- 1Evolutionary Ecology, Museo Nacional de Ciencias Naturales MNCN-CSIC, Madrid, Spain
- 2Plants and Ecosystems, Department of Biology, University of Antwerp, Antwerp, Belgium
- 3Departamento de Geologia, Museo Nacional de Ciencias Naturales, MNCN-CSIC, Madrid, Spain
The presence of nestlings and other nest dwelling organisms in cavity nests alters the composition of gasses inside the cavity. Differential concentrations of gasses could be used by some parasites as a cue to localize their hosts. Here, we explored temporal variation in the concentration and isotopic signature of carbon dioxide (CO2) and methane (CH4) inside nest boxes of blue tits (Cyanistes caeruleus) during the nestling period (on days 3, 8, 13, 20, and 21 post-hatching) as well as several variables potentially affecting such variation. Finally, we checked whether differences in gas concentrations affect the abundance of different types of parasites affecting nestlings. Gas concentration and isotopic signature were significantly different between nest boxes and the forest during the nestling period. The CO2 concentration was higher inside nests than in the forest air, whereas CH4 concentration was lower. We expected to observe a positive correlation between the abundance of parasites actively seeking nests (i.e., blackflies, biting midges, and blowflies) and differences in gas concentration for those species that use these differences as a cue for host location. We observed that biting midge abundance was positively related to differences in CO2 between nest and forest air at day 20 of nestling age, indicating that this species can use these differences to locate hosts. We also found a positive relationship between blackfly abundance and differences in CH4 concentration. However, we hypothesize that the concentration of this gas inside nests may be related with bacterial activity; therefore, this relationship may be due to an effect of bacteria on blackflies and not to the effect of CH4 as an attraction cue for blackflies.
Introduction
Several invertebrate species act as hosts and vectors for different pathogens (Marquardt et al., 2000). Vector borne diseases are usually more virulent that those transmitted by direct contact (Ewald, 1994) and mosquitoes are considered the deadliest animals in the world due to the diseases they transmit (GatesNotes, 2014). A pathogen’s necessity to reach both a host and a vector to complete its life cycle is obviously a challenge. However, evolution promotes important adaptations in pathogens to solve these challenges. Selection for such adaptations is facilitated by the advantages that flying vectors can provide such as increased dispersion, which can then lead to a significant increase in the pathogen’s fitness. In this respect, the pathogen’s successful transmission directly depends on the success of the vector to locate its host. What cues do mosquitoes and other vectors use to locate their hosts? Several studies have investigated mechanisms used by insects and other invertebrate vectors to locate their hosts. For instance, morphological studies of the sense organs of blood sucking insects have shown the kinds of substances that could act as host-specific attraction cues and have indicated that the gasses produced by host respiration may be potential cues for biting flying vectors (reviewed in Lehane, 2005). However, very little is known on the potential use of host-emitted gasses as an attraction cue for vectors in wild animal populations.
It is well known that mosquitoes, biting flies and ticks can use even slight fluctuations of CO2 as a directional cue to find their hosts (Bogner, 1992; Grant et al., 1995; Mboera and Takken, 1997; Takken and Knols, 1999). In fact, Wright and Kellogg (1962) suggested that mosquitos moved to a CO2 source specifically due to a change in CO2 concentration. Grant and Kline (2003) showed that female mosquitoes, and many other biting insects, are equipped with an array of sensors (chemoreceptor neurons) that are highly sensitive to different concentrations of CO2. Therefore, a higher CO2 fluctuation is probably the main stimulus for mosquitoes to approach their hosts (McPhatter and Gerry, 2017).
Several studies in birds have measured the concentration of CO2 during the incubation period (Lamson and Edmond, 1914; Wangensteen et al., 1971; Walsberg, 1980), however, few have investigated the effect of gas composition on vector attraction during nestling development. For example, White et al. (1978) found a higher concentration of CO2 in nest chambers of the European bee-eater (Merops apiaster) compared with outside air during the nestling period. In other study, Mondain-Monval and Sharp (2018) found a higher concentration of CO2 in occupied Sand Martin (Riparia riparia) burrows than in unoccupied ones during the nestling period. However, neither of these works explored the potential effect of these changes on vector attraction. In terms of other gasses, methane (CH4) production by domestic and wild animals, including arthropods and insects, has been studied by many authors (see e.g., Crutzen et al., 1986). However, to our knowledge, none have examined the relationship between gasses (e.g., CO2 and CH4) produced in the nest during the nesting period and parasite abundance. In fact, the effects of CH4 on birds and parasites in nests are completely unknown. In order to determine whether CH4 concentration in nests is involved in parasite attraction, it is necessary to study the evolution of this parameter during the nestling period.
Geochemical tracing using the stable carbon isotopic signature δ13C is a very useful tool for understanding biogeochemical processes in ecosystems (Peterson and Fry, 1987). δ13C is the molecular ratio between the heavy and light carbon isotopes 13C and 12C, respectively (Earth’s average relative concentration: 12C, 98.9% and 13C, 1.1%). This ratio allows for the identification of multiple carbon-producing (and removing) sources and mechanisms of CO2 and CH4 transference in natural ecosystems (Cerling et al., 1991; Yakir and Sternberg, 2000; Maseyk et al., 2009; Garcia-Anton et al., 2017). Processes that remove or produce CO2 and CH4 are characterized by a process-specific fractionation of carbon isotopes, owing to the isotope-dependent rates of the chemical reactions involved. As a result, the isotopic signature of CO2 and CH4 reflects the relative contribution of these processes. In a nest environment, variations in the concentration of CO2 and CH4 may be directly related to the metabolic activity of birds and the microbial activity in the nest. For instance, methane oxidation by methane-oxidizing bacteria (MOB) preferentially removes 12CH4 and, therefore, increases δ13C -CH4 (Fernandez-Cortes et al., 2015). To our knowledge, CH4 concentration has not, until now, been analyzed throughout the entire nestling period in any cavity-nesting bird. By measuring the concentration and isotopic signature of CH4 in nests during this period, we can determine the cause of these variations and whether they are related to the presence and abundance of parasites in the nests.
