- 1Institute of Meteorology and Climate Research - Atmospheric Environmental Research, Karlsruhe Institute of Technology, Garmisch-Partenkirchen, Germany
- 2Faculty of Geosciences, Geoengineering and Mining, Technische Universität Bergakademie Freiberg, Freiberg, Germany
- 3Research Unit Environmental Simulation (EUS), Helmholtz Zentrum München, Munich, Germany
The emission of biogenic volatile organic compounds (BVOCs) is usually thought to depend on species-specific emission capacities that vary with seasonal and phenological conditions. Actual—so called constitutive—emissions are then calculated from prevailing temperature and radiation. However, various abiotic and biotic stressors such as ozone, extreme radiation and temperature conditions, as well as wounding e.g., from insect feeding, can lead to de-novo emissions of stress-induced BVOCs (sBVOCs) that may excel constitutive emissions by more than an order of magnitude. These emissions often have a considerable different compound composition and are short-lived but can prolong under continuous stress for quite some time. Thus, they may easily have a large impact on overall regional BVOC emissions. However, sBVOCs are generally not considered in models since up to date no consistent mechanism has been proposed. This manuscript suggests a new mechanism based on the finding that sBVOCs originate from a handful of biosynthetic pathways which synthesize compounds in the groups of monoterpenes, sesquiterpenes, and green leave volatiles, as well as methyl salicylate, ethanol/acetaldehyde, methanol/formaldehyde, and acetone. Isoprene is also considered but since it is often constitutively emitted, the specific role of stress induction is difficult to determine for this compound. A function is proposed that describes the production of all de-novo sBVOCs sufficiently well and scales with stress intensity. It is hypothesized that the response delay and the form of the function is specific for the production pathway and valid for ozone as well as wounding (herbivory) induced stress. Model parameters are then derived from pooled literature data based on a meta-analysis of suitable induction-response measurements of different plant species. The overall emission amount derives from the intensity and frequency of the stress impulse. We present a number of literature studies that are used to parameterize the new model as well as a selection of evaluations for single- and multiple-stress inductions. Furthermore, coupling and interaction with constitutive emission models as well as limitations and possible further developments are discussed.
Introduction
Volatile organic compounds (VOCs) are important precursors for the formation of tropospheric ozone and secondary aerosols (Shallcross and Monks, 2000; Atkinson and Arey, 2003). Excessively high ozone concentrations in the lower atmosphere are representing a considerable threat to human health, causing yield losses in agriculture and forestry, and reduce carbon sequestration as well as other ecosystem services (Wilkinson et al., 2012; Lombardozzi et al., 2015; Silva et al., 2017). Secondary aerosols or fine and ultrafine particles are also causing pulmonary diseases, directly affect the radiation regime of the atmosphere, and are important cloud condensation nuclei (Riipinen et al., 2012; Shrivastava et al., 2017). A considerable share of VOCs originates from biogenic emissions (BVOCs) (Laothawornkitkul et al., 2009). Therefore, in order to evaluate the most efficient air pollution reduction strategies, it is important to know the quantity and composition of BVOC emissions from different plant sources and thus their spatial and temporal distribution.
BVOC emissions have been studied since the late 1980's and relationships between some main compounds and environmental conditions have been quantified for many plant species. This led to the general distinction between constitutive and induced emissions, assuming continuously developing boundary conditions to drive the first, and oxidative and mechanical stress to trigger the second (Litvak and Monson, 1998; Brilli et al., 2009; Ali et al., 2011; Joo et al., 2011; Jiang et al., 2016; Copolovici et al., 2017). Constitutive emissions are described based on genetically determined emission potentials and the ability to form compound-specific VOC storages in different types of plant tissues and organs. Various environmental conditions are responsible for the expression of this species-specific potential, from which temperature and radiation are the most important ones, both for short- and longer-term impacts. Water availability affects BVOC emission directly and indirectly and may act in different directions dependent on stress severity. CO2 and nutrient availability do also change BVOC emission intensity but possible connections to production pathways are numerous and the exact mechanism is yet not fully elucidated (Grote et al., 2013).
In contrast, BVOC emissions may also occur as responses to specific stressors. Such stressors can be oxidative stress originating from excessive light, temperature and air pollutants, or mechanical stress such as biting, sucking, cutting, or wounding including abrasion due to wind movement (Haase et al., 2011) and membrane damages due to freeze/thaw cycles (Fall et al., 2001). Although the drivers can be abiotic or biotic, the plant internal signal cascades and thus the responses of plants are often similar (Iriti and Faoro, 2009). One of the reasons for this surprising fact is that the kind of provoked damages are often the same, i.e., destroyed or impaired membrane functions which causes changes in plasma trans-membrane potential and cytosolic Ca2+concentration (Dicke and Baldwin, 2010). Another reason may be that the same substances that are used for repair of and protection against oxidative stress also serve for signaling in order to repel parasites or attract enemies of herbivores (Cape, 2008; Loreto and Schnitzler, 2010). In fact, many of the volatile terpenes that serve as antioxidative defenses, are also non-specific toxins active against a wide range of organisms (bacteria, fungi, plants, and animals) (Meena et al., 2017). Nevertheless, the large variety of the emitted compounds and the species-specific as well as stress-specific emission intensity results in a multitude of different possible blends (Kravitz et al., 2016).
Numerous structurally quite different molecules can be produced but oxygenated VOCs, and more specifically green leaf volatiles (GLVs), constitute a general element in most blends of induced emissions, in particular aldehydes, alcohols and acetates (Blande et al., 2014). The emission is generally proportional to the extent of wounding (Mithöfer et al., 2005; Niinemets et al., 2013; Portillo-Estrada et al., 2015) and occurs in attached or detached leaves. Other relevant sBVOCs are the C11 homoterpene dimethyl-nonatriene (DMNT) and the volatile benzoid methyl salicylate (MeSA). Emissions of DMNT have often been found in response to herbivore attack (e.g., Vuorinen et al., 2004b; Ibrahim et al., 2008; Tholl et al., 2011; Copolovici et al., 2014) but also as a response to ozone (Behnke et al., 2009; Carriero et al., 2016). Similarly, MeSA is often associated with ozone responses (e.g., Hartikainen et al., 2012; Cardoso-Gustavson et al., 2014; Li et al., 2017; Bison et al., 2018) but is similarly common in herbivore induced emission blends (Vuorinen et al., 2007; Kigathi et al., 2009). Since isoprene and terpenoids help to mitigate any kind of membrane damage, their production may be induced or upregulated in response to abiotic as well as biotic stress (Litvak et al., 1999; Prieme et al., 2000; Brilli et al., 2011; Achotegui-Castells et al., 2013; Faiola et al., 2015; Semiz et al., 2017; Kanagendran et al., 2018b; Visakorpi et al., 2018) but may also been downregulated in favor of GLVs (Brilli et al., 2009; Copolovici et al., 2017). The up-regulation of terpenoids have been found to increase the emissions by a factor of ~10–20 in response to needle damages of feeding insects (Ghimire et al., 2017) as well as to bark beetle infestations (Amin et al., 2012, 2013).
Similar to constitutive emissions, induced compounds may be de-novo produced or released from storages when the diffusive resistance is decreased by wounding of any kind. In some plant species, storages in specific tissues occur from which volatiles can be released that reduce the attractiveness of tissues for herbivores and protect wounds from getting infected by microbes and fungi. As far as we know, not all stored compounds but only monoterpenes are released from such storages (Litvak and Monson, 1998; Clancy et al., 2016), possibly because of their antibiotic properties (e.g., Michelozzi, 1999). Therefore, most induced BVOCs are directly emitted after production but a clear differentiation between emissions from storages and de-novo production cannot be given, except if the emissions are directly related to increased resin flow (Baier et al., 2002; Eller et al., 2013). It should be noted that wounding includes cutting which is a widespread management activity in grasslands and forests. In fact, it is known that grasslands and pastures do release high amounts of GLVs after cutting which origin from de-novo production, in particular alcohols (Karl et al., 2001; Warneke et al., 2002; Davison et al., 2008). Therefore, it has been hypothesized that the large transient fluxes caused by cutting could be in the same order of magnitude as constitutive emissions from undisturbed pasture. Considering induced emissions thus would virtually double emission estimates from these ecosystems (Kirstine et al., 1998). Forest harvests and thinning are by definition much less frequent. Nevertheless, a thinning of half the trees in a Scots pine forests has triggered a monoterpene release from wounded tissue that was 40 times the constitutive emission rate (Schade and Goldstein, 2003).
