- 1Bren School of Environmental Science and Management, University of California, Santa Barbara, Santa Barbara, CA, United States
- 2University of California Cooperative Extension, Agriculture and Natural Resources Division, Modesto, CA, United States
- 3Bren School of Environmental Science and Management, University of California, Santa Barbara, Santa Barbara, CA, United States
Forest disturbances such as wildfire and drought-related disease often lead to declines in productivity that both influence and are influenced by forest water use, particularly in the semi-arid environments of the Western US. Fuel treatments are frequently proposed to reduce vulnerability to these drought-related impacts and in some cases as an approach to increase water yield. By changing ecosystem structure, fuel treatments alter ecosystem function (including hydrologic cycling, carbon sequestration, energy partitioning, and biogeochemical cycling). Empirical studies of the impacts of changing ecosystem structure, either through active forest management or through natural disturbances, show a wide range of responses that include both increases and decreases in forest water use. Variation in climate and species, as well as the magnitude of forest density reduction, are commonly proposed as explanations for this variation. In this paper we use a coupled eco-hydrologic model to demonstrate that subsurface features are likely to be a critical, but often over-looked, factor that influences forest water use and regeneration following density reduction treatments. Using a case study site in the southern Sierra Nevada Mountains of California, we show that whether forest water use increases or decreases following density reduction, as well as the magnitude and rate of recovery of hydrologic changes, depends strongly on plant accessible water storage capacity within the rooting zone and the extent to which the root structures of neighboring trees interact and share water. We find that in some cases density reduction can increase water yield and productivity of remaining trees for the first few years following treatment. However, we also show that when soils are shallow and roots systems overlap, counter-intuitive increases in water use and related declines in productivity can occur due to water stress. Results highlight the importance of accounting for site-specific variation, such as soil water storage capacity, in assessing how fuel treatments may interact with ecosystem water use and drought vulnerability, and ultimately downslope impacts on streamflow.
Introduction
Forests in semi-arid climates are vulnerable to periodic disturbances, and the frequency and severity of many of these disturbances increase during drought. Fires are more frequent, larger and potentially more severe as a result of drought stress (Westerling and Bryant, 2008; Dennison et al., 2014; Littell et al., 2016). Declines in forest productivity, other disturbances (e.g., disease and insects), and increased mortality are also more frequent in drier years (Dale et al., 2001; Vicente-Serrano, 2007; Williams et al., 2010; Adams et al., 2012). Drought has additional downslope hydrologic effects by reducing streamflow and groundwater recharge that support aquatic ecosystems and human water supplies (Howitt et al., 2014). Drought-induced changes to vegetation structure through fire and other disturbances can also have hydrologic consequences, by changing vegetation water use and by altering sedimentation rates following fire (Ice et al., 2004). All of these drought-related impacts on ecosystem structure and function are likely to intensify in the future, especially in Mediterranean ecosystems where seasonal water stress is already pronounced (Hanson and Weltzin, 2000; Dai, 2011).
A primary goal of forest management is to protect the health of forests and the ecosystem services they provide, including biodiversity, water and biogeochemical cycling, recreation, habitat provision, and carbon sequestration (Trumbore et al., 2015). A number of forest management strategies have been proposed to respond to drought and its potential impacts, including increasing the extent of protected areas, eradicating high-water consumptive invasive species, and manipulation to reduce runoff losses with terracing or direct irrigation (Grant et al., 2013). To address fire hazard concerns, a widely recommended tool is vegetation density reduction through prescribed burns or forest thinning (Stephens et al., 2012; Ayres et al., 2016). Such fuel treatments have long been advocated as way to reduce fuels and thus the severity and potentially areal extent of fire, although the effectiveness of fuel treatment to reduce fire severity varies with locations and conditions. Fuel treatments may be particularly useful in regions where decades of fire suppression have lead to an unnatural accumulation of high-density fuels (Noss et al., 2008; Safford et al., 2012; Moritz et al., 2014; Hessburg et al., 2016).
While less common as a motivation, density reduction has also been proposed as a possible strategy to reduce water-stress for remaining trees. By reducing interception losses and below-ground competition for water, forest thinning may reduce productivity declines and ultimately mortality risk for remaining trees under drought conditions (Simonin et al., 2007; Dore et al., 2012; Clark et al., 2016). Reducing density may also decrease aggregate forest water use and potentially increase downstream water supply (D'Amato et al., 2013; Halofsky et al., 2014; Elkin et al., 2015). How the impacts of thinning evolve following treatments is uncertain, however, and a key challenge for science-based assessment of density reduction is that forest eco-hydrologic responses are likely to be location-specific and vary with climatic conditions. In addition, it can be challenging to derive conclusions about interactions among forest water use, climate and canopy structure from field-based studies that can rarely capture the episodic nature of both the treatments and climate forcing.
Not surprisingly, studies of the hydrologic impact of fuel treatments and forest mortality show varying impacts on forest water use. Thinning of a ponderosa pine site in the Southwestern US, for example, led to differing impacts on overstory and understory evapotranspiration for drought and non-drought years (Simonin et al., 2007). While some available field studies have found increases in water availability and reduced drought stress of remaining trees with thinning, others have shown that increased growth and leaf-to-sapwood area or species change following thinning can actually increase drought vulnerability (Clark et al., 2016). Studies of natural density reduction via forest mortality have similarly shown both declines in forest water use, but also counter-intuitive increases in evapotranspiration and declines in streamflow (Adams et al., 2012; Biederman et al., 2014). The number of field-based studies of thinning effects remains limited, and studies also typically focus only on the first few years after density reductions. Longer term studies of intensively managed plantation forest are available and show that thinning influences forest productivity for the first 30 years, although impacts diminish over time (Zhang et al., 2013). A multi-decade and site-specific perspective is clearly needed to understand the eco-hydrologic consequences of fuel treatments and to identify the climate factors that influence linkages between canopy structure and forest water use.
Given the complexity of long-term ecosystem dynamics, coupled eco-hydrologic models are useful tools for assessing how effects of forest density reductions interact with, and are governed by, site-specific biophysical and climatic factors (Balandier et al., 2006; Seidl et al., 2011). In this paper we use a mechanistic model of ecosystem carbon cycling and hydrology to evaluate how different controls on the eco-hydrologic response to forest treatments interact. We utilize a case study, described in detail below, to develop a more mechanistic understanding of why and how the eco-hydrology of density reduction treatments varies in space and time and to illustrate how models can be used to disentangle these effects. We focus on the interactions between four drivers of treatment responses that we posit are likely to be primary controls on this response: inter-annual climate variation, thinning intensity, subsurface water storage capacity, and the spatial extent of root competition for stored water.
While the importance of climate and thinning intensity has been noted by other studies (Adams et al., 2012; Clark et al., 2016), subsurface water storage and access by roots is often overlooked as a control on thinning response, despite their well-established influence on evapotranspiration in semi-arid water-limited environments (Brooks et al., 2015). Studies of root distributions and rooting strategies demonstrate widely varying degrees of shared below-ground moisture (Schenk and Jackson, 2002; Casper et al., 2003; Schenk, 2006). A key focus in this paper is therefore the importance of root competition for post-thinning water use and the degree to which trees that remain following density reduction have access to water previously utilized by removed vegetation.
Our case study is typical of mid-elevation California Sierra forests, where winter snow-dominated precipitation results in frequent summer water stress and where root access to subsurface water storage supports seasonal growth and ultimately influences downslope stream inputs (Bales et al., 2011). Understanding eco-hydrologic responses to forest treatments in this region is particularly important, given the vulnerability of such water-limited forests to drought (van Mantgem and Stephenson, 2007). It is also an area where forest thinning has been proposed to reduce fire severity and potentially influence water resources (Downing, 2015; Saksa et al., 2017). We analyze treatment impacts on water use and forest productivity for a range of biomass removal scenarios and across variation in climate and subsurface drivers of water availability. While this paper focuses on the rain-snow transition California Sierra, results also highlight the general role of subsurface properties and climate variation as controls on ecologically important hydrologic responses in other water-limited environments.
Methods
Modeling Framework
RHESSys is a coupled model of ecosystem carbon cycling and hydrology. RHESSys has been applied to estimate both water and carbon fluxes in a number of water limited environments and evaluated using a diversity of eco-hydrologic observations including streamflow (Tague and Grant, 2004; Tague and Peng, 2013), tree-rings, water, and carbon flux from flux towers (Zierl et al., 2007) and forest mortality patterns (Tague et al., 2013). RHESSys represents key feedbacks between carbon cycling and hydrology that are relevant for estimating the ecohydrologic impacts of fuel treatments as summarized in Figure 1 and described in more detail below.
Although RHESSys can model hillslope to watershed scale lateral moisture redistribution; we first examine density reduction effects at a stand scale, and assume that between stand interactions are negligible. These interactions are likely to be minimal for upslope forests. Further work will explore the implications of hillslope scale redistribution and specifically how downslope trees or riparian trees may take advantage of upslope changes in water consumption. Thus, in this paper, we focus on stand scale effects where redistribution of water occurs only between local trees. Vertical hydrologic processes modeled in RHESSys include transpiration and evaporation of water intercepted by the canopy and litter, as well as soil evaporation. All processes are modeled at a daily time step. Both evapotranspiration and transpiration are modeled using a Penman Monteith approach (Monteith et al., 1965). For transpiration, stomatal conductance is modified based on environmental controls (Jarvis, 1976) including moisture potential in the rooting zone. Energy, moisture, and carbon fluxes are computed separately for overstory and understory canopies. Radiation includes both diffuse and direct radiative fluxes and their attenuation through the canopy. Snowmelt is based on a quasi-energy budget radiation model. Infiltration of snowmelt or effective precipitation beneath litter and canopy layers is modeled using the Green and Ampt approach (Dingman, 2015).
