 182, 491–506. doi: 10.1007/s00360-011-0634-7
Loubens, G., and Osorio, F. (1991). “Especies introducidas: Basilichthyes bonariesis,” in El Lago Titicaca: Síntesis del Conocimiento Limnológico Actual, eds C. Dejoux and A. Iltis (La Paz, BO: ORSTOM/HISBOL), 431–449.
Lovejoy, N. R., Albert, J. S., and Crampton, W. G. R. (2006). Miocene marine incursions and marine/freshwater transitions: evidence from Neotropical fishes. J. South Am. Earth Sci. 21, 5–13. doi: 10.1016/j.jsames.2005.07.009
McCallister, C., Kdeiss, B., Oliverio, R., and Nikolaidis, N. (2016). Characterization of the binding between a 70-kDa heat shock protein, HspA1A, and phosphoinositides. Biochem. Biophys. Res. Commun. 472, 270–275. doi: 10.1016/j.bbrc.2016.02.103
Menone, M. L., Aizpún de Moreno, J. E., Moreno, V. J., Lanfranchi, A. L., Metcalfe, T. L., and Metcalfe, C. D. (2000). Environmental contamination and toxicology PCBs and organochlorines in tissues of silverside (Odontesthes bonariensis) from a coastal lagoon in Argentina. Arch. Environ. Contam. Toxicol. 38, 202–208. doi: 10.1007/s002449910027
Morais-Cabral, J. H., and Robertson, G. A. (2015). The enigmatic cytoplasmic regions of KCNH channels. J. Mol. Biol. 427, 67–76. doi: 10.1016/j.jmb.2014.08.008
Morimoto, R. I. (1998). Regulation of the heat shock transcriptional response: cross talk between a family of heat shock factors, molecular chaperones, and negative regulators. Genes Dev. 12, 3788–3796. doi: 10.1101/gad.12.24.3788
Nilsen, T. O., Ebbesson, L. O. E., Madsen, S. S., McCormick, S. D., Andersson, E., Björnsson, B. T., et al. (2007). Differential expression of gill Na+,K+-ATPase alpha- and beta-subunits, Na+,K+,2Cl- cotransporter and CFTR anion channel in juvenile anadromous and landlocked Atlantic salmon Salmo salar. J. Exp. Biol. 210, 2885–2896. doi: 10.1242/jeb.002873
Occhiodoro, T., Bernheim, L., Liu, J. H., Bijlenga, P., Sinnreich, M., Bader, C. R., et al. (1998). Cloning of a human ether-a-go-go potassium channel expressed in myoblasts at the onset of fusion. FEBS Lett. 434, 177–182. doi: 10.1016/S0014-5793(98)00973-9
Pardo, L. A., and Stühmer, W. (2013). The roles of K+ channels in cancer. Nat. Rev. Cancer 14, 39–48. doi: 10.1038/nrc3635
Peng, G., Zhao, W., Shi, Z., Chen, H., Liu, Y., Wei, J., et al. (2016). Cloning HSP70 and HSP90 genes of kaluga (Huso dauricus) and the effects of temperature and salinity stress on their gene expression. Cell Stress Chaperones 21, 349–359. doi: 10.1007/s12192-015-0665-1
Piedras, S. R. N., Fernandes, J. L. O., Motoyama, I. S., and Martins, G. B. (2009). Efeito de diferentes concentrações de salinas (NaCl) na sobrevivência de embriões de peixe – rei Odontesthes bonariensis e Odontesthes humensis. Biotemas 22, 235–238. doi: 10.5007/2175-7925.2009v22n3p235
Place, S. P., and Hofmann, G. E. (2005). Constitutive expression of a stress-inducible heat shock protein gene, hsp70, in phylogenetically distant Antarctic fish. Polar Biol. 28, 261–267. doi: 10.1007/s00300-004-0697-y