Induced emissions from abiotic as well as biotic disturbances can thus be considered as extremely important as they can increase short- and medium term BVOC fluxes by at least one order of magnitude. They also have a different blend than emissions in undisturbed conditions. Nevertheless, there is currently no relationship implemented in emission models that account for induced emissions in response to air pollution, insect gradations, or mechanical disturbances although this issue has been recognized as important (Arneth and Niinemets, 2010). The only approach that we are aware of uses experimentally determined relations between stress-induced and constitutive emissions to quantify the impact of spruce aphids in the field (Bergström et al., 2014). A general model that relates induced emissions to stress severity and intensity is thus still missing. We therefore suggest a globally applicable approach that describes induced emission strength directly in dependence on environment and can be parameterized for different species. We hypothesize that all compounds synthesized by a specific pathway have the same dynamics (delay time between stress and emission and duration of emission after a single stress event) and only stress sensitivity depends on species. This enables to pool normalized data from various compounds and species for parametrization. We thus use experimental data obtained from a meta-analysis investigating ozone as well as herbivore-induced BVOC emission pattern. The new algorithm is then applied on three independent data sets that either use ozone or herbivores as stressors over a defined time period so that simulated and measured emissions could be compared for different compound emissions over the course of several days.
Model Development and Parameterization
Due to a lack of clear indication of storage-related induced emissions and in order to keep the approach simple, we only consider compound releases that originate from de-novo synthesis. De-novo synthesis in response to wounding generally occurs within very short time periods after the induction event independent of the kind of induction. For example, maximum emission rates of acetaldehyde were reached within minutes after the start of the treatment (Portillo-Estrada et al., 2015). This type of behavior is well-represented with the so-called Fraser-Suzuki function (Equation 1) which has first been used to describe band shapes (Fraser and Suzuki, 1969) and is still popular for the representation of chemical responses (Stankovic et al., 2018).
Ei being the emission rate for the compound “I”, Fdel and Fbr are parameters that define the delay of the exponential increase with time and the broadness of the function, respectively. Fsc is a species- and compound-specific scaling parameter that describes the maximum response per unit stress, and NSE is the number of stress events during a time interval (t-t0). This implies that the emission response scales linearly with the experienced stress severity, which is an assumption that holds some pitfalls. Regarding ozone, Carriero et al. (2016) indeed describe a weak linear response of induced emissions. However, the response should be related to ozone uptake (Li et al., 2017) while there is not always a linear relation between uptake and ozone exposure. Thus, responses to exposure might change for example with stomatal acclimation or damage. Emission in response to herbivore damage is linearly related to damaged tissue (e.g., Niinemets et al., 2013) and an increasing number of individuals should be related to NSE, while differences in stress intensity—that might be related to the different kind of damages (such as sucking and biting)—are difficult to determine yet. The responsiveness of the function to changes of parameters is demonstrated in Figure 1 (with Fsc set to 1 in all simulations). It demonstrates that small Fbr values result in left-skewed distributions that develop to into Gaussian distributions and increase their width when increasing. Fdel shifts the whole distribution to the right, accounting for response delays that occur in particular when emitted compounds need to be de-novo synthesized.
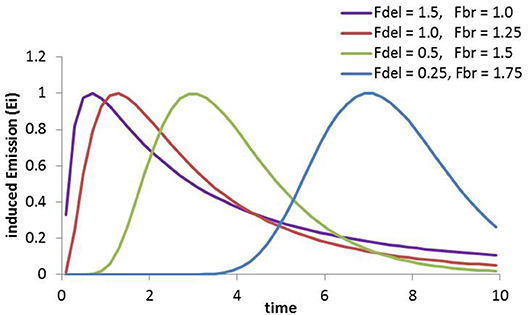
Figure 1. Sensitivity of the Fraser-Suzuki function to changes in the parameters. The unit of time is not specific but a reasonable order of magnitude would be hours for the distributions shown.
We assume that specific compounds are always produced in a similar way, respectively by a specific synthesis pathway, which enables representing the dynamics of this pathway generaly and refrain from species-specific parameterization. Thereby we differentiate between 12 compounds that are produced from 7 pathways (Figure 2). The indications of pathways are based on previous publications (Pare and Tumlinson, 1997; Kesselmeier and Staudt, 1999; Laothawornkitkul et al., 2009; Loreto and Schnitzler, 2010; Schnitzler et al., 2010; Tholl et al., 2011; Dudareva et al., 2013; McCormick et al., 2014b). If this assumption holds, the production of sBVOC emissions can be described with compound-specific emission parameters and the intensity of the response is simply scaled with the parameter Fsc, including the option to be set to 0 in case a specific compound emission is below the detection threshold.
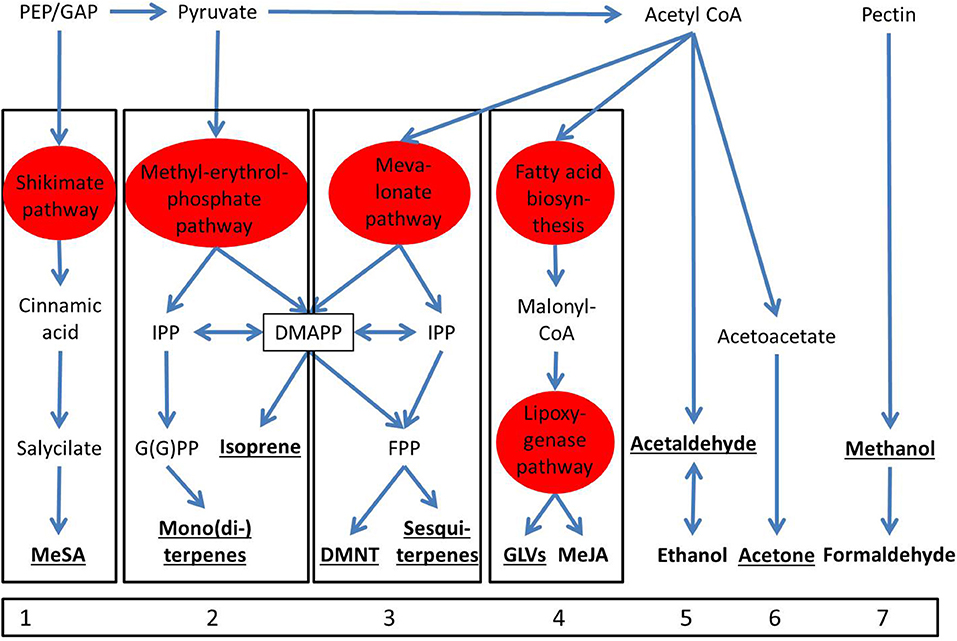
Figure 2. Biosynthetic pathways of the major compounds that are emitted from induction (numbered 1–7). The potentially emitted compounds are indicated in bold letters from which those that are investigated here are underlined. Pathways that comprise several intermediate products are shown in red. (abbreviations: PEP, phenolenolpyruvate; GAP, glyceraldehyde 3-phosphate; MeSA, methyl salicylate; IPP, isopentenyl diphosphate; G(G)PP, geranylgeranyl pyrophosphate; DMAPP, dimethylallyl pyrophosphate; FPP, farnesyl pyrophosphate; DMNT, (E)-4,8-dimethyl-1,3,7-nonatriene; GLVs, green leaf volatiles; MeJA, methyl jasmonate). Despite their common components, the methylerythritol phosphate (MEP) and the mevalonate pathway (MVP) are distinguished because they are occurring in the plastids and in the cytosol, respectively.
In order to parameterize the emission pattern of the different compounds we have collected data from 11 studies that are providing response patterns for 9 of the 12 compounds (MeSA, monoterpenes, DMNT, sesquiterpenes, GLVs, acetaldehyde, acetone, and methanol) from all of the seven pathways. From these studies, five use ozone as the inducing abiotic stress (Beauchamp et al., 2005; Behnke et al., 2009; Pazouki et al., 2016; Li et al., 2017; Acton et al., 2018), six others apply biotic stressors and one both (Kanagendran et al., 2018b). From the abiotic stress experiments, two have exposed experimental plants to real insects (Mengistu et al., 2014; Yli-Pirilä et al., 2016), while four studies use mechanical wounding (Brilli et al., 2011; Erb et al., 2015; Portillo-Estrada et al., 2015; Kanagendran et al., 2018b) and two studies applied methyl-jasmonate (Faiola et al., 2015; Jiang et al., 2017) to mimic herbivory. Data are extracted from the literature either directly using the Engauge digitizer 10.11 software or from presented graphs by determining the turning points of emission developments. These data have been normalized for stress intensity (setting the largest obtained emission rate as 1) and parameters of Equation 1 are defined for each of the investigated 9 compounds (using the solver tool in Excel, Microsoft Cooperation).
