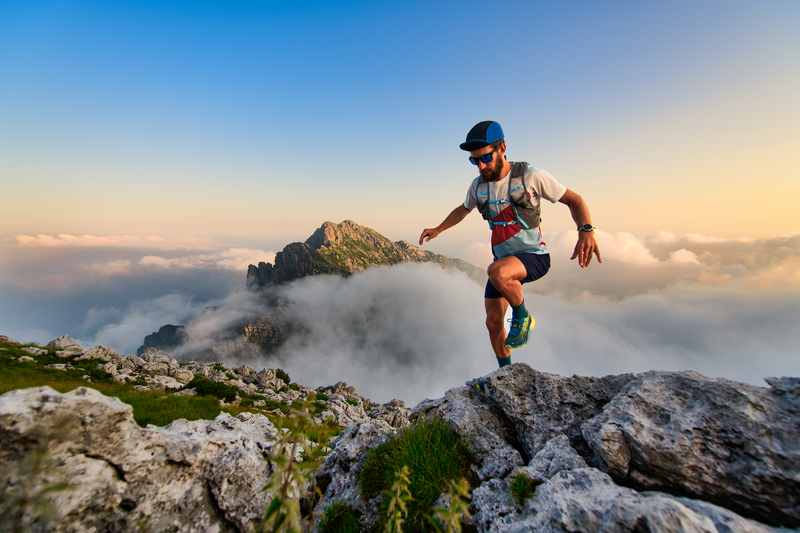
94% of researchers rate our articles as excellent or good
Learn more about the work of our research integrity team to safeguard the quality of each article we publish.
Find out more
PERSPECTIVE article
Front. Ecol. Evol. , 18 September 2020
Sec. Behavioral and Evolutionary Ecology
Volume 8 - 2020 | https://doi.org/10.3389/fevo.2020.564343
This article is part of the Research Topic Ecology and Evolution of Non-Consumptive Effects in Host-Parasite Interactions View all 11 articles
Fear of natural enemies in non-human animals is a concept dating back to the time of Darwin. Now recognized as a non-consumptive effect, the ecological and evolutionary impact of fear has been studied in a number of predator-prey systems within the last few decades. However, comparatively little consideration has been given to the non-consumptive effects that parasites have on their hosts, which have evolved behaviors to avoid parasites, impacting habitat selection, mate choice, and foraging activity. These avoidance behaviors create a “landscape of disgust,” wherein hosts navigate to avoid parasites, akin to the “landscape of fear” in prey. Evolutionarily speaking, however, predators and parasites are but two examples of natural enemies. Pathogens, parasites, predators, and parasitoids, among others, each exert their own fitness cost on the victims they attack. Since animals likely evolve in the presence of multiple natural enemies, they must adopt a range of avoidance behaviors to navigate through the resulting “landscape of peril.” Therefore, in line with recent efforts to combine the landscapes of fear and disgust, we offer a theoretical framework to better understand the impacts of natural enemies on the evolution of trait-mediated avoidance behaviors in animals. More precisely, we look at how victims may evolve to allocate energy optimally among distinct avoidance behaviors under the selective pressures imposed by different types of natural enemy. This framework is then put into the more realistic context of a food web, which highlights the impact of trophic interactions and trophic level on the evolution of avoidance behaviors.
When imagining a pride of lions chasing down an antelope in sub-Saharan Africa, few would consider that these apex predators have any enemies of their own. As it turns out, even the “king of the jungle” has its own set of natural enemies [i.e., any organism that has evolved to exploit the resources of a victim, incurring a negative or lethal impact on the latter (Raffel et al., 2008)] which, apart from humans and sometimes hyenas, are mostly parasites (Bjork et al., 2000; Berentsen et al., 2012). In fact, it is likely that every metazoan species has evolved with at least one parasite (Poulin, 2014; Costello, 2016), therefore it is reasonable to assume that no animal species is devoid of natural enemies (now referred to simply as enemies). Following this assumption, we would expect lions to have evolved traits that reduce the probability of acquiring parasites, such as avoiding the carcasses of conspecifics and other carnivores even though they represent an easy energy source (Moléon et al., 2017). Avoidance behaviors such as this are termed non-consumptive effects because the victim (i.e., the lion) is responding directly to a potential act of consumption by an enemy (i.e., a parasite), without consumption actually taking place (Curtis, 2014; Buck et al., 2018). Further, if lions evolved behaviors to mostly avoid parasites, antelopes may have evolved an even greater number of behaviors to avoid lions, other predators, and parasites.