In RHESSys, subsurface moisture storage is maintained in a rooting zone, unsaturated zone and saturated layers. Under wet conditions the water table can rise to intersect the rooting zone. Drainage between subsurface stores and laterally saturated fluxes from the patch depend on soil parameters, specifically pore size index, and air entry pressure, which together define field capacity storage. Saturated hydraulic conductivity and its decay with depth determine the rate of drainage. Water storage accessible to plants depends upon species-specific characteristics such as rooting depth and available water in the rooting zone. Storage capacity in the rooting zone is computed as the difference between field capacity and a species specific wilting point integrated over the rooting depth. Additional details are provided in Tague and Band (2004).
RHESSys links hydrologic processes with a carbon cycling model. Photosynthesis is computed using the Farquhar approach (Farquhar and Von Caemmerer, 1982) and respiration as a function of temperature and biomass (Ryan, 1995). RHESSys provides several different carbon allocation approaches. For this study, net primary productivity is allocated to leaves, stems and roots using a resource-based approach developed by Landsberg and Waring (1997). Additional details on carbon cycling parameters can be found in Garcia et al. (2016).
Location Description
In this paper we estimate thinning impacts for a mid-elevation Sierra forest composed primarily of mixed-conifer trees, which includes white fir (Abies concolor), incense cedar (Calocedrus decurrens), ponderosa pine (Pinus ponderosa), Jeffery pine (Pinus jeffreyi), and sugar pine (Pinus lambertiana). For this study, we therefore use vegetation parameters that approximate a mixed conifer forest plant functional type. Values of key water use parameters (e.g., maximum stomatal conductance, leaf water potential at stomatal closure) and parameters related to carbon cycling are assigned based on median values of observations from these species (Anderson et al., 2008) and are documented in RHESSys vegetation parameter libraries.
For meteorologic inputs we use data from the Grant Grove National Climate Data Center Station (Lat: 36.73603°N, Lon: 118.96122°W, elevation 2,005 m). We use daily minimum and maximum air temperature and precipitation from 1950 to 2015. This is a mid-elevation station, noteworthy for the availability of a long-term (50 years) data set that allows us to evaluate the impact of thinning over a range of climate forcing as discussed in more detail below.
Scenarios
We quantified the impact of forest thinning on water use for the first 14 years following thinning, allowing the RHESSys carbon cycling model to simulate regrowth during this period. We considered 4 density reduction or thinning intensity scenarios ranging from 10 to 40% of pre-thinning canopy cover. In the area targeted for density reduction we removed all vegetation carbon stores (leaf, stem, and root carbon) within the modeling unit. We do not differentiate between overstory and understory biomass in this study, and thus our scenarios can be assumed to remove both in equal proportions. Future work will assess sensitivity to how canopy structure is changed, and here we focus simply on the impact of overall biomass removal. We also remove root carbon. While root carbon does decompose following above ground biomass removal, we acknowledge that some of this carbon may remain for multiple years. Decomposition rates of root carbon are highly variable ranging between 10 and 120 years for 95% removal for western US forests (Edmonds, 1991). Thus, our model may initially overestimate post-density reduction in below-ground carbon stores, but this will not substantially change above- ground recovery dynamics and water availability estimate which are the focus of this paper. Since RHESSys does not explicitly model reproduction, we then initialize the density reduction area with seedling carbon stores: leaf area index (LAI) of 0.1, root carbon of 0.05 kgC/m2 which is within ranges observed for conifer seedlings (Law et al., 2003). We assume that resprouting occurs in the first year following thinning.
Plant available water storage capacity (PAWSC) is frequently a first-order control on plant transpiration (Asbjornsen et al., 2011; McNamara et al., 2011) and can determine whether changes in precipitation or snowmelt recharge translate into changes in plant water stress (Tague and Peng, 2013). Note that subsurface storage includes any layer that is accessible by plant roots and can store water. Thus, subsurface storage includes organic and mineral soil layers but also sapprolite and fractured bedrock. Given its importance as a control on transpiration and the sensitivity of plant function to climate drivers, PAWSC is also likely to be a crucial, site-specific control on ecosystem response to thinning. PAWSC also shows substantial heterogeneity within and between forest stands in the California Sierra, and Mediterranean ecosystems in general. One reason for this is that PAWSC depends on geological subsurface properties that determine how much water can be held against gravity drainage integrated over an area accessible by roots. In the California Sierra, water storage capacities range from negligible in areas where bedrock is close to the surface (Hahm et al., 2014) to areas where geophysical measurements show water storage capacities of >4 m (Holbrook et al., 2014). A second reason for such variation is that physiological controls on PAWSC properties also have substantial spatial heterogeneity within forest stands and across landscapes, due to species differences and plasticity of rooting characteristics within species (Schenk, 2006).
In order to establish a range of values for PAWSC for our study, we relied on recent geophysics measurement as well as water balance and flux tower observations at the Sierra Critical Zone Observatory (SCZO). This is a mid-elevation (1,790–2,117 m) conifer site located in the southwestern Sierra Nevada. SCZO measurements at this site indicate that roots have access to more than 4 m meters of water, and our mid-elevation site likely reflects water storages toward the higher end of possible ranges (Bales et al., 2011). For this paper we compared estimates using relatively deep subsurface storage similar to those at the Sierra CZO and results for relatively shallow soils. To determine storage and drainage parameter values for our deep subsurface storage scenario (DEEP) we selected parameters that gave RHESSys flux estimates that are similar to those measured at the SCZO. Figure 2 shows a comparison between observed and modeled water flux at the SCZO site, computed using a maximum rooting depth of 4 m, resulting in a PAWSC of approximately 2 m of water.
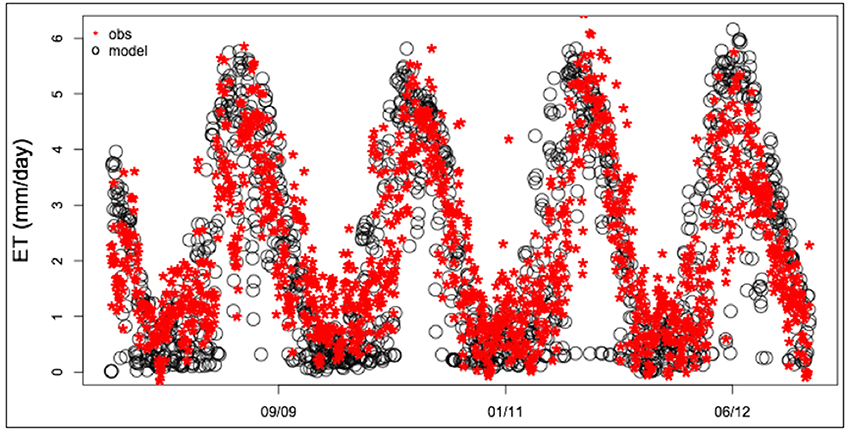
Figure 2. Flux tower observations [Mid-elevation (2,018 m) flux tower of Sierra Critical Zone Observatory] and RHESSys estimates of evapotranspiration.
To determine values for shallow storages, we note that observations in the Southern Sierra region include locations with essentially no soil, however these sites do not support forests (Hahm et al., 2014). To provide a shallow soil end-member scenario (SHALLOW), we used a maximum rooting depth that supplied sufficient water storage to support a conifer forest with a 30 m patch LAI of 2, which is toward the low end of conifer forest LAI in the water limited ranges of the Sierra. We used trial and error application of the RHESSys carbon cycling model to find the approximate minimum soil moisture storage capacity (PAWSC of 300 mm with a 0.5 m maximum rooting depth) that would support this forest. Finally we also test a midpoint PAWSC (MID) where maximum rooting depth is set to 1.0 m to represent forest stand conditions between SHALLOW and DEEP. We also note that although we generated these differences in PAWSC by varying the effective soil depth in the model, spatial differences in PAWSC may occur as a result of spatial differences in the water holding capacity of soil and weathered bedrock rather than differences in maximum possible rooting depth. Within RHESSYs, PAWSC is computed as the integration of field capacity above wilting point over the rooting depth. Thus, varying either the parameters that control soil storage or depth can be used to vary PAWSC, and DEEP vs. SHALLOW can be interpreted as modeling large vs. small PAWSC.
In assessing the impact of density reduction on forest water use, we also considered the spatial extent of subsurface interactions between neighboring trees as another potential determinant of eco-hydrologic response and another important source of uncertainty. This is because the trees remaining after a forest thinning treatment may be able to access the water that was previously used by competing vegetation. In water-limited environments some plants can be very deeply rooted (Hubbard et al., 2013) and the degree of sharing of plant available water storage may be particularly important. In general, the extent to which roots of spatially proximate individuals share water remains highly uncertain and depends on density, depth distribution of roots and mycorrhizal networks (Coomes and Grubb, 2008). While roots may be concentrated beneath the canopy, studies in water-limited environments have shown that roots from an individual plant can impact an area more than 10 times its crown area (Casper and Jackson, 1997). Root competition (or access to the same water) has also been shown to have high spatial heterogeneity (Schenk and Jackson, 2002; Casper et al., 2003).
To evaluate the implications of shared rooting zone water by neighboring trees, we recognize a continuum of competition and variation in the “belowground neighborhood” utilized by roots (Casper and Jackson, 1997). At the most isolated end of the spectrum, individual trees may have locally segregated root systems and largely avoid competition for water (Schenk, 2006). At some intermediate scale of competition, tree root systems might be deeper, wider, and overlap substantially with other neighboring trees; competition in this case might be experienced across multiple individuals in a given forest stand (e.g., Brassard et al., 2009). Positive effects of overlapping root systems might also be expected, due to hydraulic lift of water from lower to higher levels of soil (e.g., Dawson, 1993). At the most widely connected end of the spectrum, trees may be deeply and permanently rooted into groundwater sources [e.g., via cracks in weathered bedrock (Hubbard et al., 2013) and thus competing with other trees across wider spatial scales (Casper and Jackson, 1997)]. Here we consider the two finer scales of water access and root competition, namely LOCAL (i.e., trees are locally segregated and do not share water with neighbors) and DIFFUSE (i.e., interacting with nearest neighbors) scenarios. In the DIFFUSE scenario, all vegetation in a 30 m patch is assumed to share soil water. Thus, following density reduction, the remaining trees can access PAWSC that was previously used by the vegetation removed. The LOCAL scenario on the other hand assumes that forests in the vegetated area utilize local water stores and there is no competition for water among vegetation in the thinned area—either before or after thinning. We note that DIFFUSE and LOCAL are essentially hypothetical conditions that represent distinct scenarios. For a given thinning density, an actual thinning implementation for a given forest stand could instead lead to a scenario somewhere between DIFFUSE and LOCAL, and this will depend on species, size of trees removed, and the spatial pattern of tree removal within a stand that gives rise to the thinning density. Individual trees are not explicitly represented in RHESSys and our approach is thus to simply estimate upper and lower bounds on the amount of water sharing by using our DIFFUSE and LOCAL root sharing scenarios. We repeat DIFFUSE and LOCAL simulations for DEEP, MID, and SHALLOW soils.
