- 1School of Environmental Sciences, University of Liverpool, Liverpool, United Kingdom
- 2R&D Seismology and Acoustics, Royal Netherlands Meteorological Institute (KNMI), De Bilt, Netherlands
- 3Department of Wildlife Ecology and Conservation, Fort Lauderdale Research and Education Center, University of Florida, Davie, FL, United States
- 4Department of Botany and Zoology, Stellenbosch University, Stellenbosch, South Africa
- 5Institute of Marine Sciences, University of California, Santa Cruz, Santa Cruz, CA, United States
- 6Department of Geoscience and Engineering, Delft University of Technology, Delft, Netherlands
- 7Global Fishing Watch Inc., Washington, DC, United States
- 8Cogitamus Lab, CNRS, Centre d’Écologie Fonctionnelle et Évolutive, Montpellier, France
- 9Department of Biology, University of Southern Denmark, Odense, Denmark
- 10Department of Zoology, University of Oxford, Oxford, United Kingdom
- 11Cluster of Excellence “Hearing4all” and Research Centre Neurosensory Science, Department of Neuroscience, School of Medicine and Health Science, Carl von Ossietzky University Oldenburg, Oldenburg, Germany
- 12Max Planck Research Group Neurobiology of Magnetoreception, Center of Advanced European Studies and Research (CAESAR), Bonn, Germany
- 13Asociación Ipar Perspective, Sopela, Spain
- 14British Antarctic Survey, Natural Environment Research Council, Cambridge, United Kingdom
- 15Netherlands Organisation for Applied Scientific Research (TNO), The Hague, Netherlands
- 16Institute for Biodiversity and Ecosystem, Dynamics, University of Amsterdam, Amsterdam, Netherlands
Seabirds are amongst the most mobile of all animal species and spend large amounts of their lives at sea. They cross vast areas of ocean that appear superficially featureless, and our understanding of the mechanisms that they use for navigation remains incomplete, especially in terms of available cues. In particular, several large-scale navigational tasks, such as homing across thousands of kilometers to breeding sites, are not fully explained by visual, olfactory or magnetic stimuli. Low-frequency inaudible sound, i.e., infrasound, is ubiquitous in the marine environment. The spatio-temporal consistency of some components of the infrasonic wavefield, and the sensitivity of certain bird species to infrasonic stimuli, suggests that infrasound may provide additional cues for seabirds to navigate, but this remains untested. Here, we propose a framework to explore the importance of infrasound for navigation. We present key concepts regarding the physics of infrasound and review the physiological mechanisms through which infrasound may be detected and used. Next, we propose three hypotheses detailing how seabirds could use information provided by different infrasound sources for navigation as an acoustic beacon, landmark, or gradient. Finally, we reflect on strengths and limitations of our proposed hypotheses, and discuss several directions for future work. In particular, we suggest that hypotheses may be best tested by combining conceptual models of navigation with empirical data on seabird movements and in-situ infrasound measurements.
Introduction
Birds conduct some of the longest migrations in the animal kingdom (e.g., Croxall et al., 2005; Egevang et al., 2010), and are considered model organisms for the study of animal navigation (Wallraff, 2005; Guilford et al., 2011). Navigation is a complex multi-sensory behavioural task and the importance of visual, olfactory and magnetic senses for birds is long-established (Wiltschko and Wiltschko, 2003; Lohmann et al., 2008; Nevitt, 2008; Mouritsen, 2018). Many seabird species spend the majority of their lives navigating the oceans and are known to rely heavily on visual and olfactory cues. Albatrosses and petrels (Procellariiformes) in particular have a highly developed sense of smell, with among the largest olfactory bulbs of any bird species (Bang, 1966; Corfield et al., 2015). When their sense of smell is experimentally impaired, seabirds not only take longer to home (Pollonara et al., 2015), but follow coastlines to a greater degree, emphasising that visual cues become more important when olfactory cues are unavailable (Pollonara et al., 2015; Padget et al., 2017). While recent work has also shown that seabirds can use a time-compensated sun compass (Padget et al., 2018), a reliance on vision, celestial cues or an olfactory map are unlikely to fully explain the ability of some seabirds to navigate under all conditions, including homing to the breeding colony from thousands of km away, over visually featureless terrain and regardless of variation in wind which influences odor plume dynamics (Nevitt et al., 2008). As such, there are gaps in our understanding of navigation (Alerstam, 2006), where commonly studied cues may be absent or not sufficiently informative (Bonadonna et al., 2003).
Many animals, including birds, in both marine and terrestrial environments, can use geomagnetic information for navigation (e.g., homing pigeons Columba livia, green turtles Chelonia mydas, sockeye salmon Oncorhynchus nerka; Dennis et al., 2007; Benhamou et al., 2011; Putman et al., 2013). Seabirds may also rely on a magnetic compass, i.e., to get directional information from the geomagnetic field. There was, however, no convincing evidence from studies that birds, including seabirds, extracted locational information from the geomagnetic field (Benhamou et al., 2003; Bonadonna et al., 2005), until a recent study suggested that juveniles use such information when returning to the colony for the first time (Wynn et al., 2020). The magnetic cues may provide locational information at intermediate scales (tens of kilometers) because the magnetic sensory resolution is probably too low to enable an animal to perform small-scale navigation. Equally, the geomagnetic anomalies crossed at large scale may lead birds to misinterpret their current location, and to be trapped in wrong places characterised by the same geomagnetic signature as its goal (Benhamou et al., 2011). Furthermore, the use of the Earth’s geomagnetic field as a bi-coordinate map may be limited in some regions (Åkesson and Alerstam, 1998). Hence, although an ability to use magnetism is intuitively the most likely of the candidate sensory mechanisms, studies have thus far failed to prove its role in explaining the impressive long-distance navigational capabilities of seabirds, despite attempts to interrupt magnetic navigation in homing experiments (Gagliardo et al., 2013; Pollonara et al., 2015).
Another potential source of sensory information that has received less attention is sound, despite its prevalence in the environment and the sophisticated hearing of birds (Theunissen and Shaevitz, 2006). In particular, infrasound (i.e., low-frequency inaudible sound) is a key feature of the earth-ocean-atmosphere system that can propagate over extremely long ranges (of the order of thousands of kilometers). Empirical work has found evidence that homing pigeons can perceive infrasound at frequencies as low as 0.05 Hz (Kreithen and Quine, 1979), suggesting they could respond to infrasound as an environment-borne stimulus (Hagstrum, 2000, 2013, 2019). Long-distance avoidance of meteorological events by birds might also be explained by their ability to detect, and respond navigationally, to infrasound (e.g., Streby et al., 2015). While soundscape ecology has become a well-established field (Farina, 2014), the idea of infrasound use for navigation remains controversial (e.g., Wallraff, 2014; Lisovski et al., 2018), as little is known about infrasound detection mechanisms in birds (Zeyl et al., 2020), and what information infrasound would provide as a navigational cue.
Here, we integrate knowledge and ideas from several research fields to provide a framework for investigating the potential use of infrasound for navigation of seabirds (and birds more generally) in the aerial marine environment. We briefly review the physics of infrasound, including sources, propagation dynamics and detection, and then review the known infrasonic auditory capabilities of birds. We evaluate the potential for birds to use infrasound cues as beacons, landmarks, or gradients for navigation tasks and propose future directions to advance this field.
Atmospheric Infrasound in the Marine Environment
Sound waves in air can be represented as elastic waves that temporarily bring air particles locally in motion while propagating at approximately 334 m/s at mean sea level temperature (∼15 °C). As a sound wave passes by, air particles oscillate from side to side around their resting positions and alternate between being compressed or rarefied (i.e., thinned out; Figure 1A). Acoustic energy consists of a continuous alteration of potential energy, akin to when a pendulum is lifted and air particles are compressed, and kinetic energy which is associated with the pendulum movement itself and particle motion (Pain, 2006; Pierce, 2019).
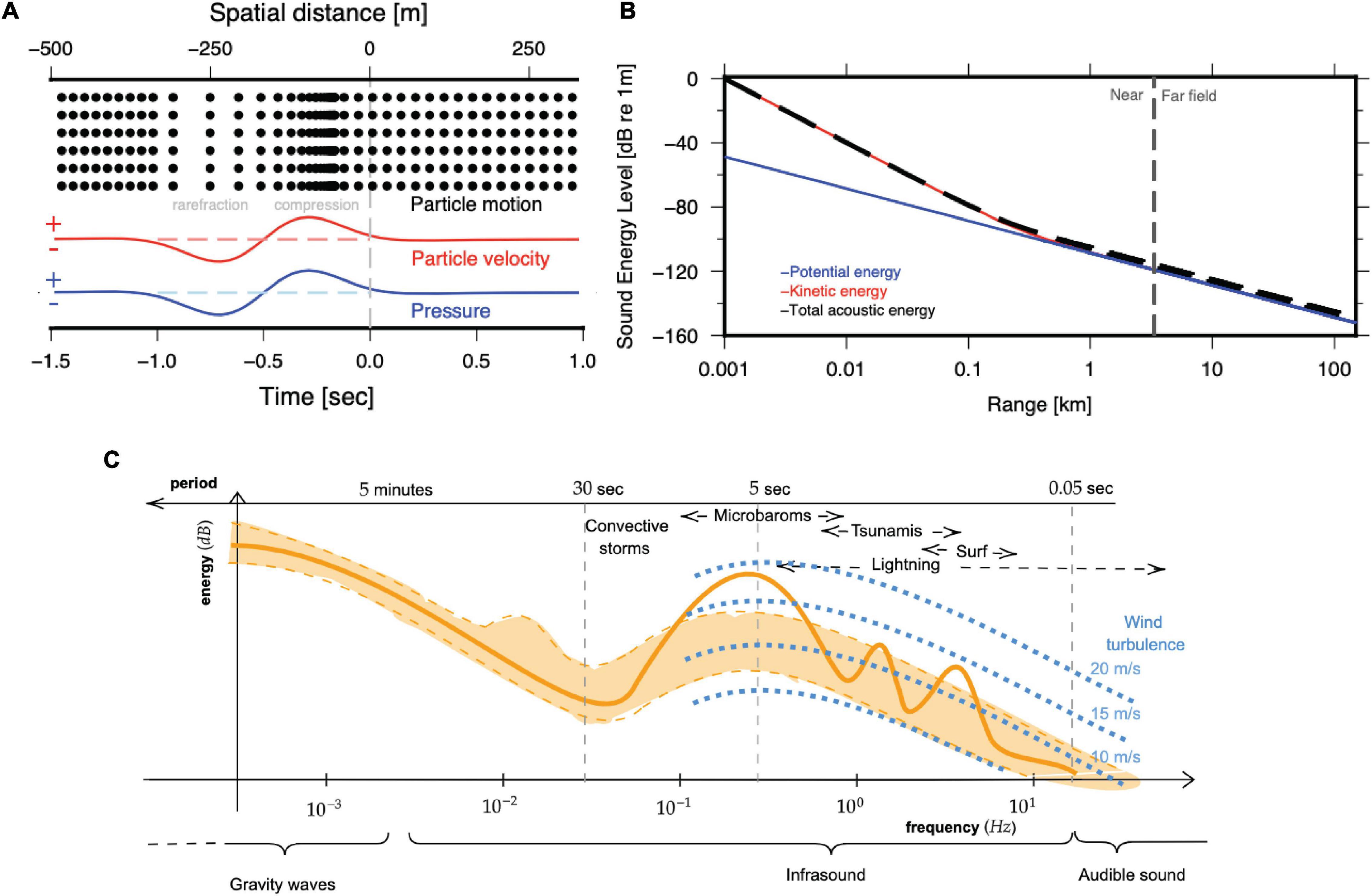
Figure 1. Infrasonic wave-field parameters. (A) Depiction of an acoustic wave (black dots) as alternating compressions and rarefactions, leading to perturbations in particle velocity (red) and pressure (blue). (B) The decrease and partitioning of acoustic energy with range and transition from near- to far-field for a spherical sound wave at 0.2 Hz. The total acoustic energy (dashed black) is composed of potential (blue) and kinetic (red) energy, related to pressure and particle motion, respectively. While kinetic energy dominates in the near-field, both forms are in equilibrium in the far-field. (C) Schematic representation of pressure spectra due to coherent infrasound (shaded orange) and wind noise (blue); the orange shaded area between the dashed lines represent average background quantities, while the solid line represents a random snapshot with three spectral speaks. Acoustic signal detection is possible if wind noise levels are below the acoustic signal power, i.e., when the dashed blue line is below the solid orange line, which depends on the source power as well as the wind speed.