During the exploration of the data, we noticed that several compounds typically exhibit a bimodal emission pattern to one single stress event, i.e., monoterpenes, sesquiterpenes, GLVs, and methanol. The pattern is characterized by a fast response that peaks within the first 30 min after stress induction and is quickly fading thereafter. In parallel and without any further stress event, a slower response builds up within 5–15 h which needs ~1–2 days to fade out. However, the pattern has not been observed in all investigations which might partly be related to methods that are too coarse to detect such fast responses (Behnke et al., 2009; Pazouki et al., 2016; Acton et al., 2018; Kanagendran et al., 2018a). In these cases, normally only the second peak is described in the publication. We considered the bimodal pattern by introducing a second emission term into Equation 1b:
Efast and Eslow are calculated according to equation 1a with separately derived parameters for delay and broadness (Fdel, Fbr) (see Table 1). The indicator “j” represents the six compounds for which this differentiation is used (monoterpenes, sesquiterpenes, GLVs, MeSA, methanol, and DMNT). It should be noted, however, that only for monoterpenes, GLVs, and methanol (only in ozone-induced emissions) this pattern has actually observed simultaneously in one or more of the datasets. For sesquiterpenes, fast and slow responses originate from different datasets: for MeSA and DMNT only the slow response is backed by experimental data, and regarding DMNT the data are from one experimental source only (Behnke et al., 2009). While no short-term response has been recorded, the similarity of the response to that of methanol let us assume that a fast response might have occurred but gone unnoticed since the first measurements have been carried out after 3 h. Similarly, the currently used data sets for MeSA emissions seem to indicate that a fast induction response is minor or non-existent but other studies report strongly increased emissions only 1 h after stress exposure (Filella et al., 2006). Therefore, we decided to assume a short term response for both of these compounds too. The remaining three compounds show only fast responses derived from one single observation each, although a slow response cannot be excluded since the measurement might not have been long enough. Simulated mono- and bimodal responses for all investigated compound groups are presented in Figure 3, distinguished into data from herbivory and ozone stressed plants.
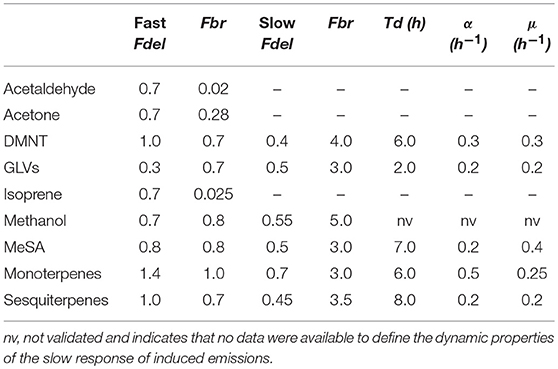
Table 1. Parameter values for Equations (1) and (2) as estimated for different inducible compounds from published studies.
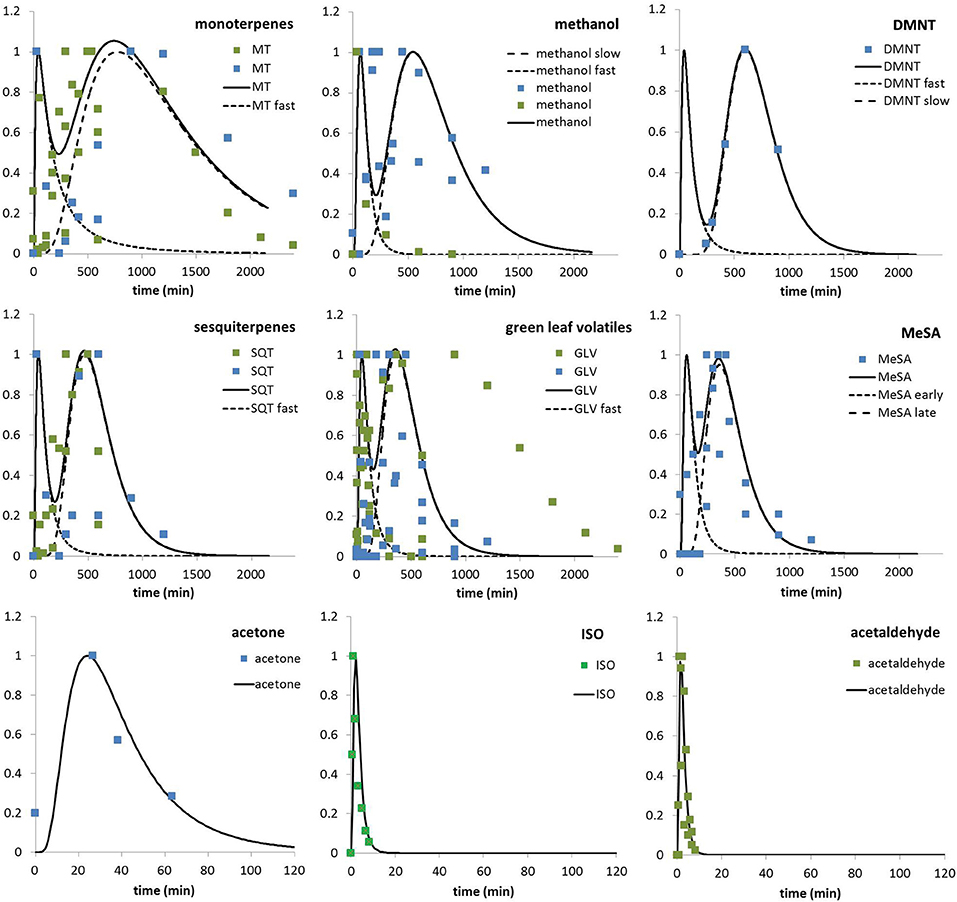
Figure 3. Normalized temporal development of emissions in response to induced ozone (blue dots) and herbivory (green dots) stress as derived from 13 publications (5 for ozone, 7 for herbivory, and one for both). Lines are representing simulated transients of emissions. In case of bi-model developments, the single representations for slow and fast responses are separately normalized as indicated with broken lines. Compounds are sorted according to response time. It should be noted that the last row is given in a different temporal scale than the rows above. See text for literature sources.
While the fast emission term is assumed to rely on available precursors, the slow emission term represents the intermediates supply from biosynthesis pathways that requires enzymatic reactions. Enzyme activity (EA) is regulated on biochemical level by fast activation/deactivation and on a protein level requiring a gene expression and respective protein biosynthesis. To represent the development of EA we assume a protein synthesis rate (α) that linearly increases enzyme activity during the period where stress events occur and a constant protein decomposition rate (μ). Both parameters are given per unit of time (e.g., 1 h). This approach is similar to the one developed for the build-up of isoprene synthase activity (Lehning et al., 2001) although widely simplified since no temperature dependency is implemented so far. Two further restrictions to the enzyme production term are applied: First, enzyme formation only starts after a threshold time period (Td) that accounts for a delay between stress application and signal recognition and processing. Again, we assume that this threshold period is compound specific and does not change with species or stress type. Second, while protein degradation is independent of the diurnal cycle, biosynthesis of the compounds only occurs in the model during the light phase, assuming that the biochemical precursors need to be supplied from photosynthetic intermediates and not from storages. The parameters Td, α, μ for all compounds as derived from experiments where stress was applied over a certain period of time (not as pulse) and which prolonged over several days (see below), are given in Table 1.
Evaluation Results
In order to evaluate the derived and parameterized function, we used three literature sources that provide emission responses to 5 h of ozone fumigation (Heiden et al., 1999) or 1.5–2 days of herbivore exposure (Ghirardo et al., 2012; McCormick et al., 2014a). Emissions of selective GLVs, monoterpenes, and sesquiterpenes were calculated before, during and after the respective induction signal that prolonged during a certain period (from the green to the red arrows in Figures 4–6). Since emissions from one signal are not faded out before the next signal occurs, emissions at a particular point in time may originate from several induction events if these events occur in shorter time intervals than complete fading is achieved. The overall emission strength is scaled with the Fsc parameter that is linearly related to a specific induction interval. The examples presented are simulated for a time interval of 1 h, a time resolution frequently used by mechanistic models. Since GLVs and monoterpene emissions were also observed before stress induction, these compounds are assumed to be emitted also constitutively. This fraction has therefore been separately calculated using Equation 1 and has been added into the sum of total emission displayed in Figures 4, 5. Parameters for control emissions are adjusted to measurements and are: Fdel 1.5, Fbr 20, Fsc for GLV: 50, Fsc for MONO: 30 (Figure 4), 2 (Figure 5). Note that the broadness parameter is relatively large, leading to a function maximum toward the end of the day. The driver (t-t0) is the daytime since sunrise and the emission is set to 0 during dark hours, reflecting the assumption that control emissions are directly related to photosynthesis. It should also be noted that parameters for enzyme kinetics (Td, α, μ) are not parameterized separately for each evaluation data set but are set independent from the plant species or the kind of stress.
