- 1School of Biological Sciences, The University of Western Australia, Crawley, WA, Australia
- 2The UWA Oceans Institute, The University of Western Australia, Crawley, WA, Australia
- 3Marine Science Program, Parks and Wildlife Service, Department of Biodiversity, Conservation and Attractions, Kensington, WA, Australia
- 4Biodiversity and Conservation Science, Parks and Wildlife Service, Department of Biodiversity, Conservation and Attractions, Kensington, WA, Australia
Just as organisms do not passively exist in their environment, developing sea turtle embryos affect their own incubation microclimate by producing metabolic heat and other waste products. This metabolic heat ultimately contributes to successful development, but it is unclear how it influences embryonic traits such as hatchling sex, and whether trends exist in the magnitude of metabolic heat produced by different species. In this systematic review, we document all empirical measurements of metabolic heat in sea turtle species, its impact on their development, and explore the methods used to predict nest temperature and how these methods account for metabolic heat. Overall we found metabolic heat increases throughout incubation in all seven species. Typically, the temperature at the center of an egg clutch peaked during the final third of incubation, and exceeded the adjacent sand temperature by 2.5°C. Metabolic heat was not influenced by species or clutch size (n = 16 studies), but this finding was likely influenced by inconsistency in the methods used to measure metabolic heat, and by localized differences in sand properties, such as moisture, albedo, and thermal conductivity. The influence of metabolic heat on embryo sex determination and survival was dependent on the sand temperature surrounding the nest chamber, and had an appreciable affect only when it caused nest temperatures to exceed key thresholds for sex determination and survival. Methods for modeling nest temperature were either correlative (70% of publications) or mechanistic (30% of publications). Correlative models describe relationships between empirical data to predict sand temperature, while mechanistic models are based on physical laws and do not require extrapolation when predicting novel situations, such as climate change. Most correlative models (74%) accounted for metabolic heat when predicting nest temperatures, but no mechanistic models did—largely because they were developed for predicting primary sex ratios, which are widely believed to be unaffected by metabolic heat. Consequently, developing a generalisable mechanistic model that incorporates metabolic heat will be critical for predicting incubation success as the sand temperatures at sea turtle beaches increase due to anthropogenic climate change.
Introduction
Climate change presents major physiological challenges to many organisms, and ectotherms are particularly vulnerable because temperature has a profound effect on all aspects of their life cycle (Angilletta, 2009). Temperature-dependent sex determination (TSD) occurs in many reptile species (Bull, 1980), and in sea turtles males develop at cooler incubation temperatures and females develop at warmer temperatures (Wibbels, 2003). Sea turtle embryos are particularly sensitive to small changes in sand temperature, as mixed sexes are produced within a narrow range of incubation temperatures known as the transitional range of temperatures (TRT), beyond this range single-sexes are produced (Ackerman, 1977). Further, at high incubation temperatures hatchlings may develop to be smaller, with reduced crawling and swimming speeds (Booth and Astill, 2001; Booth et al., 2004; Booth and Evans, 2011), and may have higher instances of developmental abnormalities (Zimm et al., 2017). Prolonged exposure to temperatures above the TRT can also result in death (Howard et al., 2014).
A range of factors define the temperatures that a sea turtle embryo experiences throughout its terrestrial incubation—primarily the temperature of the sand bounding the nest chamber which is influenced by air temperature, but also by nest depth and distance to the sea, shade from vegetation (Loop et al., 1995; Kamel, 2013), sand reflectance (albedo), and thermal conductivity (Speakman et al., 1998). Biological processes, such as decomposition of organic material and metabolic heat generated by developing embryos, also contribute to incubation temperature. Metabolic heat is produced through the process of converting yolk and albumen into embryonic tissue and other byproducts, including carbon dioxide and water, while oxygen is consumed (Deeming, 2004). Although the metabolic heat produced by a reptile embryo is small, in larger clutches of reptile eggs, including those of sea turtles (Broderick et al., 2001), alligators (Ewert and Nelson, 2003), and freshwater turtles (Massey et al., 2019), this heat is sufficient to warm the nest well above the temperature of the surrounding sand. Generally eggs at the top of a nest chamber are warmer during the earlier stages of incubation (Kaska et al., 1998a,b), but around halfway through incubation eggs at the center become warmest. Throughout incubation eggs at the periphery and base of the nest chamber are the coolest (Vázquez Luna et al., 2000).
Sea turtles lay their nests 40–80 cm below the surface of nesting beaches, and average nest depth varies between species (Kaska et al., 1998b; Koch et al., 2007; Santidrián Tomillo et al., 2017). Sand temperatures at these depths are frequently used to estimate temperatures inside the sea turtle nest chamber (e.g., Bentley et al., 2020), and much work has gone into predicting how climate change will drive changes in sand temperature, and thereby alter hatching traits (e.g., Hawkes et al., 2007; Fuentes et al., 2009; Stubbs et al., 2014). Globally female-biased primary sex ratios are prevalent at nesting beaches (Hays et al., 2014), and wide-scale feminisation of populations is a persistent threat in a rapidly warming climate (Hawkes et al., 2009; Fuentes et al., 2011; Jensen et al., 2018). However, because males mate more frequently than females, female biases in the adult population translate to a more balanced operational sex ratio (Hays et al., 2010). Of more immediate concern is high embryonic mortality at incubation temperatures above ~35°C (Howard et al., 2014). Consequently, predictive models that account for metabolic heat—alongside other biotic and abiotic drivers—will be critical for evaluating whether sea turtle rookeries can continue to produce viable hatchlings under a warming climate.
Nest temperatures often deviate from sand temperatures toward the end of embryonic development when the amount of waste products produced, including heat, is greatest. The onset of metabolic heat production in sea turtle nests occurs when the temperature of a nest exceeds the temperature of the surrounding sand by more than 1°C. Using this definition metabolic heat generally becomes apparent in the final third of embryo development, and therefore should not influence sex determination, which occurs during a thermosensitive period (TSP) in the middle third to one half of development to hatching stage (Woolgar et al., 2013; Howard et al., 2014; Stubbs et al., 2014; Sari and Kaska, 2016). Despite this general assumption, there is some evidence of substantial metabolic heat during the TSP in green turtle nests (e.g., Cheng et al., 2015). While the impact of metabolic heat on early-stage embryos may be undetectable, its greater effect on late-stage embryos may be consequential if it leads to mortality—e.g., metabolic heating of 8°C during the final third of development has been documented in leatherback turtle nests (Binckley et al., 1998).
Although early studies showed that metabolic heat warms sea turtle nests above sand temperature (Hendrickson, 1958; Carr and Hirth, 1961), using sand temperature as a proxy for nest temperature is a popular approach for predicting thermal impacts on primary sex ratios and embryonic survival (e.g., Fuentes et al., 2009; Jensen et al., 2018). Most studies use correlative methods to predict sand temperature by describing the relationship between climate variables (such as sea surface temperature and air temperature) and sand temperature (e.g., Godley et al., 2001) and using these relationships to forecast sand temperature under climate change scenarios (Fuentes et al., 2009; Katselidis et al., 2012; Patino-Martinez et al., 2012). Using correlative models to investigate a novel environment (e.g., climate change) requires extrapolation of the model beyond the data underlying it, consequently correlative models have limited use in the context of climate change (Dormann et al., 2012). In contrast, mechanistic models are based on physical laws (such as heat and mass balance) and allow microclimates to be predicted under novel combinations of drivers, and so are ideal for exploring global change scenarios. In the context of reptile ecology and conservation, mechanistic models have been widely used to investigate how climate, soil, and topography interact to impact nest site suitability in species with TSD (e.g., Mitchell et al., 2008; Fuentes and Porter, 2013; Dudley and Porter, 2014; Hays et al., 2014; Stubbs et al., 2014; Cavallo et al., 2015; Kearney et al., 2018; Bentley et al., 2020).
Applications of sand temperature models are not confined to studies of climate change; models are also valuable tools in conservation programs that relocate nests to avoid tidal inundation and predation (Dutton et al., 2005; Kornaraki et al., 2006). For example, to ensure nest relocation results in high survival and maintains natural sex ratios, the ability to accurately predict nest temperatures, and to include the influence of metabolic heat, is important. Yet for both modeling applications the methods for incorporating the influence of metabolic heat on incubation temperature are inconsistent, and there is little consensus on how metabolic heat affects hatchling traits and survival. Here we systematically review all literature on metabolic heat in the nests of all species of sea turtle: flatback (Natator depressus), green (Chelonia mydas), hawksbill (Eretmochelys imbricate), Kemp's ridley (Lepidochelys kempii), leatherback (Dermochelys coriacea), loggerhead (Caretta caretta), and olive ridley (Lepidochelys olivacea), and focus on three key questions. Firstly, what are the measured values of metabolic heat in sea turtle nests; secondly, what effect does metabolic heat have on embryonic development and survival; and thirdly, what are the strengths and weaknesses of existing modeling methods for incorporating metabolic heat into predictions of nest temperature?
Methods
We followed the Preferred Reporting Items for Systematic Reviews and Meta-analyses [PRISMA; Moher et al. (2009), Figure 1], and conducted a literature search of the Web of Science, Scopus, and BIOSIS databases using the following search strategy (adapted for each style of search engine): (“metabolic heating in nest*” OR “metabolic heat*” OR “metabolic warming” OR “incubation temperature*” OR “nest* environment*” OR “nest* temperature*” OR “nest* chamber*” OR “nest* parameters” OR “clutch environment” OR “egg chamber”) AND (“marine turtle species” OR “sea turtle*” OR chelonioidea OR “chelonia mydas” OR reptilian OR testudines OR cryptodira OR flatback OR “natator depressus” OR leatherback OR “dermochelys coriacea” OR “green turtle*” OR “chelonian mydas” OR “loggerhead turtle*” OR “caretta caretta” OR “olive ridley” OR “lepidochelys olivacea” OR hawksbill OR “eretmochelys imbricate” OR “kemp* ridley turtle*” OR “atlantic ridley turtle*” OR “lepidochelys kempii”) AND (Sex OR Feminization OR female-bias OR “sex ratio” OR “sex ratio estimation” OR “sex determination” OR “temperature dependent sex determination” OR “hatchling sex ratio” OR “sexual differentiation” OR “sex allocation” OR “temperature-dependent sex determination” OR TSD) AND (estimat* AND calculat* OR “temperature data-loggers” OR method* OR predict* OR “temperature recorder*” OR measure*).