The sound frequency represents the number of these oscillations per second and a Power Spectral Density (PSD) quantifies how the signal power varies with frequency. The computation of the PSD involves a Fourier transform. The wavelength is defined as the distance between two crests or zero crossings (e.g., Figure 1A). The relationship between the frequency f, wavelength λ and speed of sound c is given as λf = c.
The acoustic field at any location is fully described by the perturbations in pressure p (sound pressure; unit pascal or Pa) and particle speed (unit m/s) as a function of frequency (unit Hertz or Hz). The sound pressure level (SPL) in air is commonly expressed in decibel (dB), relative to the reference value of p0 = 20μPa and is computed as
Particle velocity defines the propagation direction of a passing sound wave and is proportional to the spatial gradient of the acoustic pressure. The sound intensity quantifies the acoustic power and directivity and is calculated as the product of sound pressure and particle velocity, i.e., (Pierce, 2019). Sound intensity is a vector that points away from the sound source. To determine the direction to the sound source in the free-field, multiple pressure sensors must be positioned in an array (den Ouden et al., 2020) or alternatively, particle motion sensors can be utilised to directly measure acoustic particle motion direction, although with 180° ambiguity.
The intensity of the signal decreases with distance from the source, with most rapid decrease in the near-field (Figure 1B). The total sound energy density is computed as the sum of both acoustic kinetic and potential energy densities (note the logarithmic scale in Figure 1B). Note that while the kinetic energy dominates in the near-field, both are at equilibrium in the far-field.
The frequency range of atmospheric infrasound is typically defined between 3.3 mHz and 20 Hz. Infrasonic waves with frequencies below 3.3 mHz do not fit within the Earth’s atmosphere. Above 20 Hz, sound becomes audible to humans.
Infrasound can be produced across various frequency bands (Figure 1C), by displacements of large volumes of air, either from geophysical or anthropogenic sources. We provide an overview of infrasound source types in Supplementary Material Appendix 1 (adapted from Campus and Christie, 2010). Two main signal types are defined: (1) transient signals are considered to have a finite, short-term duration, in contrast to (2) continuous signals. In practice, signals may also share characteristics from both signal types.
In the marine environment, several infrasonic sources have been identified. Standing ocean waves produce a near-continuous hum in a broad frequency range as microbaroms (0.1–1.0 Hz), both in the open sea and near coastlines (den Ouden et al., 2020). The interaction of the ocean waves produces pressure waves that reverberate within the ocean and radiate into the atmosphere and solid earth. Microbarom source regions in the ocean can be quasi-stationary as well as transitory (e.g., when associated with marine storms). Surf can also be observed near coastlines at higher frequencies, typically above 1.0 Hz (Park et al., 2008). Transient sources of infrasound that are routinely detected in the marine environment include severe weather, earthquakes, meteor explosions and volcanic eruptions. Notably, infrasound can radiate from sources underwater as sound passes readily between air and water at low frequencies (Evers et al., 2014). Figure 2 shows an illustration of the infrasonic wavefield at sea.
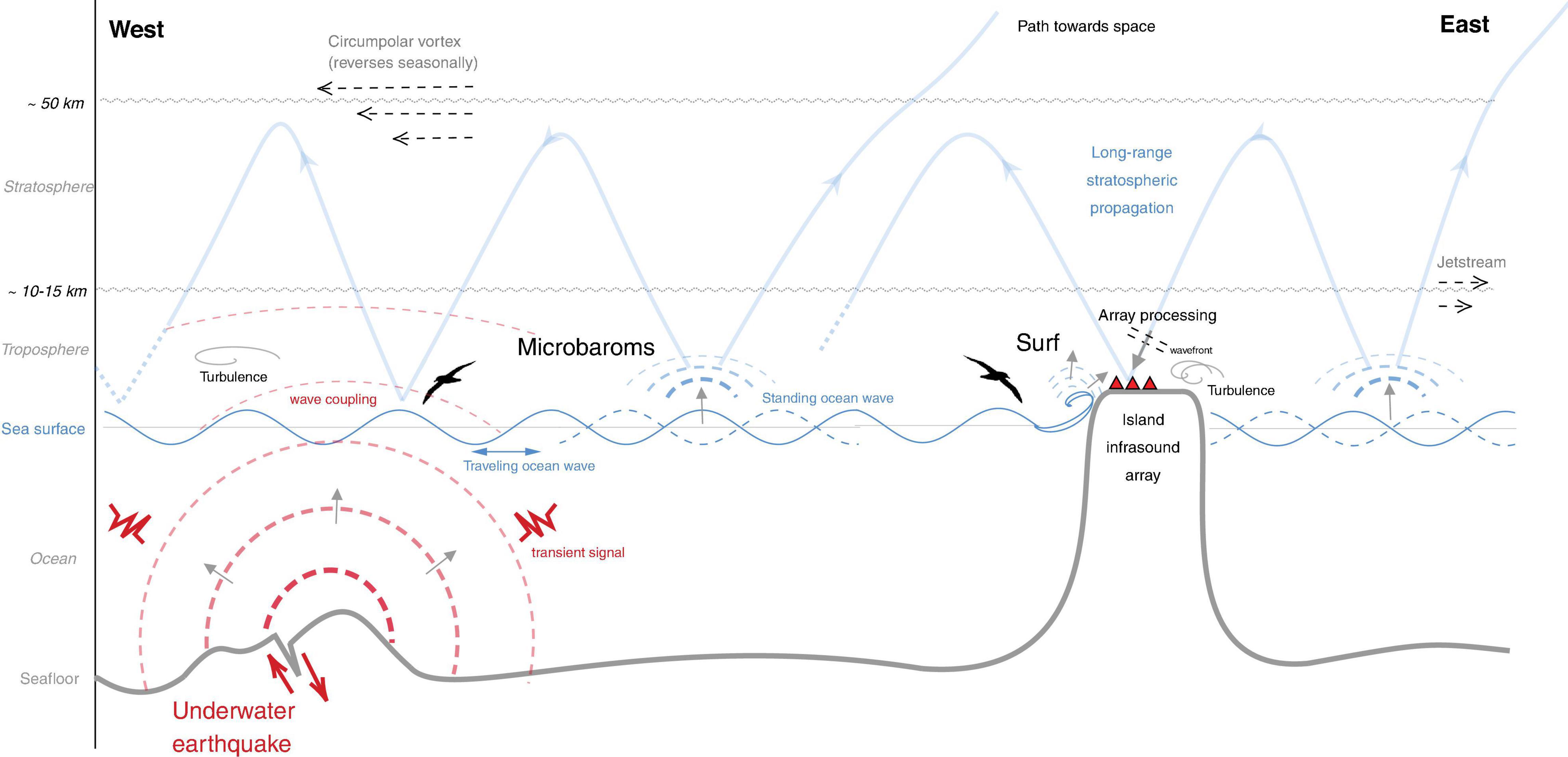
Figure 2. A schematic representation of the marine infrasonic wavefield. The ocean produces continuous acoustic background noise (i.e., microbaroms and surf; depicted in blue). Microbaroms can propagate over long distances downwind (i.e., from east to west) in the stratospheric waveguide. In the upwind direction, microbaroms are not guided and propagate toward space. The wind direction changes seasonally. Surf infrasound is generated near coastlines and does not propagate over long distances. Transient acoustic signals (red) also occur in the marine environment, e.g., following an underwater earthquake. These vibrations can couple into the atmosphere. Besides the acoustic signals, the wavefield also consists of turbulence, which may mask signals of interest. Array processing techniques can be used to detect coherent acoustic waves in a turbulent atmosphere.
In the study of infrasoundscapes (den Ouden et al., 2021b), various factors must be considered. The infrasonic wavefield is complex and is composed of diverse source contributions (Campus and Christie, 2010). The atmospheric environment plays an important role in detection (also see Figure 2):
(1) The wind and temperature distribution throughout the atmosphere determine along which paths infrasonic waves can propagate (Waxler and Assink, 2019) as well as the absorption along the propagation path (Sutherland and Bass, 2004).