For a primary consumer like the antelope, the non-consumptive effects of predation on their reproduction and even survival can sometimes surpass the direct effects of consumption (Sheriff and Thaler, 2014; MacLeod et al., 2018). These processes are now well established in predator-prey interactions (Gaynor et al., 2019), even though the overall impacts on prey population size are still poorly understood (Sheriff et al., 2020). Parasites can also induce non-consumptive effects in their hosts, a topic that has received more recognition in recent years (Sarabian et al., 2018b; Buck, 2019). Hosts can avoid getting infected through changes in behavior (Hart and Hart, 2018), such as limiting interactions with conspecifics that appear infected (Stephenson et al., 2018), directly avoiding parasitic infective stages through the detection of cues (Strauss et al., 2019), or changing feeding habits (Sarabian et al., 2018a) or habitat use (Amoroso et al., 2019). These avoidance behaviors differ from other defensive strategies in that they are a direct and dynamic response to environmental cues, as opposed to constitutional defense strategies such as morphology (e.g., the long hairs on a caterpillar). As a result, hosts have evolved to invest resources in behavioral or sensory traits that decrease the probability of infection. Animals can thus navigate more safely through their environment, which has aptly been named the “landscape of disgust” (Weinstein et al., 2018a) as opposed to the “landscape of fear” in predator-prey systems (Laundré et al., 2001). The degree to which a species experiences fear or disgust is difficult to assess (Mendl et al., 2010), but regardless of the avoidance behavior, it is likely that many species have evolved with more than one enemy to contend with.
As enemies go, predators and parasites are but broad generalizations of a multitude of trophic strategies that exist, each one having its own fitness cost on the victims that are successfully attacked (Lafferty and Kuris, 2002). For example, parasitic castrators do not kill their hosts, but they effectively suppress host reproduction, thereby reducing host fitness to zero (Lafferty and Kuris, 2009). Other enemies, such as micropredators (e.g., mosquitoes and vampire bats), may feed on multiple hosts without drastically reducing their fitness (Poulin, 2011). Animals that have evolved with multiple enemies, each with specific trophic strategies, may allocate their resources toward avoidance behaviors that balance immediate danger with potential fitness loss. Tadpoles, for example, prefer to forage in the presence of trophically transmitted parasites rather than predators, likely because infection is relatively less costly to fitness than being eaten (Koprivnikar and Penalva, 2015). Considering the above, it is sensible to combine the landscapes of fear and disgust into a broader landscape of enemies (Buck et al., 2018; Sarabian et al., 2018b), which we call here the “landscape of peril.” Only then could we understand how animals invest energy in their evolutionary toolkit of avoidance behaviors to safely navigate under the near constant threat of attack.
In light of recent efforts to unite the landscapes of fear and disgust (Buck et al., 2018; Weinstein et al., 2018a), we discuss here the broad evolutionary implications of animals that evolve in the presence of one or more enemies with different types of trophic strategy, each of which incurring a differential fitness cost upon the victim. By illustrating how victims may evolve to detect enemy cues and invest their resources into avoidance behaviors to navigate safely through the landscape of peril, we can predict how animals allocate energy for optimal survival. This depends ultimately on the selective pressures or risk imposed by enemies, which can be measured with abundance, lethality, or species richness (Bordes and Morand, 2009). These predictions are then verified with recent examples in the literature, putting into evolutionary context the landscape of peril. We then explore the idea of how, like for lions and antelopes, the trophic level in a food web may impact the avoidance behaviors in animals, along with some examples from the literature. We show that, regardless of trophic level, it is likely that all animal species have inevitably evolved with their own set of enemies. However, the composition of enemy types for a particular victim could depend on its relative position in the food web, in addition to other characteristics such as body size or sociality. Thus, we provide a theoretical backdrop for the evolution of avoidance behaviors in animals subjected to the selective pressures imposed by one or more types of enemy.
Trophic strategies in animals are not limited to predators and parasites. Lafferty and Kuris (2002) recognized a total of ten trophic strategists, consisting of seven types of parasites and three types of predators. Each strategist incurs a negative fitness cost on the victims that they effectively consume or attack (see Figure 1 in Lafferty and Kuris, 2002), which varies in relation to the evolutionary history between both antagonistic species. An enemy that successfully attacks a victim will either eliminate its fitness completely or reduce it partially. So, if victim fitness has a maximum value of 1 in a continuum ranging from 0 to 1 inclusively, an enemy, depending on its trophic strategy, can either reduce it to 0 (i.e., a “lethal” enemy) or reduce it to a number between 0 and 1 (i.e., a non-lethal enemy) (Figure 1A). Of course, there are exceptions to this (e.g., a host with an extraordinary number of non-lethal parasites may die), but on an evolutionary timescale, we argue that this dichotomy represents a strong selective pressure exerted by enemies on the evolution of avoidance behaviors in victims.