We cross-referenced this search with two key qualitative reviews by Booth (2017) and Howard et al. (2014), and included any papers cited therein missed in our initial search. To supplement the primary literature, we screened conference proceedings for the Annual Symposium on Sea Turtle Biology and Conservation1. An incomplete collection of proceedings is available for the years 1998−2017 (proceedings from 2012 and 2015 are missing). Two book chapters were also included (Ackerman, 1997; Deeming, 2004) and one Master's thesis (Woolgar, 2012).
The title and abstract of each record were screened, and the record excluded from the review if it did not relate to sea turtles, or general nest biology for reptiles. Entire records were then read and included if they met at least one of the following criteria: (1) measured metabolic heat in sea turtle nests; (2) referenced metabolic heat in the context of embryonic survival and development; and (3) used a model or equation to predict nest or sand temperature at nesting beaches. Studies meeting these criteria were summarized in a spreadsheet and used to address the key questions of this review.
Studies were further selected for a quantitative summary of empirical records of metabolic heat and only studies that met the following two criteria were included: (1) metabolic heat was measured at the center of the egg mass, relative to sand temperature; and (2) metabolic heat was recorded as an average for each trimester of incubation. Records were excluded if only an average for the entire incubation period was given. If a range of metabolic heat was provided for each trimester of incubation, the mid-point of this range was used. For data which met these conditions, the mean and standard error for metabolic heat per incubation trimester, and for each species was calculated. A non-parametric Kruskal–Wallis H-test was used to test for significant differences between group means. Of the studies that included data on clutch size, we explored the influence of clutch size and species on metabolic heat during each trimester using linear models. The statistical package R (version 3.6.0; R Core Team, 2019) was used for all analyses and the Lme4 extension used for linear models (Bates et al., 2015) which included metabolic heat as the response variable, and clutch size and species as fixed covariates.
Results
Our database search of peer-reviewed publications generated 365 unique papers and yielded an unbiased sample of studies, indexed up until Tuesday 18th February 2020, and 40 records were located via conference proceedings, book chapters and by cross-referencing with existing reviews. A total of 126 studies were included in this review, comprising 93 peer-reviewed papers, 30 conference abstracts, two book chapters and one thesis (Figure 1). Studies of loggerhead (n = 46) and green (n = 42) turtles had the highest representation, while flatback (n = 7), olive ridley (n = 5), and Kemp's ridley (n = 5) turtles had the lowest representation (Figure 1).
Metabolic Heat Measured in Natural Nests
Not all studies provided information on clutch size, so sample size was reduced to 32, across 16 studies, for the linear model analysis (Table S1). While there was no significant effect of clutch size (F1,24 = <0.05, p = 0.99) or species (F 4, 24 = 1.21, p = 0.33) on metabolic heat, the trimester of incubation had a significant effect on metabolic heat (F2,24 = 12.86, p < 0.05). A total of 51 measurements, made across 31 studies, were used to quantify the average metabolic heat produced in sea turtle nests during each trimester (Table S1). No studies of Kemp's ridley turtle met the specified requirements, hence data for this species are not shown. A Kruskal–Wallis H-test showed a significant difference in mean metabolic heat between trimesters of incubation, χ2(2) = 28.23, p < 0.001, with mean metabolic heat of 0.5 ± 0.2°C (standard error) in the first trimester of incubation, rising to 0.9 ± 0.1°C in the middle trimester, and 2.6 ± 0.3°C in the final trimester (Figure 2). Variation in metabolic heat was greatest in the final trimester, ranging from 1.2°C in loggerhead nests (Kuriat Island, Tunisia) (Jribi and Bradai, 2014) to 4–8°C in leatherback nests (Playa Grande) (Binckley et al., 1998). One study estimated metabolic heat generated by olive ridley embryos from a thermal balance model based on temperatures measured at the center of a nest, and in the sand above and beside the nest (Sandoval et al., 2011). No metabolic heat was detected before day 19 of incubation, but it then increased to a maximum of 0.84 Watts on day 34 when the nest was destroyed by a storm. Most studies reported that metabolic heat peaked in the final trimester, across all species (Figure 2: e.g., Howard et al., 2014; Sari and Kaska, 2016), and was generally followed by a decrease in nest temperature 0–9 days before emergence (Chan and Liew, 1995; Neville et al., 1998; Koch et al., 2007; Uçar et al., 2013; Sari and Kaska, 2016). However, one study of green sea turtle nests at Lanyu Island, Taiwan reported that average metabolic heat across 14 nests peaked during the middle trimester, although the difference in average metabolic heat between the second and third semester was not statistically significant (Cheng et al., 2015).
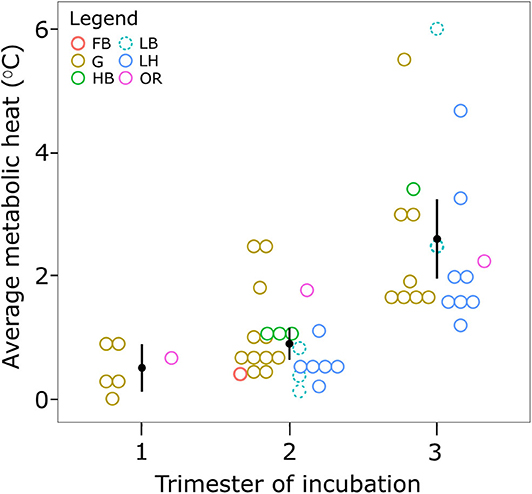
Figure 2. Average metabolic heat recorded in the nests of sea turtles in each trimester of development. Only studies that measured metabolic heat by comparing the temperature in the center of a clutch to that of the surrounding sand are included. Metabolic heat is reported as the difference in these two values. Symbols represent species where FB, flatback; G, green; HB, hawksbill; LB, leatherback; LH, loggerhead; OR, olive ridley.
The Effect of Metabolic Heat on Embryonic Development and Survival
Any influence of metabolic heat on sex determination can only be apparent if metabolic heat is pronounced during the TSP. Twenty percent of 40 studies that documented metabolic heat found metabolic heat exceeding 1°C during the TSP (Hays et al., 2001; Glen and Mrosovsky, 2004; Chen et al., 2010; Valverde et al., 2010; Barrientos-Muñoz et al., 2012; Cheng et al., 2015). Three studies calculated the feminizing effect of metabolic heat in the nests of green sea turtles. In these instances, the effect was estimated by calculating the primary sex ratio based on the nest temperature during the TSP, and contrasting this to the sex ratio predicted from sand temperatures over the same range of dates. These studies concluded that metabolic heat accounted for up to 30% more females produced on Ascension Island (Broderick et al., 2001), and between 7% (Sönmez, 2018) and 10% (Önder and Candan, 2016) more females produced in Turkey.
All studies, except one, that documented metabolic heat (n = 40) found it was most evident in the third trimester when the sex of embryos is already determined. Metabolic heating is higher at the center than the side of a clutch (Godfrey et al., 1997; Booth and Astill, 2001), and one study reported that the periphery of loggerhead nests is 0.4–0.9°C cooler than the nest center during the TSP (Hanson et al., 1998). For studies that analyzed nests that produced mixed sexes, male hatchlings more often developed at the periphery of nests (Standora et al., 1982; Patino-Martinez et al., 2012).
Sand temperatures moderate the effect of metabolic heat on embryos, as only when sand temperatures at a nesting beach are just below or within the TRT, or nearing upper limits of thermal tolerance, does metabolic heat have an appreciable effect. For example, if sand temperatures are already high enough to produce 100% females, metabolic heat has no effect on primary sex ratio (Binckley et al., 1998), but may still lower survival. This effect was documented by Bladow and Milton (2019), where late-stage green turtle and loggerhead turtle embryos experience high mortality due to exposure to elevated temperatures resulting from high sand temperatures and increasing metabolic heat.
Modeling of Sand or Nest Temperatures
This review identified 30 studies that used relationships between environmental variables to predict nest or sand temperatures on sea turtle nesting beaches. We broadly categorized these methods as either correlative or mechanistic (Figure 3). Correlative models (22 publications; 70% of total) were mostly linear regressions based on readily sourced environmental data, such as ambient air temperatures and sea surface temperatures. In contrast, mechanistic models (eight publications, 30% of total) applied mass and energy balance dynamics to simulate nest temperatures.
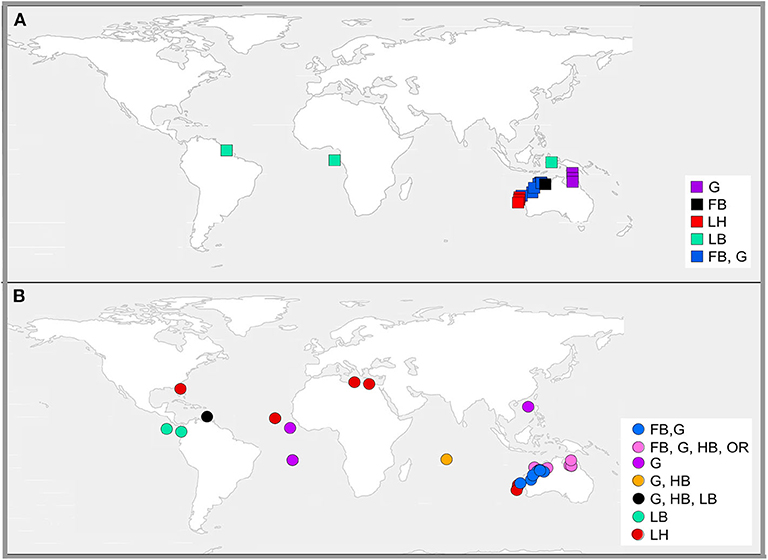
Figure 3. Locations where mechanistic models [square symbols, (A)] and correlative models [circle symbols, (B)] have been used to predict sand or nest temperature at turtle rookeries. Symbol colors represent the focal species nesting at each site, where FB, flatback; G, green; HB, hawksbill; LB, leatherback; LH, loggerhead; and OR, olive ridley.