(2) Wind conditions near infrasound sensors determine local turbulence levels that are detected as (non-acoustic) pressure variations, along with infrasound (Raspet et al., 2019).
The observations on the global infrasound array network (Marty, 2019) have advanced knowledge of infrasound sources, atmospheric infrasound propagation and the effects of turbulence on detection. The global infrasound network is part of the International Monitoring System (IMS), which is being installed for the verification of the Comprehensive Nuclear-Test-Ban Treaty. Since the certification of the first array in 2001, 54 out of the eventual 60 arrays have been installed. The IMS infrasound arrays are distributed rather uniformly over the globe.
Earlier studies have shown that the infrasound detection statistics vary significantly between IMS arrays (Matoza et al., 2013; Assink et al., 2014; Ceranna et al., 2019). Each array has its own characteristic background noise with specific infrasonic sources that are routinely detected (see Supplementary Material Appendix 4). Microbarom signals tend to be present at most infrasound arrays, in particular when arrays are situated near the ocean. While some of the observed infrasound originates more locally to the array, other signals originate from much further away: propagation can occur over thousands of kilometres and can even be global for exceptionally powerful signals, such as the 2013 Chelyabinsk meteor airburst (Brown et al., 2013).
Besides differences, observed pressure spectra also show commonalities, both in terms of infrasonic content as well as pressure due to wind noise. Figure 1C shows schematic PSDs. The shaded orange area, between the dashed lines, represent average acoustic background noise levels (Matoza et al., 2013). The solid orange line represents a snapshot for a shorter time-period. During the period three source contributions are highlighted as spectral peaks. The (non-acoustic) wind noise levels are described by a turbulence spectrum (Raspet et al., 2019), which depends on the wind speed. The acoustic signals can be detected if the wind noise levels (dashed blue lines) are below the acoustic signal power (orange line). The signal strength depends on the acoustic source strength and the transmission loss that a signal experiences propagating from source to receiver.
Propagation losses are determined by geometrical spreading, i.e., the increase in area the sound wave covers (Figure 1B), and intrinsic absorption. Because the acoustic absorption strongly increases with frequency, it follows that signals with higher frequency content tend to originate from shorter ranges. Conversely, lower frequencies can originate from much larger distances. The absorption coefficients are dependent on the temperature and air composition, including humidity near the surface (Sutherland and Bass, 2004).
Up to distances of a few kilometres, it can be assumed that the atmospheric environment is homogeneous, implying that infrasound propagates along straight paths. More generally, the atmosphere is an inhomogeneous medium that is predominantly vertically layered. Within the atmosphere, infrasound is refracted by gradients in temperature and wind and can efficiently propagate downwind in waveguides that form between the Earth surface and the atmosphere aloft (Figure 2). Large-scale wind currents around the tropopause (the jet stream around 10–15 km) and stratopause (the circumpolar vortex around 50 km), form the upper limits of the tropospheric and stratospheric waveguides, respectively. The characteristic waveguide propagation paths arise because vertical temperature and wind gradients within waveguides are such that upward refracted sound waves are bent back down to a reflective Earth surface (Figure 2). In the upwind direction, propagation efficiency is less and therefore infrasound propagation is highly anisotropic (Waxler and Assink, 2019).
The seasonal reversal of the circumpolar vortex, combined with enhanced downwind propagation efficiency, leads to seasonal variations in long-range infrasound propagation conditions that affect the observations. The dynamics of these winds strongly varies with latitude and are intimately connected to the global atmospheric circulation (Smets, 2018). From the perspective of a bird flying over the ocean, this could imply that a bird located downwind of a sound source would experience higher levels of sound, while a bird located upwind would sense lower sound levels.
Can Infrasound Be Detected by Birds?
Despite the vast diversity of birds (>9,000 species), we only know the hearing capabilities of <50 species (Dooling et al., 2000; Gleich and Langemann, 2011). Among bird species whose hearing has been tested, only five species have been measured in the low frequency range using electrophysiological or behavioural approaches, with four out of five species demonstrating hearing ability below 20 Hz, i.e., in the infrasound range (Kreithen and Quine, 1979; Theurich et al., 1984; Hill et al., 2014; Heffner et al., 2020). Hearing thresholds below 20 Hz are generally greater than 50 dB relative to 20 μPa sound level pressure (SPL), and increase toward lower frequencies, although this varies across species (see Figure 1 in Zeyl et al., 2020). The more sensitive species tested such as the domestic chicken Gallus gallus have infrasonic hearing thresholds comparable to those recorded for Indian elephants Elephas maximus (Zeyl et al., 2020). Thus, there is evidence that some birds can hear infrasound, but due to the low taxonomic coverage, whether this ability is more widespread remains unknown. Furthermore, distinguishing responses of birds to acoustic as opposed to non-acoustic perturbations can be challenging (Zeyl et al., 2020), and there are currently no empirical data on potential infrasonic hearing sensitivity in any seabird species.
The degree to which infrasound could provide useful navigational information to birds depends not only on their ability to perceive it, but critically on the acuity with which birds can resolve small differences in amplitude and frequency that allow for sound localization. Unfortunately, current knowledge of the hearing mechanisms at infrasonic frequencies is limited. Multiple hearing pathways and receptor organs could be involved in the perception of infrasound, reaching the inner ear either through the middle ear, or through extra-tympanic pathways that rely on body or head vibrations. In addition to the auditory inner ear receptors, vestibular receptors could be involved (Zeyl et al., 2020). The auditory system of birds encodes changes in infrasound frequency better than amplitude (Warchol and Dallos, 1989; Schermuly and Klinke, 1990). Behavioural sound localisation thresholds in the infrasonic range have not been determined in any bird.
We propose three potential mechanisms by which birds could detect infrasound direction. First, as an organ sensitive to acoustic pressure (a scalar quantity), the avian middle and inner ear do not inherently encode direction relative to the bird. However, sound direction could be estimated by comparing sound received across two sensors to infer interaural time and level differences (Klump, 2000). As the wavelengths of infrasound are much larger than the distance between the ears, there should be minimal differences in the amplitude and phase of the cycle as the sound travels from one ear to the other. Interaural differences could be extended by a coupling of the middle ears by cranial air spaces or interaural canals, acting as pressure difference receivers (Michelsen and Larsen, 2007; Christensen-Dalsgaard, 2011; Supplementary Material Appendix 2). Interaural enhancements have been demonstrated in several bird species, such as budgerigars Melopsittacus undulatus (Larsen et al., 2006), owls Tyto alba (Kettler et al., 2016), and domestic chickens (Hyson et al., 1994). The magnitude of the interaural time and level cues available to birds at infrasonic frequencies remains unquantified with experimental data, as well as their ability to encode those differences. The interaural cues will vary among birds due to different interaural attenuation through the cranial air spaces. Experimental data and mathematical models suggest that interaural differences in time rather than level may play a dominant role at low frequencies (Supplementary Material Appendix 2).
Second, sound direction could be determined by the frequency shift caused by the Doppler effect: flying toward the sound source will shift the perceived frequency upward as a function of flight speed, whereas flying away from the sound source will shift frequency downward (Figure 3A; similar to the sound of a siren shifting pitch down as it moves away from the receiver).
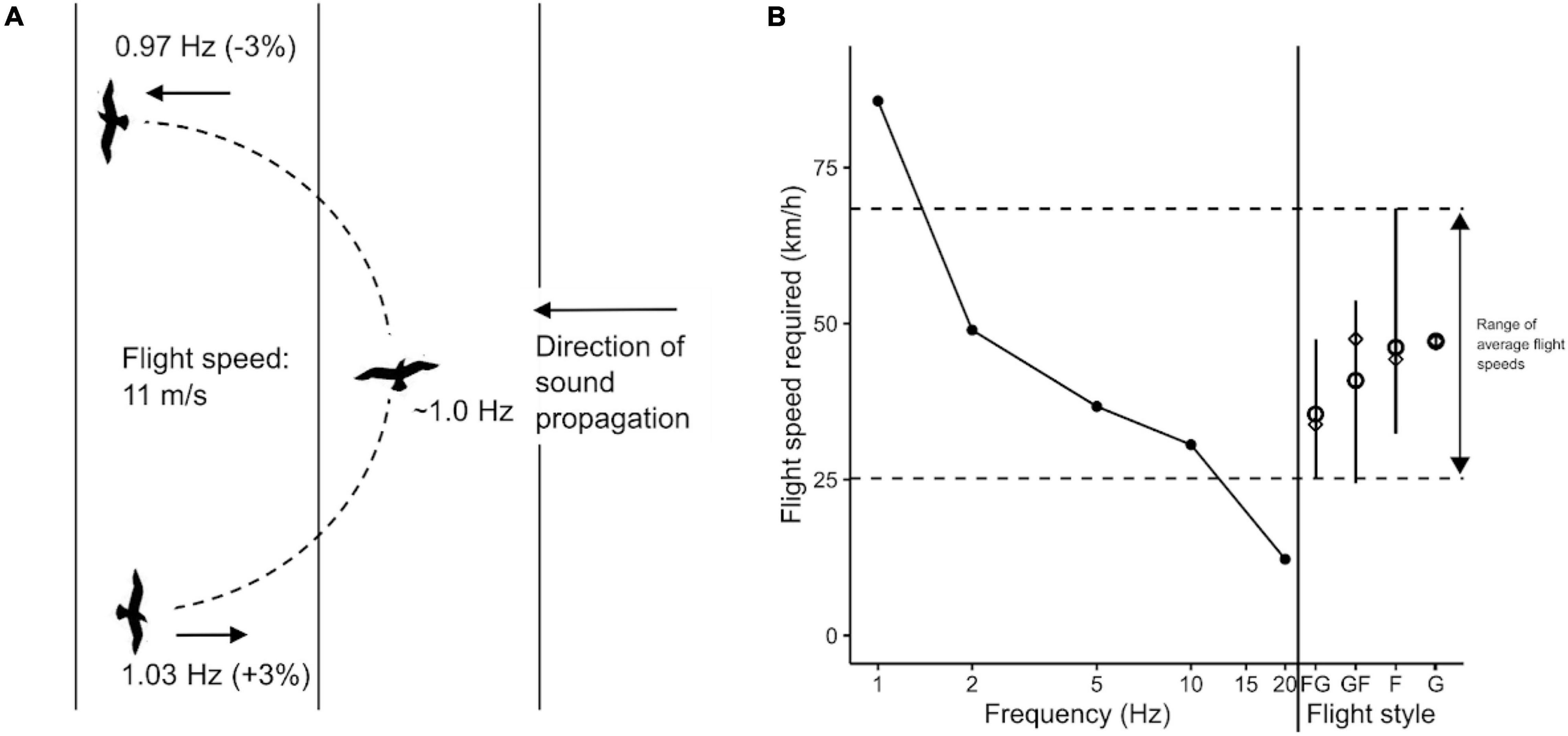
Figure 3. Perception of direction through frequency shift caused by the Doppler effect during flight. (A) Given a stationary 1 Hz infrasound signal, a bird flying in the acoustic far-field at a ground speed of 40 km/h (11 m/s) toward or away from the source receives a frequency that is shifted up or down by 3% (i.e., to 1.03 or 0.97 Hz), relative to the frequency received by a stationary bird, or a bird flying perpendicularly to the signal. For indirect flight angles relative to the signal direction, the frequency shift will be less than ±3%. Wavefronts of the propagating sound wave are indicated with vertical lines. (B) The range in average ground speeds of seabirds in crosswind flight (dashed lines) are compared to the estimated flight speeds that would be required to produce the frequency shift thresholds determined empirically in pigeons by Quine and Kreithen (1981) (black dots). To the right, average flight speeds are grouped by different flight styles (FG, flap-gliders; GF, glide-flappers; G, gliders; F, flappers), with means (dots), medians (diamonds), and range (lines). Data for average flight speeds are taken from Table 3 of Spear and Ainley (1997), with each flight group containing 2–11 mean flight speeds. These results suggest that seabird flight speeds could be sufficient to produce a detectable frequency shift (for details of calculations, see Supplementary Material Appendix 3).