The microclimate inside a nest cavity may vary considerably between nests due to differences in nest material composition and the level of bird activity (Mainwaring, 2017; Maziarz, 2019). Nestling development also affects the microclimate by adding different wastes (i.e., feces and feather debris) to nests, leading to increased heat and changes in relative humidity by evapotranspiration, which could both affect the gas composition inside nests. All these changes may affect the attraction and development of parasites and other arthropods inside nests. Here, we assess the effect of different variables (brood size, hatching date, nestling age, humidity, and temperature) on the concentration of gasses inside nest boxes and evaluate differences in gas concentrations between nest boxes and the forest air. We expect that nests with larger broods and older nestlings to show higher concentration differences. We also examine whether some ectoparasites (fleas, blowflies, mites, biting midges, and blackflies) potentially use CO2 and CH4 concentration differences as attraction cues. Finally, we determine whether ectoparasite abundance is positively correlated with gas concentration differences between nest boxes and forest air at any nestling age.
Materials and Methods
Study Population
The study was conducted during the 2016 bird breeding season in a Pyrenean Oak (Quercus pyrenaica) deciduous forest located within a protected area of Sierra de Guadarrama National Park (Montes de Valsaín) near Valsaín (Segovia, central Spain, 40° 53′ 74 N, 4° 01′ W, 1,200 m.a.s.l). The geographic area of Valsain (Segovia) is characterized by a Mediterranean climate, a subtype of a warm temperate climate with a dry warm summer and a cool mild winter (Csb climate type, Köppen–Geiger Classification slightly modified, AEMET-IM, 2011). Annual mean temperature in 2016 at the study area was 10.2°C, and total annual precipitation was 556 mm1. A blue tit C. caeruleus population breeding in wooden nest boxes has been studied in this area since 1991 (Sanz, 2002; Tomás et al., 2006). Nest boxes were 17.5 cm high, 11.7 cm wide, and 12.5 cm deep. The entrance hole of each box was 4.5 cm in diameter, and for airing the nest, there was a small uncovered hollow space (11.7 cm × 1.3 cm) located just under the nest-box roof. Total nest volume was 2,559 cm3. A plastic tube with a diameter of 5 cm was attached to the outside of the entrance hole to prevent nestling predation by genets. The tubes likely reduce gas exchange with the outside air when birds enter or exit the nests because of the limited space around the bird’s body and the fact that they are forced to move slowly while in the tube. Each breeding season, nest boxes are periodically inspected to determine reproductive parameters such as laying date, clutch size and hatching date (Merino et al., 2000; Tomás et al., 2007). The present study is considered observational work as it only involves ringing and measuring the birds. Ringing permits were granted by the Directorate General of the Natural Environment and the Junta of Castille and León (permit numbers: EP/SG/705/2015 and PNSG_SG_2015_0323). No other animal use permits were required.
Measuring Gasses
Between 24 May and 20 June 2016, we monitored and sampled 44 nest boxes occupied by blue tits. First, the ecosystem was characterized from both a climatic (meteorological data during the 2016 monitoring period see footnote 1) and a geochemical perspective (CO2 and CH4 concentration and δ13C-CO2 and δ13C-CH4 from oak forest air). For this purpose, we measured the atmospheric air, soil air and air inside nest boxes during the nesting period at a specific location in the middle of the study area. Four samples of each soil and forest air were taken each sampling day and used to calculate the average measurement for that day. In addition, a total of 148 samples of air from inside nest boxes were taken during the nestling period at different nestling ages (44 samples, one from each nest at 3, 8, and 13 days post-hatching, and 16 samples at 20 days post-hatching as nestlings had already fledged from the other 28 nests). The air inside four empty nest boxes was also sampled prior to their occupation (one box had a nest but no eggs or nestlings and the other three were completely empty). These samples were collected on May 18 (7:00–9:00 AM) in order to have a reference value for the concentration of gasses in empty nest boxes. Once the nestlings fledged (20 or 21 days post-hatching), a final sample was taken from the 44 nest boxes. Nest boxes were hanging from tree branches about 5 m above the ground. For gas collection, nest boxes were first carefully taken down from the tree branches and placed on the ground. Gasses were extracted through the entrance hole of the nest boxes. After gas collection, nest boxes were opened and the number of nestlings counted. Then, nest boxes were closed and returned to the same position on the tree branch until the next sampling. Sampling of gasses was always conducted between 7:00 and 10:00 AM for forest air and soil and between 8:30 AM and 16:30 PM for nest-box air. Sampling time had no effect on the results in our analyses.
For the extraction of gasses from nest boxes and soil, we used a micro-diagraph gas pump (NMP-830-KNDC-12V; KNF, Germany) at 1.5 l min–1 at atmospheric pressure. Gas extraction was carried out slowly and for the same duration (20 s) in order to obtain approximately 0.5 L of air from each nest box. Similarly, atmospheric forest air was collected using a battery operated portable air pump (Aquanic S790, 0.010 Mpa 0.4 l/min Battery 1.5V; ICA, S.A, Spain). Grab air samples were collected through a tube connected directly to the pump, while another tube expelled the air from the pump and stored it in a 1-L Tedlar® gas sampling bag (Supelco; United States). The bags were sealed and subsequently analyzed in the laboratory within 24 h. The same sampling method and pump were used in other studies (e.g., Garcia-Anton et al., 2014, 2017; Fernandez-Cortes et al., 2015, 2018). CO2 and CH4 molar fractions and stable isotope ratios of carbon in both gasses were measured with a G2201-i analyser (Picarro Inc., United States) that used cavity ring-down spectroscopy (CRDS) technology to identify and quantify the compounds contained in the air being analyzed (Crosson, 2008). Stable isotope ratios of carbon are reported in standard δ-notation with units of ‰ (δ13C). The CRDS analyser measures the molar fractions and δ13C with a dynamic range of 100 to 4,000 ppmv for CO2 and 0 to 1,000 ppmv for CH4 and a precision greater than 0.16‰ for δ13C-CO2 and 1.15‰ for δ13C-CH4.
Measuring Temperature and Relative Humidity
Environmental conditions (i.e., temperature and relative humidity) were recorded inside and outside nests boxes during the nestling period (days 3, 8, 13, and 20 post-hatching). Sensors that register both variables were fitted to the inner wall of the nest box just below the rim (iButton Hygrochron Temperature/Humidity Logger with 8KB Data-Log Memory DS 1923; 6 mm × 17 mm, temperature range: −20–85°C; resolution 0.0625°C; humidity range: 0–100% with a resolution 0.04%; Maxim Integrated, CA, United States). Temperature and relative humidity were recorded every 45 min during the nesting period. Similarly, four external sensors (HOBO U23-001; data logger; 10.2 cm × 3.8 cm, temperature range: −40–70°C; resolution 0.02°C; humidity range: 0–100% with a resolution 0.03%; Onset Data Loggers, Massachusetts, United States) were located underneath empty nest boxes to register temperature and relative humidity in the study area during the nesting period.