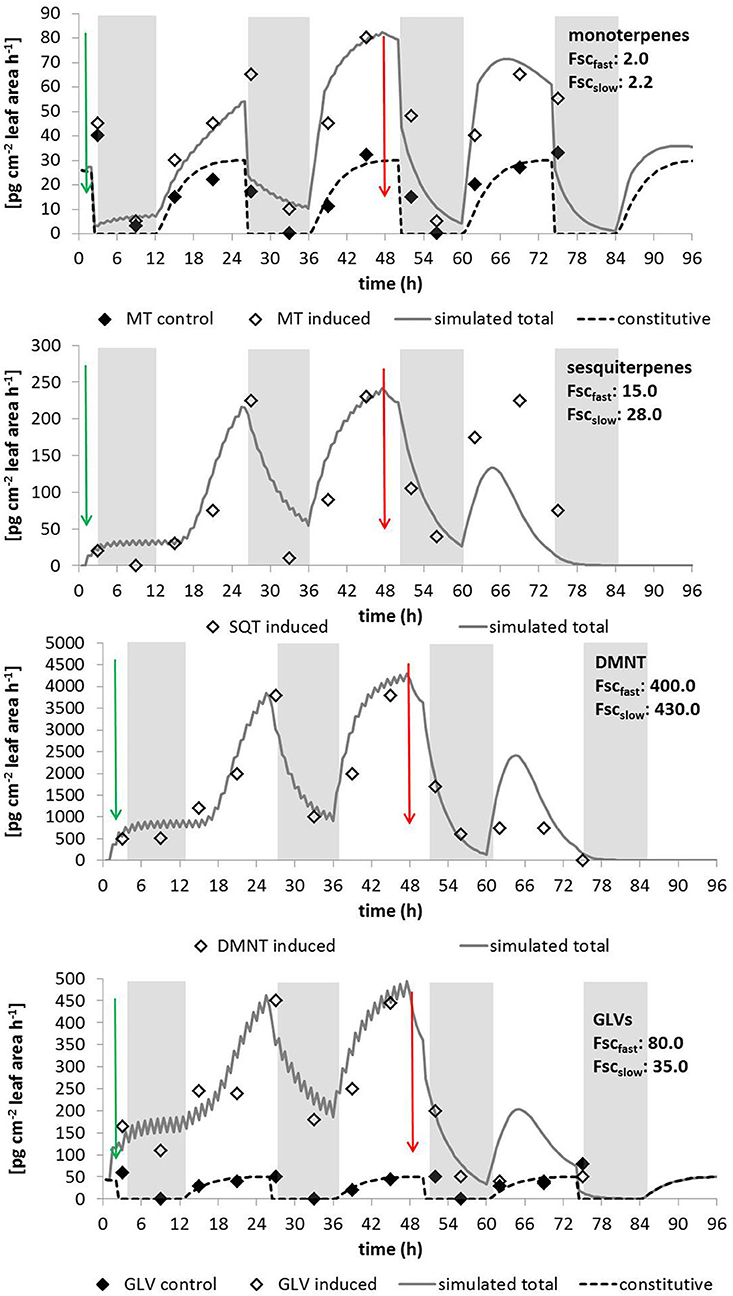
Figure 4. Simulated and measured emissions of (from the top to the bottom) α-pinene, β-caryophyllene, DMNT, and the GLV hexenyl acetate which are typical representatives for the indicated emission types. Data are taken from McCormick et al. (2014a) measured at young poplar trees (Populus nigra) exposed to gypsy moth larvae (Lymantria dispar). Shaded areas denote dark periods without de-novo biosynthesis; green arrows show the start of the exposure to larvae and red arrows the end of larvae feeding. Indicated scaling parameters are given for 1-h induction cycles; for other parameters see text and Table 1.
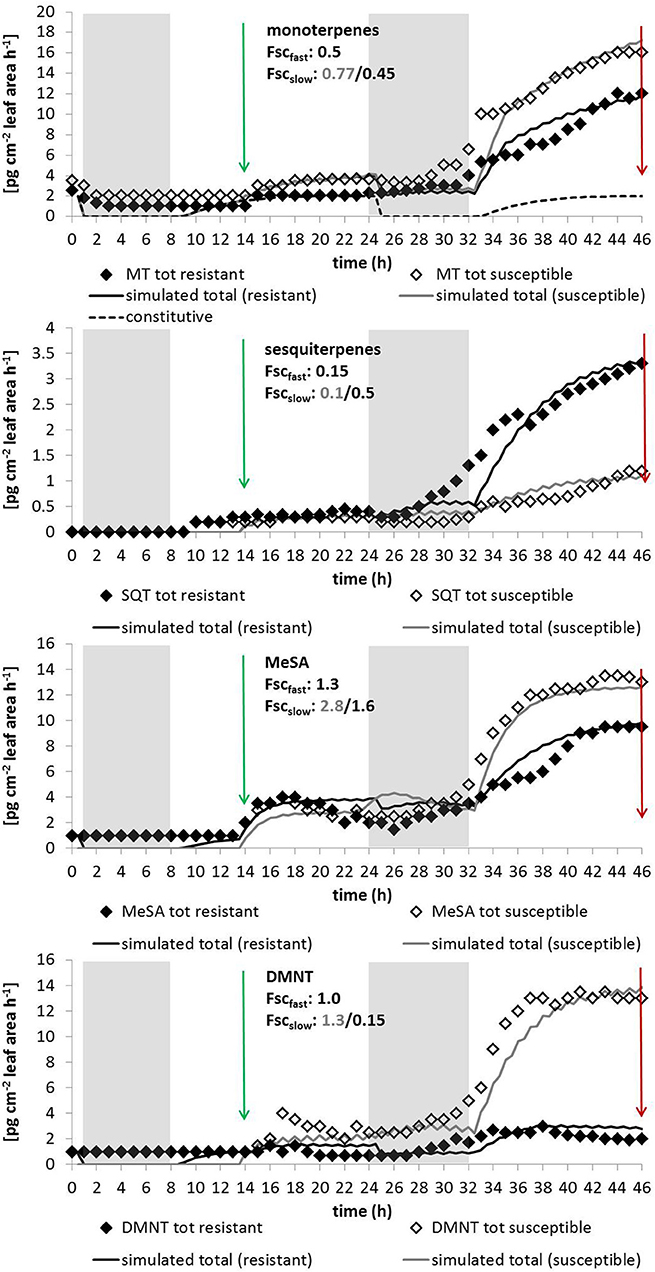
Figure 5. Simulated and measured emissions of (from the top to the bottom) pooled monoterpenes and sesquiterpenes, as well as of MeSA and DMNT for plants that are susceptible (gray lines and open symbols) and tolerant (black lines and closed symbols) to caterpillar feeding. Data are taken from Ghirardo et al. (2012) measured on young oak seedlings (Quercus robur) exposed to larvae of Tortrix viridana. Shaded areas denote dark periods without de-novo biosynthesis; green arrows show the start of the larvae exposure and red arrows the end of larvae feeding. Indicated scaling parameters are given for 1-h induction cycles; for other parameters see Table 1.
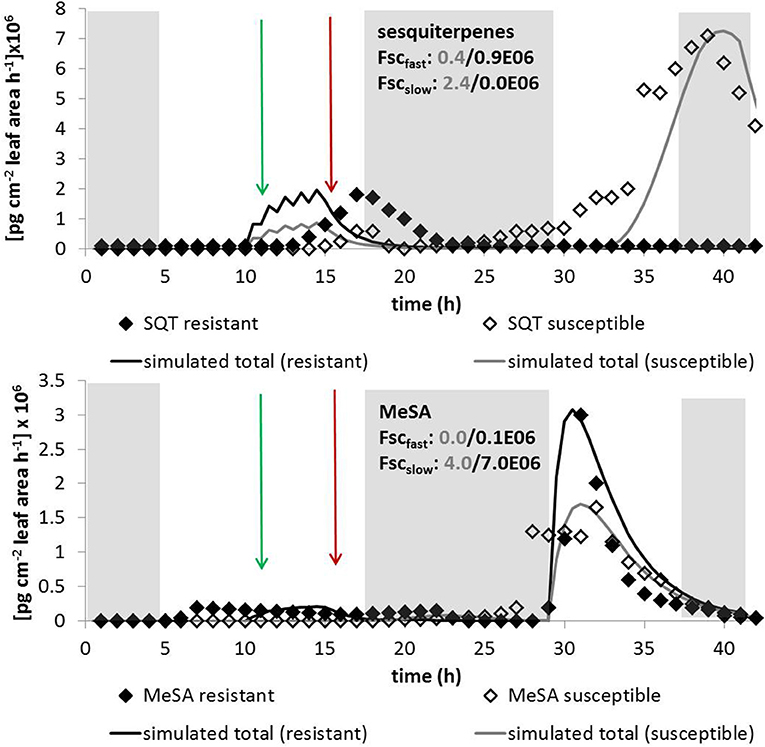
Figure 6. Simulated and measured emissions of the sesquiterpene valencene (top) and MeSA (bottom) for susceptible (gray lines and open symbols) and stress tolerant variants (black lines and closed symbols). Data are taken from Heiden et al. (1999) measured at tobacco plants (Nicotiana tabacum) exposed to ozone. Shaded areas denote dark periods without de-novo biosynthesis; green arrows show the start and red arrows the end of the fumigation. Indicated scaling parameters are given for 1-h induction cycles; for other parameters see Table 1.