The equation for the frequency shift caused by the Doppler effect is: f = ((c + vr)/(c + vs)) × f0, where f identifies the received frequency, f0 is the emitted frequency, c is the speed of sound, vr is the velocity of the receiver/bird (positive values indicating movement toward the sound), and vs is the velocity of the sound source (positive values indicating movement toward the receiver/bird). The magnitude of the frequency shift depends on the bird’s flight velocity and the direction of the flight path relative to the sound source. A greater flight velocity produces a larger frequency shift relative to a stationary position, and a flight path oriented directly toward or away from the sound source will elicit the greatest relative velocity between the receiver and source.
The hypothesis that birds use the Doppler shift to determine sound direction requires that the bird has an ability to perceive small frequency modulations at infrasonic frequencies. There are almost no empirical data on the perceptual abilities of birds in this regard for low frequencies, but a study in pigeons by Quine and Kreithen (1981) found that as frequencies were lowered from 20 Hz down to 1 Hz, the thresholds for the detection of frequency shifts increased from 1 to 7 %. If sensitivities of seabirds to frequency shifts across frequencies are similar to those determined for pigeons (Quine and Kreithen, 1981), use of the Doppler effect is plausible but would be more effective at higher infrasonic frequencies, where the sensitivity to frequency shifts is greater (Figure 3B and Supplementary Material Appendix 3).
Third, birds could perceive the particle motion direction directly through stimulation of the otolithic vestibular organs (saccule, utricle, and lagena). The different orientations of the hair cells on each vestibular organ, and of the organs themselves, allow for animals to detect vibration in multiple directions (Si et al., 1997), although with a 180° ambiguity, as the particle motion is occurring equally toward and away from the sound source (Sisneros and Rogers, 2016). Though not studied in birds, evidence in other species (frogs and humans) indicates that low frequency airborne sound can stimulate the otolith organs (Moffat and Capranica, 1976; Rosengren et al., 2010). Theoretically, if both pressure and particle motion are simultaneously perceived by the inner ear, the 180° ambiguity could also be resolved by a neural computation of the phase differences between the two acoustic components, so-called “phase models” of directional hearing (Sisneros and Rogers, 2016).
Infrasound as a Navigational Cue
Organisms can use several mechanisms for navigation, relying on environmental information and spatial memory, available simultaneously at different spatial scales (Mueller and Fagan, 2008). Here, we focus on navigation based on the perception of the infrasound signature of spatial features, such as a target location. From a signal perspective, this acoustic information can be perceived as discrete objects (beacons, landmarks) or continuous signals (gradients) (Figure 4).
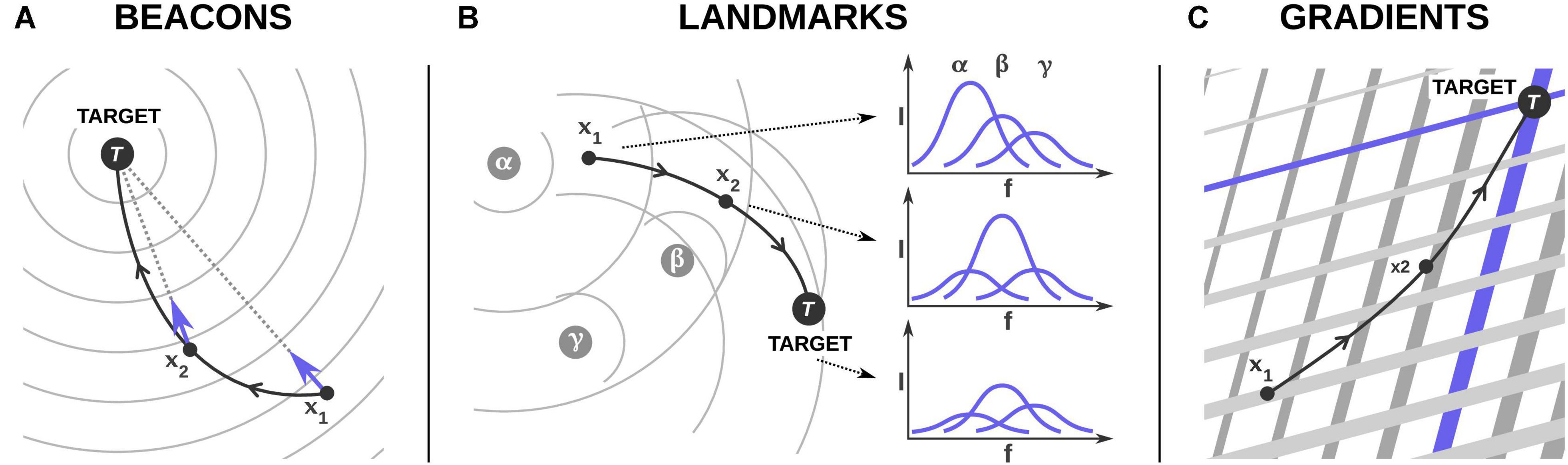
Figure 4. A navigation framework for infrasound. We propose three non-exclusive scenarios for the use of infrasound for navigation by birds as (A) beacons, (B) landmarks, and (C) gradients. We consider a hypothetical bird trajectory (in black) sampled at locations x1, x2 and the target at times 1, 2, and 3. In each panel, blue elements indicate the relevant information used by the bird. (A) In the beacon hypothesis, the target location, e.g., the bird’s colony is the infrasound source that the bird detects and moves toward. This hypothesis requires the bird either to perceive directly the direction of the beacon, shown here by the blue arrows and optimise its path toward it (taxis), or at least to detect whether it is approaching or moving away the target. In this case, the bird could rely only on variations of field intensities (differential klinokinesis) without the need to sense the gradient direction. (B) α, β, and γ are three infrasound sources (e.g., islands). The combination of their infrasound signals in space provide a unique acoustic landmark in the form of an infrasound signature at the target (power spectrum in blue, I is the sound intensity and f the frequency); power spectra at x1 and x2 are compared to the power spectra at x1 to reassess location. (C) Large-scale infrasonic amplitude gradient fields can provide a functional coordinate reference system. We illustrate this scenario with two gradient fields from two different sources (gradient isolines are represented by grey parallel lines, with directions orthogonal to the gradient directions, and widths proportional to the signal intensities). The bird could use the gradient fields as a coordinate system, guiding it to navigate toward its desired target characterised by specific intensities (blue arrows).
A beacon is a detectable feature with a characteristic, but not necessarily unique, signature that stands at a target location and provides information linked to its direction, assuming the source is stable over time, like a light bulb for a moth (Figure 4A). The beacon can generate a radial gradient field around it, which can be used by an organism to move toward it. A classic example is provided by bacteria orienting in a chemical gradient field (Benhamou, 2010). The presence of acoustic beacons is supported by empirical (e.g., Moron and Andriolo, 2015) and experimental studies (e.g., Shettleworth and Sutton, 2005; Stansbury et al., 2015). For instance, sound made by conspecifics has been shown to act as a beacon in wood frogs Lithobates sylvaticus (Bee, 2007), and spinner dolphins Stenella longirostris (Moron and Andriolo, 2015). Animals may use any acoustic source as a beacon if they can perceive the direction of the source of sound.
In contrast, landmarks are also detectable features, but do not stand at a target location; they are used instead as an element of a frame of reference in which the animal can encode both its position and the goal location (Benhamou, 2010; Chan et al., 2012; Figure 3B). Landmarks must provide a signature that is stable and distinguishable in the environment, in direction and/or intensity. While use of a visual landmark is thought to be common in animals (including humans) in route finding (Nardini et al., 2008; Chan et al., 2012), the use of acoustic landmarks has been poorly studied, with most research focused on humans (Jetzschke et al., 2017), or echolocating species (e.g., bats; Jensen et al., 2005). Several acoustic sources may result in a mixture of acoustic signals at a given location providing a unique signature, possibly in both the frequency and amplitude domains (Figure 3B). As different types of infrasound sources differ in frequency and amplitude profiles, it may be possible that an animal can distinguish acoustic landmarks from the acoustic profiles at specific locations and use them until it reaches the target (Figure 3B).
A gradient field occurs when some physical or chemical factors of the environment vary monotonically in space; the gradient giving the rate of change in intensity in every point in the field. When there are several gradient fields intersecting with some marked angle, the intensity, or ratio of intensities, and the direction of gradients may be directly perceived (e.g., as proposed by Wallraff (2000) for homing pigeons). Thus intersecting gradient fields can provide a functional coordinate system (Benhamou, 2003; Figure 4C). To our knowledge, the use of acoustic gradients has not been tested yet. As microbaroms and surf are quasi-continuous in nature, they could act as infrasonic gradient fields for navigation over long distances. For example, an animal could use a static source such as a gradient field created by microbaroms on a continental coastline to orient relative to the coast. Given that microbarom source regions are louder in areas with persistently strong winds (Smets, 2018; De Carlo et al., 2020), the general trend for increased ocean surface wind speeds at higher latitudes may potentially provide a large-scale gradient field. Although microbaroms propagate over large distances, less information is provided in the far- than near-field as the intensity decreases exponentially with distance (Figure 1B).