Figure 1. How animal victims may evolve to allocate energy toward avoidance behaviors against natural enemies. (A) Ten recognized trophic strategies in natural enemies toward victims. “Lethal” natural enemies do not necessarily kill their victims, but effectively eliminate victim fitness. The dichotomy between lethal and non-lethal natural enemies represents one of the evolutionary pressures to evolve avoidance behaviors. (B) Theoretical evolution of energy allocation toward avoidance behaviors based solely on the probability that a victim will be attacked by a natural enemy during its lifetime. Each circle represents one victim population that evolves with a certain probability of being attacked by a natural enemy. (C) How victim populations theoretically evolve to allocate energy toward avoidance behaviors under the risk imposed by natural enemies. Risk results from the product of the probability of attack multiplied by the fitness cost imposed by a certain natural enemy. Therefore, in the case of victims that evolve exclusively with lethal natural enemies (red dashed line), the risk imposed by the latter equals to the probability of being attacked. With victims that evolve exclusively with non-lethal natural enemies (blue dashed line), the risk can range from anywhere between 0 and 1, depending on the fitness cost imposed by the natural enemy (in this case, the fitness cost is 0.2). The colored areas represent a plastic response of resource allocation, whereas the dashed lines represent a fixed response.
Upon consumption, enemies can reduce victim fitness either partially or completely, but this is not the only selective pressure imposed on victims. We hypothesize that another important evolutionary pressure is the probability of a successful attack on a victim, which depends on a number of variables, such as the population densities of both enemies and victims (Ioannou et al., 2008) and the resulting encounter rate between them (Mols et al., 2004). However, regardless of the determining factors, a victim is most likely subjected to a certain probability of being attacked by an enemy during its lifetime, ranging from 0 to 1 inclusively. In some host-parasite systems, the lifetime probability of being attacked is almost guaranteed to equal 1 (e.g., an abundant parasite present in the only water source of a particular area), therefore what matters in these cases is the probability of sufficient repeated attacks which results in an intensity of infection that lowers fitness. Nevertheless, if the probability of attack by an enemy remains constant or varies little over many generations, we predict that a victim will evolve to allocate resources toward their avoidance behaviors proportionally to this lifetime probability of attack (Figure 1B). This relationship may not be linear, but we would still expect a positive correlation between energy allocation and the probability of attack. If the probability of attack is more variable over evolutionary time, we would expect a more plastic response of resource allocation toward avoidance behaviors.
We suggest that victims have a risk of completely losing their fitness or dying from a lethal enemy that is equal to the lifetime probability of being successfully attacked by said enemy (Figure 1C). Therefore, a victim that evolves with a probability of 0.4 of being attacked by a lethal enemy during its lifetime should invest relatively less in their avoidance behaviors than a victim that evolves with a probability of 0.8 of being attacked (Figure 1C). Realistically, we could predict that this resource allocation translates into the amount of time that victims spend scanning their environment for enemy cues or how much they limit habitat use (see introduction). Regardless of the behavior employed, the more a victim invests resources into avoiding a potential enemy, the more likely it is to lose foraging opportunities. For example, deer that evolve with predators limit their foraging activities to twilight and night hours, whereas deer that have lived on predator-free islands for several generations forage more during the day, thus increasing their energy intake considerably (Bonnot et al., 2016). This appears to be due to a relaxed selection on foraging traits linked to predator avoidance. Still, the increased foraging activity during twilight hours is observed in deer on predator-free islands, suggesting that this avoidance trait persists even after long-term absence of predators. We could also predict that the energy investment into avoidance behaviors translates into the physiological sensitivity that victims have toward enemy cues, if the enemy is at all detectable. For example, pea aphids appear to respond rapidly to the presence of generalist parasitoids by dropping from the plant they are feeding on (Fill et al., 2012). Even though this species is not a host of the parasitoid, the aphid appears to be very sensitive to the presence of potential enemies. This high sensitivity results in the aphid losing a considerable amount of time away from the leaf, resulting in a reduction of energy intake.
The allocation of resources toward avoidance behaviors may differ if a victim evolves exclusively in the presence of a non-lethal enemy. Since they do not eliminate victim fitness upon consumption, the actual risk of dying (or potential loss of fitness) from a non-lethal enemy results from the product of the probability of attack multiplied by the fitness cost (Figure 1C). If non-lethal enemies impose the same amount of risk over time, victims should evolve to allocate resources accordingly. Therefore, even if non-lethal enemies pose less risk than lethal ones, we would still expect victims to invest resources into avoidance behaviors proportionally to the risk imposed by non-lethal enemies (e.g., lions avoiding carcasses, see introduction). As for lethal enemies, this relationship is certainly positive, but it may not be linear. As stated above, if the level of risk varies more over time, victims may evolve plastic traits to allocate energy responsively toward avoidance behaviors depending on perceived risk (Figure 1C). For example, the metabolic rate in Drosophila flies increases when they are either indirectly or directly exposed to parasitic mites, but to different degrees, suggesting that the avoidance behaviors of the fly may have evolved to contend with different levels of risk imposed by the enemy (Luong et al., 2017).