Quantifying Nest Ectoparasites
Several biting flying insects and nest-dwelling ectoparasites, many acting as vectors for blood parasites, are commonly found in this bird population. Biting midges (Culicoides spp.) are very small dipterans whose females need bird blood to lay eggs (although some autogenous species can lay the first batch of eggs without a blood feed). Biting midges are vectors of haemosporidian parasites of the genus Haemoproteus (Valkiunas, 2005; Martínez-de la Puente et al., 2011), which are known to have detrimental effects on bird reproduction and survival (Merino et al., 2000; Martínez–de la Puente et al., 2010). Blue tit nests are also visited by blackflies (Diptera: Simuliidae) looking for bird blood to complete their life cycle. Blackflies are vectors of another common blood parasite of birds, Leucocytozoon spp. (Order Haemosporida), which cause chronic diseases in infected birds (Merino et al., 2000; Martínez–de la Puente et al., 2010). Mosquitoes are scarcely found in the bird nests of our population; however, the transmission of Plasmodium species to blue tits in this area has been previously reported (Martínez-de la Puente et al., 2011).
Nest-dwelling parasites typically found in blue tit nests in Valsain include a dipteran flying insect, the blowfly Protocalliphora azurea. Only blowfly larvae feed on blood and their role as vectors is unclear. Fleas (Ceratophyllus gallinae) are also common in nests. The presence of adult fleas is mainly evidenced by the presence of their larvae as adults typically remain attached to the birds or easily escape when the nest is collected to quantify parasites (see below). Only adult fleas feed on blood, and it is unclear whether they are vectors of any diseases in blue tits. Haematophagous mites (Dermanyssus spp.), which can act as vectors for trypanosomes (Macfie and Thomson, 1929), also attack blue tits. Both fleas and mites reach new nests by attaching to adult birds as they inspect other cavities. Mites may also reach nests by phoresy on midges and blackflies (Marshall, 1981), although this is unlikely their main means of transport.
Biting midges and blackflies parasitizing nests were collected by using traps located inside the nest boxes (see Tomás et al., 2008). The traps consisted of a plastic petri dish (8.5 cm in diameter; 55.67 cm2) containing a commercially available baby oil gel (Johnson’s Baby Oil Gel with Camomile; Johnson & Johnson, Dusseldorf, Germany). The petri dishes were placed within nest boxes at day 10 post-hatching and retrieved at day 13. Afterward, petri dishes were observed under a magnifying glass and the number of biting midges and blackflies that adhered to the gel were counted. Once nestlings fledged (20 or 21 days post-hatching), the sensors were removed, and the nest material was collected in a sealed labeled plastic bag and transported to the laboratory to quantify ectoparasite abundance. Nests were stored at 4°C for 2–4 days prior to ectoparasite extraction using Berlese funnels. Nests were kept in funnels for 48 h under constant temperature and light conditions provided by a lamp placed above the nests (see Tomás et al., 2007). Small nest ectoparasites (mites and fleas) were collected in vials containing 70% ethanol and examined under a stereomicroscope (OLYMPUS-SZX7; ACH1x, Tokyo, Japan; see Merino and Potti, 1995). Their abundance was estimated from this material. The nest material was then manually searched for blowfly pupae.
We also evaluated the relationship between ectoparasite abundance and bird body condition. For this, 75 blue tit adults (37 males and 38 females) were captured at 13 days post-hatching with traps mounted in the nest boxes. Adults were measured and, when necessary, ringed with numbered aluminum rings. Nestlings were also measured and ringed at 13 days post-hatching. Adult and nestling body mass was measured with an electronic balance (±0.1 g). Tarsus length was measured with a digital caliper (±0.1 mm), and wing length with a ruler (±0.5 mm). The body condition index in adults and nestlings was calculated as the residuals of body mass on tarsus length.
Statistical Analyses
Our first aim was to select a number of potential explanatory variables for the variation in gas concentration in nests. We hypothesized that the number of nestlings (brood size) and nestling age would significantly affect gas concentration (i.e., the greater the nestling number or the larger the nestlings, the greater the CO2 concentration). The time in the season as measured by hatching date may also influence gas concentration, although this variable could be correlated with brood size. Temperature and relative humidity could influence gas concentration, for example, by favoring dilution effects. However, these two variables have also been shown to be negatively correlated with each other (see e.g., Castaño-Vázquez et al., 2018). We checked the variance inflation factor (VIF) for these variables (brood size, hatching date, temperature and relative humidity) in multiple correlation analyses with each of the gas concentration variables. VIFs estimate how much the variance of a coefficient is “inflated” because of linear dependence with other predictors. In all cases, the VIF values were low (less than 2). Thus, we included all of these variables in a mixed model analysis with gas concentration (CO2/δ13C-CO2 or CH4/δ13C-CH4 measured both inside nest boxes and in the forest) as the dependent variable (variables were log transformed in order to comply with normality assumptions), measurement location (inside nest box or forest) and nestling age as fixed factors and brood size, hatching date, temperature and relative humidity as covariables. The interaction of measurement location × nestling age was included as a repeated measures effect on nests. Then, we conducted a backward stepwise procedure to reduce the model to the significant variables.