How Might Seabirds Use Infrasound for Navigation?
We have outlined how infrasound could act as a navigational cue, likely as a component of a multimodal system, whereby animals use several cues across a range of spatial scales. As many infrasound sources may also provide information alongside other types of navigational cues, such as the visual and infrasound information gained from islands, it may not be possible to determine whether birds use one or several types of information. However, infrasound is likely to provide information over much larger spatial scales than many other navigational cues, and so we suggest it can provide additional information to more widely studied cues (Figure 5 and Supplementary Material Appendix 1). In Figure 5 we provide a schematic proposing possible spatial scales at which key sensory modalities (vision, olfaction, magnetoreception, hearing) and proposed infrasound scenarios may be used by seabirds to obtain spatial information.
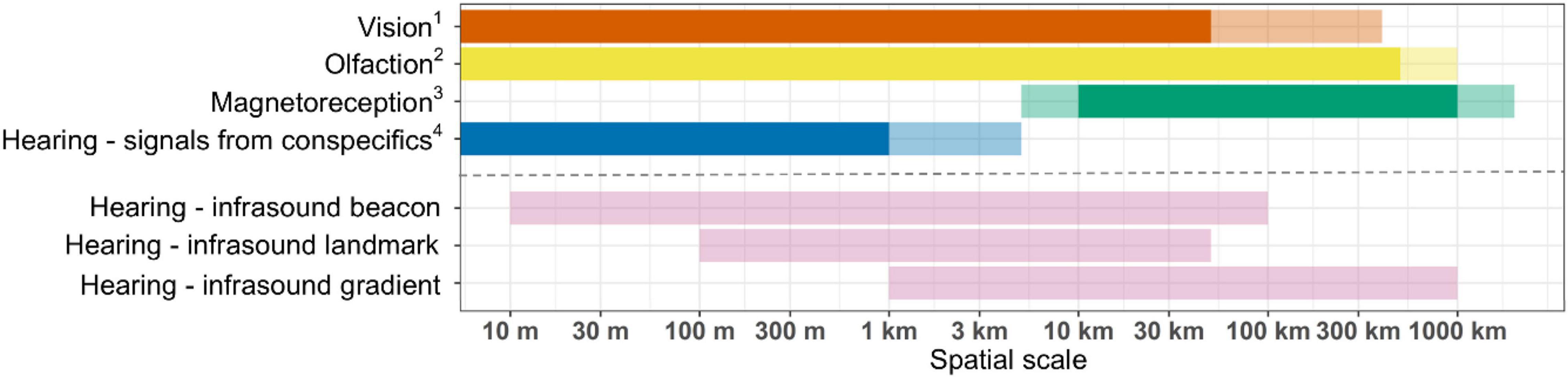
Figure 5. The predominant sensory modalities used by seabirds and the hypothesised spatial scales over which they operate (above dotted line) as well as the hypothesised scales over which infrasound hypotheses are proposed to provide information (below dotted line). The known range at which animals may be using each sensory modality is based on the literature and highlighted by a bright colour, while the probable or hypothesised range is shown by a faded colour. Each infrasound hypothesis is provided separately, shown in faded pink. Note that for several senses (e.g., vision, olfaction) the hypothesised scales incorporate the ranges at which animals have been proposed to use learned map-like information. The spatial scale is presented on a log10 scale and ranges from 0 m to 2,000 km. 1Collet et al. (2015), Pollonara et al. (2015), and Padget et al. (2017). 2Nevitt (2008), Nevitt et al. (2008), and Reynolds et al. (2015). 3Wynn et al. (2020). 4Thiebault et al. (2016).
Some seabird species home in a straight line (Catard and Weimerskirch, 1999, movement data drawn as Figure 2 in Benhamou et al., 2003; Padget et al., 2019), and because infrasound is not influenced by surface-level wind fields to the same degree as odour plumes, birds could use an infrasound beacon over large spatial scales, provided that they are able to identify the direction of the sound source and where this source is located close to the goal (Figure 4A). However, there are several limitations with a directional hearing mechanism along these lines, given seabird flight speeds and interaural time differences (see section “Can Infrasound be Detected by Birds?”). Infrasound landmarks have been suggested for pigeons (e.g., Hagstrum, 2013), but there is a lack of comprehensive empirical support (Wallraff, 2014). Research into other navigational cues has shown that birds (including seabirds) do rely on olfactory and visual landmarks (e.g., Nevitt, 2008; Guilford and Biro, 2014; Pollonara et al., 2015). To use infrasonic landmarks, seabirds would need to recognise acoustic signatures in the infrasonic range, based on frequency and amplitude (Figure 4B), and memorise features associatively or encode their location in space (Biro et al., 2004). The auditory pathway of birds has the capacity to encode changes in amplitude and frequency at infrasonic ranges (Warchol and Dallos, 1989; Schermuly and Klinke, 1990), but the acuity for resolving subtle spectral differences is unknown.
Evidence of use of navigation gradient fields by seabirds is mixed, particularly variations in the geomagnetic field as a possible component of a gradient map (Åkesson and Alerstam, 1998; Bonadonna et al., 2005). To rely on an infrasonic gradient field, birds need to detect either particle motion, with relatively large values in the near-field, or the pressure amplitude of the signal. Moving up an infrasound gradient field (whether the bird is sensitive to particle motion or pressure) would require birds to sample the environment as they move.
Although the long-range propagation of infrasound, and the quasi-continuous nature of microbaroms and surf sources, makes it an appealing cue for navigation, the signal-to-noise ratio of infrasound diminishes with increasing distance from the source. Seabirds nest predominantly on islands or at the coast, and hence the stable infrasound from surf offers a predictable cue to locate coastlines. Infrasonic landmarks could be used predominantly at medium scales and in situations where different local sources would provide unique signatures from their power spectra (Figure 4B). Similarly, as total acoustic energy declines rapidly with distance to the sound source (Figure 1B), it would be easier for a bird to detect an amplitude gradient when nearby. Although we propose three principal mechanisms by which birds could detect and use infrasound for navigation, they are not mutually exclusive. Birds would likely navigate using multiple properties of sound, depending on several factors including the nature of the source and the navigation task. Further research should focus on whether different infrasonic sources are distinguishable over incoherent noise (e.g., from winds) and have stable spectral features, and the amount of additional information that they would provide over other cues.
Where to Next?
A scientific demonstration of the use of infrasound for navigation requires evidence that birds can detect and extract useful information from an infrasound cue and use it in a navigational context. Our understanding of the ability of birds to detect infrasound and signal directivity over long distances is limited and requires further testing. However, we have established several hypothetical mechanisms for how infrasound could be used in navigation and a framework in which these questions can be addressed.
There are considerable technical challenges associated with testing the role of infrasound in navigation. Studies that have examined whether a particular sensory modality is involved in navigation have generally impaired its function and compared navigational performance to that of control animals (e.g., Gagliardo et al., 2013; Padget et al., 2017). A surgical removal of cochlea in pigeons was associated with changes in flight direction (Hagstrum and Manley, 2015). However, infrasound detection could involve both the vestibular and auditory end organs (Zeyl et al., 2020), and impairment of either system raises practical and ethical concerns. A useful next step in determining infrasonic hearing directionality and sensitivity would be to assess electrophysiological sensitivities to infrasonic vibration in three dimensions (e.g., using the auditory brainstem response; Christensen et al., 2012), and quantify eardrum and whole-head vibrations elicited by airborne infrasound. Furthermore, a better understanding of the information held by birds would help resolve whether animals require only directional information, or whether they are also able to encode distance, which would likely rely on different mechanisms. Studies of navigation are largely correlative, measuring a behavioural response to natural environmental variation. Experimental modifications of the marine environment, especially acoustic, can be practically impossible and so the response to extreme events, such as volcanic eruptions and hurricanes, offers the best opportunity to look at changes in navigation in response to changes in infrasound.
An alternative would be to either compare seabird navigation performance in scenarios with contrasting infrasound source and/or propagation conditions or to construct and test conceptual models for navigation (e.g., Postlethwaite and Walker, 2011; Putman et al., 2012; Putman, 2015; Berdahl et al., 2018; Taylor et al., 2021). The former may involve correlating movement decisions with infrasound along foraging trips and comparing outbound and return legs (Guilford et al., 2011) as return legs may well be made in the presence of putative stable sources of infrasound (e.g., surf from coastlines). The latter may involve creating algorithmic rules based on specific hypotheses regarding (1) which infrasound cues would be used, (2) how they are used and (3) how they are translated into movement patterns, using approaches such as agent-based models (Painter and Plochocka, 2019). This may be challenging, and would require extensive knowledge of the species and its environment to arrive to realistic rules, which will critically rely on input from biologging devices that measure movement (e.g., GPS, accelerometers and magnetometers; Williams et al., 2020) and both in-situ infrasound and infrasound estimates across a broader area. Relatively simple models would still allow researchers to gauge a hypothesised navigation strategy’s feasibility, or help to place bounds on what a given sensor would have to be able to do.
The characterisation and modelling of infrasoundscapes at high spatiotemporal resolution are also challenging due to the dynamic nature of the infrasonic wavefield in the atmosphere. Observed infrasoundscapes, often measured as part of the IMS as discussed in Section “Atmospheric Infrasound in the Marine Environment,” infrasound detection statistics vary significantly between IMS infrasound arrays around the globe. The modelling of infrasonic soundscapes (den Ouden et al., 2021b) will play a central role in the geographical mapping of infrasonic energy from specific marine acoustic sources, such as microbaroms (Waxler and Gilbert, 2006; Smets, 2018; De Carlo et al., 2020) and surf (Park et al., 2008). Moreover, the deployment of mobile loggers (Harcourt et al., 2019) will be useful to provide information on infrasound levels near ecological sites of interest and complement the existing IMS infrasound network. Finally, the development of biologging devices to capture avian movement along with infrasound measures will be crucial in advancing this field of research. Indeed, we have developed a miniature multidisciplinary sensor platform to collect geophysical data to investigate the use of infrasound and weather patterns in navigation decisions (den Ouden et al., 2021a). These techniques will improve our understanding of how seabirds use information from other geophysical cues at sea (e.g., wave direction; Lohmann and Lohmann, 1996; Serres et al., 2019), for which little is known.