Pouey, J. L. O. F., Rocha, C. B., Tavares, R. A., Portelinha, M. K., and Piedras, S. R. N. (2012). Frequência alimentar no crescimento de alevinos de peixe-rei Odontesthes humensis. Semin. Agrar. 33, 2423–2427. doi: 10.5433/1679-0359.2012v33n6p2423
Rajeshkumar, S., Mini, J., and Munuswamy, N. (2013). Effects of heavy metals on antioxidants and expression of HSP70 in different tissues of Milk fish (Chanos chanos) of Kaattuppalli Island, Chennai, India. Ecotoxicol. Environ. Saf. 98, 8–18. doi: 10.1016/j.ecoenv.2013.07.029
Scott, G. R., Baker, D. W., Schulte, P. M., and Wood, C. M. (2008). Physiological and molecular mechanisms of osmoregulatory plasticity in killifish after seawater transfer. J. Exp. Biol. 211, 2450–2459. doi: 10.1242/jeb.017947
Scott, G. R., and Schulte, P. M. (2005). Intraspecific variation in gene expression after seawater transfer in gills of the euryhaline killifish Fundulus heteroclitus. Comp. Biochem. Physiol. A Mol. Integr. Physiol. 141, 176–182. doi: 10.1016/j.cbpb.2005.05.002
Somoza, G. M., Miranda, L. A., Berasain, G. E., Colautti, D., Remes Lenicov, M., and Strüssmann, C. A. (2008). Historical aspects, current status and prospects of pejerrey aquaculture in South America. Aquacult. Res. 39, 784–793. doi: 10.1111/j.1365-2109.2008.01930.x
Stefansson, S. O., Nilsen, T. O., Ebbesson, L. O. E., Wargelius, A., Madsen, S. S., Björnsson, B. T., et al. (2007). Molecular mechanisms of continuous light inhibition of Atlantic salmon parr–smolt transformation. Aquaculture 273, 235–245. doi: 10.1016/j.aquaculture.2007.10.005
Stengel, R., Rivera-Milla, E., Sahoo, N., Ebert, C., Bollig, F., Heinemann, S. H., et al. (2012). Kcnh1 voltage-gated potassium channels are essential for early zebrafish development. J. Biol. Chem. 287, 35565–35575. doi: 10.1074/jbc.M112.363978
Strüssmann, C. A., Moriyama, S., Hanke, E. F., Cota, J. C. C., and Takashima, F. (1996). Evidence of thermolabile sex determination in pejerrey. J. Fish Biol. 48, 643–651. doi: 10.1111/j.1095-8649.1996.tb01459.x
Tamura, K., Stecher, G., Peterson, D., Filipski, A., and Kumar, S. (2013). MEGA6: molecular evolutionary genetics analysis version 6.0. Mol. Biol. Evol. 30, 2725–2729. doi: 10.1093/molbev/mst197
Taugbøl, A., Arntsen, T., Østbye, K., and Vøllestad, L. A. (2014). Small changes in gene expression of targeted osmoregulatory genes when exposing marine and freshwater threespine stickleback (Gasterosteus aculeatus) to abrupt salinity transfers. PLOS ONE 9:e106894. doi: 10.1371/journal.pone.0106894
Tavares, R. A., Fernandes, J. M., Garcia, V. H., Piedras, S. R. N., Pouey, J. L. O. F., Dionello, N. J. L., et al. (2014). Growth performance of three pejerrey genetic groups in intensive culture system. Semin. Cienc. Agrar. 35, 2749–2758. doi: 10.5433/1679-0359.2014v35n5p2749
Tipsmark, C. K., Madsen, S. S., and Borski, R. J. (2004). Effect of salinity on expression of branchial ion transporters in striped bass (Morone saxatilis). J. Exp. Zool. Comp. Exp. Biol. 301A, 979–991. doi: 10.1002/jez.a.119
Tipsmark, C. K., Madsen, S. S., Seidelin, M., Christensen, A. S., Cutler, C. P., and Cramb, G. (2002). Dynamics of Na+,K+,2Cl- cotransporter and Na+,K+-ATPase expression in the branchial epithelium of brown trout (Salmo trutta) and Atlantic salmon (Salmo salar). J. Exp. Zool. 293, 106–118. doi: 10.1002/jez.10118