If a victim evolves with lethal and non-lethal enemies that impose similar levels of risk, we predict that more energy would be invested into avoiding the lethal enemy, simply because of the potential fitness loss it imposes. Even though both types of enemy can have a synergistic negative effect on victim survival (Marino and Werner, 2013; Shang et al., 2019), when given the choice, victims (e.g., tadpoles, see introduction) may choose to forage more under the threat of a non-lethal enemy than under the threat of a lethal one. This obviously depends on the risk associated with a certain enemy, which may vary in time and space. Moreover, victims that evolve plastic trait-mediated avoidance behaviors should be better at responding to the dynamic threats imposed by multiple types of enemy. Interestingly, some avoidance behaviors may evolve as a response to multiple enemy cues. A good example of this is the general avoidance of feces by animals that are susceptible to disease (Weinstein et al., 2018a). Victims under selective pressures to avoid both lethal and non-lethal enemies may have evolved traits to avoid feces altogether, which simultaneously reduces the risk of encountering predators and acquiring parasites (Weinstein et al., 2018b). However, the research on avoidance behaviors in response to both lethal and non-lethal enemies is limited to a few studies and even less study systems (Buck et al., 2018). Therefore, more research is needed to better understand the impacts of risk and enemy type on the evolution of avoidance behaviors in victims.
In this section, we argue that the dichotomy of fitness effects between lethal and non-lethal enemies and the lifelong probability of a successful attack represent the two strongest selective pressures imposed by enemies on the evolution of resource allocation toward avoidance behaviors. Of course, the probability of attack is variable and is also likely to be context-dependent, but if it remains somewhat constant over an evolutionary timescale, we would expect victims to evolve avoidance behaviors accordingly (see above). Another possible selective pressure imposed by enemies is the loss of energy intake or foraging opportunities due to the time victims spend detecting enemy cues or avoiding patches of food. While this indirect effect may also have implications in the evolution of avoidance behaviors, we suggest that a loss in energy intake results from the dynamic changes in behavior that evolved in response to the two pressures described above. Therefore, we argue that a loss of foraging opportunities is highly variable and context-dependent and represents a weaker selective pressure. For example, non-parasitized sheep avoid patches of vegetation contaminated with feces, which decreases food intake and activity levels. Contrarily, parasitized sheep graze more in contaminated patches, which increases food intake (Hutchings et al., 2001). Here, the trade-off between nutrition and parasitism appears to depend mainly on the infection status of the sheep.
Trophically transmitted parasites impose a unique type of selective pressure on victims. Their general life cycle includes at least one intermediate host that must then be consumed by a definitive host in order for the parasite to mature and reproduce (Poulin, 2011). These parasites can increase the likelihood that the intermediate host gets eaten by the definitive host through alteration of its behavior or appearance, making it more conspicuous (see Moore, 2002 for examples). For the intermediate host, this non-lethal enemy increases the probability of attack from a lethal enemy, meaning that the level of risk increases after infection. This evolutionary pressure may select for victims that allocate more resources toward avoiding trophically transmitted parasites, which also reduces the probability of being eaten by the definitive host of the parasite. For the definitive host, a conspicuous prey is easier to identify, providing more resources, however, this increase in energy intake also increases exposure to infection. Although trophically transmitted parasites are unlikely to have evolved to harm or kill their definitive hosts, since they help disperse the parasite into the environment, the host may have evolved traits to avoid heavily parasitized prey in order to mitigate the reward of eating conspicuous prey with the cost of acquiring heavy parasite burdens. For example, oystercatchers consume mainly medium-sized cockles and avoid larger, energy-rich cockles that are likelier to have heavy parasite loads (Norris, 1999).
Animals at the base or at the top of the food web probably evolve with different ratios of lethal to non-lethal enemies. If an apex predator, such as a lion, evolves avoidance behaviors mainly against non-lethal enemies like parasites (i.e., a lower relative number of lethal enemies), it is reasonable to suggest that, as we move down the food web, primary and secondary consumers may evolve avoidance behaviors mainly against lethal enemies (i.e., a higher relative number of lethal enemies). To test this idea, we look at food web data for a shallow brackish water ecosystem from Germany and Denmark (Zander et al., 2011). By counting the number of trophic links between victim species and enemy species provided in this study, it was possible to estimate the species richness of lethal and non-lethal enemies for each victim. Victim species were then grouped qualitatively into “organismal groups” identified by the authors of the study, in order to approximate the trophic level of each victim species. From these data, we are able to observe that the total number of lethal enemies, along with the ratio of lethal to non-lethal enemies, decrease in victims as we move up the food web of aquatic and semi-aquatic animal groups (Figure 2 and Supplementary Material). This suggests that lower-level consumers, such as invertebrates and small vertebrates, probably evolve to invest more resources into avoidance behaviors against both non-lethal and lethal enemies than do tertiary consumers. Therefore, a primary consumer like an amphipod may invest heavily into avoiding lethal enemies, whereas a secondary consumer like a solitary fish may invest relatively more resources toward non-lethal ones. In fact, in this aquatic food web, the ratio of lethal to non-lethal enemies is almost completely inversed between primary consumers and tertiary ones (Figure 2). Here, the framework of the landscape of peril appears to apply more to victims situated in the middle trophic levels, which have to contend with both lethal and non-lethal enemies. Victims at the bottom of the food chain may navigate more through a landscape of fear, whereas victims at the top of the food chain may navigate more through a landscape of disgust.