To account for the impact of inter-annual climate variation on ecosystem responses we also varied the year in which the density reduction occurs from 1955 to 2015 in 5-year increments (Figure 3). Thus, we have 13 possible start dates followed by a 15-year recovery period. For simulations with start years 2010 and 2015 we recycle the historic record to allow for a full 15 year recovery (e.g., we extend the record by appending climate from 1955 and onward). While we could have used 2000 as the latest start year, we included these later years in order to capture impacts of thinning during the significant drought period between 2012 and 2015. We refer to these 13 time series of meteorological forcing data (a start date followed by 15 years of daily precipitation and minimum and maximum air temperature) as climate trajectories or climate scenarios. Thus, for each climate scenario, the meteorological forcing obtained in the nth year following thinning treatment will be different, and the distribution of changes in the nth year following thinning will reflect the influence of historic inter-annual climate variation. Figure 3 shows the sequence of annual precipitation for these different climate scenarios. Note that because continuous climate sequences are used there is substantial overlap in the 15 climate trajectories. While this means that replicates (e.g., each scenario of density reduction in initial year followed by 15 years of recovery) are not independent and partially share climate with other scenarios, we use this sampling approach because it maintains the multi-year structure of climate sequences (e.g., ENSO in western US). At the same time, we take full advantage of the available climate data to specifically quantify how timing of thinning relative to climate variability can influence ecohydrologic responses.
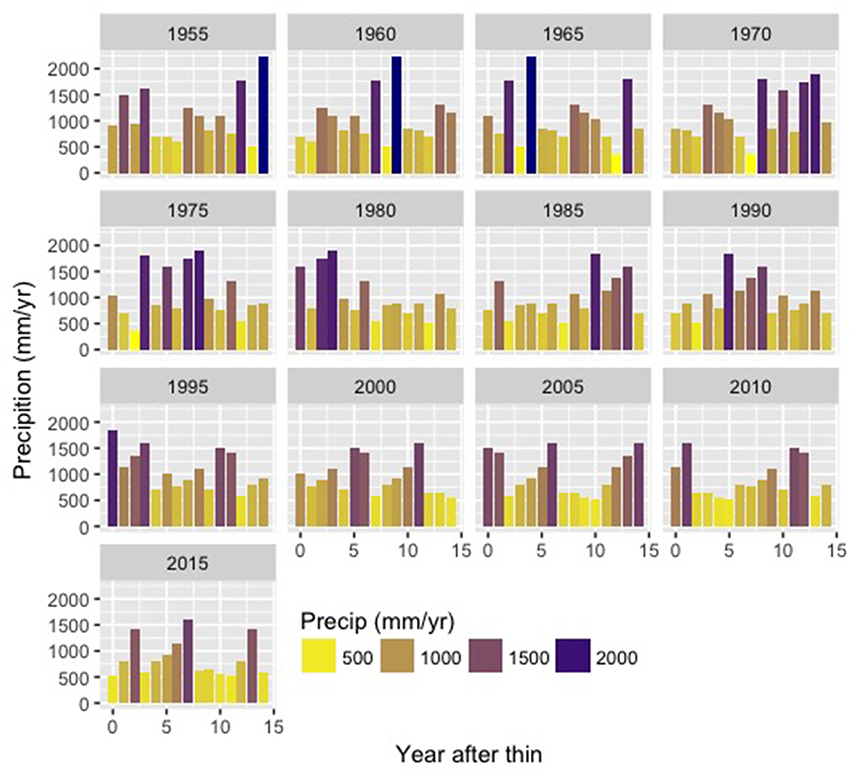
Figure 3. Annual precipitation (mm/yr) for each of the 13 climate trajectories. Labels give the start year of the climate sequence. Note that 2015 scenario “recycles” historic climate data to provide precipitation input for years following 2018.
In summary we ran 520 scenarios: DIFFUSE vs. LOCAL root competition, with either DEEP, MID, or SHALLOW PAWSC, for density reduction from 10 to 40%, computed for 13 different climate trajectories (Figure 4). Each scenario comprises 15 years of daily time step model runs. To examine the potential for thinning to reduce water stress in remaining trees, we compare model estimates of LAI, biomass and evapotranspiration at annual time scales across scenarios. These estimates simulate stand-level behavior and integrate both thinned and unthinned portions of the canopy. Precipitation in modeled scenarios varies between 364 and 2,234 mm/yr; thus simulations include both relatively dry and wet years. Even in high precipitation years, summer rainfall is typically very low, and forests in this region experience some degree of late summer water limitation in most years (Hanson and Weltzin, 2000). To highlight whether thinning is likely to reduce drought stress in the remaining trees, we also compare the net primary productivity (NPP) estimates for the remaining trees with NPP in the unthinned stand.
Results
Figure 5 illustrates the simulated LAI trajectories for unthinned forest stands given the 13 initial start dates and 15 years of recovery, for SHALLOW, MID, DEEP levels of PAWSC. Lines are averaged across all climate scenarios. LAI is greatest for DEEP and lowest for SHALLOW PAWSC, reflecting the influence of decreasing water availability with shallower soil water storage capacity. Note that prior to thinning, estimates of LAI, carbon stores (biomass), NPP and evapotranspiration also decrease with decreasing PAWSC (Table 1).
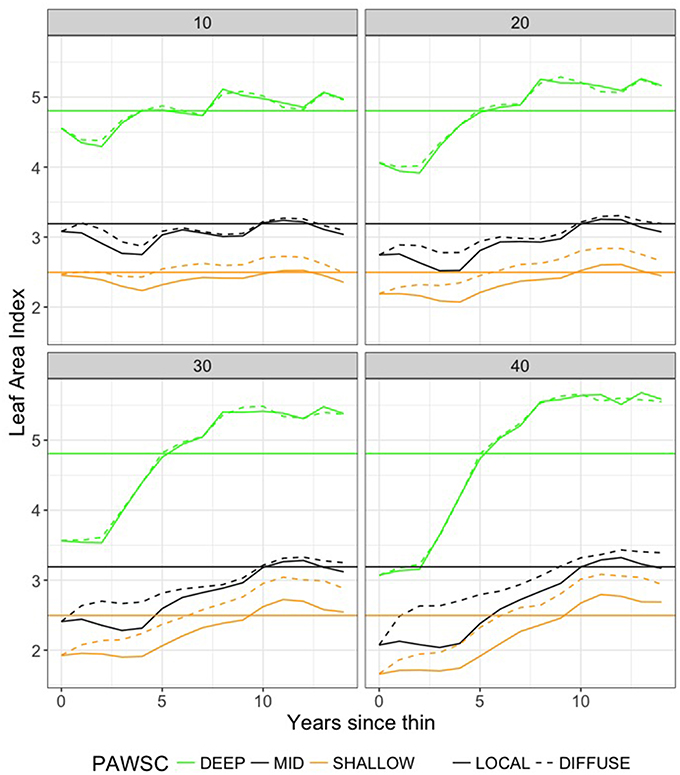
Figure 5. RHESSys estimates of forest recovery as LAI (leaf area index) following thinning. Results show average values across all thinning intensities and across the 13 climate trajectories. Plots compare recovery for DIFFUSE and LOCAL rooting zone water sharing (between thinned and remaining vegetation) and for forests with DEEP, MID, and SHALLOW PAWSC (plant available water storage capacity). Horizontal lines show pre-thinning mean values of LAI for each PAWSC scenario.
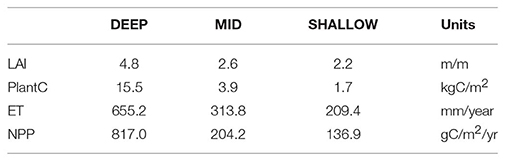
Table 1. Mean (across 13 start dates and 15 years of climate forcing) simulated values of leaf area index (LAI), total plant carbon storage (PlantC), evapotranspiration (ET), and net primary productivity (NPP) for baseline unthinned scenario.
For all PAWSC levels, LAI reaches pre-thinned values within the first decade following thinning and then often exceeds pre-thinned LAI. LAI becomes greater than pre-thinned values due to the additional growth of remaining trees, stimulated by additional water availability, as well as higher LAI in young re-growing vegetation. This is consistent with observations that find aggrading forests with slightly high LAI relative to older stands. Field-based studies often show peak LAI occurring early in forest regeneration (Landsberg and Waring, 1997).
There are substantial differences between root competition scenarios where we assume that remaining trees have access to the soil water previously utilized by the thinned biomass (DIFFUSE) and scenarios where we assume that this unused water cannot be accessed by remaining vegetation (LOCAL). For SHALLOW and MID levels of PAWSC, LAI recovers more quickly and reaches higher post-thinning values for the DIFFUSE rooting scenario. Thinning in these scenarios increases the productivity of remaining trees enough that the overall stand productivity actually exceeds pre-thinned levels. This effect suggests that thinning may help these more water-limited forests to achieve greater long-term productivity. However, although long-term average productivity and biomass is higher, the greater biomass associated with the thinned DIFFUSE scenarios can lead to greater water use and water stress and declines in productivity in low water years. We discuss NPP of remaining trees following thinning in more detail below.