To explore the relationship between gas concentration and ectoparasite abundance, we used generalized linear models (GzLM) with a negative binomial distribution and log link function. The number of each parasite type (flea larvae, mites, blowfly pupae, biting midges, and blackflies) was used as the dependent variable with the following independent variables: the differences in the concentration of CO2 and CH4 (i.e., the concentration inside the nest box less the concentration in the forest air), the differences in relative humidity inside nest boxes and in the forest air and brood size. We conducted one analysis for each day the gasses were measured, thus analyzing the relationship between the abundance of each parasite and the difference in gas concentration at the different ages independently. We assumed that the abundance of the different parasites measured at the end of the nestling period was directly related to their abundance at other stages of nestling development (see e.g., Dube et al., 2018). We selected gas concentration differences between nest boxes and forest air to reduce the number of variables in the model and because it could be the cue used by some ectoparasites to locate potential hosts. We expected that a lack of clear gas concentration differences between forest and nest boxes would result in a lack of signal for parasites; however, if concentration differences were observed from hatching to fledging stages, then we assumed that there was a potential attraction cue for ectoparasites. We first used an Omnibus test to check that the analysis could be performed. Omnibus tests assess the significant of the overall model by calculating whether explained variance is significantly higher than unexplained variance. A positive Omnibus test suggested that the model fitted the data. In this case, at least one independent variable was significant and the other independent variables could be included in the model under the assumption of non-colinearity between independent variables. Conversely, a negative test suggested that the model was not sufficient to determine model fit for the predictors and, therefore, the analysis could not be performed. The Omnibus test used to compare the current versus the null (in this case, intercept) model was a likelihood-ratio chi-square test. Only when this test was significant did we explored the effect of the significant independent variables. Models were simplified by backward stepwise elimination of those variables with the highest P-value. Thus, only significant variables remained in the final models.
In addition, we used multiple linear regressions to test the relationship between parasite abundance and body condition of adult and nestling blue tits. Statistical analyses were performed in STATISTICA 72 and SPSS (IBM Corp. Released 2017. IBM SPSS Statistics for Windows, Version 25.0. Armonk, NY, United States: IBM Corp).
Data Deposition
Data are available at the Digital CSIC repository.
Results
Concentration of Gasses in the Ecosystem
During the nesting period (24 May–20 June), the study area experienced eight rainy days with a total rainfall of 39.8 mm, and the average temperature was 13.7°C (Table 1). The mean concentration of CO2 of the forest air ranged from 411 to 547 ppm, and the isotopic signal (δ13CO2) from −8.56 to −12.21‰. The highest CO2 values for forest air were recorded on the warmest days due to increased flow from the soil: during drier periods, soil humidity decreases, which induces increased gas diffusivity (Werner et al., 2006; Garcia-Anton et al., 2017). CO2 mean values from soil air ranged from 887 to 15,025 ppm, and the isotopic signature (δ13CO2) varied from −16.5 to −22.4‰. These data are consistent with the local climate and C3 vegetation. The CO2/δ13C-CO2 results of the forest and soil air sample analyses were studied using the Keeling approach. This method is widely used to characterize the δ13C-CO2 of ecosystem respiration (Garcia-Anton et al., 2014). The intercept value of the Keeling plot (δ13Cs) for the samples collected over the monitoring period was −22.77‰, indicating a prevalence of C3 plant activity in the oak woodland study area (C3 plant activity reference value is around −27‰, Amundson et al., 1998), plus a 4.4‰ diffusional enrichment from soil (Yakir and Sternberg, 2000).
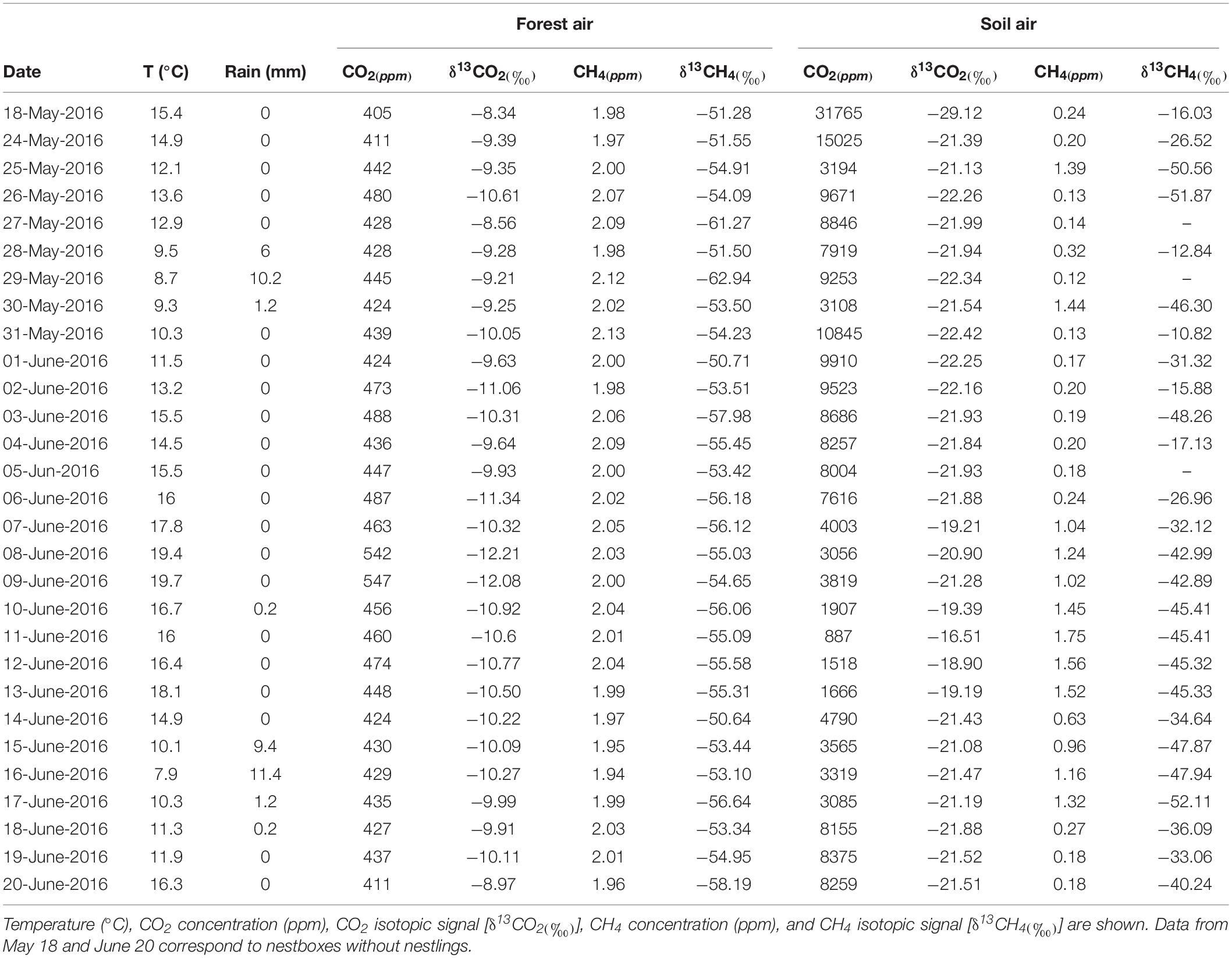
Table 1. Average data of environmental conditions of the study area (Valsain; Segovia, central Spain, 40° 53′ 74 N, 4° 01′ W, 1,200 m a.s.l) during blue tit nesting period.