Figure 2. Number of lethal natural enemy species (i.e., enemies that effectively eliminate the fitness of victims, such as predators and parasitic castrators) with the ratio of lethal to non-lethal natural enemy species (i.e., enemies that reduce fitness to between 0 and 1, such as trophically transmitted parasites and pathogens) in parentheses for different organismal animal groups (body size is not to scale) in a simplified food web of a tidal basin that approximates trophic level. From left to right, primary consumers in green include annelids, snails, and amphipods; secondary consumers in orange include schooling fishes and solitary fishes; tertiary consumers in red include birds and mammals. The numbers for each animal group are median values calculated for a number of species that were included in each group (Supplementary Material). Note that, for mammals, only one species was available from the dataset.
The complex interactions between species in a food web may change depending on how victims evolve to invest in their avoidance behaviors. For example, if a victim avoids conspecifics to reduce the likelihood of acquiring non-lethal enemies, this could simultaneously increase the likelihood of being detected and eaten by a lethal one. Rainbow trout tend to form smaller and less cohesive shoals in the presence of conspecifics infected with eye flukes (a trophically transmitted parasite), which may in turn increase the chance of individual trout getting eaten by birds (Seppälä et al., 2008). Here, there is a clear trade-off between parasitism and predation in the trout, with social grouping behaviors playing a key balancing role. Thus, the evolution of avoidance behaviors toward non-lethal enemies may drastically change the energy flow in trophic networks. It is recognized that parasites alone are important in sustaining diverse and complex food webs (Dunne et al., 2013). However, there remains much to be discovered about the hidden diversity of non-lethal enemies such as parasites and pathogens and the roles they play in maintaining the structure and health of food webs (Lafferty et al., 2008; Sukhdeo, 2012).
If a certain victim evolves with a particular set of enemies due mainly to its position in the food web, it is possible that others lessen this particular pressure by growing larger. For instance, mammal species that grow beyond a certain body mass threshold are less susceptible to predation, but may still evolve avoidance behaviors toward parasites (Sinclair et al., 2003). Asian elephants, primary consumers that are seldom predated upon (except perhaps for small juveniles), have evolved the avoidance behavior of “fly switching,” in which an individual fashions a swatting tool out of a tree branch to decrease the risk of getting bitten or infected by parasitic flies (Hart and Hart, 1994; Hart et al., 2001). Therefore, even if growing big reduces the probability of being attacked by a lethal enemy, larger victims may still invest energy in avoidance behaviors against smaller, non-lethal ones. Actually, the diversity of non-lethal enemies tends to increase for larger animal victim species. In a meta-analysis from Kamiya et al. (2014), it was shown that parasite species richness increases generally with host body size, a trend that appears to be universal across taxa and study scales. So, if larger animals harbor more diverse parasite communities than do smaller ones in general, it is reasonable to assume that they evolved to invest relatively more in their avoidance behaviors against non-lethal enemies.
All species likely evolve under the selective pressures imposed by enemies and therefore have to adapt in order to safely navigate through their environment with the use of avoidance behaviors. We argue that the lifelong probability of a successful attack and the fitness cost imposed by enemies (lethal or non-lethal) create risk, which ultimately selects for victims that allocate energy toward avoidance behaviors that reduce the risk of an enemy attack, thus optimizing survival. Both the fitness cost and the probability of attack represent strong selective pressures for the evolution of resource allocation toward avoidance behaviors. Moreover, depending on how variable the probability of attack is over evolutionary time, victims may adapt plastic trait-mediated behaviors to evade the dynamic pressures exerted by one or more enemies. We also argue that, depending on the position of a victim in the food web, resource allocation may be focused more into behaviors for avoiding lethal enemies as opposed to non-lethal ones, or vice versa.