Natural events have been suggested to provide quasi-experimental data for assessing changes in movement patterns. For example, infrasonic shock waves from a supersonic plane have been proposed to interrupt homing of pigeons (Hagstrum, 2000). Similarly, evasive movements of passerines away from an approaching cyclone might be the result of birds detecting and responding to infrasound rather than meteorological cues (Streby et al., 2015; but see Lisovski et al., 2018). However, the inability to control for confounding factors makes interpreting these results, and isolating the effect of infrasound, challenging. Studies which are able to monitor fine-scale animal behaviour before and after transient geological or meteorological events such as storms or earthquakes along with infrasound and other environmental (e.g., meteorological) variables should be better placed to isolate any potential behavioural responses to infrasound.
Although our understanding of avian responses to infrasound remains limited, we have recommended some key avenues for future research that would allow us to investigate its potential use for seabird navigation, and more broadly across avian species. Rather than design invasive experiments that impair hearing capabilities, we suggest the development of movement models that simulate the use of infrasound for navigation may be used alongside field studies that either correlate bird movements with in-situ infrasound measurements or track movement in response to transient infrasound sources. These strands of research are likely to add crucial pieces to the longstanding puzzle of whether birds really use infrasound for navigation.
Data Availability Statement
The original contributions presented in the study are included in the article/Supplementary Material, further inquiries can be directed to the corresponding author.
Author Contributions
SP, JA, MB, SC-T, TC, OO, RJ, and JZ contributed equally to the perspective, leading the development of ideas, creation of the framework, and wrote the first draft. All other authors contributed to the refinement of hypotheses, predictions and commented on drafts of the manuscript, and approved the submitted version.
Funding
This project was funded by a Human Frontiers Science Programme Young Investigator grant RGY0072/2017 to SP, JA, MB, and SC-T.
Conflict of Interest
RJ was employed by the company Global Fishing Watch Inc.
The remaining authors declare that the research was conducted in the absence of any commercial or financial relationships that could be construed as a potential conflict of interest.
Publisher’s Note
All claims expressed in this article are solely those of the authors and do not necessarily represent those of their affiliated organizations, or those of the publisher, the editors and the reviewers. Any product that may be evaluated in this article, or claim that may be made by its manufacturer, is not guaranteed or endorsed by the publisher.
Acknowledgments
We would like to thank Mario Zampolli, who helped develop the initial idea, and all attendees at the infrasound – seabird navigation workshop held in Liverpool in 2018. We also thank Tim Guilford and Henri Weimerskirch for helpful discussions early in the project.
Supplementary Material
The Supplementary Material for this article can be found online at: https://www.frontiersin.org/articles/10.3389/fevo.2021.740027/full#supplementary-material
References
Åkesson, S., and Alerstam, T. (1998). Oceanic navigation: are there any feasible geomagnetic bi-coordinate combinations for albatrosses? J. Avian Biol. 29, 618–625. doi: 10.2307/3677182
Alerstam, T. (2006). Conflicting evidence about long-distance animal navigation. Science 313, 791–794. doi: 10.1126/science.1129048
Assink, J. D., Pichon, A. L., Blanc, E., Kallel, M., and Khemiri, L. (2014). Evaluation of wind and temperature profiles from ECMWF analysis on two hemispheres using volcanic infrasound. J. Geophys. Res. Atmos. 119, 8659–8683. doi: 10.1002/2014JD021632
Bang, B. G. (1966). The olfactory apparatus of tubenosed birds (Procellariiformes). Cells Tissues Organs 65, 391–415. doi: 10.1159/000142884
Bee, M. A. (2007). Selective phonotaxis by male wood frogs (Rana sylvatica) to the sound of a chorus. Behav. Ecol. Sociobiol. 61, 955–966. doi: 10.1007/s00265-006-0324-8
Benhamou, S. (2003). Bicoordinate navigation based on non-orthogonal gradient fields. J. Theor. Biol. 225, 235–239. doi: 10.1016/s0022-5193(03)00242-x
Benhamou, S. (2010). “Orientation and navigation,” in Encyclopedia of Behavioral Neurosciences, eds G. F. Koob, M. LeMoal, and R. F. Thomson (Oxford: Academic Press), 497–503.
Benhamou, S., Bonadonna, F., and Jouventin, P. (2003). Successful homing of magnet-carrying white-chinned petrels released in the open sea. Anim. Behav. 65, 729–734.
Benhamou, S., Sudre, J., Bourjea, J., Ciccione, S., Santis, A. D., and Luschi, P. (2011). The role of geomagnetic cues in green turtle open sea navigation. PLoS One 6:e26672. doi: 10.1371/journal.pone.0026672
Berdahl, A. M., Kao, A. B., Flack, A., Westley, P. A. H., Codling, E. A., Couzin, I. D., et al. (2018). Collective animal navigation and migratory culture: from theoretical models to empirical evidence. Philos. Trans. R. Soc. B Biol. Sci. 373:20170009. doi: 10.1098/rstb.2017.0009
Biro, D., Meade, J., and Guilford, T. (2004). Familiar route loyalty implies visual pilotage in the homing pigeon. Proc. Natl. Acad. Sci. U.S.A. 101, 17440–17443. doi: 10.1073/pnas.0406984101
Bonadonna, F., Bajzak, C., Benhamou, S., Igloi, K., Jouventin, P., Lipp, H. P., et al. (2005). Orientation in the wandering albatross: interfering with magnetic perception does not affect orientation performance. Proc. R. Soc. Lond. B Biol. Sci. 272, 489–495. doi: 10.1098/rspb.2004.2984
Bonadonna, F., Chamaillé-Jammes, S., Pinaud, D., and Weimerskirch, H. (2003). Magnetic cues: are they important in black-browed albatross Diomedea melanophris orientation? IBIS 145, 152–155. doi: 10.1046/j.1474-919X.2003.00117.x
Brown, P. G., Assink, J. D., Astiz, L., Blaauw, R., Boslough, M. B., Borovièka, J., et al. (2013). A 500-kiloton airburst over Chelyabinsk and an enhanced hazard from small impactors. Nature 503, 238–241. doi: 10.1038/nature12741
Campus, P., and Christie, D. R. (2010). “Worldwide observation of infrasonic waves,” in Infrasound Monitoring for Atmospheric Studies, eds A. Le Pichon, E. Blanc, and A. Hauchecorne (Dordrecht: Springer), 195–234. doi: 10.1007/978-1-4020-9508-5_6
Catard, A., and Weimerskirch, H. (1999). “Satellite tracking of white-chinned petrels and comparison with other procellariiformes,” in Proceedings of the 22nd International Ornithological Congress, eds N. Adams and R. H. Slotow (Johannesburg: BirdLife South Africa), 3008–3023.
Ceranna, L., Matoza, R., Hupe, P., Le Pichon, A., and Landès, M. (2019). “Systematic array processing of a decade of global IMS infrasound data,” in Infrasound Monitoring for Atmospheric Studies: Challenges in Middle Atmosphere Dynamics and Societal Benefits, eds A. Le Pichon, E. Blanc, and A. Hauchecorne (Cham: Springer International Publishing), 471–482. doi: 10.1007/978-3-319-75140-5_13
Chan, E., Baumann, O., Bellgrove, M. A., and Mattingley, J. B. (2012). From objects to landmarks: the function of visual location information in spatial navigation. Front. Psychol. 3:304. doi: 10.3389/fpsyg.2012.00304
Christensen, C. B., Christensen-Dalsgaard, J., Brandt, C., and Madsen, P. T. (2012). Hearing with an atympanic ear: good vibration and poor sound-pressure detection in the royal python, Python regius. J. Exp. Biol. 215, 331–342. doi: 10.1242/jeb.062539
Christensen-Dalsgaard, J. (2011). Vertebrate pressure-gradient receivers. Hear. Res. 273, 37–45. doi: 10.1016/j.heares.2010.08.007
Collet, J., Patrick, S. C., and Weimerskirch, H. (2015). Albatrosses redirect flight towards vessels at the limit of their visual range. Mar. Ecol. Prog. Ser. 526, 199–205. doi: 10.3354/meps11233
Corfield, J. R., Price, K., Iwaniuk, A. N., Gutierrez-Ibañez, C., Birkhead, T., and Wylie, D. R. (2015). Diversity in olfactory bulb size in birds reflects allometry, ecology, and phylogeny. Front. Neuroanat. 9:102. doi: 10.3389/fnana.2015.00102
Croxall, J. P., Silk, J. R. D., Phillips, R. A., Afanasyev, V., and Briggs, D. R. (2005). Global circumnavigations: tracking year-round ranges of nonbreeding albatrosses. Science 307, 249–250. doi: 10.1126/science.1106042
De Carlo, M., Ardhuin, F., and Le Pichon, A. (2020). Atmospheric infrasound generation by ocean waves in finite depth: unified theory and application to radiation patterns. Geophys. J. Int. 221, 569–585. doi: 10.1093/gji/ggaa015
den Ouden, O. F. C., Assink, J. D., Smets, P. S. M., Shani-Kadmiel, S., Averbuch, G., and Evers, L. G. (2020). CLEAN beamforming for the enhanced detection of multiple infrasonic sources. Geophys. J. Int. 221, 305–317. doi: 10.1093/gji/ggaa010