Gas Concentrations, Relative Humidity, Age and Brood Size
At the start of the sampling period (May 18, 2016), we did not observe any significant difference in the concentration of CO2 between the air inside empty nest boxes (without eggs or nestlings) and the forest air (unpaired t-test: t = 1.27, df = 5, p = 0.259; Tables 1, 2). However, there was a significant difference in their isotopic signal, indicating that nest air was isotopically lighter than forest air [δ13CO2(‰); unpaired t-test: t = −2.90, df = 5, p = 0.033; Tables 1, 2]. CH4 concentration was significantly lower inside the empty boxes compared with the forest air (unpaired t-test: t = −5.73, df = 5, p = 0.002); however, no significant difference was observed in the CH4 isotopic signal [δ13CH4(‰); unpaired t-test: t = 2.27, df = 5, p = 0.072; Tables 1, 2].
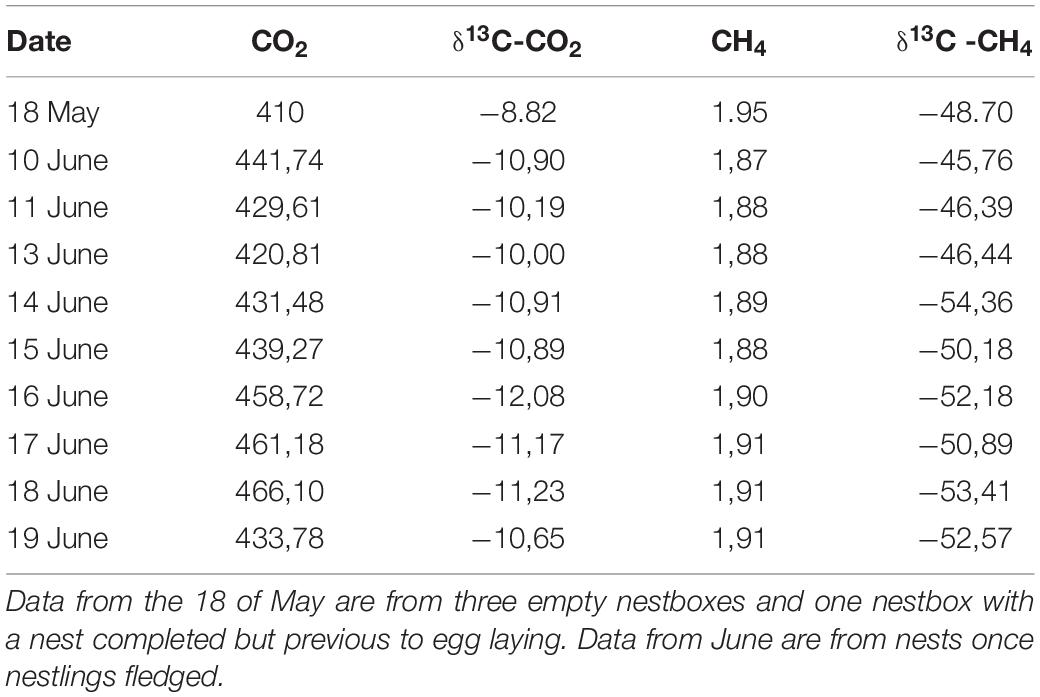
Table 2. Average values of concentration (ppm) and isotopic signals (‰) of CO2 and CH4 from nest-boxes without nestlings.
CO2 concentration was higher inside nest boxes than in the forest air during the nesting period (ANOVA mixed model, F1,250.96 = 219.10, p < 0.0001); this difference was maintained at all nestling ages (ANOVA mixed model, measurement location × nestling age interaction: F3,259.65 = 21.34, p < 0.0001, Figure 1). Moreover, CO2 concentration inside nests differed significantly according to nestling age with the highest concentrations observed at 8 and 20 days of age and the lowest at 3 and 13 days of age (ANOVA mixed model, F3,250.77 = 30.12, p < 0.0001; Figure 1). Likewise, CO2 concentration was positively and significantly related to relative humidity (ANOVA mixed model, F1,263.32 = 13.71, p < 0.0001) and to brood size (F1,57.65 = 4.51, p = 0.038). The results of the CO2 isotopic signal [δ13CO2(‰)] analysis gave similar but inverse results (lower level inside nests than outside; data not shown) (Measurement location: ANOVA mixed model, F1,268.60 = 1134.56, p < 0.0001; Nestling age: F3,263.75 = 38.10, p < 0.0001; Measurement location × nestling age interaction: F3,247.37 = 22.43, p < 0.0001]. Temperature, instead of relative humidity, was negatively and significantly related to δ13CO2(‰) (ANOVA mixed model, F1,270.95 = 4.13, p < 0.043), whereas brood size was positively related to δ13CO2(‰) (F1, 51.26 = 13.60, p = 0.001).