In practice, we predict that the allocation of resources toward avoidance behaviors may translate into the amount of time that victims spend scanning their environment for enemy cues. Victims may also evolve to invest more or less of their time or energy avoiding certain areas or activities, based on perceived risk in the landscape of peril. Such activities could result in the loss of foraging opportunities and potential energy intake. Additionally, we predict that the evolution of resource allocation impacts the sensitivity of victims toward enemy cues. Therefore, depending on the pressures imposed by the enemies they evolve with, victims may be more or less attuned to the environmental signals left by enemies. To date, there are only a few studies that simultaneously look at the complex interactions between victims and their multiple types of enemies; the ones that do exist focus on a limited set of study systems (Buck et al., 2018). In sum, this evolutionary framework stresses the importance of considering the impacts of multiple enemies with differential fitness costs and levels of risk. There is still much to understand in this burgeoning field of research and this perspective will hopefully provide ideas for stimulating new studies about the landscape of peril.
All datasets generated for this study are included in the article/Supplementary Material.
J-FD and BR conceptualized the idea of the manuscript. J-FD wrote the manuscript. BR critically revised it. All authors contributed to the article and approved the submitted version.
This work was supported by the University of Otago Doctoral Scholarships to the authors and a Natural Sciences and Engineering Research Council of Canada (NSERC) scholarship (PGSD3-530445-2019) to J-FD.
The authors declare that the research was conducted in the absence of any commercial or financial relationships that could be construed as a potential conflict of interest.
We thank JK for the invitation (on behalf of the other topic editors) to contribute to this Research Topic. We also thank Robert Poulin for feedback and constructive criticism on an earlier draft.
The Supplementary Material for this article can be found online at: https://www.frontiersin.org/articles/10.3389/fevo.2020.564343/full#supplementary-material
Amoroso, C. R., Kappeler, P. M., Fichtel, C., and Nunn, C. L. (2019). Fecal contamination, parasite risk, and waterhole use by wild animals in a dry deciduous forest. Behav. Ecol. Sociobiol. 73:153. doi: 10.1007/s00265-019-2769-6
Berentsen, A. R., Becker, M. S., Stockdale-Walden, H., Matandiko, W., McRobb, R., and Dunbar, M. R. (2012). Survey of gastrointestinal parasite infection in African lion (Panthera leo), African wild dog (Lycaon pictus) and spotted hyaena (Crocuta crocuta) in the Luangwa Valley, Zambia. Afr. Zool. 47, 363–368. doi: 10.3377/004.047.0204
Bjork, K. E., Averbeck, G. A., and Stromberg, B. E. (2000). Parasites and parasite stages of free-ranging wild lions (Panthera leo) of northern Tanzania. J. Zoo Wildl. Med. 31, 56–61. doi: 10.1638/1042-7260(2000)031[0056:papsof]2.0.co;2
Bonnot, N. C., Morellet, N., Hewison, A. J. M., Martin, J.-L., Benhamou, S., and Chamaille-Jammes, S. (2016). Sitka black-tailed deer (Odocoileus hemionus sitkensis) adjust habitat selection and activity rhythm to the absence of predators. Can. J. Zool. 94, 385–394. doi: 10.1139/cjz-2015-0227
Bordes, F., and Morand, S. (2009). Parasite diversity: an overlooked metric of parasite pressures? Oikos 118, 801–806. doi: 10.1111/j.1600-0706.2008.17169.x
Buck, J. C. (2019). Indirect effects explain the role of parasites in ecosystems. Trends Parasitol. 35, 835–847. doi: 10.1016/j.pt.2019.07.007
Buck, J. C., Weinstein, S. B., and Young, H. S. (2018). Ecological and evolutionary consequences of parasite avoidance. Trends Ecol. Evol. 33, 619–632. doi: 10.1016/j.tree.2018.05.001
Costello, M. J. (2016). Parasite rates of discovery, global species richness and host specificity. Integr. Comp. Biol. 56, 588–599. doi: 10.1093/icb/icw084
Curtis, V. A. (2014). Infection-avoidance behaviour in humans and other animals. Trends Immunol. 35, 457–464. doi: 10.1016/j.it.2014.08.006