den Ouden, O. F. C., Smets, P. S. M., Assink, J. D., and Evers, L. G. (2021b). A Bird’s-eye view on ambient infrasonic soundscapes. Geophys. Res. Lett. 48:e2021GL094555. doi: 10.1029/2021GL094555
den Ouden, O. F. C., Assink, J. D., Oudshoorn, C. D., Filippi, D., and Evers, L. G. (2021a). The INFRA-EAR: a low-cost mobile multidisciplinary measurement platform for monitoring geophysical parameters. Atmospheric Meas. Tech. 14, 3301–3317. doi: 10.5194/amt-14-3301-2021
Dennis, T. E., Rayner, M. J., and Walker, M. M. (2007). Evidence that pigeons orient to geomagnetic intensity during homing. Proc. R. Soc. B Biol. Sci. 274, 1153–1158. doi: 10.1098/rspb.2007.3768
Dooling, R. J., Lohr, B., and Dent, M. L. (2000). “Hearing in birds and reptiles,” in Comparative Hearing: Birds and Reptiles Springer Handbook of Auditory Research, eds R. J. Dooling, R. R. Fay, and A. N. Popper (New York, NY: Springer), 308–359. doi: 10.1007/978-1-4612-1182-2_7
Egevang, C., Stenhouse, I. J., Phillips, R. A., Petersen, A., Fox, J. W., and Silk, J. R. D. (2010). Tracking of Arctic terns Sterna paradisaea reveals longest animal migration. Proc. Natl. Acad. Sci. 107, 2078–2081. doi: 10.1073/pnas.0909493107
Evers, L. G., Brown, D., Heaney, K. D., Assink, J. D., Smets, P. S. M., and Snellen, M. (2014). Evanescent wave coupling in a geophysical system: airborne acoustic signals from the Mw 8.1 Macquarie Ridge earthquake. Geophys. Res. Lett. 41, 1644–1650. doi: 10.1002/2013GL058801
Farina, A. (2014). Soundscape Ecology: Principles, Patterns, Methods and Applications. Cham: Springer, doi: 10.1007/978-94-007-7374-5
Gagliardo, A., Bried, J., Lambardi, P., Luschi, P., Wikelski, M., and Bonadonna, F. (2013). Oceanic navigation in Cory’s shearwaters: evidence for a crucial role of olfactory cues for homing after displacement. J. Exp. Biol. 216, 2798–2805. doi: 10.1242/jeb.085738
Gleich, O., and Langemann, U. (2011). Auditory capabilities of birds in relation to the structural diversity of the basilar papilla. Hear. Res. 273, 80–88. doi: 10.1016/j.heares.2010.01.009
Guilford, T., Åkesson, S., Gagliardo, A., Holland, R. A., Mouritsen, H., Muheim, R., et al. (2011). Migratory navigation in birds: new opportunities in an era of fast-developing tracking technology. J. Exp. Biol. 214, 3705–3712. doi: 10.1242/jeb.051292
Guilford, T., and Biro, D. (2014). Route following and the pigeon’s familiar area map. J. Exp. Biol. 217, 169–179. doi: 10.1242/jeb.092908
Hagstrum, J. T. (2000). Infrasound and the avian navigational map. J. Exp. Biol. 203, 1103–1111. doi: 10.1242/jeb.203.7.1103
Hagstrum, J. T. (2013). Atmospheric propagation modeling indicates homing pigeons use loft-specific infrasonic ‘map’ cues. J. Exp. Biol. 216, 687–699. doi: 10.1242/jeb.072934
Hagstrum, J. T. (2019). A reinterpretation of “Homing pigeons’ flight over and under low stratus” based on atmospheric propagation modeling of infrasonic navigational cues. J. Comp. Physiol. A 205, 67–78. doi: 10.1007/s00359-018-1304-y
Hagstrum, J. T., and Manley, G. A. (2015). Releases of surgically deafened homing pigeons indicate that aural cues play a significant role in their navigational system. J. Comp. Physiol. A Neuroethol. Sens. Neural Behav. Physiol. 201, 983–1001. doi: 10.1007/s00359-015-1026-3
Harcourt, R., Sequeira, A. M. M., Zhang, X., Roquet, F., Komatsu, K., Heupel, M., et al. (2019). Animal-borne telemetry: an integral component of the ocean observing toolkit. Front. Mar. Sci. 6:326. doi: 10.3389/fmars.2019.00326
Heffner, R., Cumming, J. F., Koay, G., and Heffner, H. E. (2020). Hearing in Indian peafowl (Pavo cristatus): sensitivity to infrasound. J. Comp. Physiol. A 206, 899–906. doi: 10.1007/s00359-020-01446-2
Hill, E. M., Koay, G., Heffner, R. S., and Heffner, H. E. (2014). Audiogram of the chicken (Gallus gallus domesticus) from 2 Hz to 9 kHz. J. Comp. Physiol. A 200, 863–870. doi: 10.1007/s00359-014-0929-8
Hyson, R. L., Overholt, E. M., and Lippe, W. R. (1994). Cochlear microphonic measurements of interaural time differences in the chick. Hear. Res. 81, 109–118. doi: 10.1016/0378-5955(94)90158-9
Jensen, M. E., Moss, C. F., and Surlykke, A. (2005). Echolocating bats can use acoustic landmarks for spatial orientation. J. Exp. Biol. 208, 4399–4410. doi: 10.1242/jeb.01901
Jetzschke, S., Ernst, M. O., Froehlich, J., and Boeddeker, N. (2017). Finding home: landmark ambiguity in human navigation. Front. Behav. Neurosci. 11:132. doi: 10.3389/fnbeh.2017.00132
Kettler, L., Christensen-Dalsgaard, J., Larsen, O. N., and Wagner, H. (2016). Low frequency eardrum directionality in the barn owl induced by sound transmission through the interaural canal. Biol. Cybern. 110, 333–343. doi: 10.1007/s00422-016-0689-3
Klump, G. M. (2000). “Sound localization in birds,” in Comparative Hearing: Birds and Reptiles Springer Handbook of Auditory Research, eds R. J. Dooling, R. R. Fay, and A. N. Popper (New York, NY: Springer), 249–307. doi: 10.1007/978-1-4612-1182-2_6
Kreithen, M. L., and Quine, D. B. (1979). Infrasound detection by the homing pigeon: a behavioral audiogram. J. Comp. Physiol. 129, 1–4. doi: 10.1007/BF00679906
Larsen, O. N., Dooling, R. J., and Michelsen, A. (2006). The role of pressure difference reception in the directional hearing of budgerigars (Melopsittacus undulatus). J. Comp. Physiol. A 192, 1063–1072. doi: 10.1007/s00359-006-0138-1
Lisovski, S., Schmaljohann, H., Bridge, E. S., Bauer, S., Farnsworth, A., Gauthreaux, S. A., et al. (2018). Inherent limits of light-level geolocation may lead to over-interpretation. Curr. Biol. 28, R99–R100. doi: 10.1016/j.cub.2017.11.072
Lohmann, K. J., and Lohmann, C. M. F. (1996). Orientation and open-sea navigation in sea turtles. J. Exp. Biol. 199, 73–81. doi: 10.1242/jeb.199.1.73
Lohmann, K. J., Lohmann, C. M. F., and Endres, C. S. (2008). The sensory ecology of ocean navigation. J. Exp. Biol. 211, 1719–1728. doi: 10.1242/jeb.015792
Marty, J. (2019). “The IMS infrasound network: current status and technological developments,” in Infrasound Monitoring for Atmospheric Studies: Challenges in Middle Atmosphere Dynamics and Societal Benefits, eds A. Le Pichon, E. Blanc, and A. Hauchecorne (Cham: Springer International Publishing), 3–62. doi: 10.1007/978-3-319-75140-5_1
Matoza, R. S., Landès, M., Le Pichon, A., Ceranna, L., and Brown, D. (2013). Coherent ambient infrasound recorded by the International Monitoring System. Geophys. Res. Lett. 40, 429–433. doi: 10.1029/2012GL054329
Michelsen, A., and Larsen, O. N. (2007). Pressure difference receiving ears. Bioinspir. Biomim. 3:011001. doi: 10.1088/1748-3182/3/1/011001
Moffat, A. J. M., and Capranica, R. R. (1976). Auditory sensitivity of the saccule in the American toad (Bufo americanus). J. Comp. Physiol. 105, 1–8. doi: 10.1007/BF01380048
Moron, J. R., and Andriolo, A. (2015). Preliminary evidence for signature and copied whistles among spinner dolphins in the Southwest Atlantic Ocean: beacon purpose? J. Acoust. Soc. Am. 138, 1904–1904. doi: 10.1121/1.4933987
Mouritsen, H. (2018). Long-distance navigation and magnetoreception in migratory animals. Nature 558, 50–59. doi: 10.1038/s41586-018-0176-1
Mueller, T., and Fagan, W. F. (2008). Search and navigation in dynamic environments: from individual behaviors to population distributions. Oikos 117, 654–664. doi: 10.1111/j.0030-1299.2008.16291.x
Nardini, M., Jones, P., Bedford, R., and Braddick, O. (2008). Development of cue integration in human navigation. Curr. Biol. 18, 689–693. doi: 10.1016/j.cub.2008.04.021
Nevitt, G. A. (2008). Sensory ecology on the high seas: the odor world of the procellariiform seabirds. J. Exp. Biol. 211, 1706–1713. doi: 10.1242/jeb.015412
Nevitt, G. A., Losekoot, M., and Weimerskirch, H. (2008). Evidence for olfactory search in wandering albatross, Diomedea exulans. Proc. Natl. Acad. Sci. U.S.A. 105, 4576–4581. doi: 10.1073/pnas.0709047105
Padget, O., Bond, S. L., Kavelaars, M. M., van Loon, E., Bolton, M., Fayet, A. L., et al. (2018). In situ clock shift reveals that the sun compass contributes to orientation in a pelagic seabird. Curr. Biol. 28, 275–279.e2. doi: 10.1016/j.cub.2017.11.062
Padget, O., Dell’Ariccia, G., Gagliardo, A., González-Solís, J., and Guilford, T. (2017). Anosmia impairs homing orientation but not foraging behaviour in free-ranging shearwaters. Sci. Rep. 7:9668. doi: 10.1038/s41598-017-09738-5
Padget, O., Stanley, G., Willis, J. K., Fayet, A. L., Bond, S., Maurice, L., et al. (2019). Shearwaters know the direction and distance home but fail to encode intervening obstacles after free-ranging foraging trips. Proc. Natl. Acad. Sci. U.S.A. 116, 21629–21633. doi: 10.1073/pnas.1903829116
Painter, K. J., and Plochocka, A. Z. (2019). Efficiency of island homing by sea turtles under multimodal navigating strategies. Ecol. Model. 391, 40–52. doi: 10.1016/j.ecolmodel.2018.10.025