Discussion and Conclusion
Despite a rather huge number of investigations regarding induced BVOCs, still only few data are available providing high resolution continuous measurements for the main emission types and compounds. Thus, our meta-analysis sometimes only yielded one or few datasets per compound group that not always covered a period necessary to determine short- as well as long-term responses. Nevertheless, it is interesting to note that the general pattern of immediate responses that are fueled by existing biochemical precursors in combination with responses that develop and decompose on an hourly time scale as proposed by Jiang et al. (2017) is persistent throughout the investigated plant species. It also seems to apply for ozone as well as herbivory-induced stress which supports the view that plant stress which reduces primary production simultaneously enhances secondary metabolic pathways (Iriti and Faoro, 2009) and that responses may have developed due to adaptive co-evolution (Vuorinen et al., 2004a). However, plant species might still only display either immediate or slow responses and specific volatile compounds within one compound group seem to show a rather large variability regarding reaction speed and decay of the emission rates. Accordingly, the data base for parameterization and possible further differentiation of compound groups should be elaborated when new data will become available. The precision of modeling might also be increased if the intensity of the stress events could be normalized, which particularly relates to methyl-jasmonate treatments where the induction is supposed to start at the time of spraying while the arrival time at sensitive sites in the leaves and the actually effective dose is not defined (Jiang et al., 2017). Similar, ozone treatments might differ in their effectiveness depending whether the ozone dose induces an acute or chronic response and on the physiological state of the plant, in particular regarding uptake resistance of stomata and mesophyll. The latter point indicates a general link to ecosystem models since uptake resistance certainly depends on drought stress—an issue that often coincides with high ozone episodes as well as herbivore abundance.
Simple assumptions about the kinetics of enzyme formation and degradation with time only differentiating between night- and day-time dynamics enabled scaling of emission responses over several days. Simulations using the same parameters per emission type were in good accordance with measurements, independent from the kind of stress (caterpillar feeding or ozone fumigation). Remaining differences indicate that enzyme biosynthesis and hence the increase in biosynthetic capacity also occurs during nighttime explaining the start of slow-response emissions even before the light is switched on again (Heiden et al., 1999; Ghirardo et al., 2012). Also, the applied 1-h time step showed to lead to small oscillations of emissions originating from fast responses, indicating that a higher temporal resolution would be preferable. Nevertheless, the presented scaling procedure provides a mechanistic basis for further emission estimations at the stand- or ecosystem scale, considering total foliage area and stress abundance. In case of herbivore damage, the situation is more complicated since the leaf area is dynamically reduced during feeding, an effect that has to be considered during extended disturbance events. Also, the number of herbivores are difficult to estimate and develop dynamically, requiring a separate model approach (Pitt et al., 2007; Trnka et al., 2007; Pinkard et al., 2010; Battaglia et al., 2011).
It should be noted that the assumption of a constant effectiveness of induced emissions so that emission responses scale with stress severity might not always hold. This is because a continuous stress can trigger an adjustment of the plant so that the sensitivity to the stress decreases with time (Achotegui-Castells et al., 2015). The adjustment might include increased uptake resistances for air pollution or the production of compounds that are difficult to digest by herbivores. In addition, also BVOCs, i.e., mono- and sesquiterpenes can reduce the effectiveness of induced stressors as they can detoxify ozone already within the leaf (Vickers et al., 2009) and might directly repel herbivores or indirectly reduce their impact by attracting predators (Meena et al., 2017; Turlings and Erb, 2018). In general, very strong or prolonged induction is also likely to pose a feedback to stress-induced emissions since it decreases the amount of emitting tissue (i.e., by herbivore feeding) and damages the photosynthetic process (i.e., due to ozone) thus leading to supply limitation (Visakorpi et al., 2018). Such limitation may also be triggered by other stressors such as drought as has been demonstrated in a field study by Scott et al. (2019). In this case, specific site conditions and plant behavior would need to be considered for whole-ecosystem estimations.
We also acknowledge that an explicit dependence on temperature could serve the general applicability of the approach. It is obvious that the speed of biosynthesis and thus in particular the slow-response term should depend on this impact. However, available data and the practice to keep temperatures constant during experiments to concentrate on the exposure effect didn't allow to incorporate the temperature effect yet. The suggested model for sBVOC emissions is based on simple assumptions regarding the emission behavior of the most common compounds but also accounts for species-specific differences in stress sensitivity as well as stress severity and duration. It can be applied in addition to simulating constitutive emission without necessary interactions. Therefore in can easily be combined with temperature and light dependent models (Guenther, 1999; Niinemets et al., 1999; Monson et al., 2012; Grote et al., 2014). Consistency could be assured simply by using leaf area or biomass for scaling in both approaches. Coupled to ecosystem models, the approach could contribute to an improved ability to estimate climate change impacts on air chemistry by considering the secondary effects that originate from increased insect aggregations (Seidl et al., 2017) and ozone concentrations (Sicard et al., 2017).
The proposed model describes emission responses to oxidative and wounding stress by compound and stress severity. In addition, the strength of emission response seems to be species-specific although a comparison of experimental results is hampered due to different stress intensities applied. If it is assumed that the parameterization is independent of further environmental conditions and that stress intensity could be generally defined (e.g., as feeding intensity of one insect per time period), it should be possible to scale modeled emissions to the ecosystem level (e.g., based on estimates of insect number and activity). Such an endeavor, however, demands for future experiments with relevant plant species and insect-plant combinations as well as for large-scale field measurements for evaluation. For evaluation purposes, further processes such as the dynamic development of stress needs to be considered. This might be more easy for stress related to air pollution than to insect abundance and development, the latter requiring dynamic gradation models (Nedorezov and Sadykova, 2015). In addition, likely physiological adaptations or increased resource competition of herbivores might render the assumption of a linear stress-response relation invalid, which demands for further model refinements. Nevertheless, we suppose that despite these challenges the model could eventually contribute to the determination of full BVOC emission inventories.
Data Availability
The raw data supporting the conclusions of this manuscript will be made available by the authors, without undue reservation, to any qualified researcher.
Author Contributions
RG has developed and parameterized the model while MS conducted the meta-analysis and extracted the data to be compared with model outputs. J-PS and AG contributed substantially to the model concept and design of the work and supported the use of data produced in their laboratory. All co-authors worked on the data analysis, wrote text passages, and critically revised the text.
Conflict of Interest Statement
The authors declare that the research was conducted in the absence of any commercial or financial relationships that could be construed as a potential conflict of interest.
Acknowledgments
We acknowledge support by Deutsche Forschungsgemeinschaft and Open Access Publishing Fund of Karlsruhe Institute of Technology.
References
Achotegui-Castells, A., Danti, R., Llusià, J., Rocca, G. D., Barberini, S., and Peñuelas, J. (2015). Strong induction of minor terpenes in Italian Cypress, Cupressus sempervirens, in response to infection by the fungus Seiridium cardinale. J. Chem. Ecol. 41, 224–243. doi: 10.1007/s10886-015-0554-1