LAI recovery also varies substantially across climate scenarios. Figure 6 summarizes variation due to climate in LAI recovery time. Vegetation in DEEP grows more quickly and thus LAI recovers earlier than for other PAWSC levels. Relative to MID and SHALLOW scenarios, the time required for LAI to recover to pre-thinned values for DEEP scenarios shows only small differences between DIFFUSE vs. LOCAL rooting scenarios; recovery times also vary less across climate scenarios than for shallower PAWSC. For MID and SHALLOW levels of PAWSC, timing of LAI recovery varies substantially with climate trajectories (box-widths), particularly for the DIFFUSE rooting scenarios. As noted above, LOCAL root competition scenarios take substantially longer to recover. Climate impacts on LAI recovery rate are greatest for MID levels of PAWSC and DIFFUSE rooting scenarios. In this case, the additional water released by thinning stimulates the growth of remaining trees; however, the amount of excess water and consequently regrowth rates varies substantially from year to year. Additional discussion of climate sensitivity is included in the discussion of ET below.
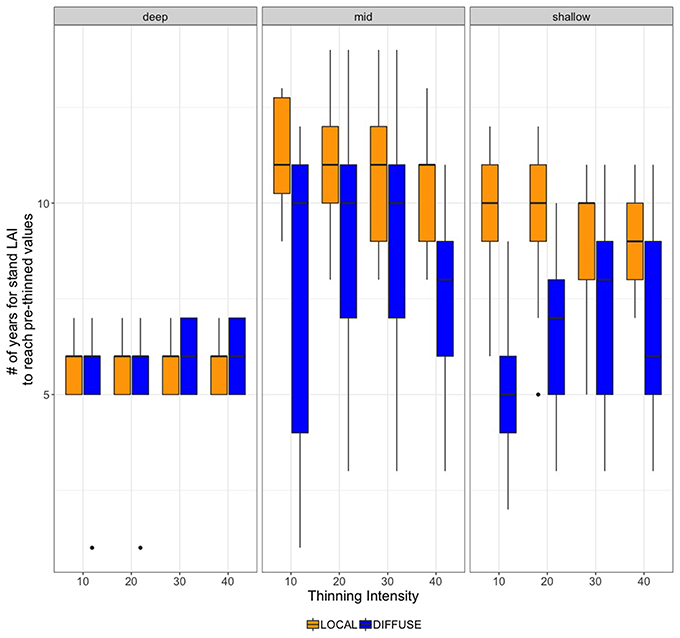
Figure 6. RHESSys estimates of the number of years required to reach pre-thinned LAI values for each thinning intensity scenario (as percent biomass removed) and for DEEP, MID and SHALLOW PAWSC and DIFFUSE and LOCAL rooting zone water.
Total biomass (as carbon) recovery follows a similar pattern to LAI, although the recovery is slower (not shown) such that none of the scenarios have returned to pre-thinning carbon stores by the 15th year following recovery. Table 2 summarizes final biomass as a percentage of pre-thinning values. As with LAI, the recovery of carbon storage is substantially faster for DIFFUSE root competition scenarios. For SHALLOW levels of PAWSC, for example, total carbon stores for the LOCAL rooting scenarios have recovered only to only 75% of pre-thinned values by the 15th year following thinning, while they are 86% for DIFFUSE rooting scenarios. Lowest recovery percentages occur for DEEP PAWSC scenarios. While productivity is greater when PAWSC is higher, DEEP scenarios have substantially greater initial above ground carbon stores (e.g., 15 kgC/m2 for DEEP vs. 3.9 and 1.7 kgC/m2 for MID and SHALLOW, Table 1), and since thinning intensities are based on a percentage, DEEP scenarios have substantially more carbon to recover.
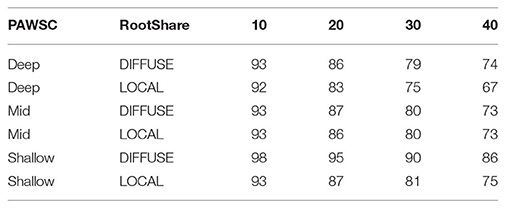
Table 2. Simulated carbon store (total biomass) by the 15th year following recovery as a percentage of pre-thinning values for all PAWSC (plant available water storage capacity) and root competition scenarios.
Stand-level water use generally follows LAI recovery, although water fluxes recover more quickly than LAI and carbon stores (Figures 7, 8). Note we show only results for 20 and 40% thinning intensities as lower thinning intensities show similar patterns; although the magnitude of changes in ET scale increases with thinning intensity (Figure 8). Thinning impacts on ET also increase with PAWSC. Note however that biomass also increases with PAWSC so this greater loss in ET reflects greater biomass reductions for a given thinning intensity. Recovery of water use generally occurs more quickly with greater subsurface storage (greater PAWSC).
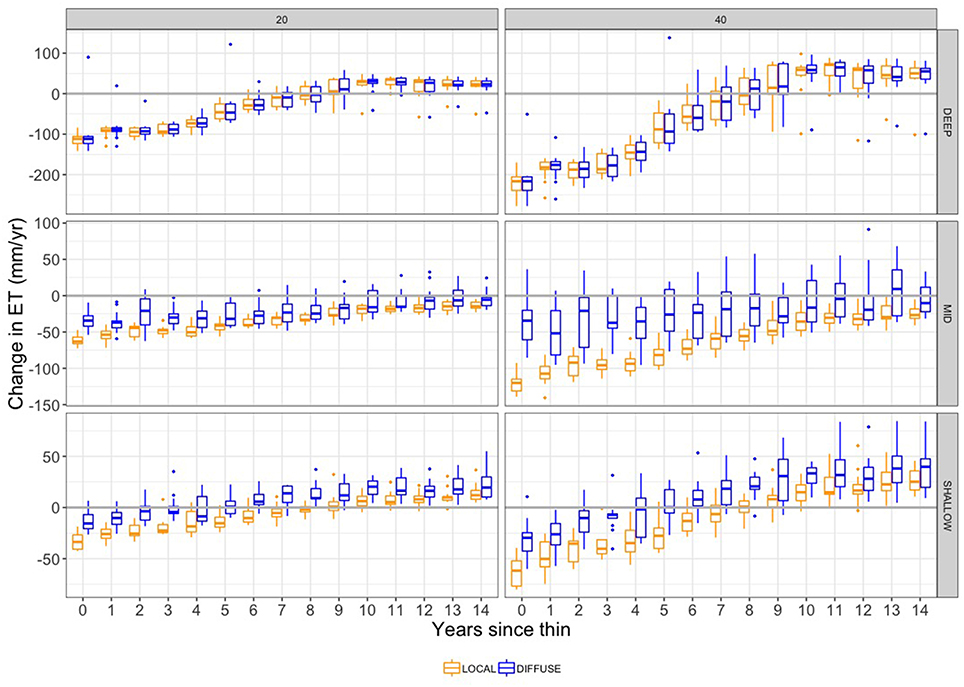
Figure 7. Change in annual evapotranspiration (ET) (mm/yr) due to thinning. Plots show difference in ET between thinned and unthinned control plots for DIFFUSE (blue) and LOCAL (orange) rooting zone water. Box-plot widths illustrate variation across the 13 climate trajectories. Separate plots are shown for two thinning intensities (20 and 40% biomass removal) and DEEP, MID, and SHALLOW PAWSC.
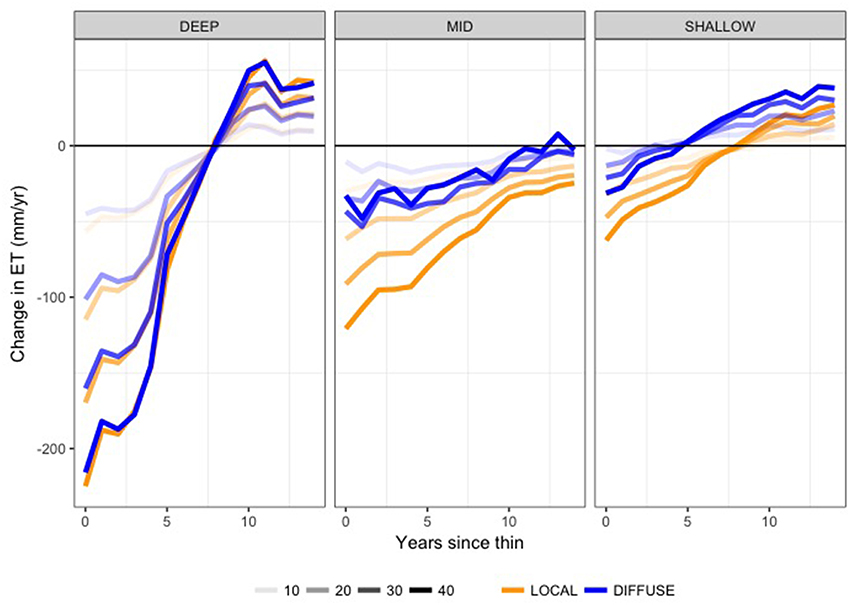
Figure 8. Mean change in annual evapotranspiration (mm/yr) due to thinning. Plots show mean difference in ET between thinned and unthinned control plot, averaged across climate trajectories for DIFFUSE (blue) and LOCAL (orange) rooting zone water. Line shading varies with thinning intensities (10, 20, 30, and 40% biomass removal) and separate plots show results for DEEP, MID, and SHALLOW PAWSC.
On average for scenarios with large subsurface storage (DEEP), evapotranspiration fluxes return to pre-thinned levels within the first 8 years (Figures 7, 8), although changes in ET vary substantially across the different climate trajectories (box-plot widths, Figure 7). Recall that each climate trajectory will result in a different meteorologic forcing for any given post-treatment year. Once initial recovery occurs, whether ET is greater or less than baseline un-thinned scenarios depends on this climate forcing. For example, for MID, DIFFUSE scenarios, the change in ET, in the second year following a 40% intensity thinning, ranges from −100 to +30 mm/yr depending on the climate forcing used. This climate sensitivity is particularly notable for higher thinning intensities and is greater for DIFFUSE root competition scenarios. Higher post-thinning ET corresponds with the greater LAI of the recovering forest, including greater LAI of younger trees and the enhanced growth of non-thinned trees.