Correlative Models
Correlative models (Table 1; Figure 3B) were either based on linear equations (19 publications, 86% of correlative models) or generalized models (3 publications, 14% of correlative models) (Girondot and Kaska, 2015; Montero et al., 2018, 2019). Long term (both historical and projected) nest or incubation temperatures were most often predicted from ambient air temperatures recorded over long periods (Godley et al., 2002; Hays et al., 2003; Hawkes et al., 2007; Furst et al., 2012; Katselidis et al., 2012; Patino-Martinez et al., 2012; Laloë et al., 2014, 2016; Santidrian Tomillo et al., 2015; Patrício et al., 2017) or from ambient air temperature and sea surface temperature combined (Fuentes et al., 2009; Woolgar, 2012; Jensen et al., 2018; Bentley et al., 2020). Studies either used the empirical relationships between weather variables and nest or sand temperatures to predict nest temperatures within the same nesting season (Godley et al., 2002; King et al., 2013; Esteban et al., 2016), or reconstructed historical sand temperatures (e.g., Hays et al., 2003), or predicted sand temperatures into the future (e.g., Fuentes et al., 2009).
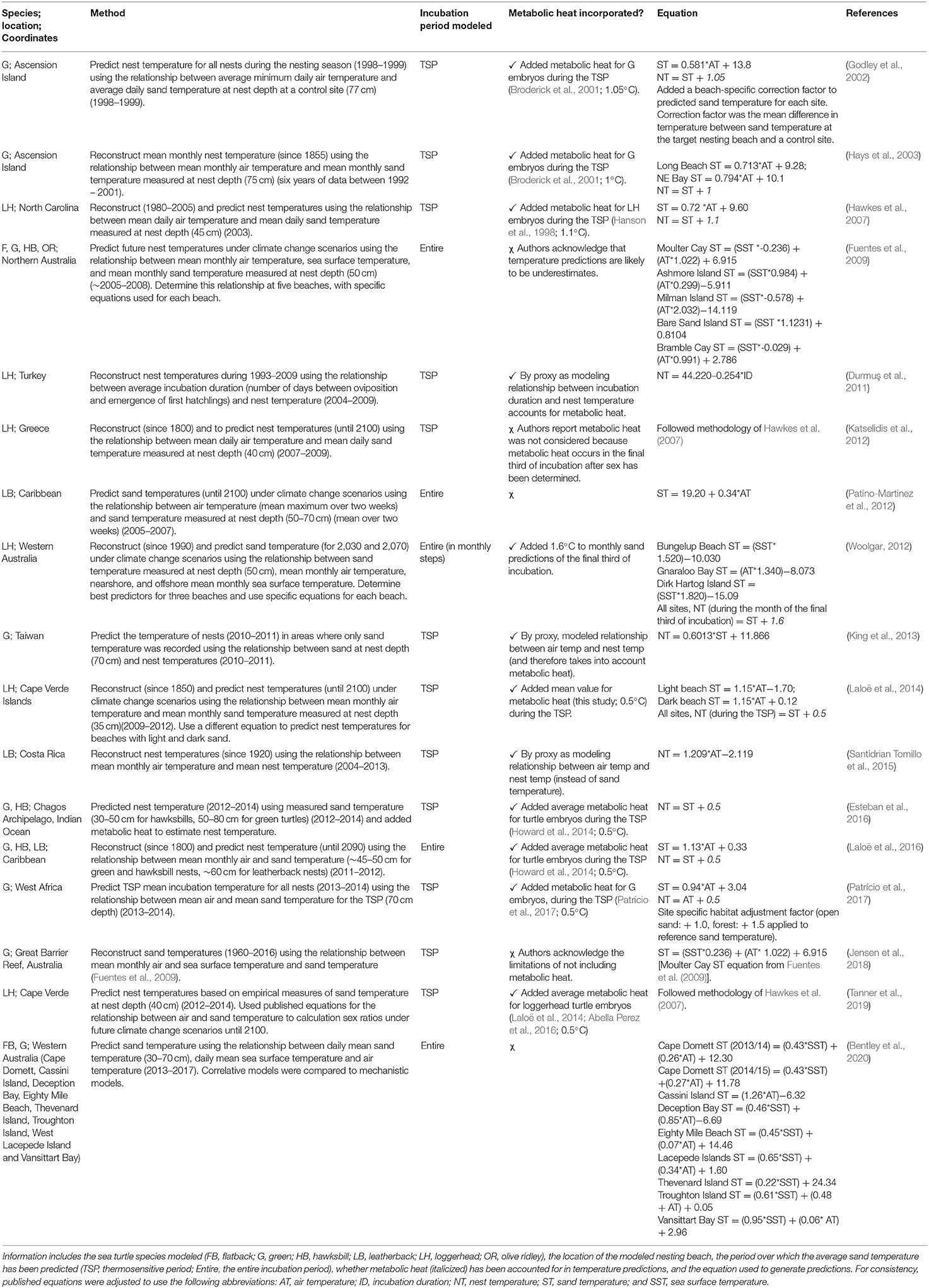
Table 1. An overview of all correlative models used to predict the temperature of marine turtle nests, or sand temperature on nesting beaches, as generated by systematic review, and shown in chronological order.
Linear Models
Seventy percent of linear models (13 publications) predicted nest temperature for the TSP, while the remainder predicted nest temperature for the entire incubation period (Table 1). Most studies (74%, 14 publications) accounted for metabolic heat, often by adding an amount of metabolic heat to sand temperature predictions, thereby converting these to nest temperature (Table 1; e.g., Godley et al., 2002; Laloë et al., 2014). This conversion was not necessary for studies that made predictions based on the relationships between weather variables and nest temperature (instead of sand temperature) (e.g., Santidrián Tomillo et al., 2014), or incubation duration (Durmuş et al., 2011), as metabolic heat was already accounted for.
Generalized Models
Three studies used generalized models to determine the relationship between weather variables, nest temperature, hatching success, and emergence success (Girondot and Kaska, 2015; Montero et al., 2018, 2019). Generalized models were selected when the distribution of errors was binomial (Montero et al., 2018, 2019), and this allowed inclusion of random effects such as nest identity (Girondot and Kaska, 2015) and the year nests were laid (Montero et al., 2018). Girondot and Kaska (2015) identified percentage of development a proxy measure for the amount of metabolic heat, as a useful variable from which to predict nest temperature.
Average air temperature during incubation and average precipitation during the months nests were laid were found to be the best predictors of emergence success in hawksbill nests in Brazil (Rio Grande and Bahia) (Montero et al., 2018), while average air temperature during incubation, in combination with accumulated rainfall during incubation, were the best predictors of emergence success in loggerhead nests, also in Brazil (Bahia, Espirito Santo, and Rio de Janeiro) (Montero et al., 2019). Using generalized models with autocorrelation, Girondot and Kaska (2015) predicted nest temperature in loggerhead turtle nests at Dalyan beach, Turkey. They found a linear relationship between the predictor variables and nest temperature, and showed that the effect of sea surface temperature lagged by one day, while the effect of air temperature lagged by two days.
Mechanistic Models
Relative to correlative methods, fewer studies (8 publications) used a mechanistic approach to predict sand temperatures (Figure 3A). Most (75%) mechanistic models used the microclimate model package in the software NicheMapR, and its earlier iteration Niche MapperTM, available for general applications in mechanistic and physiological ecology (Kearney and Porter, 2019). These six studies are summarized in Table 2. The remaining two studies developed alternative mechanistic models based on similar principles to NicheMapR's microclimate model (Mota and Carthy, 2004; Mota et al., 2006). Mota and Carthy (2004) created a series of equations to model sand temperature at different depths on nesting beaches. Their model first accounted for the transfer of solar radiation from the beach surface through partitioned layers of sand and into heat stores at different depths. It then considered moisture content, compaction levels, and conductance values, and modeled how these interactions influence heat flow. Mota et al. (2006) developed a one-dimensional model to predict sand temperature at different depths on nesting beaches, based on air temperature and beach-specific sand properties, including sand color, albedo, sand compactness, and moisture.