Park, J., Garcés, M., Fee, D., and Pawlak, G. (2008). Collective bubble oscillations as a component of surf infrasound. J. Acoust. Soc. Am. 123, 2506–2512. doi: 10.1121/1.2885743
Pierce, A. D. (2019). Acoustics: An Introduction to Its Physical Principles and Applications, 3rd Edn. Cham: Springer International Publishing, doi: 10.1007/978-3-030-11214-1
Pollonara, E., Luschi, P., Guilford, T., Wikelski, M., Bonadonna, F., and Gagliardo, A. (2015). Olfaction and topography, but not magnetic cues, control navigation in a pelagic seabird: displacements with shearwaters in the Mediterranean Sea. Sci. Rep. 5:16486. doi: 10.1038/srep16486
Postlethwaite, C. M., and Walker, M. M. (2011). A geometric model for initial orientation errors in pigeon navigation. J. Theor. Biol. 269, 273–279. doi: 10.1016/j.jtbi.2010.10.036
Putman, N. F. (2015). Inherited magnetic maps in salmon and the role of geomagnetic change. Integr. Comp. Biol. 55, 396–405. doi: 10.1093/icb/icv020
Putman, N. F., Lohmann, K. J., Putman, E. M., Quinn, T. P., Klimley, A. P., and Noakes, D. L. G. (2013). Evidence for geomagnetic imprinting as a homing mechanism in Pacific salmon. Curr. Biol. 23, 312–316. doi: 10.1016/j.cub.2012.12.041
Putman, N. F., Verley, P., Shay, T. J., and Lohmann, K. J. (2012). Simulating transoceanic migrations of young loggerhead sea turtles: merging magnetic navigation behavior with an ocean circulation model. J. Exp. Biol. 215, 1863–1870. doi: 10.1242/jeb.067587
Quine, D. B., and Kreithen, M. L. (1981). Frequency shift discrimination: Can homing pigeons locate infrasounds by Doppler shifts? J. Comp. Physiol. 141, 153–155. doi: 10.1007/BF01342661
Raspet, R., Abbott, J.-P., Webster, J., Yu, J., Talmadge, C., Alberts, K. II, et al. (2019). “New systems for wind noise reduction for infrasonic measurements,” in Infrasound Monitoring for Atmospheric Studies: Challenges in Middle Atmosphere Dynamics and Societal Benefits, eds A. Le Pichon, E. Blanc, and A. Hauchecorne (Cham: Springer International Publishing), 91–124. doi: 10.1007/978-3-319-75140-5_3
Reynolds, A. M., Cecere, J. G., Paiva, V. H., Ramos, J. A., and Focardi, S. (2015). Pelagic seabird flight patterns are consistent with a reliance on olfactory maps for oceanic navigation. Proc. Royal Soc. B Biol. Sci. 282:20150468. doi: 10.1098/rspb.2015.0468
Rosengren, S. M., Welgampola, M. S., and Colebatch, J. G. (2010). Vestibular evoked myogenic potentials: past, present and future. Clin. Neurophysiol. 121, 636–651. doi: 10.1016/j.clinph.2009.10.016
Schermuly, L., and Klinke, R. (1990). Infrasound sensitive neurones in the pigeon cochlear ganglion. J. Comp. Physiol. 166, 355–363. doi: 10.1007/BF00204808
Serres, J. R., Evans, T. J., Åkesson, S., Duriez, O., Shamoun-Baranes, J., Ruffier, F., et al. (2019). Optic flow cues help explain altitude control over sea in freely flying gulls. J. R. Soc. Interface 16:20190486. doi: 10.1098/rsif.2019.0486
Shettleworth, S. J., and Sutton, J. E. (2005). Multiple systems for spatial learning: dead reckoning and beacon homing in rats. J. Exp. Psychol. Anim. Behav. Process. 31, 125–141. doi: 10.1037/0097-7403.31.2.125
Sisneros, J. A., and Rogers, P. H. (2016). “Directional hearing and sound source localization in fishes,” in Fish Hearing and Bioacoustics: An Anthology in Honor of Arthur N. Popper and Richard R. Fay Advances in Experimental Medicine and Biology, ed. J. A. Sisneros (Cham: Springer International Publishing), 121–155. doi: 10.1007/978-3-319-21059-9_7
Si, X., Angelaki, D. E., and Dickman, J. D. (1997). Response properties of pigeon otolith afferents to linear acceleration. Exp. Brain Res. 117, 242–250. doi: 10.1007/s002210050219
Smets, P. S. M. (2018). Infrasound and the Dynamical Stratosphere: A new application for operational Weather and Climate Prediction. Ph.D. thesis. Delft: Delft University of Technology, doi: 10.4233/uuid:517f8597-9c24-4d01-83ed-0f430353e905
Spear, L. B., and Ainley, D. G. (1997). Flight speed of seabirds in relation to wind speed and direction. IBIS 139, 234–251. doi: 10.1111/j.1474-919X.1997.tb04621.x
Stansbury, A. L., Götz, T., Deecke, V. B., and Janik, V. M. (2015). Grey seals use anthropogenic signals from acoustic tags to locate fish: evidence from a simulated foraging task. Proc. R. Soc. B Biol. Sci. 282:20141595. doi: 10.1098/rspb.2014.1595
Streby, H. M., Kramer, G. R., Peterson, S. M., Lehman, J. A., Buehler, D. A., and Andersen, D. E. (2015). Tornadic storm avoidance behavior in breeding songbirds. Curr. Biol. 25, 98–102. doi: 10.1016/j.cub.2014.10.079
Sutherland, L. C., and Bass, H. E. (2004). Atmospheric absorption in the atmosphere up to 160 km. J. Acoust. Soc. Am. 115, 1012–1032. doi: 10.1121/1.1631937
Taylor, B. K., Bernish, M. K., Pizzuti, S. A., and Kehl, C. E. (2021). A bioinspired navigation strategy that uses magnetic signatures to navigate without GPS in a linearized northern Atlantic ocean: a simulation study. Bioinspir. Biomim. 16:046006. doi: 10.1088/1748-3190/abe7cd
Theunissen, F. E., and Shaevitz, S. S. (2006). Auditory processing of vocal sounds in birds. Curr. Opin. Neurobiol. 16, 400–407. doi: 10.1016/j.conb.2006.07.003
Theurich, M., Langner, G., and Scheich, H. (1984). Infrasound responses in the midbrain of the guinea fowl. Neurosci. Lett. 49, 81–86. doi: 10.1016/0304-3940(84)90140-X
Thiebault, A., Pistorius, P., Mullers, R., and Tremblay, Y. (2016). Seabird acoustic communication at sea: a new perspective using bio-logging devices. Sci Rep 6, 30972. doi: 10.1038/srep30972
Wallraff, H. G. (2000). Simulated navigation based on observed gradients of atmospheric trace gases (Models on pigeon homing part 3). J. Theor. Biol. 205, 133–145.
Wallraff, H. G. (2005). Avian Navigation: Pigeon Homing as a Paradigm. Berlin: Springer-Verlag, doi: 10.1007/b137573
Wallraff, H. G. (2014). Pigeon homing from unfamiliar areas. Commun. Integr. Biol. 7:e28565. doi: 10.4161/cib.28565
Warchol, M. E., and Dallos, P. (1989). Neural response to very low-frequency sound in the avian cochlear nucleus. J. Comp. Physiol. A 166, 83–95. doi: 10.1007/BF00190213
Waxler, R., and Assink, J. (2019). “Propagation modeling through realistic atmosphere and benchmarking,” in Infrasound Monitoring for Atmospheric Studies: Challenges in Middle Atmosphere Dynamics and Societal Benefits, eds A. Le Pichon, E. Blanc, and A. Hauchecorne (Cham: Springer International Publishing), 509–549. doi: 10.1007/978-3-319-75140-5_15
Waxler, R., and Gilbert, K. E. (2006). The radiation of atmospheric microbaroms by ocean waves. J. Acoust. Soc. Am. 119, 2651–2664. doi: 10.1121/1.2191607
Williams, H. J., Taylor, L. A., Benhamou, S., Bijleveld, A. I, Clay, T. A., Grissac, S., et al. (2020). Optimizing the use of biologgers for movement ecology research. J. Anim. Ecol. 89, 186–206. doi: 10.1111/1365-2656.13094
Wiltschko, R., and Wiltschko, W. (2003). Avian navigation: from historical to modern concepts. Anim. Behav. 65, 257–272. doi: 10.1006/anbe.2003.2054
Wynn, J., Padget, O., Mouritsen, H., Perrins, C., and Guilford, T. (2020). Natal imprinting to the Earth’s magnetic field in a pelagic seabird. Curr. Biol. 30, 2869–2873.e2. doi: 10.1016/j.cub.2020.05.039
Keywords: low frequency sound, animal movement, avian hearing, seabirds, migration
Citation: Patrick SC, Assink JD, Basille M, Clusella-Trullas S, Clay TA, den Ouden OFC, Joo R, Zeyl JN, Benhamou S, Christensen-Dalsgaard J, Evers LG, Fayet AL, Köppl C, Malkemper EP, Martín López LM, Padget O, Phillips RA, Prior MK, Smets PSM and van Loon EE (2021) Infrasound as a Cue for Seabird Navigation. Front. Ecol. Evol. 9:740027. doi: 10.3389/fevo.2021.740027
Received: 12 July 2021; Accepted: 21 October 2021;
Published: 26 November 2021.
Edited by:
Guy Gilles Beauchamp, Independent Researcher, Montréal, QC, CanadaReviewed by:
Paolo Luschi, University of Pisa, ItalyBrian Taylor, University of North Carolina at Chapel Hill, United States
Andy Reynolds, Rothamsted Research, United Kingdom
Copyright © 2021 Patrick, Assink, Basille, Clusella-Trullas, Clay, den Ouden, Joo, Zeyl, Benhamou, Christensen-Dalsgaard, Evers, Fayet, Köppl, Malkemper, Martín López, Padget, Phillips, Prior, Smets and van Loon. This is an open-access article distributed under the terms of the Creative Commons Attribution License (CC BY). The use, distribution or reproduction in other forums is permitted, provided the original author(s) and the copyright owner(s) are credited and that the original publication in this journal is cited, in accordance with accepted academic practice. No use, distribution or reproduction is permitted which does not comply with these terms.
*Correspondence: Samantha C. Patrick, c2FtYW50aGEucGF0cmlja0BsaXZlcnBvb2wuYWMudWs=
†These authors have contributed equally to this work