For moderate available water capacity (MID), the DIFFUSE assumption leads to persistently greater ET (smaller declines relative to baseline) throughout the recovery scenario (Figure 8). For the DIFFUSE scenario, even in the year immediately following thinning, ET can sometimes be greater than in the pre-thinning scenarios. This somewhat counter-intuitive result is due to efficient water use by the remaining trees following thinning as well as greater evaporative fluxes from canopy gaps. For high thinning intensity, the greater water use of DIFFUSE rooting is substantial relative to LOCAL, although DIFFUSE also shows greater sensitivity to climate. SHALLOW PAWSC scenarios are similar to MID (Figure 7), with DIFFUSE root competition scenarios showing substantially greater ET; however, the additional water use with DIFFUSE is less.
Figure 9 compares the NPP estimates for the remaining trees with NPP in the unthinned stand. Here we show the DIFFUSE root competition scenario, since under a LOCAL scenario, neighboring trees would not be able to access additional water made available by thinning. In the first year following thinning, remaining trees have higher NPP than in the unthinned scenario. After 15 years of recovery, however, this effect is substantially reduced and distributions converge. Further, while for most years and thinning intensities NPP is greater following thinning, there are some cases, where NPP of remaining trees is less following thinning, particularly for MID and DEEP levels of PAWSC (Figure 9). A histogram of change in NPP for remaining trees across all thinning scenarios and all post-thinning years (Figure 10) shows that this decline in remaining tree NPP occurs most often in the MID PAWSC scenario. For MID, post-thinning NPP in remaining trees is at least 10% lower than un-thinned values in 25% of years. For DEEP and SHALLOW, this lower NPP occur in only 18 and 12% of years, respectively.
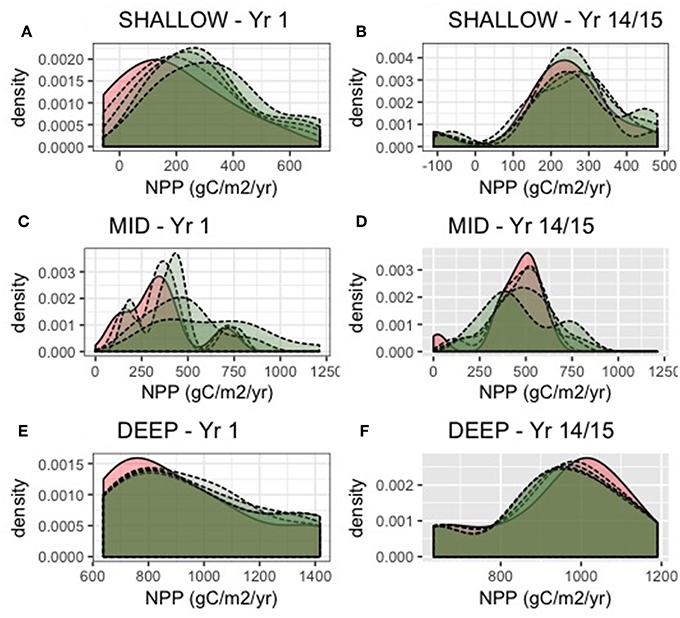
Figure 9. NPP (gC/m2/yr) for remaining trees for unthinned (solid line) and thinned (dotted line) scenarios. For the first year following thinning (A,C,E) and 14 years after regrowth (B,D,F), and for SHALLOW (A,B), MID (C,D), and DEEP (E,F) PAWSC. Distributions (density plot) reflect variation due to different climate trajectories and shading shows the level of thinning intensity (0–40%).
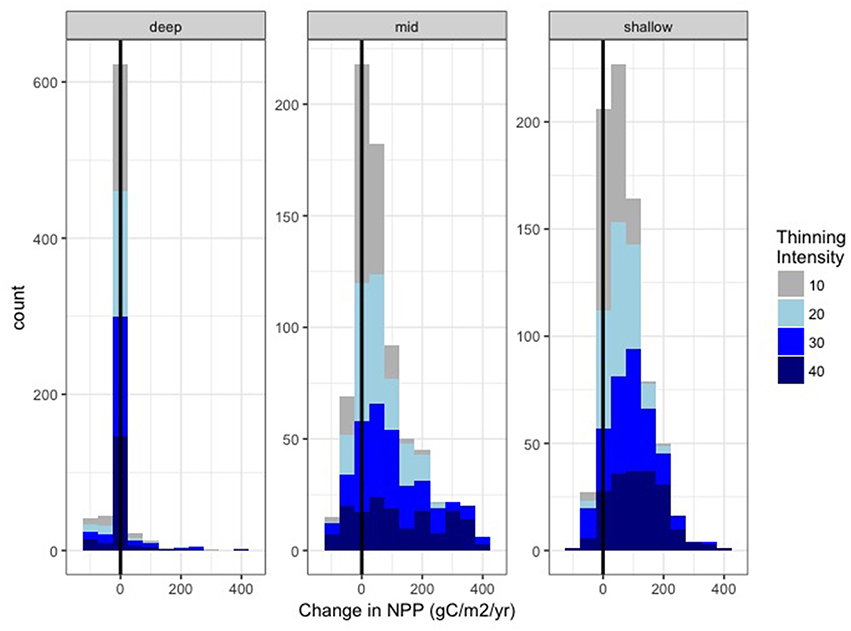
Figure 10. Histogram of the change in NPP (gC/m2/yr) for remaining trees, following thinning for all post-thinning recovery years and all climate trajectories. Colors show each level of thinning intensity (0–40%) and individual plots show results for DEEP, MID, and SHALLOW PAWSC.
Lower NPP in the remaining trees reflects the costs of stimulated growth in “good” years following thinning, which may lead to increased water stress in subsequent dry, low NPP years. For example, consider NPP for the climate trajectory that begins in 2005 and includes the 2013–2015 drought (Figure 3). In this scenario, the drought occurs more than 10 years after the initial thinning takes place. Figure 11 shows how thinning increases plant carbon by providing additional stimulating growth in remaining trees for the MID PAWSC scenario. Note also that this only occurs for the DIFFUSE rooting scenario where we assume that neighboring trees compete for water such that all trees share the same water stores. While thinning increases initial productivity in remaining trees, the resulting biomass accumulation allows for greater carbon losses as maintenance respiration during subsequent drought years. Particularly after several years of drought in 2014 and for the most intensive thinning scenarios, the additional biomass accumulation in remaining trees leads to substantially lower NPP in several of the drought years. For more moderate thinning scenarios, where additional growth in remaining trees is less, however, thinning still provides a benefit and maintains higher NPP during the drought relative to the unthinned case.
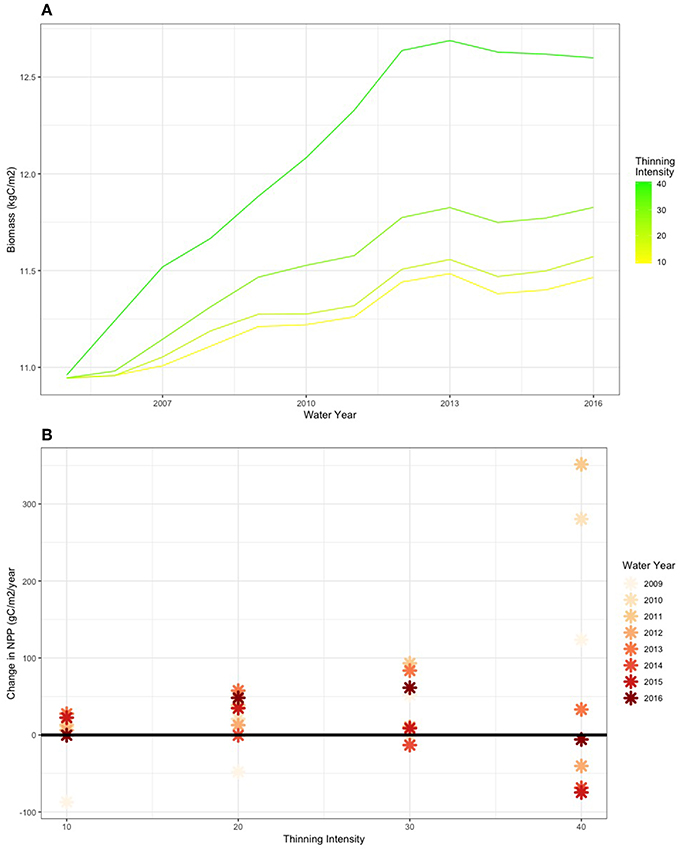
Figure 11. Biomass recovery (A) and change in NPP (B) for following thinning for the climate trajectory that begins in 2005 and include the 2013–2105 drought. Results are shown for the MID PAWSC scenarios.
Discussion
In this study, we evaluated the sensitivity of forest regrowth and water use across thinning intensity and inter-annual variation in climate using different assumptions about subsurface properties: high, med, and low plant available water storage capacity (DEEP, MID, and SHALLOW levels of PAWSC), and different assumptions about sharing of available water in the rooting zone (LOCAL and DIFFUSE competition). While results vary across thinning intensities and with inter-annual climate, the impact of subsurface properties is particularly striking. Although our findings simply provide estimates for end member scenarios, results highlight that details of soil water storage and root system competition can strongly influence whether forest water use increases or decreases within several years following recovery. Subsurface properties also substantially affect the length of time required for ecosystem services to return to pre-thinning levels. Results therefore provide upper and lower bounds on potential thinning responses and highlight critical information needed to reduce uncertainty. The range of modeled responses shown here also contributes to a mechanistic explanation for the diversity of field-based eco-hydrologic responses to thinning, which include both increased and decreased forest water use and varying vulnerability to drought stress (Clark et al., 2016; Hessburg et al., 2016; Tague et al., 2019).
Ecosystem Service Impacts
Net primary productivity and its accumulation as biomass or sequestered carbon are important measures of ecosystem services. While the relationship between forest productivity and health is a complex one, forest productivity is commonly used in inventories of forest health, and changes in productivity may have implications for habitat quality and carbon storage (O'Laughlin and Cook, 2003). Consistent with other field and modeling studies, our results show that initially site carbon storage and productivity are reduced following thinning, generally proportionally with thinning intensity (Campbell et al., 2011). Our results, however, demonstrate that for forests like those in the water-limited Sierra, the time period of recovery is much faster if roots are assumed to strongly compete with neighboring trees for water (DIFFUSE) or for the scenarios where soils have low water storage capacity (SHALLOW), because the remaining trees quickly become more effective users of infiltrated water. Further, our results suggest that the relationship between thinning intensity and the magnitude of changes to water use or carbon-related variables is not always linear. Thus, a more nuanced approach is needed when assessing tradeoffs associated with the relative costs of different thinning intensities and expected impacts to water and carbon.