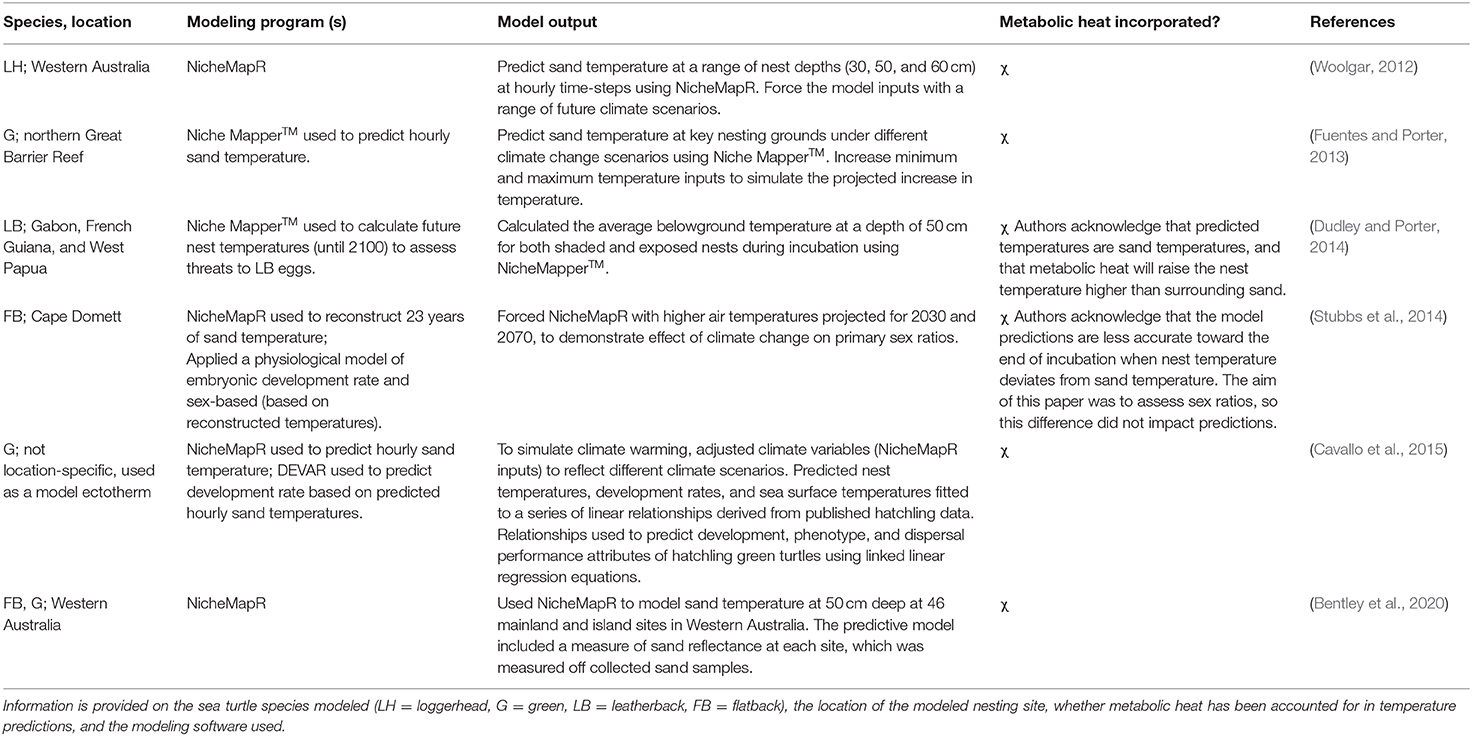
Table 2. An overview of all studies that used the mechanistic NicheMapR modeling platform to predict the temperature of sea turtle nests, or sand temperature on nesting beaches, shown in chronological order.
Three studies simultaneously compared correlative and mechanistic modeling approaches for predicting sand temperature on nesting beaches in Australia (Woolgar, 2012; Fuentes and Porter, 2013; Bentley et al., 2020). Each model type produced strong correlations with measured sand temperature (typically r2 > 0.7; Bentley et al., 2020). In the most comprehensive assessment of the two approaches, Bentley et al. (2020) tested the ability of microclimate models to predict sand temperatures at 50 cm depth at 46 mainland and island sea turtle rookeries in Western Australia. This assessment concluded that, despite similar model fits, key limitations of correlative models make mechanistic models a more robust method for predicting sand temperature. In particular, correlative models rely on the availability of long-term empirical data to produce strong fits, and these data may not be available for remote rookeries (e.g., those on offshore islands) that are difficult to access. Additionally, because mechanistic models are based on general physical laws (e.g., heat and mass balance) and an understanding of a system's components, they allow for microclimate predictions to be made under novel climates, such as those expected under climate change scenarios.
Discussion
Despite publication of over 700 studies on the early development of sea turtles, records of metabolic heat are relatively few, as are any reported influences of metabolic heat on key population parameters such as primary sex ratio and embryonic survival. Variation in the measured amount of metabolic heat is marked, but clear patterns emerged; metabolic heat warms nests above the temperature of the surrounding sand, increases throughout incubation, and peaks (averaging around 2.5°C) 0–9 days before hatchlings emerge from the nest. Metabolic heat also varies within and between species with metabolic heat in the final semester ranging from 1.2°C in loggerhead nests (Jribi and Bradai, 2014) to 4–8°C in leatherback nests (Binckley et al., 1998). While most studies measured metabolic heat as the difference in temperature between the clutch and the adjacent sand at the same depth, the placement of temperature loggers within the nest and at reference sites was inconsistent across studies.
Many biotic factors, including clutch size (Chan et al., 2006; Koch et al., 2007; Zbinden et al., 2007; Önder and Candan, 2016; Sönmez, 2018; García–Grajales et al., 2019) and microbial activity in dead eggs (Valverde et al., 2010), also contribute to incubation temperature, and in turn influence development rate (Koch et al., 2007) and emergence success (Mrosovsky and Yntema, 1980). This relationship between clutch size and metabolic heat has led to the inference that species showing geographic variation in clutch size (e.g., loggerheads, where Mediterranean populations lay larger clutches than Atlantic populations) may be differentially affected by metabolic heat (Zbinden et al., 2006), but we did not find any support for a relationship between clutch size and metabolic heat in our review.
Eggs Within the Same Nest Experience Different Temperatures Throughout Development
Metabolic heat contributes to temperature variation within the nest. As a result individual eggs may experience an incubation regime that is quite different to an egg deposited in a different region of the nest. In nests that are warm enough to produce 100% females, emergence success is reduced if metabolic heat causes central eggs to exceed their upper thermal tolerance limits toward the end of development. Because female clutches are at greater risk of mortality due to high temperatures, this effect can alter the primary sex ratio of a nesting population. For example, Santidrián Tomillo et al. (2014) quantified the effect of embryonic mortality on primary sex ratios in a population of leatherbacks at Playa Grande, Costa Rica over nine nesting seasons. The number of female hatchlings emerging from nests increased with increasing temperature until nest temperatures reached the upper limit of the transitional range of temperatures (~30°C in this population), and then decreased as embryos breached their thermal tolerance limits. Embryonic development at temperatures which intermittently exceed thermal limits also leads to sub-lethal effects such as poor swimming performance (Mueller et al., 2019), decreased crawling and self-righting speeds (Rivas et al., 2019), and scute abnormalities (Zimm et al., 2017).
Temperature variation within and between sea turtle nests also results in different development rates for individual eggs (Marco et al., 2017). For example, in loggerhead nests an approximately 1°C difference in nest temperature was attributed to a 4-day (Uçar et al., 2013) or 7-day (Kaska and Sezgin, 2019) difference in incubation duration. Further, temperature varaition within the nest chamber increases the asynchrony of hatchling emergence (Houghton and Hays, 2001). While hatchlings from the same nest generally emerge from the nest together, there is increasing evidence of asynchronous hatching (Houghton and Hays, 2001; Glen et al., 2005; Koch et al., 2008). For example, flatback turtle nests at a beach hatchery took an average of 3.1 days for all hatchlings to emerge, and shallower nests (35 cm deep) had greater emergence asynchrony (4.5 days) than deeper nests (50 cm) which took 1.7 days for all hatchlings to emerge (Koch et al., 2008).
Within-nest temperature variation makes the placement of temperature loggers important if the data they produce is used to develop models of nest temperature, or to broadly infer thermal effects on embryonic development. This review highlighted inconsistencies between studies in the placement of temperature loggers in nests and at reference sites. Researchers often count eggs while a female is nesting and position a logger when half the expected number of eggs are laid. Temperature loggers are generally placed in the center of the egg mass. Placing temperature loggers precisely while turtles are laying is challenging because clutch size can vary markedly from the average size. As a result, loggers may inadvertently be positioned closer to the clutch base or surface. Reference temperature loggers are also placed at a variety of locations, including adjacent to a nest (DeGregorio and Williard, 2011), at one location for all nests on the same beach (Zbinden et al., 2006), or along beach profile transects in different zones (forest, forest border, and low-lying vegetation) (Kamel and Mrosovsky, 2006). These differences in the selection of a reference temperature dataset could partially explain the variation in metabolic heat found by this review. Importantly, variation in temperature logger placement can lead to different estimates of both ambient sand temperature and metabolic heat, each of which is critical to predicting how sea turtles will develop under climate change. Hence, a consistent approach is recommended to allow comparisons between studies and to better understand the importance of metabolic heat as ambient sand temperatures warm due to climate change. We recommend calculating metabolic heat as the difference between temperature at the center of the clutch, and sand temperature at the same depth 1 m from the nest chamber, parallel to the shoreline. If the latter is not possible, then reference sand temperatures should be calculated at appropriate depths for different beach zones where sand temperatures vary due to distance to the high-tide mark, or shading, for example.
The Period When Metabolic Heat is Detectable Impacts Embryonic Development
While metabolic heat is most pronounced in the final third of incubation (Chan and Liew, 1995), some studies report metabolic heat during the middle third of incubation (Broderick et al., 2001; Önder and Candan, 2016; Sönmez, 2018). As this period corresponds with the TSP in sea turtle species (Mohanty-Hejmadi and Dimond, 1986; Desvages et al., 1993; Woolgar et al., 2013; Stubbs et al., 2014), metabolic heat can potentially influence offspring sex, and in one study was shown to account for up to a 30% increase in female production (Broderick et al., 2001). Temperature variation within the nest means each egg has a unique developmental trajectory, ultimately leading to asynchronous TSP's (Kaska and Sezgin, 2019). While this process promotes the production of mixed sexes from nests, it can be problematic if primary sex ratios are estimated from a sub-sample of hatchlings from a nest. For example, if hatchlings are collected simultaneously upon emergence, which in many cases may be logistically and ethically necessary, the sample may not accurately represent the sex ratio of the clutch, as hatchlings that emerge together are likely to be the same sex due to similar development rates and TSP.