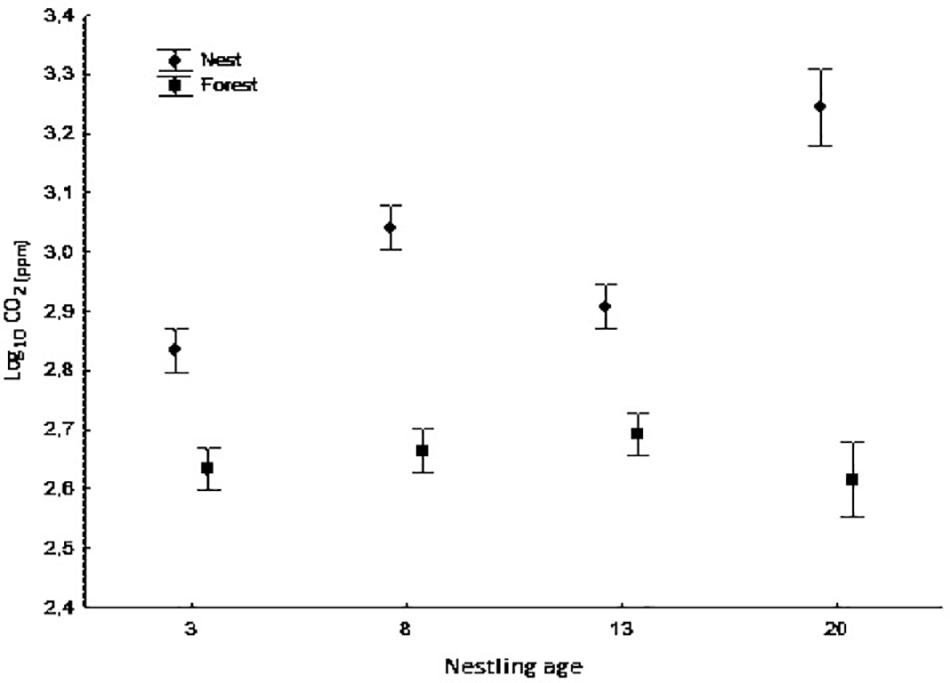
Figure 1. Average concentration of CO2 (log10) inside nest-boxes and in forest air at different nestling ages (days 3, 8, 13, and 20). Bars show mean ± SE.
CH4 concentration was lower inside occupied nests boxes than in the forest air (ANOVA mixed model, F1,240.53 = 659.99, p < 0.0001) at all nestling ages (ANOVA mixed model, measurement location × nestling age interaction: F3,240.86 = 3.30, p = 0.021, Figure 2). Moreover, CH4 concentration inside nests differed significantly according to nestling age (ANOVA mixed model, F3,249.13 = 12.19, p < 0.0001). The highest concentration was observed at day 3 and the lowest at day 20. In addition, CH4 concentration was negatively and significantly related to hatching date (ANOVA mixed model, F1,51.24 = 9.40, p = 0.003) and positively to relative humidity (F1,223.18 = 16.10, p < 0.0001). The results of the CH4 isotopic signal [δ13CH4 (‰)] analysis showed similar but inverse patterns (a higher isotopic signal in nests that in the forest; see also Figure 3) (Measurement location: ANOVA mixed model, F1,265.55 = 427.87, p < 0.0001; Nestling age: F3,259.21 = 4.11, p = 0.007; Measurement location × nestling age interaction: F3,241.77 = 11.82, p < 0.0001). δ13CH4(‰) was positively related to temperature and relative humidity (ANOVA mixed model, F1,281.50 = 10.82, p = 0.001 and F1,273.79 = 9.95, p = 0.002, respectively) and negatively to hatching date (F1,47.65 = 11.27, p = 0.002).
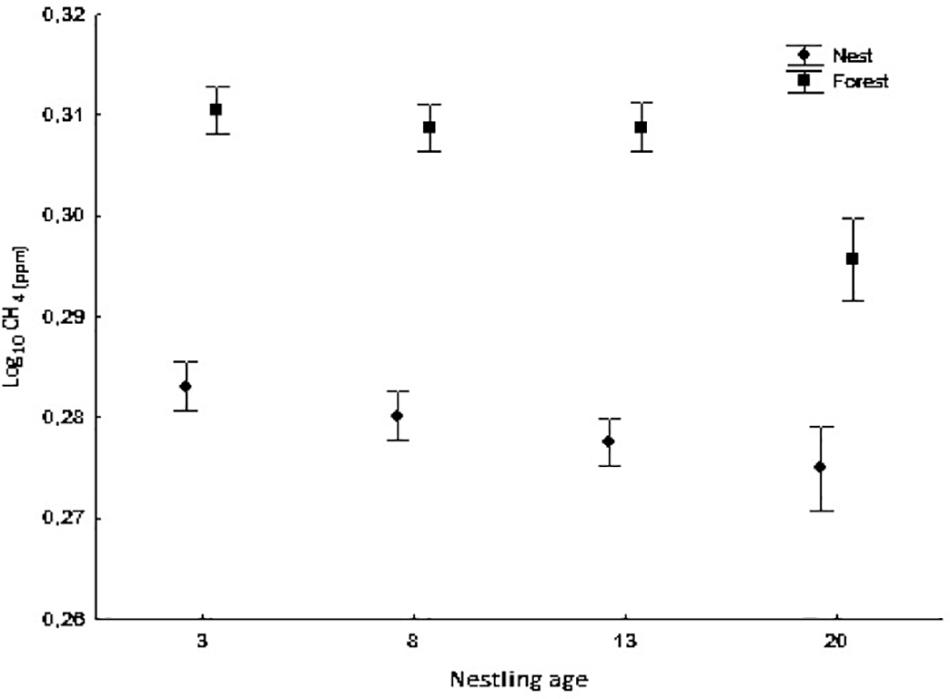
Figure 2. Average concentration of CH4 (log10) in nest boxes and forest air at different nestling ages (days 3, 8, 13, and 20). Bars show mean ± SE.
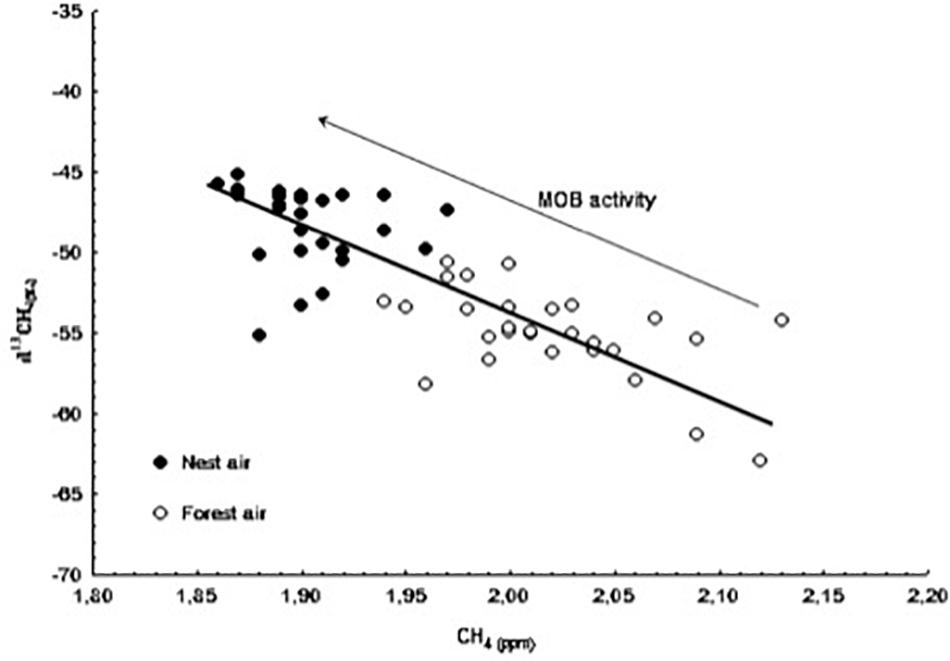
Figure 3. Concentration values of methane (CH4) and δ13C-CH4 from samples taken at the nest-boxes (black dots) and from the outdoor air samples (white dots) throughout the monitoring period. The direction of increase in methane oxidizing bacteria (MOB) activity is shown by an arrow.