Perhaps the most interesting case is where total LAI increases to values greater than pre-thinning levels. For the DIFFUSE, MID, and SHALLOW PAWSC scenarios, post-thinning LAI is greater than pre-thinning biomass within several years following thinning. This possibly counter-intuitive result reflects “competitive release” through thinning and a more efficient use of resources in water-limited environments. When this water is shared among fewer individuals, the resulting higher NPP supports the development of larger trees, with more photosynthetic capacity, more root biomass and greater storage. During years of at least average precipitation and evaporative demand, the increased productivity in this scenario can represent a “win-win” situation. These model-based results are consistent with some field-based observations and theory from other semi-arid environments (Clark et al., 2016).
How much water plants have access to and ultimately use is also an important ecosystem function. Years when plant actual evapotranspiration is relatively high are typically associated with high rates of productivity (Stephenson, 1998). On the other hand, reduced water use by forests can increase groundwater recharge and in some cases increase streamflow, thus providing ecosystem service-related benefits for downstream users. Our estimates of net water use by forests, however, point to a range of responses to thinning. Many, but not all, of our scenarios initially following thinning reflect conventional thinking that forest water use declines with thinning (and runoff production increases); although, for the SHALLOW PAWSC scenario with DIFFUSE rooting, forest water use following thinning can actually increases in some years. However, for all soils, as the forest recovers, our model ultimately shows potential increases in longer term stand scale water use due to stimulated growth following thinning.
In terms of thinning to promote drought resilience, we also find that some counter-intuitive responses are possible. For example, we show that the greater biomass and productivity supported by thinning can ultimately, in some cases, lead to drought related declines in NPP. The increase in biomass following thinning can become “too much of a good thing” in drier years when water to support the increased biomass is not available. In these cases, our results show how lower NPP can occur within 15 years of thinning. This is consistent with studies in semi-arid Southwest, which found that thinning led to increased leaf-to-sapwood area in remaining trees and increased tree water demand, ultimately increasing vulnerability to subsequent drought stress (McDowell et al., 2006). More generally our “too much of a good thing” result from thinning is an example of “structural overshoot” where growth during favorable conditions can increase drought vulnerability (Jump et al., 2017). Note that we use low NPP here as an indicator of water stress. Future work will link this NPP to other physiological indicators of drought response and examine response to more severe drought conditions. Regardless, these findings suggest that a broad conclusion that thinning will decrease forest water use and water stress is an over-simplification, and whether or not these “benefits” occur and for how long will depend strongly on subsurface characteristics and future climate specifics.
These results emphasize that properties of the subsurface environment can be a first order, dominant control on the impact of thinning on both water use and ecosystem services related to forest productivity and biomass accumulation. We note that this study focused on a single patch, thus did not evaluate whether excess runoff reaches downslope stream networks or is instead used by downslope vegetation. Recent work highlights the importance of lateral subsidies for forest water use in water limited environments (Thompson et al., 2011; Brooks et al., 2015). Water use in the DIFFUSE root competition scenario reflects shared water use by spatially disparate trees but we assume that there is no delay in transport of water between roots. The contrast between DIFFUSE and LOCAL scenarios shown here is an oversimplified dichotomy, and it is likely that most situations will fall along a gradient in spatial root competition. In addition, even though competition between shallow rooted smaller trees and understory vegetation may be more consistent with the DIFFUSE scenario, competition with large and deeply rooted overstory trees may be more consistent with a LOCAL characterization. Root distribution strategies are also likely to vary by species and local soil and geologic conditions (Brassard et al., 2009), and the time taken for roots to extend in to the “water space” of neighboring trees may also vary with gap size, site conditions and species (Parsons et al., 2011). Similarly our definition of DEEP vs. SHALLOW soil PAWSC may reflect a range of possible conditions. While here we associate DEEP-MID-SHALLOW with the actual depth of soil, PAWSC may often depend on spatial differences in the water holding capacities of soil rather than actual depth. Further work will explore this sharing of water through hillslope transport and intermediate, time varying degrees of sharing of water by roots. Nonetheless our results show the importance of vertical storage or PAWSC.
Capturing spatial heterogeneity and parameterization of PAWSC within models is challenging. PAWSC and the extent to which roots in neighboring trees share water are both essentially a function of geologically mediated soil and saprolite storage properties and eco-physiologic controls on root distributions. High spatial heterogeneity in plant available water storage is well-documented at plot to regional scales (McNamara et al., 2011). While spatial information on soil properties, such as STATSGO, are available, the information is rarely sufficient to characterize PAWSC; consequently most hydrologic models still require calibration of subsurface properties to match observed streamflow or water flux data. Similarly, within and between species differences in how much carbon is allocated to roots, and how root growth responds to plant environments, remains highly uncertain (Litton et al., 2007) but is likely to show substantial spatial heterogeneity at local to watershed scales. To support local decision making around thinning strategies, constraining these parameters may be critical.
There are a number of ways that we can address the issue of uncertainty in PAWSC. The high sensitivity to PAWSC parameters (DEEP vs. MID vs. SHALLOW) suggests that pre-thinning ecosystem productivity, particularly in drier years, can be used to infer possible ranges for PAWSC. Strategic calibration of PAWSC parameters in models, such as RHESSys, using measurements ranging from remote sensing of NPP, tree rings or flux towers, can be used to reduce uncertainty in PAWSC (Tague, 2009; Klein et al., 2013). Recent advances in geophysical instrumentation may also provide data that can help to identify spatial patterns of water storage within and between watersheds (Holbrook et al., 2014). Determining the extent of sharing of root zone soil water (along the LOCAL to DIFFUSE gradient), across a range of thinning intensities is likely to be more challenging. The extent of DIFFUSE water availability after thinning will likely depend on spatial configuration of treatments, as well as species, size and other properties of both removed and remaining vegetation. Further research, where post-thinning trees are monitored, is needed to begin to develop generalizable principles.
Management Considerations
This study also quantified the substantial impact of inter-annual variation in air temperature and precipitation patterns on thinning responses for a water-limited forest. Climate variation at such sites is substantial, with mean annual precipitation varying from 360 to over 2,000 mm/yr and mean annual minimum and maximum daily temperatures ranging from 4 to 7°C and 11 to 15°C, respectively. This high inter-annual climate variability, as well as the seasonality of precipitation, is common to many Mediterranean snow-dominated regions. Thus, results demonstrate that the timing of a thinning event and precipitation and temperature patterns in post-thinning years can strongly influence ecohydrologic impacts of thinning. Climate sensitivity was substantial for all scenarios. For example, the impact of thinning in the SHALLOW-DIFFUSE combination, even after 5 years of recovery, ranges from climate scenarios where productivity and water use increase to those where productivity and water use decline relative to pre-thinning conditions. We note that in the first year the impact on climate variation on water fluxes is substantial for all soils and for most thinning intensities. Field observations of thinning effects are often limited to measuring responses following a thinning event in a single year, within a particular climate sequence following that event. These results demonstrate some of the challenges inherent in extrapolating from observations taken from single thinning events.
Although fire hazard reduction was not the explicit focus of our study, some results are worth noting. Because the different thinning treatments did not target specific diameter classes of fuels—simple percent biomass removal scenarios were examined instead—it is difficult to comment on the lifespan of treatment effectiveness (i.e., how long before surface fuels build up again). Even so, it is notable that temporal trends in a measure like LAI, which may be a good surrogate for the accumulation of fine fuels (i.e., leaves and small twigs), and NPP, which is a good indicator of water stress, show a strong sensitivity to assumptions about PAWSC and the degree of root competition for water. Although future work is needed to examine flammability traits like live fuel moisture, it is clear that these characteristics will also depend on subsurface properties that are not often considered.
Conclusions
Forest density reduction is one of the strategies available to forest managers to maintain healthy forests. Density reduction may be motivated by a range of objectives, including reducing fire risk, climate change adaptation, water management and reducing the risks associated with other drought-related disturbances (e.g., beetle infestation). To strategically allocate limited resources, it is necessary to understand the likelihood of meeting these objectives, given a density reduction prescription that is to be implemented in a particular place and time. Here we provide estimates of changes in forest water use, biomass, productivity, and leaf area following thinning and for a 15-year recovery trajectory. Our estimates for a representative mid-elevation site within the California Sierra near the rain-snow transition line provide insights into how local subsurface properties determine forest access to water and substantially influence thinning responses. The degree to which trees compete for water and gradients in soil water storage capacity control not only the magnitude of changes in water use, water stress, and carbon sequestration, but even whether these fluxes increase or decrease. Of particular interest are scenarios where low plant available water storage capacity and a high degree of interaction in the root systems of neighboring trees can lead to a situation where net stand water use actually increases during stand recovery, relative to baseline untreated conditions. In this scenario, the biomass of remaining trees increases as these trees access surplus water in wet years. This increased growth, however, can increase overall ecosystem water use and lead to larger trees that may experience greater water stress in subsequent drier years—a “too much of a good thing” consequence. Results also show how inter-annual climate variation plays a key role in determining forest water use changes in the years following recovery, and for more shallow soil sites, long-term recovery trajectories overall. While our work focuses on the possible range of responses to thinning scenarios for a particular representative site, the methods presented here can be generalized to apply to many water-limited forests. Sensitivity analysis using ecohydrologic models can also be used as a tool for interpreting results from field experiments, guiding future data collection and developing applied forest management strategies in a changing climate.
Data Availability
The datasets generated for this study are available on request to the corresponding author.
Author Contributions
CT lead the development of the research design, model implementation, analysis, and manuscript writing. MM contributed to research design, analysis of model results, and manuscript writing.