Dunne, J. A., Lafferty, K. D., Dobson, A. P., Hechinger, R. F., Kuris, A. M., Martinez, N. D., et al. (2013). Parasites affect food web structure primarily through increased diversity and complexity. PLoS Biol. 11:e1001579. doi: 10.1371/journal.pbio.1001579
Fill, A., Long, E. Y., and Finke, D. L. (2012). Non-consumptive effects of a natural enemy on a non-prey herbivore population. Ecol. Entomol. 37, 43–50. doi: 10.1111/j.1365-2311.2011.01333.x
Gaynor, K. M., Brown, J. S., Middleton, A. D., Power, M. E., and Brashares, J. S. (2019). Landscapes of fear: spatial patterns of risk perception and response. Trends Ecol. Evol. 34, 355–368. doi: 10.1016/j.tree.2019.01.004
Hart, B. L., and Hart, L. A. (1994). Fly switching by Asian elephants: tool use to control parasites. Anim. Behav. 48, 35–45. doi: 10.1006/anbe.1994.1209
Hart, B. L., and Hart, L. A. (2018). How mammals stay healthy in nature: the evolution of behaviours to avoid parasites and pathogens. Philos. Trans. R. Soc. B Biol. Sci. 373:20170205. doi: 10.1098/rstb.2017.0205
Hart, B. L., Hart, L. A., McCoy, M., and Sarath, C. R. (2001). Cognitive behaviour in Asian elephants: use and modification of branches for fly switching. Anim. Behav. 62, 839–847. doi: 10.1006/anbe.2001.1815
Hutchings, M. R., Gordon, I. J., Kyriazakis, I., and Jackson, F. (2001). Sheep avoidance of faeces-contaminated patches leads to a trade-off between intake rate of forage and parasitism in subsequent foraging decisions. Anim. Behav. 62, 955–964. doi: 10.1006/anbe.2001.1837
Ioannou, C. C., Ruxton, G. D., and Krause, J. (2008). Search rate, attack probability, and the relationship between prey density and prey encounter rate. Behav. Ecol. 19, 842–846. doi: 10.1093/beheco/arn038
Kamiya, T., O’Dwyer, K., Nakagawa, S., and Poulin, R. (2014). What determines species richness of parasitic organisms? A meta-analysis across animal, plant and fungal hosts. Biol. Rev. 89, 123–134. doi: 10.1111/brv.12046
Koprivnikar, J., and Penalva, L. (2015). Lesser of two evils? Foraging choices in response to threats of predation and parasitism. PLoS One 10:e0116569. doi: 10.1371/journal.pone.0116569
Lafferty, K. D., Allesina, S., Arim, M., Briggs, C. J., de Leo, G., Dobson, A. P., et al. (2008). Parasites in food webs: the ultimate missing links. Ecol. Lett. 11, 533–546. doi: 10.1111/j.1461-0248.2008.01174.x
Lafferty, K. D., and Kuris, A. M. (2002). Trophic strategies, animal diversity and body size. Trends Ecol. Evol. 17, 507–513. doi: 10.1016/s0169-5347(02)02615-0
Lafferty, K. D., and Kuris, A. M. (2009). Parasitic castration: the evolution and ecology of body snatchers. Trends Parasitol. 25, 564–572. doi: 10.1016/j.pt.2009.09.003
Laundré, J. W., Hernández, L., and Altendorf, K. B. (2001). Wolves, elk, and bison: reestablishing the “landscape of fear” in Yellowstone National Park, USA. Can. J. Zool. 79, 1401–1409. doi: 10.1139/cjz-79-8-1401
Luong, L. T., Horn, C. J., and Brophy, T. (2017). Mitey costly: energetic costs of parasite avoidance and infection. Physiol. Biochem. Zool. 90, 471–477. doi: 10.1086/691704
MacLeod, K. J., Krebs, C. J., Boonstra, R., and Sheriff, M. J. (2018). Fear and lethality in snowshoe hares: the deadly effects of non-consumptive predation risk. Oikos 127, 375–380. doi: 10.1111/oik.04890
Marino, J. A., and Werner, E. E. (2013). Synergistic effects of predators and trematode parasites on larval green frog (Rana clamitans) survival. Ecology 94, 2697–2708. doi: 10.1890/13-0396.1
Mendl, M., Burman, O. H. P., and Paul, E. S. (2010). An integrative and functional framework for the study of animal emotion and mood. Proc. R. Soc. B Biol. Sci. 277, 2895–2904. doi: 10.1098/rspb.2010.0303
Moléon, M., Martinez-Carrasco, C., Muellerklein, O. C., Getz, W. M., Muñoz-Lozano, C., and Sánchez-Zapata, J. A. (2017). Carnivore carcasses are avoided by carnivores. J. Anim. Ecol. 86, 1179–1191. doi: 10.1111/1365-2656.12714
Mols, C. M. M., van Oers, K., Witjes, L. M. A., Lessells, C. M., Drent, P. J., and Visser, M. E. (2004). Central assumptions of predator-prey models fail in a semi-natural experimental system. Proc. R. Soc. B Biol. Sci. 271, S85–S87. doi: 10.1098/rsbl.2003.0110
Norris, K. (1999). A trade-off between energy intake and exposure to parasites in oystercatchers feeding on a bivalve mollusc. Proc. R. Soc. B Biol. Sci. 266, 1703–1709. doi: 10.1098/rspb.1999.0835