Modeling Metabolic Heat in Sea Turtle Nests: Current Status and Future Directions
In this review we categorized all studies that predicted sand or nest temperature into those that used correlative or mechanistic methods. While the majority of correlative models accounted for metabolic heat, no mechanistic models did. Most correlative models predicted nest temperatures for the TSP (as their interest was usually to estimate the primary sex ratio of a focal nesting population), and many accounted for metabolic heat by adding a value representing metabolic heat to a predicted sand temperature. These values were either measured during the same study or were published values that typically ranged from 0.5–1°C (Godley et al., 2002; Hays et al., 2003; Hawkes et al., 2007; Laloë et al., 2014, 2016; Esteban et al., 2016; Patrício et al., 2017). Conversely, mechanistic models tended to predict sand temperature for the entire incubation period. However, because they did not account for metabolic heat, their accuracy declined toward the end of development when nest temperature deviates most from sand temperature.
Although our review showed that correlative and mechanistic methods produce similar fits to empirical data, mechanistic models have not been used extensively in sea turtle research because they require a greater range of inputs and can be challenging to employ. However, mechanistic models are best suited for modeling novel environments as they are based on the underlying physics of a system and can be extrapolated beyond the bounds of underlying data (Dormann et al., 2012). Therefore, mechanistic models can be used to forecast population traits such as primary sex ratios under future climates (e.g., Mitchell et al., 2008; Fuentes and Porter, 2013; Stubbs et al., 2014). Mechanistic models can also dynamically incorporate projected changes in environmental drivers such as mean air temperature, rainfall amount and timing, evaporation rates, and wind speeds—all of which are expected to change in future climates (Charles et al., 2015). Crucially, they can test the sensitivity of model outputs to changes in one driver, or a combination of drivers (Mitchell et al., 2008), and can be used to simulate manipulations, such as nest shading, as a way to mitigate impacts of climate change on primary sex ratios (Mitchell et al., 2010).
Correlative methods, which establish empirical relationships between variables, are attractive for their simplicity, and are especially useful when long-term datasets of key predictor variables are available. Site-specific correlative models are often generated where a study focuses on multiple beaches (Table 1; e.g., Woolgar, 2012; Laloë et al., 2014; Bentley et al., 2020). This is necessary because variation in local climate factors and beach characteristics impact nest temperature, and site-specific data are required to generate equations with suitable predictive accuracy. Mechanistic models however, are more generalisable and can use coarse-resolution climate surfaces, including global surfaces, along with site-specific beach characteristics (e.g., slope, aspect, sand albedo) to predict sand and nest temperatures (Bentley et al., 2020). Mechanistic models are particularly useful for predicting nest temperature at remote nesting sites, such as turtle nesting beaches in Northern Australia, because many types of gridded climate surfaces cover these areas and can be used to force the model (Bentley et al., 2020). However, the use of coarse climate surfaces (e.g., global surfaces) often require site-specific calibration to provide more realistic simulations of local conditions (Bentley et al., 2020; Kearney et al., 2020). This is particularly relevant for island and coastal sites where beach temperatures are buffered by the surrounding ocean, as climate surfaces are not always corrected for the moderating influence of the ocean at the edge of continents or islands.
The dominant mechanistic model used to predict the microclimate of sea turtle nests was NicheMapR. This modeling platform (originally Niche Mapper TM) includes a microclimate subroutine designed to calculate ground surface microclimate conditions (such as temperature), based on information on the physical habitat and weather conditions 1–2 m above the ground (Kearney and Porter, 2017). NicheMapR has been used in sea turtle research to predict the sand temperatures at typical nest depths using macroscale climate data at nesting beaches, along with physical properties of the beach (e.g., slope and aspect) and the beach sand (e.g., albedo). Sand temperatures have been calculated for the portions of the year when eggs are incubating. By forcing the model with projected future air temperatures, rainfall, humidity, wind speeds, and cloud cover, sand temperatures many decades into the future can be predicted (e.g., Bentley et al., 2020). However, none of these mechanistic approaches have attempted to incorporate biological sources of heat, such as that from metabolic heat or microbial activity, into thermal models of nest chambers.
A number of mechanistic approaches to modeling animal development and growth exist, with one approach—Dynamic Energy Budget (DEB) theory—now applied to most species of sea turtles. DEB theory describes how organisms' partition energy fluxes into structure, maturation, and maintenance budgets from fertilization through to death, in response to two main drivers: body temperature and food (Kooijman, 2010). Several DEB models have been developed for sea turtles (Stubbs et al., 2014; Marn et al., 2017a,b), and there is potential for the heat flux equations in DEB theory to be used to predict individual nodes of heat for each embryo developing in a nest. This offers a route to solve the heat and energy balance of a nest chamber throughout incubation, via integration of DEB model and NicheMapR equations, which has already been achieved, but for different purposes (Kearney and Porter, 2019). Such an innovation should enable more accurate predictions of hatchling phenotypes, and could be used to explore how nest manipulations, such as dividing egg clutches in half, could reduce lethal heating of sea turtle nests in warmer climates.
The advantages and disadvantages of the available methods for modeling incubation conditions for sea turtle embryos lie in the way in which models are used (or abused). An attribute of mechanistic models is that they can test hypotheses about dominant interactions, and not simply demonstrate an ability to accurately simulate observed temperatures. For example, a hypothesis that sand temperatures are higher at lower latitude beaches was tested by modeling sand temperatures at 50 cm depth across more than 10° of latitude in Western Australia (Bentley et al., 2020). Mechanistic models also offer the potential to pose “what if” questions (Huey and Janzen, 2008) and can simulate microclimates over long timeframes to add value to short-term field observations. Disadvantages however, stem from their complexity and more exhaustive data requirements. Most mechanistic models require information on a range of sand/soil parameters (e.g., sand albedo, thermal conductivity, specific heat), which can be estimated from the literature or measured empirically. Surprisingly, models forced with parameters customized for a particular soil type do not necessarily produce better fits than models forced with generalized parameters (Carter et al., 2015). Further, structural errors in a model can lead to mismatches between modeled and observed results, particularly when the number of processes and the associated number of adjustable and uncertain model parameters increases (Kirchner, 2006). Most mechanistic models do not address model input uncertainty, model parameter uncertainty, nor assess model structural errors in a systematic manner (Montanari, 2007). The extent to which this is an issue depends upon the research question, but when applied to conservation management decisions, then honest accounting of model uncertainty is essential.
Conclusion
This systematic review captured all available peer-reviewed literature on metabolic heat in sea turtle nests, and included studies from the gray literature to limit publication bias. Our review emphasizes the need for a standard methodology to quantify metabolic heat, including the importance of positioning temperature loggers in consistent positions within the nest and in reference locations. Based on a subset of papers where methods for estimating metabolic heat were very similar, we showed that metabolic heat substantially increases nest temperatures in all seven species of sea turtle, and in many cases influenced embryonic development rate, sex determination, and survival. However, the biological impact of metabolic heat is heavily moderated by sand temperatures at typical nest depths, as well as by daily fluctuations in sand surface temperature. Accounting for metabolic heat and the many other factors discussed in this review will be necessary for evaluating the future viability of sea turtle populations, particularly as it will greatly improve our ability to predict embryonic mortality.
Data Availability Statement
All datasets generated for this study are included in the article/Supplementary Material.
Author Contributions
MG, NM, and SF conceived the questions addressed in the systematic review, and inclusion criteria were determined by MG who also screened and extracted relevant information from all papers. The first draft of the manuscript was written by MG, with all authors contributing to the final draft and approving it for submission. All authors contributed to the article and approved the submitted version.
Funding
This project was funded by the Department of Biodiversity, Conservation and Attractions, and the University of Western Australia.
Conflict of Interest
The authors declare that the research was conducted in the absence of any commercial or financial relationships that could be construed as a potential conflict of interest.
Acknowledgments
We thank Canaan Perry for helping to determine the literature review search string. The authors acknowledge the work and effort of all studies summarized in this review.
Supplementary Material
The Supplementary Material for this article can be found online at: https://www.frontiersin.org/articles/10.3389/fevo.2020.556379/full#supplementary-material
Footnotes
1. ^Available at: internationalseaturtlesociety.org
References
Abella Perez, E., Marco, A., Martins, S., and Hawkes, L. A. (2016). Is this what a climate change-resilient population of marine turtles looks like? Biol. Conserv. 193, 124–132. doi: 10.1016/j.biocon.2015.11.023
Ackerman, R. A. (1977). The respiratory gas exchange of sea turtle nests (Chelonia, Caretta). Respir. Physiol. 31, 19–38. doi: 10.1016/0034-5687(77)90062-7
Ackerman, R. A. (1997). “The nest environment and the embryonic development of sea turtles,” in The Biology of Sea Turtles, eds P. Lutz and J. Musick (Boca Raton, FL: CRC Press), 83–106.
Angilletta, M. J. (2009). Thermal Adaptation: A Theoretical and Empirical Synthesis. Oxford, England: Oxford University Press.
Barrientos-Muñoz, K., Ramirez-Gallego, C., Diez, C. E., and van Dam, R. P. (2012). “Metabolic warming in Hawksbill turtle nests at Mona Island, Puerto Rico,” in The Thirty-First Annual Symposium on Sea Turtle Biology and Conservation (San Diego, CA: NOAA Technical Memorandum NMFS-SEFSC-631).