Tsuzuki, M. Y., Aikawa, H., Strüssmann, C. A., and Takashima, F. (2000a). Comparative survival and growth of embryos, larvae, and juveniles of pejerrey Odontesthes bonariensis and O. hatcheri at different salinities. J. Appl. Ichthyol. 16, 126–130. doi: 10.1046/j.1439-0426.2000.00227.x
Tsuzuki, M. Y., Aikawa, H., Strüssmann, C. A., and Takashima, F. (2000b). Physiological responses to salinity increases in the freshwater silversides Odontesthes bonariensis and O. hatcheri (Pisces, Atherinidae). Rev. Bras. Oceanogr. 48, 81–85. doi: 10.1590/S1413-77392000000100007
Tsuzuki, M. Y., Ogawa, K., Strüssmann, C. A., Maita, M., and Takashima, F. (2001). Physiological responses during stress and subsequent recovery at different salinities in adult pejerrey Odontesthes bonariensis. Aquaculture 200, 349–362. doi: 10.1016/S0044-8486(00)00573-1
Tsuzuki, M. Y., Ogawa, K., Strüssmann, C. A., Maita, M., Takashima, F., and Melo, C. M. R. (2007). The significance of cortisol on acclimation to salinity in pejerrey Odontesthes bonariensis. Arq. Bras. Med. Vet. Zootec. 59, 1301–1307. doi: 10.1590/S0102-09352007000500030
Whitehead, A., Roach, J. L., Zhang, S., and Galvez, F. (2012). Salinity- and population-dependent genome regulatory response during osmotic acclimation in the killifish (Fundulus heteroclitus) gill. J. Exp. Biol. 215(Pt 8), 1293–1305. doi: 10.1242/jeb.062075
Yamashita, M., Yabu, T., and Ojima, N. (2010). Stress protein HSP70 in fish. Aqua Biosci. Monogr. 3, 111–141. doi: 10.5047/absm.2010.00304.0111
Keywords: acclimation, blood, brackish water, fish, freshwater, genes, salt, transfer
Citation: Silveira TLR, Martins GB, Domingues WB, Remião MH, Barreto BF, Lessa IM, Santos L, Pinhal D, Dellagostin OA, Seixas FK, Collares T, Robaldo RB and Campos VF (2018) Gene and Blood Analysis Reveal That Transfer from Brackish Water to Freshwater Is More Stressful to the Silverside Odontesthes humensis. Front. Genet. 9:28. doi: 10.3389/fgene.2018.00028
Received: 26 October 2017; Accepted: 22 January 2018;
Published: 06 February 2018.
Edited by:
Roberto Ferreira Artoni, Ponta Grossa State University, BrazilReviewed by:
Daniele Aparecida Matoso, Federal University of Amazonas, BrazilRicardo Shohei Hattori, Agência Paulista de Tecnologia dos Agronegócios, Brazil
Copyright © 2018 Silveira, Martins, Domingues, Remião, Barreto, Lessa, Santos, Pinhal, Dellagostin, Seixas, Collares, Robaldo and Campos. This is an open-access article distributed under the terms of the Creative Commons Attribution License (CC BY). The use, distribution or reproduction in other forums is permitted, provided the original author(s) and the copyright owner are credited and that the original publication in this journal is cited, in accordance with accepted academic practice. No use, distribution or reproduction is permitted which does not comply with these terms.
*Correspondence: Vinicius F. Campos, ZmFyaWFzY2FtcG9zQGdtYWlsLmNvbQ==
† These authors have contributed equally to this work.