Once nestlings fledged, CO2 concentration did not differ between nest and forest air (unpaired t-test: t = 0.60, df = 16, p = 0.554); however, the isotopic signal [δ13CO2(‰)] was lower inside nests (unpaired t-test: t = −2.59, df = 16; p = 0.019). By contrast, CH4 concentration was significantly lower and the isotopic signal [δ13CH4(‰)] heavier inside nests once nestlings fledged compared with the forest air (unpaired t-test: t = −8.07, df = 16, p < 0.001 and unpaired t-test: t = 3.22, df = 16, p = 0.005, respectively; see Tables 1, 2).
Nest Ectoparasites
The abundances of the different parasites collected in the blue tit nest boxes are shown in Table 3. We found a significant positive relationship between the abundance of biting midges (Culicoides spp.) and differences in CO2 levels between forest air and air inside nest boxes at day 20 of nestling age (B = 0.001, F1,14 = 5.78, p = 0.031; Figure 4). The presence of an influential data point could drive this relationship; however, the binomial approach was used to control for the aggregated distribution of parasites in the wild. The presence of high numbers of Culicoides in a few nests is frequently observed in our study area and, therefore, is part of the natural variation. When we excluded this data point from the comparison, the relationship was no longer significant (B = 0.001, F1,13 = 0.33, p = 0.577).
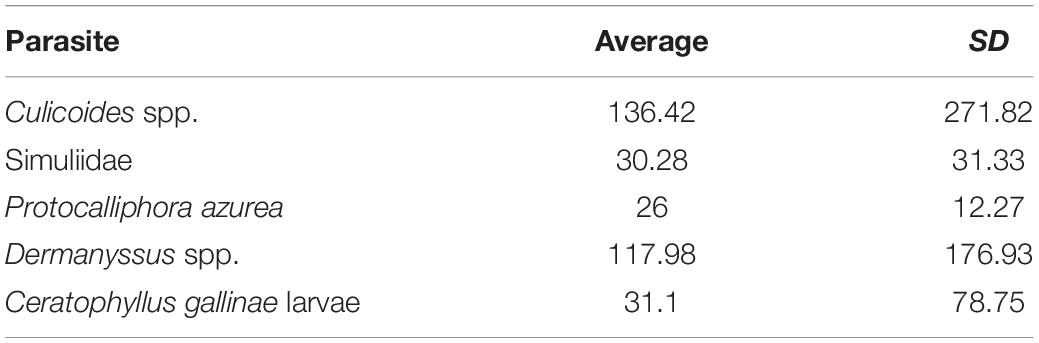
Table 3. Abundance and standard deviation of the different ectoparasites collected in blue tit nest-boxes.
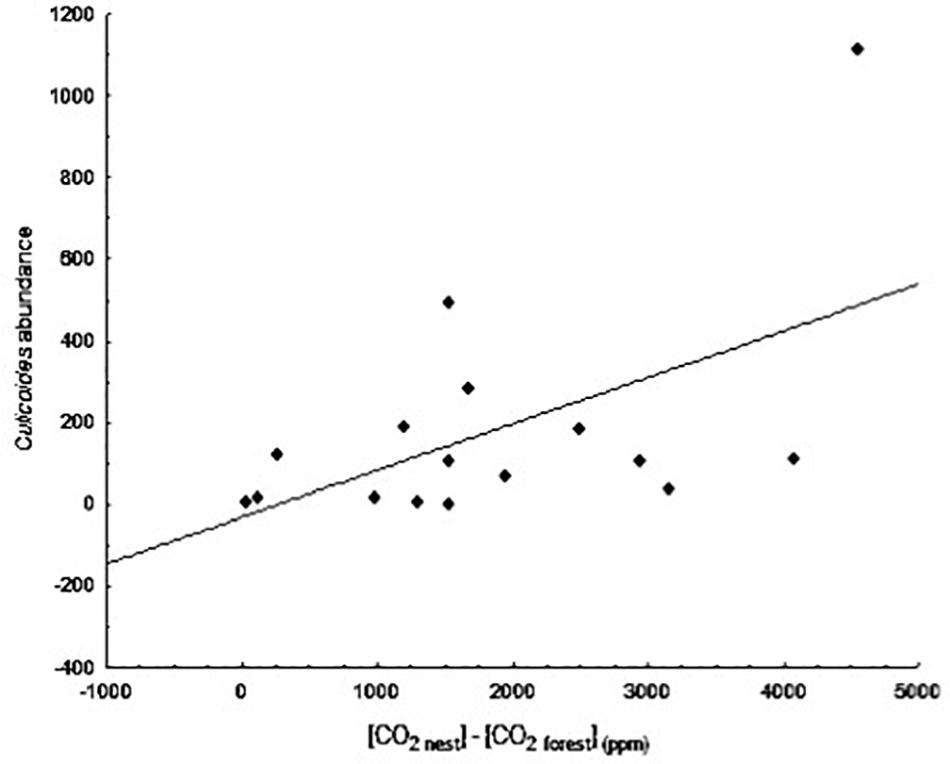
Figure 4. Relationship between the difference in concentration of CO2 between nest and forest ([CO2 nest] – [CO2 forest]) at day 20 of nestling age and abundance of biting midges at day 13.