Bates, D., Maechler, M., Bolker, B., and Walker, S. (2015). Fitting linear mixed-effects models using lme4. J. Stat. Softw. 67, 1–48. doi: 10.18637/jss.v067.i01
Bentley, B. P., Kearney, M. R., Whiting, S. D., and Mitchell, N. J. (2020). Microclimate modelling of beach sand temperatures reveals high spatial and temporal variation at sea turtle rookeries. J. Therm. Biol. 88:102522. doi: 10.1016/j.jtherbio.2020.102522
Binckley, C. A., Spotila, J. R., Wilson, K. S., and Paladino, F. V. (1998). Sex determination and sex ratios of Pacific leatherback turtles, Dermochelys coriacea. Copeia 1998, 291–300. doi: 10.2307/1447425
Bladow, R. A., and Milton, S. L. (2019). Embryonic mortality in green (Chelonia mydas) and loggerhead (Caretta caretta) sea turtle nests increases with cumulative exposure to elevated temperatures. J. Exp. Mar. Biol. Ecol. 518:151180. doi: 10.1016/j.jembe.2019.151180
Booth, D. T. (2017). Influence of incubation temperature on sea turtle hatchling quality. Integr. Zool. 12, 352–360. doi: 10.1111/1749-4877.12255
Booth, D. T., and Astill, K. (2001). Incubation temperature, energy expenditure and hatchling size in the green turtle (Chelonia mydas), a species with temperature-sensitive sex determination. Aust. J. Zool. 49, 389–396. doi: 10.1071/ZO01006
Booth, D. T., Burgess, E., McCosker, J., and Lanyon, J. M. (2004). The influence of incubation temperature on post-hatching fitness characteristics of turtles. Int. Congr. Ser. 1275, 226–233. doi: 10.1016/j.ics.2004.08.057
Booth, D. T., and Evans, A. (2011). Warm water and cool nests are best. How global warming might influence hatchling green turtle swimming performance. PloS ONE 6:e23162. doi: 10.1371/journal.pone.0023162
Broderick, A. C., Godley, B. J., and Hays, G. C. (2001). Metabolic heating and the prediction of sex ratios for green turtles (Chelonia mydas). Physiol. Biochem. Zool. 74, 161–170. doi: 10.1086/319661
Carr, A., and Hirth, H. (1961). Social facilitation in green turtle siblings. Anim. Behav. 9, 68–70. doi: 10.1016/0003-3472(61)90051-3
Carter, A., Kearney, M., Mitchell, N., Hartley, S., Porter, W., and Nelson, N. (2015). Modelling the soil microclimate: does the spatial or temporal resolution of input parameters matter? Front. Biogeogr. 7, 138–154. doi: 10.21425/F5FBG27849
Cavallo, C., Dempster, T., Kearney, M. R., Kelly, E., Booth, D., Hadden, K. M., et al. (2015). Predicting climate warming effects on green turtle hatchling viability and dispersal performance. Funct. Ecol. 29, 768–778. doi: 10.1111/1365-2435.12389
Chan, E., and Liew, H. (1995). Incubation temperatures and sex-ratios in the Malaysian leatherback turtle Dermochelys coriacea. Biol. Conserv. 74, 169–174. doi: 10.1016/0006-3207(95)00027-2
Chan, S., Liew, H. C., and Chan, E. H. (2006). “Thermal condition in nests of varying clutch sizes of the green turtle (Chelonia mydas) in Redang Island, Terengganu,” in Twenty-Third International Symposium on Sea Turtle Biology and Conservation (Kuala Lumpar: NOAA Technical Memorandum NMFS-SEFS C-536).
Charles, S., Fu, G., McFarlane, D., Hodgson, G., Teng, J., Barron, O., et al. (2015). Hydroclimate of the Pilbara: Past, Present and Future. A Report to the Government of Western Australia and Industry Partners From the CSIRO Pilbara Water Resource Assessment. CSIRO Land and Water, Australia.
Chen, C. L., Wang, C. C., and Cheng, I. J. (2010). Effects of biotic and abiotic factors on the oxygen content of green sea turtle nests during embryogenesis. J. Comp. Physiol. B Biochem. Syst. Environ. Physiol. 180, 1045–1055. doi: 10.1007/s00360-010-0479-5
Cheng, I. J., Lin, C.-H., and Tseng, C.-T. (2015). Factors influencing variations of oxygen content in nests of green sea turtles during egg incubation with a comparison of two nesting environments. J. Exp. Mar. Biol. Ecol. 471, 104–111. doi: 10.1016/j.jembe.2015.05.013
Deeming, D. C. (2004). Reptilian Incubation: Environment, Evolution and Behaviour. Nottingham: Nottingham University Press.
DeGregorio, B. A., and Williard, A. S. (2011). Incubation temperatures and metabolic heating of relocated and in situ loggerhead sea turtle (Caretta caretta) nests at a northern rookery. Chelonian Conserv. Biol. 10:54–61. doi: 10.2744/CCB-0880.1
Desvages, G., Girondot, M., and Pieau, C. (1993). Sensitive stages for the effects of temperature on gonadal aromatase activity in embryos of the marine turtle Dermochelys coriacea. Gen. Comp. Endocrinol. 92, 54–61. doi: 10.1006/gcen.1993.1142
Dormann, C. F., Schymanski, S. J., Cabral, J., Chuine, I., Graham, C., Hartig, F., et al. (2012). Correlation and process in species distribution models: bridging a dichotomy. J. Biogeogr. 39, 2119–2131. doi: 10.1111/j.1365-2699.2011.02659.x
Dudley, P. N., and Porter, W. P. (2014). Using empirical and mechanistic models to assess global warming threats to leatherback sea turtles. Mar. Ecol. Prog. Ser. 501, 265–278. doi: 10.3354/meps10665
Durmuş, S. H., Ilgaz, Ç., Güçlü, Ö., and Özdemir, A. (2011). The effect of the predicted air temperature change on incubation temperature, incubation duration, sex ratio and hatching success of loggerhead turtles. Anim. Biol. 61, 369–383. doi: 10.1163/157075511X596864
Dutton, D. L., Dutton, P. H., Chaloupka, M., and Boulon, R. H. (2005). Increase of a Caribbean leatherback turtle Dermochelys coriacea nesting population linked to long-term nest protection. Biol. Conserv. 126, 186–194. doi: 10.1016/j.biocon.2005.05.013
Esteban, N., Laloe, J. O., Mortimer, J. A., Guzman, A. N., and Hays, G. C. (2016). Male hatchling production in sea turtles from one of the world's largest marine protected areas, the chagos archipelago. Sci. Rep. 6:20339. doi: 10.1038/srep20339
Ewert, M., and Nelson, C. (2003). Metabolic heating of embryos and sex determination in the American alligator, Alligator mississippiensis. J. Therm. Biol. 28, 159–165. doi: 10.1016/S0306-4565(02)00053-0
Fuentes, M., Limpus, C., and Hamann, M. (2011). Vulnerability of sea turtle nesting grounds to climate change. Glob. Chang. Biol. 17, 140–153. doi: 10.1111/j.1365-2486.2010.02192.x
Fuentes, M., Maynard, J. A., Guinea, M., Bell, I. P., Werdell, P. J., and Hamann, M. (2009). Proxy indicators of sand temperature help project impacts of global warming on sea turtles in northern Australia. Endanger. Species Res. 9, 33–40. doi: 10.3354/esr00224
Fuentes, M. M. P. B., and Porter, W. P. (2013). Using a microclimate model to evaluate impacts of climate change on sea turtles. Ecol. Modell. 251, 150–157. doi: 10.1016/j.ecolmodel.2012.12.020
Furst, N., Baxter, A., Lorences Camargo, A., Sanchez Salazar, C. C., and Comer Santos, K. (2012). “Analysis of nest temperatures and future global warming effects on two coasta of Mexico,” in The Thirty-First Annual Symposium on Sea Turtle Biology and Conservation (San Diego, CA: NOAA Technical Memorandum NMFS-SEFSC-631).
García–Grajales, J., Hernando, J. M., García, J. A., and Fuentes, E. R. (2019). Incubation temperatures, sex ratio and hatching success of leatherback turtles (Dermochelys coriacea) in two protected hatcheries on the central Mexican coast of the Eastern Tropical Pacific Ocean. Anim. Biodivers. Conserv. 42, 143–152. doi: 10.32800/abc.2019.42.0143
Girondot, M., and Kaska, Y. (2015). Nest temperatures in a loggerhead nesting beach in Turkey is more determined by sea surface than air temperature. J. Therm. Biol. 47, 13–18. doi: 10.1016/j.jtherbio.2014.10.008
Glen, F., Broderick, A., Godley, B., and Hays, G. (2005). Patterns in the emergence of green (Chelonia mydas) and loggerhead (Caretta caretta) turtle hatchlings from their nests. Mar. Biol. 146, 1039–1049. doi: 10.1007/s00227-004-1492-6
Glen, F., and Mrosovsky, N. (2004). Antigua revisited: the impact of climate change on sand and nest temperatures at a hawksbill turtle (Eretmochelys imbricata) nesting beach. Glob. Chang. Biol. 10, 2036–2045. doi: 10.1111/j.1529-8817.2003.00865.x
Godfrey, M. H., Barreto, R., and Mrosovsky, N. (1997). Metabolically-generated heat of developing eggs and its potential effect on sex ratio of sea turtle hatchlings. J. Herpetol. 31, 616–619. doi: 10.2307/1565626
Godley, B., Broderick, A., Downie, J., Glen, F., Houghton, J., Kirkwood, I., et al. (2001). Thermal conditions in nests of loggerhead turtles: further evidence suggesting female skewed sex ratios of hatchling production in the Mediterranean. J. Exp. Mar. Biol. Ecol. 263, 45–63. doi: 10.1016/S0022-0981(01)00269-6
Godley, B., Broderick, A., Glen, F., and Hays, G. (2002). Temperature-dependent sex determination of Ascension Island green turtles. Mar. Ecol. Prog. Ser. 226, 115–124. doi: 10.3354/meps226115
Hanson, J., Wibbels, T., and Martin, R. E. (1998). Predicted female bias in sex ratios of hatchling loggerhead sea turtles from a Florida nesting beach. Can. J. Zool. 76, 1850–1861. doi: 10.1139/z98-118