Achotegui-Castells, A., Llusià, J., Hódar, J., and Peñuelas, J. (2013). Needle terpene concentrations and emissions of two coexisting subspecies of Scots pine attacked by the pine processionary moth (Thaumetopoea pityocampa). Acta Physiol. Plantarum 35, 3047–3058. doi: 10.1007/s11738-013-1337-3
Acton, W. J. F., Jud, W., Ghirardo, A., Wohlfahrt, G., Hewitt, C. N., Taylor, J. E., et al. (2018). The effect of ozone fumigation on the biogenic volatile organic compounds (BVOCs) emitted from Brassica napus above- and below-ground. PLoS ONE 13:e0208825. doi: 10.1371/journal.pone.0208825
Ali, J. G., Alborn, H. T., and Stelinski, L. L. (2011). Constitutive and induced subterranean plant volatiles attract both entomopathogenic and plant parasitic nematodes. J. Ecol. 99, 26–35. doi: 10.1111/j.1365-2745.2010.01758.x
Amin, H., Atkins, P. T., Russo, R. S., Brown, A. W., Sive, B., Hallar, A. G., et al. (2012). Effect of bark beetle infestation on secondary organic aerosol precursor emissions. Environ. Sci. Technol. 46, 5696–5703. doi: 10.1021/es204205m
Amin, H. S., Russo, R. S., Sive, B., Hoebeke, R. E., Dodson, C., McCubbin, I. B., et al. (2013). Monoterpene emissions from bark beetle infested Engelmann spruce trees. Atmos. Environ. 72, 130–133. doi: 10.1016/j.atmosenv.2013.02.025
Arneth, A., and Niinemets, Ü. (2010). Induced BVOCs: how to bug our models? Trends Plant Sci. 15, 118–125. doi: 10.1016/j.tplants.2009.12.004
Atkinson, R., and Arey, J. (2003). Gas-phase tropospheric chemistry of biogenic volatile organic compounds: a review. Atmos. Environ. 37, 197–219. doi: 10.1016/S1352-2310(03)00391-1
Baier, P., Führer, E., Kirisits, T., and Rosner, S. (2002). Defence reactions of Norway spruce against bark beetles an the associated fungus Ceratocystis polonica in secondary pure and mixed species stands. Forest Ecol. Manage. 159, 73–86. doi: 10.1016/S0378-1127(01)00711-3
Battaglia, M., Pinkard, E. A., Sands, P. J., Bruce, J. L., and Quentin, A. (2011). Modelling the impact of defoliation and leaf damage on forest plantation function and production. Ecol. Modelling 222, 3193–3202. doi: 10.1016/j.ecolmodel.2011.06.017
Beauchamp, J., Wisthaler, A., Hansel, A., Kleist, E., Miebach, M., Niinemets, Ü., et al. (2005). Ozone induced emissions of biogenic VOC from tobacco: relationships between ozone uptake and emission of LOX products. Plant Cell Environ. 28, 1334–1343. doi: 10.1111/j.1365-3040.2005.01383.x
Behnke, K., Kleist, E., Uerlings, R., Wildt, J., Rennenberg, H., and Schnitzler, J.-P. (2009). RNAi-mediated suppression of isoprene biosynthesis in hybrid poplar impacts ozone tolerance. Tree Physiol. 29, 725–736. doi: 10.1093/treephys/tpp009
Bergström, R., Hallquist, M., Simpson, D., Wildt, J., and Mentel, T. F. (2014). Biotic stress: a significant contributor to organic aerosol in Europe? Atmos. Chem. Phys. 14, 13643–13660. doi: 10.5194/acp-14-13643-2014
Bison, J. V., Cardoso-Gustavson, P., de Moraes, R. M., da Silva Pedrosa, G., Cruz, L. S., Freschi, L., et al. (2018). Volatile organic compounds and nitric oxide as responses of a Brazilian tropical species to ozone: the emission profile of young and mature leaves. Environ. Sci. Pollut. Res. 25, 3840–3848. doi: 10.1007/s11356-017-0744-1
Blande, J. D., Holopainen, J. K., and Niinemets, Ü. (2014). Plant volatiles in a polluted atmosphere: stress response and signal degradation. Plant Cell Environ. 37, 1892–1904. doi: 10.1111/pce.12352
Brilli, F., Ciccioli, P., Frattoni, M., Prestininzi, M., Spanedda, A. F., and Loreto, F. (2009). Constitutive and herbivore-induced monoterpenes emitted by Populus × euroamericana leaves are key volatiles that orient Chrysomela populi beetles. Plant Cell Environ. 32, 542–552. doi: 10.1111/j.1365-3040.2009.01948.x
Brilli, F., Ruuskanen, T. M., Schnitzhofer, R., Müller, M., Breitenlechner, M., Bittner, V., et al. (2011). Detection of plant volatiles after leaf wounding and darkening by proton transfer reaction “Time-of-Flight” mass spectrometry (PTR-TOF). PLoS ONE 6:e20419. doi: 10.1371/2Fjournal.pone.0020419
Cape, J. N. (2008). Interactions of forests with secondary air pollutants: some challenges for future research. Environ. Pollut. 155, 391–397. doi: 10.1016/j.envpol.2008.01.038
Cardoso-Gustavson, P., Bolsoni, V. P., de Oliveira, D. P., Guaratini, M. T., Aidar, M. P., Marabesi, M. A., et al. (2014). Ozone-induced responses in Croton floribundus spreng. (Euphorbiaceae): metabolic cross-talk between volatile oganic compounds and calcium oxalate crystal formation. PLoS ONE 9:e105072. doi: 10.1371/journal.pone.0105072
Carriero, G., Brunetti, C., Fares, S., Hayes, F., Hoshika, Y., Mills, G., et al. (2016). BVOC responses to realistic nitrogen fertilization and ozone exposure in silver birch. Environ. Pollut. 213, 988–995. doi: 10.1016/j.envpol.2015.12.047
Clancy, M. V., Zytynska, S. E., Senft, M., Weisser, W. W., and Schnitzler, J.-P. (2016). Chemotypic variation in terpenes emitted from storage pools influences early aphid colonisation on tansy. Sci. Rep. 6:38087. doi: 10.1038/srep38087
Copolovici, L., Kännaste, A., Remmel, T., and Niinemets, Ü. (2014). Volatile organic compound emissions from Alnus glutinosa under interacting drought and herbivory stresses. Environ. Exp. Bot. 100, 55–63. doi: 10.1016/j.envexpbot.2013.12.011
Copolovici, L., Pag, A., Kännaste, A., Bodescu, A., Tomescu, D., Copolovici, D., et al. (2017). Disproportionate photosynthetic decline and inverse relationship between constitutive and induced volatile emissions upon feeding of Quercus robur leaves by large larvae of gypsy moth (Lymantria dispar). Environ. Exp. Bot. 138, 184–192. doi: 10.1016/j.envexpbot.2017.03.014
Davison, B., Brunner, A., Ammann, C., Spirig, C., Jocher, M., and Neftel, A. (2008). Cut-induced VOC emissions from agricultural grasslands. Plant Biol. 10, 76–85. doi: 10.1055/s-2007-965043
Dicke, M., and Baldwin, I. T. (2010). The evolutionary context for herbivore-induced plant volatiles: beyond the ‘cry for help’. Trends Plant Sci. 15, 167–175. doi: 10.1016/j.tplants.2009.12.002
Dudareva, N., Klempien, A., Muhlemann, J. K., and Kaplan, I. (2013). Biosynthesis, function and metabolic engineering of plant volatile organic compounds. New Phytol. 198, 16–32. doi: 10.1111/nph.12145
Eller, A. S. D., Harley, P., and Monson, R. K. (2013). Potential contribution of exposed resin to ecosystem emissions of monoterpenes. Atmos. Environ. 77, 440–444. doi: 10.1016/j.atmosenv.2013.05.028
Erb, M., Veyrat, N., Robert, C. A. M., Xu, H., Frey, M., Ton, J., et al. (2015). Indole is an essential herbivore-induced volatile priming signal in maize. Nat. Commun. 6:6273. doi: 10.1038/ncomms7273
Faiola, C. L., Jobson, B. T., and VanReken, T. M. (2015). Impacts of simulated herbivory on volatile organic compound emission profiles from coniferous plants. Biogeosciences 12, 527–547. doi: 10.5194/bg-12-527-2015
Fall, R., Karl, T., Jordon, A., and Lindinger, W. (2001). Biogenic C5VOCs: release from leaves after freeze-thaw wounding and occurrence in air at a high mountain observatory. Atmos. Environ. 35, 3905–3916. doi: 10.1016/S1352-2310(01)00141-8
Filella, I., Peñuelas, J., and Llusia, J. (2006). Dynamics of the enhanced emissions of monoterpenes and methyl salicylate, and decreased uptake of formaldehyde, by Quercus ilex leaves after application of jasmonic acid. New Phytol. 169, 135–144. doi: 10.1111/j.1469-8137.2005.01570.x
Fraser, R. D. B., and Suzuki, E. (1969). Resolution of overlapping bands. Functions for simulating band shapes. Anal. Chem. 41, 37–39. doi: 10.1021/ac60270a007
Ghimire, R. P., Kivimäenpää, M., Kasurinen, A., Häikiö, E., Holopainen, T., and Holopainen, J. K. (2017). Herbivore-induced BVOC emissions of Scots pine under warming, elevated ozone and increased nitrogen availability in an open-field exposure. Agric. Forest Meteorol. 242, 21–32. doi: 10.1016/j.agrformet.2017.04.008
Ghirardo, A., Heller, W., Fladung, M., Schnitzler, J.-P., and Schroeder, H. (2012). Function of defensive volatiles in pedunculate oak (Quercus robur) is tricked by the moth Tortrix viridana. Plant Cell Environ. 35, 2192–2207. doi: 10.1111/j.1365-3040.2012.02545.x
Grote, R., Monson, R., and Niinemets, Ü. (2013). “Leaf-level models of constitutive and stress-driven volatile organic compound emissions,” in Biology, Controls and Models of Tree Volatile Organic Compound Emission, eds Ü. Niinemets and R. K. Monson (Dordrecht: Springer), 315–355.
Grote, R., Morfopoulos, C., Niinemets, Ü., Sun, Z., Keenan, T. F., Pacifico, F., et al. (2014). A fully integrated isoprenoid emissions model coupling emissions to photosynthetic characteristics. Plant Cell Environ. 37, 1965–1980. doi: 10.1111/pce.12326
Guenther, A. (1999). “Modeling biogenic volatile organic compound emissions to the atmosphere,” in Reactive Hydrocarbons in the Atmosphere, ed C. N. Hewitt (San Diego, CA: Academic Press), 41–94.