Funding
This research was supported by funding from National Science Foundation [Hazard SEES program (Grant number: 094878394)]: Land Management Strategies for Confronting Risks and Consequences of Wildfire (EAR-1520847) and the Southern Sierra Critical Zone Observatory (NSF; Grant numbers: EAR-0725097, 1331939).
Conflict of Interest Statement
The authors declare that the research was conducted in the absence of any commercial or financial relationships that could be construed as a potential conflict of interest.
Acknowledgments
Authors thank Janet Choate for contributions to figures.
References
Adams, H. D., Luce, C. H., Breshears, D. D., Allen, C. D., Weiler, M., Hale, V. C., et al. (2012). Ecohydrological consequences of drought- and infestation- triggered tree die-off: insights and hypotheses. Ecohydrology 5, 145–159. doi: 10.1002/eco.233
Anderson, S. P., Bales, R. C., and Duffy, C. J. (2008). Critical zone observatories: building a network to advance interdisciplinary study of Earth surface processes. Mineral. Mag. 72, 7–10. doi: 10.1180/minmag.2008.072.1.7
Asbjornsen, H., Goldsmith, G. R., Alvarado-Barrientos, M. S., Rebel, K., Osch, F. P. V., Rietkerk, M., et al. (2011). Ecohydrological advances and applications in plant–water relations research: a review. J. Plant Ecol. 4, 3–22. doi: 10.1093/jpe/rtr005
Ayres, A., Degolia, A., Fienup, M., Kim, Y., Sainz, J., Urbisci, L., et al. (2016). Social science/natural science perspectives on wildfire and climate change. Geogr. Compass 10, 67–86. doi: 10.1111/gec3.12259
Balandier, P., Collet, C., Miller, J. H., Reynolds, P. E., and Zedaker, S. M. (2006). Designing forest vegetation management strategies based on the mechanisms and dynamics of crop tree competition by neighbouring vegetation. Forestry 79, 3–27. doi: 10.1093/forestry/cpi056
Bales, R. C., Hopmans, J. W., O'Geen, A. T., Meadows, M., Hartsough, P. C., Kirchner, P., et al. (2011). Soil moisture response to snowmelt and rainfall in a Sierra Nevada mixed-conifer forest. Vadose Zone J. 10, 786–799. doi: 10.2136/vzj2011.0001
Biederman, J. A., Harpold, A. A., Gochis, D. J., Ewers, B. E., Reed, D. E., Papuga, S. A., et al. (2014). Increased evaporation following widespread tree mortality limits streamflow response. Water Resour. Res. 50, 5395–5409. doi: 10.1002/2013WR014994
Brassard, B. W., Chen, H. Y. H., and Bergeron, Y. (2009). Influence of environmental variability on root dynamics in northern forests. Critic. Rev. Plant Sci. 28, 179–197. doi: 10.1080/07352680902776572
Brooks, P. D., Chorover, J., Fan, Y., Godsey, S. E., Maxwell, R. M., McNamara, J. P., et al. (2015). Hydrological partitioning in the critical zone: recent advances and opportunities for developing transferable understanding of water cycle dynamics. Water Resour. Res. 51, 6973–6987. doi: 10.1002/2015WR017039
Campbell, J. L., Harmon, M. E., and Mitchell, S. R. (2011). Can fuel-reduction treatments really increase forest carbon storage in the western US by reducing future fire emissions? Front. Ecol. Environ. 10, 83–90. doi: 10.1890/110057
Casper, B. B., and Jackson, R. B. (1997). Plant competition underground. Annu. Rev. Ecol. Syst. 28, 545–570, doi: 10.1146/annurev.ecolsys.28.1.545
Casper, B. B., Schenk, H. J., and Jackson, R. B. (2003). Defining a plant's belowground zone of influence. Ecology 84, 2313–2321. doi: 10.1890/02-0287
Clark, J. S., Iverson, L., Woodall, C. W., Allen, C. D., Bell, D. M., Bragg, D. C., et al. (2016). The impacts of increasing drought on forest dynamics, structure, and biodiversity in the United States. Glob. Chang. Biol. 22, 2329–2352. doi: 10.1111/gcb.13160
Coomes, D. A., and Grubb, P. J. (2008). Impacts of root competition in forests and Woodlands: a theoretical framework and review of experiments. Ecol. Monogr. 70, 171–207. doi: 10.1890/0012-9615(2000)070[0171:IORCIF]2.0.CO;2
Dai, A. (2011). Drought under global warming: a review. Wiley Interdisc. Rev. Clim. Change 2, 45–65. doi: 10.1002/wcc.81
Dale, V. H., Joyce, L. A., McNulty, S., Neilson, R. P., Ayres, M. P., Flannigan, M. D., et al. (2001). Climate change and forest disturbances. Bioscience 51, 723–734. doi: 10.1641/0006-3568(2001)051[0723:CCAFD]2.0.CO;2
D'Amato, A. W., Bradford, J. B., Fraver, S., and Palik, B. J. (2013). Effects of thinning on drought vulnerability and climate response in north temperate forest ecosystems. Ecol. Appl. 23, 1735–1742. doi: 10.1890/13-0677.1
Dawson, T. E. (1993). Hydraulic lift and water use by plants: implications for water balance, performance and plant-plant interactions. Oecologia 95, 565–574. doi: 10.1007/BF00317442
Dennison, P. E., Brewer, S. C., Arnold, J. D., and Moritz, M. A. (2014). Large wildfire trends in the western United States, 1984–2011. Geophys. Res. Lett. 41:2014GL059576. doi: 10.1002/2014GL059576
Dore, S., Montes-Helu, M., Hart, S. C., Hungate, B. A., Koch, G. W., Moon, J. B., et al. (2012). Recovery of ponderosa pine ecosystem carbon and water fluxes from thinning and stand-replacing fire. Glob. Chang. Biol. 18, 3171–3185. doi: 10.1111/j.1365-2486.2012.02775.x
Downing, J. (2015). Forest thinning may increase water yield from the Sierra Nevada. Calif. Agric. 69, 10–11. doi: 10.3733/ca.v069n01p10
Edmonds, R. L. (1991). “Organic matter decomposition in western United States forests,” in Proceedings—Management and Productivity of Western-Montane Forest Soils, eds A. E. Harvey and L. F. Neuenschwander (USDA Forest Service General Technical Report INT-280). Ogden, UT: Intermountain Research Station, 118–128.
Elkin, C., Giuggiola, A., Rigling, A., and Bugmann, H. (2015). Short- and long-term efficacy of forest thinning to mitigate drought impacts in mountain forests in the European Alps. Ecol. Appl. 25, 1083–1098. doi: 10.1890/14-0690.1
Farquhar, G. D., and Von Caemmerer, S. (1982). “Modelling of photosynthetic response to environmental conditions,” in Physiological Plant Ecology II, eds O. L. Lange, P. S. Nobel, C. B. Osmond, and H. Ziegler (Berlin; Heidelberg: Springer), 549–587. doi: 10.1007/978-3-642-68150-9_17
Garcia, E. S., Tague, C. L., and Choate, J. S. (2016). Uncertainty in carbon allocation strategy and ecophysiological parameterization influences on carbon and streamflow estimates for two western US forested watersheds. Ecol. Modell. 342, 19–33. doi: 10.1016/j.ecolmodel.2016.09.021
Grant, G. E., Tague, C. L., and Allen, C. D. (2013). Watering the forest for the trees: an emerging priority for managing water in forest landscapes. Front. Ecol. Environ. 11, 314–321. doi: 10.1890/120209
Hahm, W. J., Riebe, C. S., Lukens, C. E., and Araki, S. (2014). Bedrock composition regulates mountain ecosystems and landscape evolution. Proc. Natl. Acad. Sci. U.S.A. 111, 3338–3343. doi: 10.1073/pnas.1315667111
Halofsky, J. S., Halofsky, J. E., Burcsu, T., and Hemstrom, M. A. (2014). Dry forest resilience varies under simulated climate-management scenarios in a central Oregon, USA landscape. Ecol. Appl. 24, 1908–1925. doi: 10.1890/13-1653.1
Hanson, P. J., and Weltzin, J. F. (2000). Drought disturbance from climate change: response of United States forests. Sci. Total Environ. 262, 205–220. doi: 10.1016/S0048-9697(00)00523-4
Hessburg, P. F., Spies, T. A., Perry, D. A., Skinner, C. N., Taylor, A. H., Brown, P. M., et al. (2016). Tamm review: management of mixed-severity fire regime forests in Oregon, Washington, and Northern California. For. Ecol. Manage. 366, 221–250. doi: 10.1016/j.foreco.2016.01.034
Holbrook, W. S., Riebe, C. S., Elwaseif, M. L., Hayes, J., Basler-Reeder, K. L., Harry, D. L., Hopmans, et al. (2014). Geophysical constraints on deep weathering and water storage potential in the Southern Sierra Critical Zone Observatory. Earth Surface Proc. Landforms 39, 366–380. doi: 10.1002/esp.3502
Howitt, R., Medellín-Azuara, J., and MacEwan, D. (2014). Economic analysis of the 2014 drought for California agriculture, report UC Davis. Davis, CA: Center for Watershed Sciences.
Hubbard, R. M., Rhoades, C. C., Elder, K., and Negron, J. (2013). Changes in transpiration and foliage growth in lodgepole pine trees following mountain pine beetle attack and mechanical girdling. For. Ecol. Manage. 289, 312–317. doi: 10.1016/j.foreco.2012.09.028
Ice, G. G., Neary, D. G., and Adams, P. W. (2004). Effects of wildfire on soils and watershed processes. J. For. 102, 16–20.