Hawkes, L., Broderick, A., Godfrey, M., and Godley, B. (2007). Investigating the potential impacts of climate change on a marine turtle population. Glob. Chang. Biol. 13, 923–932. doi: 10.1111/j.1365-2486.2007.01320.x
Hawkes, L. A., Broderick, A. C., Godfrey, M. H., and Godley, B. J. (2009). Climate change and marine turtles. Endanger. Species Res. 7, 137–154. doi: 10.3354/esr00198
Hays, G., Ashworth, J., Barnsley, M., Broderick, A., Emery, D., Godley, B., et al. (2001). The importance of sand albedo for the thermal conditions on sea turtle nesting beaches. Oikos 93, 87–94. doi: 10.1034/j.1600-0706.2001.930109.x
Hays, G. C., Broderick, A. C., Glen, F., and Godley, B. J. (2003). Climate change and sea turtles: a 150-year reconstruction of incubation temperatures at a major marine turtle rookery. Glob. Chang. Biol. 9, 642–646. doi: 10.1046/j.1365-2486.2003.00606.x
Hays, G. C., Fossette, S., Katselidis, K. A., Schofield, G., and Gravenor, M. B. (2010). Breeding periodicity for male sea turtles, operational sex ratios, and implications in the face of climate change. Conserv. Biol. 24, 1636–1643. doi: 10.1111/j.1523-1739.2010.01531.x
Hays, G. C., Mazaris, A. D., and Schofield, G. (2014). Different male vs. female breeding periodicity helps mitigate offspring sex ratio skews in sea turtles. Front. Marine Sci. 1:43. doi: 10.3389/fmars.2014.00043
Hendrickson, J. R. (1958). The green sea turtle, Chelonia mydas (Linn.) in Malaya and Sarawak. Proc. Zool. Soc. Lon. 130, 455–535. doi: 10.1111/j.1096-3642.1958.tb00583.x
Houghton, J., and Hays, G. (2001). Asynchronous emergence by loggerhead turtle (Caretta caretta) hatchlings. Naturwissenschaften 88, 133–136. doi: 10.1007/s001140100212
Howard, R., Bell, I., and Pike, D. A. (2014). Thermal tolerances of sea turtle embryos: current understanding and future directions. Endanger. Species Res. 26, 75–86. doi: 10.3354/esr00636
Huey, R. B., and Janzen, F. J. (2008). Climate warming and environmental sex determination in tuatara: the last of the Sphenodontians? Proc. R. Soc. B Biol. Sci. 275, 2181–2183. doi: 10.1098/rspb.2008.0555
Jensen, M. P., Allen, C. D., Eguchi, T., Bell, I. P., LaCasella, E. L., Hilton, W. A., et al. (2018). Environmental warming and feminization of one of the largest sea turtle populations in the world. Curr. Biol. 28, 154–159.e154. doi: 10.1016/j.cub.2017.11.057
Jribi, I., and Bradai, M. N. (2014). Sex ratio estimations of loggerhead sea turtle hatchlings at Kuriat islands, Tunisia: can minor nesting sites contribute to compensate globally female-biased sex ratio? ScientificWorldJournal 2014:419410. doi: 10.1155/2014/419410
Kamel, S. J. (2013). Vegetation cover predicts temperature in nests of the hawksbill sea turtle: implications for beach management and offspring sex ratios. Endanger. Species Res. 20, 41–48. doi: 10.3354/esr00489
Kamel, S. J., and Mrosovsky, N. (2006). Deforestation: risk of sex ratio distortion in hawksbill sea turtles. Ecol. Appl. 16, 923–931. doi: 10.1890/1051-0761(2006)016[0923:DROSRD]2.0.CO;2
Kaska, Y., Downie, R., and Tippett, R. (1998a). “Intra-clutch temperature variations of Eastern Mediterranean loggerhead and green turtle nests,” in The Sixteenth Annual Symposium on Sea Turtle Biology and Conservation (Hilton Head, SC: NOAA Technical Memorandum NMFS-SEFSC-412).
Kaska, Y., Downie, R., Tippett, R., and Furness, R. W. (1998b). Natural temperature regimes for loggerhead and green turtle nests in the eastern Mediterranean. Can. J. Zool. Revue Can. De Zool. 76, 723–729. doi: 10.1139/z97-245
Kaska, Y., and Sezgin, C. (2019). “Evaluation of nest temperatures on Dalyan beach, Turkey,” in The Thirty-Sixth Annual Symposium on Sea Turtle Biology and Conservation (Lima: NOAA Technical Memorandum NOAA NMFS-SEFSC-734).
Katselidis, K. A., Schofield, G., Stamou, G., Dimopoulos, P., Pantis, J. D., and Katzner, T. (2012). Females first? Past, present and future variability in offspring sex ratio at a temperate sea turtle breeding area. Anim. Conserv. 15, 508–518. doi: 10.1111/j.1469-1795.2012.00543.x
Kearney, M. R., Gillingham, P. K., Bramer, I., Duffy, J. P., and Maclean, I. M. (2020). A method for computing hourly, historical, terrain-corrected microclimate anywhere on Earth. Methods Ecol. Evol. 11, 38–43. doi: 10.1111/2041-210X.13330
Kearney, M. R., Munns, S. L., Moore, D., Malishev, M., and Bull, C. M. (2018). Field tests of a general ectotherm niche model show how water can limit lizard activity and distribution. Ecol. Monogr. 88, 672–693. doi: 10.1002/ecm.1326
Kearney, M. R., and Porter, W. P. (2017). NicheMapR–an R package for biophysical modelling: the microclimate model. Ecography 40, 664–674. doi: 10.1111/ecog.02360
Kearney, M. R., and Porter, W. P. (2019). NicheMapR–an R package for biophysical modelling: the ectotherm and dynamic energy budget models. Ecography 43, 85–96. doi: 10.1111/ecog.04680
King, R., Cheng, W.-H., Tseng, C.-T., Chen, H., and Cheng, I. J. (2013). Estimating the sex ratio of green sea turtles (Chelonia mydas) in Taiwan by the nest temperature and histological methods. J. Exp. Mar. Biol. Ecol. 445, 140–147. doi: 10.1016/j.jembe.2013.03.016
Kirchner, J. W. (2006). Getting the right answers for the right reasons: linking measurements, analyses, and models to advance the science of hydrology. Water Resour. Res. 42:W03S04. doi: 10.1029/2005WR004362
Koch, A. U., Guinea, M. L., and Whiting, S. D. (2007). Effects of sand erosion and current harvest practices on incubation of the flatback sea turtle (Natator depressus). Aust. J. Zool. 55, 97–105. doi: 10.1071/ZO06063
Koch, A. U., Guinea, M. L., and Whiting, S. D. (2008). Asynchronous emergence of flatback seaturtles, Natator depressus, from a beach hatchery in northern Australia. J. Herpetol. 42, 1–9. doi: 10.1670/07-060.1
Kooijman, S. A. L. M. (2010). Dynamic Energy Budget Theory for Metabolic Organisation. Cambridge: Cambridge University Press.
Kornaraki, E., Matossian, D. A., Mazaris, A. D., Matsinos, Y. G., and Margaritoulis, D. (2006). Effectiveness of different conservation measures for loggerhead sea turtle (Caretta caretta) nests at Zakynthos Island, Greece. Biol. Conserv. 130, 324–330. doi: 10.1016/j.biocon.2005.12.027
Laloë, J.-O., Cozens, J., Renom, B., Taxonera, A., and Hays, G. C. (2014). Effects of rising temperature on the viability of an important sea turtle rookery. Nat. Clim. Change 4, 513–518. doi: 10.1038/nclimate2236
Laloë, J.-O., Esteban, N., Berkel, J., and Hays, G. C. (2016). Sand temperatures for nesting sea turtles in the Caribbean: Implications for hatchling sex ratios in the face of climate change. J. Exp. Mar. Biol. Ecol. 474, 92–99. doi: 10.1016/j.jembe.2015.09.015
Loop, K. A., Miller, J. D., and Limpus, C. J. (1995). Nesting by the Hawksbill turtle (Eretmochelys imbricata) on Milman Island, Great Barrier Reef, Australia. Wildlife Res. 22, 241–252. doi: 10.1071/WR9950241
Marco, A., Abella, E., Martins, S., López, O., and Patino-Martinez, J. (2017). Female nesting behaviour affects hatchling survival and sex ratio in the loggerhead sea turtle: implications for conservation programmes. Ethol. Ecol. Evol. 30, 141–155. doi: 10.1080/03949370.2017.1330291
Marn, N., Jusup, M., Legović, T., Kooijman, S., and Klanjšček, T. (2017a). Environmental effects on growth, reproduction, and life-history traits of loggerhead turtles. Ecological Modelling 360, 163–178. doi: 10.1016/j.ecolmodel.2017.07.001
Marn, N., Kooijman, S., Jusup, M., Legović, T., and Klanjšček, T. (2017b). Inferring physiological energetics of loggerhead turtle (Caretta caretta) from existing data using a general metabolic theory. Marine Environmental Research 126, 14–25. doi: 10.1016/j.marenvres.2017.01.003
Massey, M. D., Congdon, J. D., Davy, C., and Rollinson, N. (2019). First evidence of metabolic heating in a freshwater turtle (Chelydra serpentina). Chelonian Conserv. Biol. 18, 145–152. doi: 10.2744/CCB-1356.1
Mitchell, N. J., Allendorf, F. W., Keall, S. N., Daugherty, C. H., and Nelson, N. J. (2010). Demographic effects of temperature-dependent sex determination: will tuatara survive global warming? Glob. Chang. Biol. 16, 60–72. doi: 10.1111/j.1365-2486.2009.01964.x
Mitchell, N. J., Kearney, M. R., Nelson, N. J., and Porter, W. P. (2008). Predicting the fate of a living fossil: how will global warming affect sex determination and hatching phenology in tuatara? Proc. R. Soc. Lond. B Biol. Sci. 275, 2185–2193. doi: 10.1098/rspb.2008.0438
Mohanty-Hejmadi, P., and Dimond, M. T. (1986). Temperature dependent sex determination in the olive ridley turtle. Prog. Clin. Biol. Res. 217, 159–62.
Moher, D., Liberati, A., Tetzlaff, J., and Altman, D. G. (2009). Preferred reporting items for systematic reviews and meta-analyses: the PRISMA statement. Annals of Internal Medicine 151, 264–269.