Haase, K. B., Jordan, C., Mentis, E., Cottrell, L., Mayne, H. R., Talbot, R., et al. (2011). Changes in monoterpene mixing ratios during summer storms in rural New Hampshire (USA). Atmos. Chem. Phys. 11, 11465–11476. doi: 10.5194/acp-11-11465-2011
Hartikainen, K., Riikonen, J., Nerg, A. M., Kivimäenpää, M., Ahonen, V., Tervahauta, A., et al. (2012). Impact of elevated temperature and ozone on the emission of volatile organic compounds and gas exchange of silver birch (Betula pendula Roth). Environ. Exp. Bot. 84, 33–43. doi: 10.1016/j.envexpbot.2012.04.014
Heiden, A. C., Hoffmann, T., Kahl, J., Kley, D., Klockow, D., Langebartels, C., et al. (1999). Emission of volatile organic compounds from ozone-exposed plants. Ecol. Appl. 9, 1160–1167. doi: 10.2307/2641386
Ibrahim, M. A., Stewart-Jones, A., Pulkkinen, J., Poppy, G. M., and Holopainen, J. K. (2008). The influence of different nutrient levels on insect-induced plant volatiles in Bt and conventional oilseed rape plants. Plant Biol. 10, 97–107. doi: 10.1111/j.1438-8677.2007.00013.x
Iriti, M., and Faoro, F. (2009). Chemical diversity and defence metabolism: how plants cope with pathogens and ozone pollution. Int. J. Mol. Sci. 10, 3371–3399. doi: 10.3390/ijms10083371
Jiang, Y., Ye, J., Li, S., and Niinemets, Ü. (2017). Methyl jasmonate-induced emission of biogenic volatiles is biphasic in cucumber: a high-resolution analysis of dose dependence. J. Exp. Bot. 68, 4679–4694. doi: 10.1093/jxb/erx244
Jiang, Y., Ye, J., Veromann, L.-L., and Niinemets, Ü. (2016). Scaling of photosynthesis and constitutive and induced volatile emissions with severity of leaf infection by rust fungus (Melampsora larici-populina) in Populus balsamifera var. suaveolens. Tree Physiol. 36, 856–872. doi: 10.1093/treephys/tpw035
Joo, E., Dewulf, J., Amelynck, C., Schoon, N., Pokorska, O., Simpraga, M., et al. (2011). Constitutive versus heat and biotic stress induced BVOC emissions in Pseudotsuga menziesii. Atmos. Environ. 45, 3655–3662. doi: 10.1016/j.atmosenv.2011.04.048
Kanagendran, A., Pazouki, L., Bichele, R., Külheim, C., and Niinemets, Ü. (2018a). Temporal regulation of terpene synthase gene expression in Eucalyptus globulus leaves upon ozone and wounding stresses: relationships with stomatal ozone uptake and emission responses. Environ. Exp. Bot. 155, 552–565. doi: 10.1016/j.envexpbot.2018.08.002
Kanagendran, A., Pazouki, L., and Niinemets, Ü. (2018b). Differential regulation of volatile emission from Eucalyptus globulus leaves upon single and combined ozone and wounding treatments through recovery and relationships with ozone uptake. Environ. Exp. Bot. 145, 21–38. doi: 10.1016/j.envexpbot.2017.10.012
Karl, T., Guenther, A., Lindinger, C., Jordan, A., Fall, R., and Lindinger, W. (2001). Eddy covariance measurements of oxygenated volatile organic compound fluxes from crop harvesting using a redesigned proton-transfer-reaction mass spectrometer. J. Geophys. Res. 106, 24157–24167. doi: 10.1029/2000JD000112
Kesselmeier, J., and Staudt, M. (1999). Biogenic volatile organic compounds (VOC): an overview on emission, physiology and ecology. J. Atmos. Chem. 33, 23–88. doi: 10.1023/A:1006127516791
Kigathi, R. N., Unsicker, S. B., Reichelt, M., Kesselmeier, J., Gershenzon, J., and Weisser, W. W. (2009). Emission of volatile organic compounds after herbivory from Trifolium pratense (L.) under laboratory and field conditions. J. Chem. Ecol. 35, 1335–1348. doi: 10.1007/s10886-009-9716-3
Kirstine, W., Galbally, I., Ye, Y., and Hooper, M. (1998). Emissions of volatile organic compounds (primarily oxygenated species) from pasture. J. Geophys. Res. 103, 10605–10619. doi: 10.1029/97JD03753
Kravitz, B., Guenther, A. B., Gu, L., Karl, T., Kaser, L., Pallardy, S. G., et al. (2016). A new paradigm of quantifying ecosystem stress through chemical signatures. Ecosphere 7:e01559. doi: 10.1002/ecs2.1559
Laothawornkitkul, J., Taylor, J. E., Paul, N. D., and Hewitt, C. N. (2009). Biogenic volatile organic compounds in the Earth system. New Phytol. 183, 27–51. doi: 10.1111/j.1469-8137.2009.02859x
Lehning, A., Zimmer, W., Zimmer, I., and Schnitzler, J.-P. (2001). Modeling of annual variations of oak (Quercus robur L.) isoprene synthase activity to predict isoprene emission rates. J. Geophys. Res. 106, 3157–3166. doi: 10.1029/2000JD900631
Li, S., Harley, P. C., and Niinemets, Ü. (2017). Ozone-induced foliar damage and release of stress volatiles is highly dependent on stomatal openness and priming by low-level ozone exposure in Phaseolus vulgaris. Plant Cell Environ. 40, 1984–2003. doi: 10.1111/pce.13003
Litvak, M. E., Madronich, S., and Monson, R. K. (1999). Herbivore-induced monoterpene emissions from coniferous forest: potential impact on local tropospheric chemistry. Ecol. Appl. 9, 1147–1159. doi: 10.2307/2641385
Litvak, M. E., and Monson, R. K. (1998). Patterns of induced and constitutive monoterpene production in conifer needles in relation to insect herbivory. Oecologia 114, 531–540. doi: 10.1007/s004420050477
Lombardozzi, D., Levis, S., Bonan, G., Hess, P. G., and Sparks, J. P. (2015). The influence of chronic ozone exposure on global carbon and water cycles. J. Clim. 28, 292–305. doi: 10.1175/jcli-d-14-00223.1
Loreto, F., and Schnitzler, J.-P. (2010). Abiotic stresses and induced BVOCs. Trends Plant Sci. 115, 154–166. doi: 10.1016/j.tplants.2009.12.006
McCormick, A. C., Boeckler, G. A., Köllner, T. G., Gershenzon, J., and Unsicker, S. B. (2014a). The timing of herbivore-induced volatile emission in black poplar (Populus nigra) and the influence of herbivore age and identity affect the value of individual volatiles as cues for herbivore enemies. BMC Plant Biol. 14:304. doi: 10.1186/s12870-014-0304-5
McCormick, A. C., Irmisch, S., Reinecke, A., Boeckler, G. A., Veit, D., Reichelt, M., et al. (2014b). Herbivore-induced volatile emission in black poplar: regulation and role in attracting herbivore enemies. Plant Cell Environ. 37, 1909–1923. doi: 10.1111/pce.12287
Meena, R. K., Jangra, S., Wadhwa, Z., Wati, M., and Wati, L. (2017). Role of plant volatiles in defense and communication. Int. J. Curr. Microbiol. App. Sci. 6, 300–313. doi: 10.20546/ijcmas.2017.604.033
Mengistu, M. M., Kasurinen, A., Yli-Pirilä, P., Joutsensaari, J., Klemola, T., Holopainen, T., et al. (2014). Contrasting responses of silver birch VOC emissions to short- and long-term herbivory. Tree Physiol. 34, 241–252. doi: 10.1093/treephys/tpt127
Michelozzi, M. (1999). Defensive roles of terpenoid mixtures in conifers. Acta Bot. Gallica 146, 73–84. doi: 10.1080/12538078.1999.10515803
Mithöfer, A., Wanner, G., and Boland, W. (2005). Effects of feeding Spodoptera littoralis on Lima bean leaves. II. Continuous mechanical wounding resembling insect feeding is sufficient to elicit herbivory-related volatile emission. Plant Physiol. 137, 1160–1168. doi: 10.1104/pp.104.054460
Monson, R. K., Grote, R., Niinemets, Ü., and Schnitzler, J.-P. (2012). Modeling the isoprene emission rate from leaves. New Phytol. 195, 541–559. doi: 10.1111/j.1469-8137.2012.04204.x
Nedorezov, L. V., and Sadykova, D. L. (2015). Dynamics of larch bud moth populations: application of Moran – Ricker models with time lag. Ecol. Modelling 297, 26–32. doi: 10.1016/j.ecolmodel.2014.11.003
Niinemets, Ü., Kännaste, A., and Copolovici, L. (2013). Quantitative patterns between plant volatile emissions induced by biotic stresses and the degree of damage. Front. Plant Sci. 4:262. doi: 10.3389/fpls.2013.00262
Niinemets, Ü., Tenhunen, J. D., Harley, P. C., and Steinbrecher, R. (1999). A model of isoprene emission based on energetic requirements for isoprene synthesis and leaf photosynthetic properties for Liquidambar and Quercus. Plant Cell Environ. 22, 1319–1335. doi: 10.1046/j.1365-3040.1999.00505.x
Pare, P. W., and Tumlinson, J. H. (1997). De novo biosynthesis of volatiles induced by insect herbivory in cotton plants. Plant Physiol. 114, 1161–1167. doi: 10.1104/pp.114.4.1161