Poulin, R. (2011). The many roads to parasitism: a tale of convergence. Adv. Parasitol. 74, 1–40. doi: 10.1016/B978-0-12-385897-9.00001-X
Poulin, R. (2014). Parasite biodiversity revisited: frontiers and constraints. Int. J. Parasitol. 44, 581–589. doi: 10.1016/j.ijpara.2014.02.003
Raffel, T. R., Martin, L. B., and Rohr, J. R. (2008). Parasites as predators: unifying natural enemy ecology. Trends Ecol. Evol. 23, 610–618. doi: 10.1016/j.tree.2008.06.015
Sarabian, C., Belais, R., and Maclntosh, A. J. J. (2018a). Feeding decisions under contamination risk in bonobos. Philos. Trans. R. Soc. B Biol. Sci. 373:20170195. doi: 10.1098/rstb.2017.0195
Sarabian, C., Curtis, V., and McMullan, R. (2018b). Evolution of pathogen and parasite avoidance behaviours. Philos. Trans. R. Soc. B Biol. Sci. 373:20170256. doi: 10.1098/rstb.2017.0256
Seppälä, O., Karvonen, A., and Valtonen, E. T. (2008). Shoaling behaviour of fish under parasitism and predation risk. Anim. Behav. 75, 145–150. doi: 10.1016/j.anbehav.2007.04.022
Shang, G.-Z., Zhu, Y.-H., Wu, Y., Cao, Y.-F., and Bian, J.-H. (2019). Synergistic effects of predation and parasites on the overwinter survival of root voles. Oecologia 191, 83–96. doi: 10.1007/s00442-019-04455-4
Sheriff, M. J., Peacor, S. D., Hawlena, D., and Thaker, M. (2020). Non-consumptive predator effects on prey population size: a dearth of evidence. J. Anim. Ecol. 89, 1302–1316. doi: 10.1111/1365-2656.13213
Sheriff, M. J., and Thaler, J. S. (2014). Ecophysiological effects of predation risk; an integration across disciplines. Oecologia 176, 607–611. doi: 10.1007/s00442-014-3105-5
Sinclair, A. R. E., Mduma, S., and Brashares, J. S. (2003). Patterns of predation in a diverse predator-prey system. Nature 425, 288–290. doi: 10.1038/nature01934
Stephenson, J. F., Perkins, S. E., and Cable, J. (2018). Transmission risk predicts avoidance of infected conspecifics in Trinidadian guppies. J. Anim. Ecol. 87, 1525–1533. doi: 10.1111/1365-2656.12885
Strauss, A. T., Hite, J. L., Civitello, D. J., Shocket, M. S., Caceres, C. E., and Hall, S. R. (2019). Genotypic variation in parasite avoidance behaviour and other mechanistic, nonlinear components of transmission. Proc. R. Soc. B Biol. Sci. 286:20192164. doi: 10.1098/rspb.2019.2164
Sukhdeo, M. V. K. (2012). Where are the parasites in food webs? Parasit. Vectors 5:239. doi: 10.1186/1756-3305-5-239
Weinstein, S. B., Buck, J. C., and Young, H. S. (2018a). A landscape of disgust. Science 359, 1213–1214. doi: 10.1126/science.aas8694
Weinstein, S. B., Moura, C. W., Mendez, J. F., and Lafferty, K. D. (2018b). Fear of feces? Tradeoffs between disease risk and foraging drive animal activity around raccoon latrines. Oikos 127, 927–934. doi: 10.1111/oik.04866
Keywords: avoidance behavior, fear, disgust, natural enemy, predator, parasite, non-consumptive effect
Citation: Doherty J-F and Ruehle B (2020) An Integrated Landscape of Fear and Disgust: The Evolution of Avoidance Behaviors Amidst a Myriad of Natural Enemies. Front. Ecol. Evol. 8:564343. doi: 10.3389/fevo.2020.564343
Received: 21 May 2020; Accepted: 02 September 2020;
Published: 18 September 2020.
Edited by:
Janet Koprivnikar, Ryerson University, CanadaReviewed by:
Hillary Young, University of California, Santa Barbara, United StatesCopyright © 2020 Doherty and Ruehle. This is an open-access article distributed under the terms of the Creative Commons Attribution License (CC BY). The use, distribution or reproduction in other forums is permitted, provided the original author(s) and the copyright owner(s) are credited and that the original publication in this journal is cited, in accordance with accepted academic practice. No use, distribution or reproduction is permitted which does not comply with these terms.
*Correspondence: Jean-François Doherty, amVmZi1kb2hlcnR5QGhvdG1haWwuY29t
Disclaimer: All claims expressed in this article are solely those of the authors and do not necessarily represent those of their affiliated organizations, or those of the publisher, the editors and the reviewers. Any product that may be evaluated in this article or claim that may be made by its manufacturer is not guaranteed or endorsed by the publisher.
Research integrity at Frontiers
Learn more about the work of our research integrity team to safeguard the quality of each article we publish.