Montanari, A. (2007). What do we mean by ‘uncertainty'? The need for a consistent wording about uncertainty assessment in hydrology. Hydrol. Process. Int. J. 21, 841–845. doi: 10.1002/hyp.6623
Montero, N., Dei Marcovaldi, M. A. G., Lopez-Mendilaharsu, M., Santos, A. S., Santos, A. J. B., and Fuentes, M. (2018). Warmer and wetter conditions will reduce offspring production of hawksbill turtles in Brazil under climate change. PLoS ONE 13:e0204188. doi: 10.1371/journal.pone.0204188
Montero, N., Tomillo, P. S., Saba, V. S., dei Marcovaldi, M. A., López-Mendilaharsu, M., Santos, A. S., et al. (2019). Effects of local climate on loggerhead hatchling production in Brazil: implications from climate change. Sci. Rep. 9:8861. doi: 10.1038/s41598-019-45366-x
Mota, M., and Carthy, R. (2004). “Modeling sea turtle clutch temperature and development based on air temperature and sand characteristics,” in The Twenty-Fourth Annual Symposium on Sea Turtle Biology and Conservation (San Jose: NOAA Technical Memorandum NMFS-SEFSC-567).
Mota, M., Dean, R., and Carthy, R. (2006). “Clutch temperature prediction: modelling sun, sand and sea turtle sex,” in The Twenty-Seventh Annual Symposium on Sea Turtle Biology and Conservation (Myrtle Beach, SC: International Sea Turtle Society).
Mrosovsky, N., and Yntema, C. L. (1980). Temperature dependence of sexual differentiation in sea turtles - implications for conservation practices. Iheringia. Série Zool. 18:271–280. doi: 10.1016/0006-3207(80)90003-8
Mueller, M. S., Ruiz-García, N. A., García-Gasca, A., and Abreu-Grobois, F. A. (2019). Best swimmers hatch from intermediate temperatures: effect of incubation temperature on swimming performance of olive ridley sea turtle hatchlings. J. Exp. Mar. Biol. Ecol. 519:151186. doi: 10.1016/j.jembe.2019.151186
Neville, A., Webster, D., Gouveia, J. F., Hendricks, E. L., Hendricks, I., Marvin, G., et al. (1998). “The effects of nest temperature on hatchling emergence in the loggerhead sea turtle (Caretta caretta),” in The Eighth Annual Workshop on Sea Turtle Conservation and Biology (Fort Fisher, NC: NOAA Technical Memorandum NMFS-SEFC-214).
Önder, B. F., and Candan, O. (2016). The feminizing effect of metabolic heating in Green Turtle (Chelonia mydas) clutches in the eastern Mediterranean. Zool. Middle East 62, 239–246. doi: 10.1080/09397140.2016.1202927
Patino-Martinez, J., Marco, A., Quiñones, L., and Hawkes, L. (2012). A potential tool to mitigate the impacts of climate change to the caribbean leatherback sea turtle. Glob. Change Biol. 18, 401–411. doi: 10.1111/j.1365-2486.2011.02532.x
Patrício, A. R., Marques, A., Barbosa, C., Broderick, A. C., Godley, B. J., Hawkes, L. A., et al. (2017). Balanced primary sex ratios and resilience to climate change in a major sea turtle population. Mar. Ecol. Prog. Ser. 577, 189–203. doi: 10.3354/meps,12242
R Core Team (2019). R: A Language and Environment for Statistical Computing. Vienna: R Foundation for Statistical Computing.
Rivas, M. L., Esteban, N., and Marco, A. (2019). Potential male leatherback hatchlings exhibit higher fitness which might balance sea turtle sex ratios in the face of climate change. Clim. Change 156, 1–14. doi: 10.1007/s10584-019-02462-1
Sandoval, S., Gomez-Munoz, V., Gutiérrez, J., and Porta-Gándara, M. Á. (2011). Metabolic heat estimation of the sea turtle Lepidochelys olivacea embryos. J. Therm. Biol. 36, 138–141. doi: 10.1016/j.jtherbio.2010.12.007
Santidrián Tomillo, P., Fonseca, L., Paladino, F. V., Spotila, J. R., and Oro, D. (2017). Are thermal barriers “higher” in deep sea turtle nests? PLoS ONE 12:e0177256. doi: 10.1371/journal.pone.0177256
Santidrian Tomillo, P., Genovart, M., Paladino, F. V., Spotila, J. R., and Oro, D. (2015). Climate change overruns resilience conferred by temperature-dependent sex determination in sea turtles and threatens their survival. Glob. Chang. Biol. 21, 2980–2988. doi: 10.1111/gcb.12918
Santidrián Tomillo, P., Oro, D., Paladino, F. V., Piedra, R., Sieg, A. E., and Spotila, J. R. (2014). High beach temperatures increased female-biased primary sex ratios but reduced output of female hatchlings in the leatherback turtle. Biol. Conserv. 176, 71–79. doi: 10.1016/j.biocon.2014.05.011
Sari, F., and Kaska, Y. (2016). Histochemical and immunohistochemical studies of the gonads and paramesonephric ducts of male and female hatchlings of loggerhead sea turtles (Caretta caretta). Biotech. Histochem. 91, 428–437. doi: 10.1080/10520295.2016.1201143
Sönmez, B. (2018). Relationship between metabolic heating and nest parameters in green turtles (Chelonia mydas, L. 1758) on Samandag Beach, Turkey. Zool. Sci. 35, 243–248. doi: 10.2108/zs180003
Speakman, J., Hays, G., and Lindblad, E. (1998). Thermal conductivity of sand and its effect on the temperature of loggerhead sea turtle (Caretta caretta) nests. J. Mar. Biol. Assoc. UK. 78, 1337–1352. doi: 10.1017/S0025315400044532
Standora, E., Morreale, S., Ruiz, G., and Spotila, J. (1982). Sex determination in green turtle (Chelonia mydas) hatchlings can be influenced by egg position within the nest. Bull. Ecol. Soc. Am. 62, 83–84.
Stubbs, J. L., Kearney, M. R., Whiting, S. D., and Mitchell, N. J. (2014). Models of primary sex ratios at a major flatback turtle rookery show an anomalous masculinising trend. Clim. Change Responses 1:3. doi: 10.1186/s40665-014-0003-3
Tanner, C. E., Marco, A., Martins, S., Abella-Perez, E., and Hawkes, L. A. (2019). Highly feminised sex-ratio estimations for the world's third-largest nesting aggregation of loggerhead sea turtles. Mar. Ecol. Prog. Ser. 621, 209–219. doi: 10.3354/meps12963
Uçar, A. H., Kaska, Y., Ergene, S., Aymak, C., Kaçar, Y., Kaska, A., et al. (2013). Sex ratio estimation of the most eastern main loggerhead sea turtle nesting site: Anamur Beach, Mersin, Turkey. Isr. J. Ecol. Evol. 58:87–100. doi: 10.1560/IJEE.58.1.87
Valverde, R. A., Wingard, S., Gómez, F., Tordoir, M. T., and Orrego, C. M. (2010). Field lethal incubation temperature of olive ridley sea turtle Lepidochelys olivacea embryos at a mass nesting rookery. Endanger. Species Res. 12, 77–86. doi: 10.3354/esr00296
Vázquez Luna, G., Sanchez Trejo, R., Márquez Millan, R., and Castro Melendez, R. (2000). “Temporal and spatial variation of the hatching temperature in transplanted Lepidochelys kempi nestings and their influence on teh sex ratio, egg survival and mortality,” in The Eighteenth Internation Sea Turtle Symposium (Mazatlán: NOAA Technical Memorandum NMFS-SEFSC-436).
Wibbels, T. (2003). “Critical approaches to sex determination in sea turtles,” in The biology of sea turtles, Vol. III (Boca Raton, FL: CRC Press), 103–134.
Woolgar, L. (2012). Successful Microclimate Modelling of Beach sand Temperatures Reveals High Spatial and Temporal Variation at Sea Turtle Rookeries. Master of Science, The University of Western Australia, Perth, Australia.
Woolgar, L., Trocini, S., and Mitchell, N. (2013). Key parameters describing temperature-dependent sex determination in the southernmost population of loggerhead sea turtles. J. Exp. Mar. Biol. Ecol. 449, 77–84. doi: 10.1016/j.jembe.2013.09.001
Zbinden, J. A., Davey, C., Margaritoulis, D., and Arlettaz, R. (2007). Large spatial variation and female bias in the estimated sex ratio of loggerhead sea turtle hatchlings of a Mediterranean rookery. Endanger. Species Res. 3, 305–312. doi: 10.3354/esr00058
Zbinden, J. A., Margaritoulis, D., and Arlettaz, R. (2006). Metabolic heating in Mediterranean loggerhead sea turtle clutches. J. Exp. Mar. Biol. Ecol. 334, 151–157. doi: 10.1016/j.jembe.2006.01.021
Keywords: metabolic heat, sea turtle, climate change, modeling, temperature, systematic review
Citation: Gammon M, Fossette S, McGrath G and Mitchell N (2020) A Systematic Review of Metabolic Heat in Sea Turtle Nests and Methods to Model Its Impact on Hatching Success. Front. Ecol. Evol. 8:556379. doi: 10.3389/fevo.2020.556379
Received: 28 April 2020; Accepted: 12 August 2020;
Published: 17 September 2020.
Edited by:
Matthew H. Godfrey, Nicholas School of the Environment, United StatesReviewed by:
Pilar Santidrián Tomillo, The Leatherback Trust, Costa RicaJeanine M. Refsnider, University of Toledo, United States
Brett DeGregorio, United States Geological Survey (USGS), United States
Copyright © 2020 Gammon, Fossette, McGrath and Mitchell. This is an open-access article distributed under the terms of the Creative Commons Attribution License (CC BY). The use, distribution or reproduction in other forums is permitted, provided the original author(s) and the copyright owner(s) are credited and that the original publication in this journal is cited, in accordance with accepted academic practice. No use, distribution or reproduction is permitted which does not comply with these terms.
*Correspondence: Malindi Gammon, bWFsaW5kaS5nYW1tb25AcmVzZWFyY2gudXdhLmVkdS5hdQ==