Pazouki, L., Kanagendran, A., Li, S., Kännaste, A., Memari, H. R., Bichele, R., et al. (2016). Mono- and sesquiterpene release from tomato (Solanum lycopersicum) leaves upon mild and severe heat stress and through recovery: from gene expression to emission responses. Environ. Exp. Bot. 132, 1–15. doi: 10.1016/j.envexpbot.2016.08.003
Pinkard, E. A., Battaglia, M., Bruce, J., Leriche, A., and Kriticos, D. J. (2010). Process-based modelling of the severity and impact of foliar pest attack on eucalypt plantation productivity under current and future climates. Forest Ecol. Manage. 259, 839–847. doi: 10.1016/j.foreco.2009.06.027
Pitt, J. P. W., Régnière, J., and Worner, S. (2007). Risk assessment of the gypsy moth, Lymantria dispar (L), in New Zealand based on phenology modelling. Int. J. Biometeorol. 51, 295–305. doi: 10.1007/s00484-006-0066-3
Portillo-Estrada, M., Kazantsev, T., Talts, E., Tosens, T., and Niinemets, Ü. (2015). Emission timetable and quantitative patterns of wound-induced volatiles across different leaf damage treatments in Aspen (Populus Tremula). J. Chem. Ecol. 41, 1105–1117. doi: 10.1007/s10886-015-0646-y
Prieme, A., Knudsen, T. B., Glasius, M., and Christensen, S. (2000). Herbivory by the weevil, Strophosoma melanogrammum, causes severalfold increase in emission of monoterpenes from young Norway spruce (Picea abies). Atmos. Environ. 34, 711–718. doi: 10.1016/S1352-2310(99)00357-X
Riipinen, I., Yli-Juuti, T., Pierce, J. R., Petaja, T., Worsnop, D. R., Kulmala, M., et al. (2012). The contribution of organics to atmospheric nanoparticle growth. Nat. Geosci. 5, 453–458. doi: 10.1038/ngeo1499
Schade, G. W., and Goldstein, A. H. (2003). Increase of monoterpene emissions from a pine plantation as a result of mechanical disturbances. Geophys. Res. Lett. 30, 1380–1383. doi: 10.1029/2002GL016138
Schnitzler, J.-P., Louis, S., Behnke, K., and Loivamäki, M. (2010). Poplar volatiles – biosynthesis, regulation and (eco)physiology of isoprene and stress-induced isoprenoids. Plant Biol. 12, 302–316. doi: 10.1111/j.1438-8677.2009.00284.x
Scott, E. R., Li, X., Kfoury, N., Morimoto, J., Han, W.-Y., Ahmed, S., et al. (2019). Interactive effects of drought severity and simulated herbivory on tea (Camellia sinensis) volatile and non-volatile metabolites. Environ. Exp. Bot. 157, 283–292. doi: 10.1016/j.envexpbot.2018.10.025
Seidl, R., Thom, D., Kautz, M., Martin-Benito, D., Peltoniemi, M., Vacchiano, G., et al. (2017). Forest disturbances under climate change. Nat. Clim. Change 7, 395–402. doi: 10.1038/nclimate3303
Semiz, G., Erbilgin, N., and Holopainen, J. K. (2017). Hylobius abietis L. feeding on the novel host Pinus brutia Ten. increases emission of volatile organic compounds. J. Appl. Entomol. 141, 133–140. doi: 10.1111/jen.12310
Shallcross, D. E., and Monks, P. S. (2000). New directions: a role for isoprene in biosphere–climate–chemistry feedbacks. Atmos. Environ. 34, 1659–1660. doi: 10.1016/S1352-2310(99)00483-5
Shrivastava, M., Cappa, C. D., Fan, J., Goldstein, A. H., Guenther, A. B., Jimenez, J. L., et al. (2017). Recent advances in understanding secondary organic aerosol: implications for global climate forcing. Rev. Geophys. 55, 509–559. doi: 10.1002/2016rg000540
Sicard, P., Anav, A., De Marco, A., and Paoletti, E. (2017). Projected global tropospheric ozone impacts on vegetation under different emission and climate scenarios. Atmos. Chem. Phys. 17, 12177–12196. doi: 10.5194/acp-17-12177-2017
Silva, R. A., West, J. J., Lamarque, J.-F., Shindell, D. T., Collins, W. J., Faluvegi, G., et al. (2017). Future global mortality from changes in air pollution attributable to climate change. Nat. Clim. Change 7, 647–651. doi: 10.1038/nclimate3354
Stankovic, B., Jovanovic, J., and Adnadjevic, B. (2018). Application of the Suzuki–Fraser function in modelling the non-isothermal dehydroxylation kinetics of fullerol. React. Kinetics Mech. Catalysis 123, 421–438. doi: 10.1007/s11144-018-1380-6
Tholl, D., Sohrabi, R., Huh, J. H., and Lee, S. (2011). The biochemistry of homoterpenes - common constituents of floral and herbivore-induced plant volatile bouquets. Phytochemistry 72, 1635–1646. doi: 10.1016/j.phytochem.2011.01.019
Trnka, M., Muska, F., Semeradova, D., Dubrovsky, M., Kocmankova, E., and Zalud, Z. (2007). European Corn Borer life stage model: regional estimates of pest development and spatial distribution under present and future climate. Ecol. Modelling 207, 61–84. doi: 10.1016/j.ecolmodel.2007.04.014
Turlings, T. C. J., and Erb, M. (2018). Tritrophic interactions mediated by herbivore-induced plant volatiles: mechanisms, ecological relevance, and application potential. Annu. Rev. Entomol. 63, 433–452. doi: 10.1146/annurev-ento-020117-043507
Vickers, C. E., Gershenzon, J., Lerdau, M. T., and Loreto, F. (2009). A unified mechanism of action for volatile isoprenoids in plant abiotic stress. Nat. Chem. Biol. 5, 283–291. doi: 10.1038/nchembio.158
Visakorpi, K., Gripenberg, S., Malhi, Y., Bolas, C., Oliveras, I., Harris, N., et al. (2018). Small-scale indirect plant responses to insect herbivory could have major impacts on canopy photosynthesis and isoprene emission. New Phytol. 220, 799–810. doi: 10.1111/nph.15338
Vuorinen, T., Nerg, A. M., and Holopainen, J. K. (2004a). Ozone exposure triggers the emission of herbivore-induced plant volatiles, but does not disturb tritrophic signalling. Environ. Pollut. 131, 305–311. doi: 10.1016/j.envpol.2004.02.027
Vuorinen, T., Nerg, A. M., Syrjälä, L., Peltonen, P., and Holopainen, J. (2007). Epirrita autumnata induced VOC emission of silver birch differ from emission induced by leaf fungal pathogen. Arthropod Plant Interact. 1, 159–165. doi: 10.1007/s11829-007-9013-4
Vuorinen, T., Reddy, G. V. P., Nerg, A.-M., and Holopainen, J. K. (2004b). Monoterpene and herbivore-induced emissions from cabbage plants grown at elevated atmospheric CO2 concentration. Atmos. Environ. 38, 675–682. doi: 10.1016/j.atmosenv.2003.10.029
Warneke, C., Luxembourg, S. L., de Gouw, J. A., Rinne, H. J. I., Guenther, A. B., and Fall, R. (2002). Disjunct eddy covariance measurements of oxygenated volatile organic compounds fluxes from an alfalfa field before and after cutting. J. Geophys. Res. Atmosph. 107, ACH 6-1–ACH 6-10. doi: 10.1029/2001jd000594
Wilkinson, S., Mills, G., Illidge, R., and Davies, W. J. (2012). How is ozone pollution reducing our food supply? J. Exp. Bot. 63, 527–536. doi: 10.1093/jxb/err317
Yli-Pirilä, P., Copolovici, L., Kannaste, A., Noe, S., Blande, J. D., Mikkonen, S., et al. (2016). Herbivory by an outbreaking moth increases emissions of biogenic volatiles and leads to enhanced secondary organic aerosol formation capacity. Environ. Sci. Technol. 50, 11501–11510. doi: 10.1021/acs.est.6b02800
Keywords: ozone impact, herbivory, wounding, oxidative stress, induced emissions, BVOCs, modeling
Citation: Grote R, Sharma M, Ghirardo A and Schnitzler J-P (2019) A New Modeling Approach for Estimating Abiotic and Biotic Stress-Induced de novo Emissions of Biogenic Volatile Organic Compounds From Plants. Front. For. Glob. Change 2:26. doi: 10.3389/ffgc.2019.00026
Received: 06 March 2019; Accepted: 20 May 2019;
Published: 25 June 2019.
Edited by:
Silvano Fares, Council for Agricultural and Economics Research, ItalyReviewed by:
Steffen M. Noe, Estonian University of Life Sciences, EstoniaGuy Schurgers, University of Copenhagen, Denmark
Copyright © 2019 Grote, Sharma, Ghirardo and Schnitzler. This is an open-access article distributed under the terms of the Creative Commons Attribution License (CC BY). The use, distribution or reproduction in other forums is permitted, provided the original author(s) and the copyright owner(s) are credited and that the original publication in this journal is cited, in accordance with accepted academic practice. No use, distribution or reproduction is permitted which does not comply with these terms.
*Correspondence: Rüdiger Grote, cnVlZGlnZXIuZ3JvdGVAa2l0LmVkdQ==