Jarvis, P. G. (1976). The interpretation of the variations in leaf water potential and stomatal conductance found in canopies in the field. Philosop. Trans. R. Soc. Lon. Ser. B 273, 593– 610. doi: 10.1098/rstb.1976.0035
Jump, A. S., Ruiz-Benito, P., Greenwood, S., Allen, C. D., Kitzberger, T., Fensham, R., et al. (2017). Structural overshoot of tree growth with climate variability and the global spectrum of drought-induced forest dieback. Glob. Chang. Biol. 23, 3742–3757. doi: 10.1111/gcb.13636
Klein, T., Shpringer, I., Fikler, B., Elbaz, G., Cohen, S., and Yakir, D. (2013). Relationships between stomatal regulation, water-use, and water-use efficiency of two coexisting key Mediterranean tree species. For. Ecol. Manage. 302, 34–42. doi: 10.1016/j.foreco.2013.03.044
Landsberg, J. J., and Waring, R. H. (1997). A generalised model of forest productivity using simplified concepts of radiation-use efficiency, carbon balance and partitioning. For. Ecol. Manage. 95, 209–228. doi: 10.1016/S0378-1127(97)00026-1
Law, B. E., Sun, O. J., Campbell, J., Van Tuyl, S., and Thornton, P. E. (2003). Changes in carbon storage and fluxes in a chronosequence of ponderosa pine. Glob. Chang. Biol. 9, 510–524. doi: 10.1046/j.1365-2486.2003.00624.x
Littell, J. S., Peterson, D. L., Riley, K. L., and Liu, Y. (2016). A review of the relationships between drought and forest fire in the United States. Glob. Change Biol. 10. 22, 2353–2369. doi: 10.1111/gcb.13275
Litton, C. M., Raich, J. W., and Ryan, M. G. (2007). Carbon allocation in forest ecosystems. Glob. Chang. Biol. 13, 2089–2109. doi: 10.1111/j.1365-2486.2007.01420.x
McDowell, N. G., Adams, H. D., Bailey, J. D., Hess, M., and Kolb, T. E. (2006). Homeostatic maintenance of ponderosa pine gas exchange in response to stand density changes. Ecol. Appl. 16, 1164–1182. doi: 10.1890/1051-0761(2006)016[1164:HMOPPG]2.0.CO;2
McNamara, J. P., Tetzlaff, D., Bishop, K., Soulsby, C., Seyfried, M., Peters, N. E., et al. (2011). Storage as a metric of catchment comparison. Hydrol. Process. 25, 3364–3371. doi: 10.1002/hyp.8113
Monteith, J. L., Szeicz, G., and Waggoner, P. E. (1965). The measurement and control of stomatal resistance in the field. J. Appl. Ecol. 2:345. doi: 10.2307/2401484
Moritz, M. A., Batllori, E., Bradstock, R. A., Gill, A. M., Handmer, J., Hessburg, P. F., et al. (2014). Learning to coexist with wildfire. Nature 515, 58–66. doi: 10.1038/nature13946
Noss, R. F., Franklin, J. F., Baker, W. L., Schoennagel, T., and Moyle, P. B. (2008). Managing fire-prone forests in the western United States. Front. Ecol. Environ. 4, 481–487. doi: 10.1890/1540-9295(2006)4[481:MFFITW]2.0.CO;2
O'Laughlin, J., and Cook, P. S. (2003). Inventory-based forest health indicators: implications for National Forest Management. J. For. 101, 11–17. doi: 10.1093/jof/101.2.11
Parsons, W. F., Miller, S. L., and Knight, D. H. (2011). Root-gap dynamics in a lodgepole pine forest: ectomycorrhizal and nonmycorrhizal fine root activity after experimental gap formation. Can. J. For. Res. 24, 1531–1538. doi: 10.1139/x94-200
Ryan, M. G. (1995). Foliar maintenance respiration of subalpine and boreal trees and shrubs in relation to nitrogen content. Plant Cell Environ. 18, 765–772. doi: 10.1111/j.1365-3040.1995.tb00579.x
Safford, H. D., Stevens, J. T., Merriam, K., Meyer, M. D., and Latimer, A. M. (2012). Fuel treatment effectiveness in California yellow pine and mixed conifer forests. For. Ecol. Manage. 274, 17–28. doi: 10.1016/j.foreco.2012.02.013
Saksa, P. C., Conklin, M. H., Battles, J. J., Tague, C. L., and Bales, R. C. (2017). Forest thinning impacts on the water balance of Sierra Nevada mixed-conifer headwater basins. Water Resour. Res. 53, 5364–5381. doi: 10.1002/2016WR019240
Schenk, H. J. (2006). Root competition: beyond resource depletion. J. Ecol. 94, 725–739. doi: 10.1111/j.1365-2745.2006.01124.x
Schenk, H. J., and Jackson, R. B. (2002). Rooting depths, lateral root spreads and below-ground/above-ground allometries of plants in water-limited ecosystems. J. Ecol. 90, 480–494. doi: 10.1046/j.1365-2745.2002.00682.x
Seidl, R., Fernandes, P. M., Fonseca, T. F., Gillet, F., Jönsson, A. M., Merganičová, K., et al. (2011). Modelling natural disturbances in forest ecosystems: a review. Ecol. Model. 222, 903–924. doi: 10.1016/j.ecolmodel.2010.09.040
Simonin, K., Kolb, T. E., Montes-Helu, M., and Koch, G. W. (2007). The influence of thinning on components of stand water balance in a ponderosa pine forest stand during and after extreme drought. Agric. For. Meteorol. 143, 266–276. doi: 10.1016/j.agrformet.2007.01.003
Stephens, S. L., McIver, J. D., Boerner, R. E. J., Fettig, C. J., Fontaine, J. B., Hartsough, B. R., et al. (2012). The effects of forest fuel-reduction treatments in the United States. Bio Sci. 62, 549–560. doi: 10.1525/bio.2012.62.6.6
Stephenson, N. (1998). Actual evapotranspiration and deficit: biologically meaningful correlates of vegetation distribution across spatial scales. J. Biogeogr. 25, 855–870. doi: 10.1046/j.1365-2699.1998.00233.x
Tague, C., and Grant, G. E. (2004). A geological framework for interpreting the low-flow regimes of Cascade streams, Willamette River Basin, Oregon. Water Resour. Res. 40, 1–9. doi: 10.1029/2003WR002629
Tague, C., and Peng, H. (2013). The sensitivity of forest water use to the timing of precipitation and snowmelt recharge in the California Sierra: implications for a warming climate. J. Geophys. Res. Biogeosci. 118, 875–887. doi: 10.1002/jgrg.20073
Tague, C. L. (2009). Assessing climate change impacts on alpine stream-flow and vegetation water use: mining the linkages with subsurface hydrologic processes. Hydrol. Proc. 23, 1815–1819. doi: 10.1002/hyp.7288
Tague, C. L., and Band, L. E. (2004). RHESSys: regional hydro-ecologic simulation system-an object-oriented approach to spatially distributed modeling of carbon, water, and nutrient cycling. Earth Interact. 8, 1–42. doi: 10.1175/1087-3562(2004)8<1:RRHSSO>2.0.CO;2
Tague, C. L., McDowell, N. G., and Allen, C. D. (2013). An integrated model of environmental effects on growth, carbohydrate balance, and mortality of pinus ponderosa forests in the Southern Rocky Mountains. PLoS ONE 8:e80286. doi: 10.1371/journal.pone.0080286
Tague, C. L., Moritz, M., and Hanan, E. (2019). The changing water cycle: the eco-hydrologic impacts of forest density reduction in Mediterranean (seasonally dry) regions. WIREs Water 6:e1350. doi: 10.1002/wat2.1350
Thompson, S. E., Harman, C. J., Troch, P. A., Brooks, P. D., and Sivapalan, M. (2011). Spatial scale dependence of ecohydrologically mediated water balance partitioning: a synthesis framework for catchment ecohydrology. Water Resour. Res. 47, 1–20. doi: 10.1029/2010WR009998
Trumbore, S., Brando, P., and Hartmann, H. (2015). Forest health and global change. Science 349, 814–818. doi: 10.1126/science.aac6759
van Mantgem, P. J., and Stephenson, N. L. (2007). Apparent climatically induced increase of tree mortality rates in a temperate forest. Ecol. Lett. 10, 909–916. doi: 10.1111/j.1461-0248.2007.01080.x
Vicente-Serrano, S. M. (2007). Evaluating the impact of drought using remote sensing in a Mediterranean, Semi-arid region. Natural Hazards 40, 173–208. doi: 10.1007/s11069-006-0009-7
Westerling, A. L., and Bryant, B. P. (2008). Climate change and wildfire in California. Clim. Change 87, 231–249. doi: 10.1007/s10584-007-9363-z
Williams, A. P., Allen, C. D., Millar, C. I., Swetnam, T. W., Michaelsen, J., Still, C. J., et al. (2010). Climate change and water in Southwestern North America special feature: forest responses to increasing aridity and warmth in the southwestern United States. Proc. Natl. Acad. Sci. U.S.A. 107, 21289–21294 doi: 10.1073/pnas.0914211107
Zhang, J., Powers, R. F., Oliver, W. W., and Young, D. H. (2013). Response of ponderosa pine plantations to competing vegetation control in Northern California, USA: a meta-analysis. Forestry 86, 3–11. doi: 10.1093/forestry/cps054
Keywords: forest fuel management, drought, evapotranspiration mediterranean ecosystem, water yield, fire hazard reduction
Citation: Tague CL and Moritz MA (2019) Plant Accessible Water Storage Capacity and Tree-Scale Root Interactions Determine How Forest Density Reductions Alter Forest Water Use and Productivity. Front. For. Glob. Change 2:36. doi: 10.3389/ffgc.2019.00036
Received: 02 April 2019; Accepted: 19 June 2019;
Published: 05 July 2019.
Edited by:
Stephen Douglas Sebestyen, Northern Research Station - US Forest Service (USDA), United StatesReviewed by:
Salli F. Dymond, University of Minnesota Duluth, United StatesJohn T. Van Stan, Georgia Southern University, United States
Copyright © 2019 Tague and Moritz. This is an open-access article distributed under the terms of the Creative Commons Attribution License (CC BY). The use, distribution or reproduction in other forums is permitted, provided the original author(s) and the copyright owner(s) are credited and that the original publication in this journal is cited, in accordance with accepted academic practice. No use, distribution or reproduction is permitted which does not comply with these terms.
*Correspondence: Christina L. Tague, Y3RhZ3VlQGJyZW4udWNzYi5lZHU=