We also found a significant positive relationship between blackfly abundance and CH4 differences at day 8 of nestling age (B = 11.26, F1,39 = 10.78, p = 0.002; Figure 5). As in the case of the biting midges, it is not uncommon to observe high numbers of blackflies in some nests in the wild. In this case, however, the relationship was still significant after excluding the potentially influential data point (B = 11.28, F1,38 = 17.38, p < 0.001). Blowfly abundance was not significantly related to any of the variables at any nestling age (data not shown, p > 0.05 in all cases).
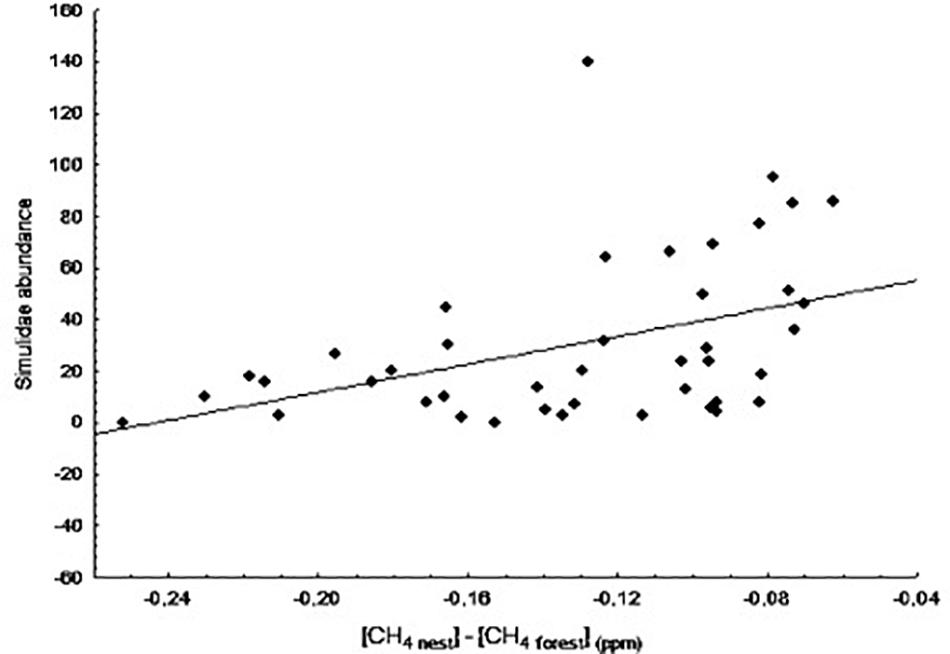
Figure 5. Relationship between the difference in concentration of CH4 between nest and forest ([CH4 nest] – [CH4 forest]) at day 8 of nestling age and abundance of Simuliidae at day 13.
We observed a significant positive relationship between mite abundance and CO2 differences at days 3 and 20 of nestling age (B = 0.002, F1,38 = 9.59, p = 0.004 and B = 0.001, F1,12 = 6.75, p = 0.023, respectively) and between mite abundance and CH4 differences at day 20 of nestling age (B = 35.86, F1,12 = 14.37, p = 0.003). Mite abundance also appeared to have a significant positive relationship with brood size at day 3 of nestling age (B = 0.287, F1,38 = 4.43, p = 0.042) but a negative one at day 20 (B = −0.475, F1,12 = 4.93, p = 0.046).
Ceratophyllus gallinae (flea) larval abundance was significantly and negatively related to brood size at days 13 and 20 of nestling age (B = −0.613, F1,40 = 9.32, p = 0.004 and B = −0.493, F1,14 = 7.33, p = 0.017, respectively), and positively to relative humidity at day 13 (B = 11.26, F1,40 = 9.38, p = 0.004). The relationships among the other variables including gasses, humidity, brood size and ectoparasites were not significant according to the results of an Omnibus test (P > 0.05, data not shown).
The female body condition was not related to any of the variables except blowfly pupal abundance, with which it had a significant negative relationship (multiple linear regression, F1,33 = 10.17, p = 0.003). Male and nestling body condition were not significantly related to the abundance of any of the ectoparasites (p > 0.05 in all cases).
Discussion
The concentration of gasses (i.e., CO2 and CH4) inside bird breeding cavities has been poorly studied, much less the effects of these gasses on the attraction of parasites to nests, and their growth once inside the nests. In this study, we showed that CO2 and CH4 concentration and isotopic signal [δ13CO2(‰) and δ13CH4(‰)] inside blue tit (C. caeruleus) nest boxes differed from forest air during the nestling period (from 24 May to June 20) in an ecosystem dominated by the activity of C3 plants. Air inside nest boxes had a higher concentration of CO2 and a lighter δ13CO2(‰) than forest air. A similar result has also been observed in studies of burrow nesting birds [e.g., the European bee-eater M. apiaster (White et al., 1978) and the Sand Martin R. riparia, (Mondain-Monval and Sharp, 2018)]. Before and after the nestling period, we did not find any significant differences between the concentration of CO2 in nest-box and forest air. Thus, as expected, nestlings increase the concentration of CO2 inside nests and more nestlings imply a higher concentration of this gas. Slight but significant differences in the isotopic signal of CO2 in the absence of nestlings suggest the presence of microbial activity in the nesting material.
In our study, the greatest differences in CO2 concentration between air inside nest boxes and forest air were observed at days 8 and 20 of nestling age. Morganti et al. (2017) showed that blue tit nestlings have a greater mass gain between days 4 and 8 of nestling age. Therefore, changes in the CO2 concentration observed on day 8 may have been due to an increase in growth and, in turn, metabolic rate after hatching, resulting in a higher level of CO2 inside nest boxes. An increase in the metabolic rate of nestlings may also be due to the initiation of thermoregulatory development, a process that usually begins around 8 days of age in passerine birds (Visser, 1998; Pereyra and Morton, 2001). In addition to higher CO2 production by nestlings due to thermoregulation is the respiratory effect of adult females that may have been still brooding small chicks and staying inside the nests for long periods. The greatest difference in CO2 concentration between nest and forest air was found on day 20 of nestling age. This difference could have been due to the higher level of activity that nestlings display just before leaving the nest. Previous studies have also found a relationship between nestling age and gas concentration in nests. For example, Wickler and Marsh (1981) reported increases in CO2 concentration as nestlings aged in the nest chambers of bank swallows (R. riparia), and Mersten-Katz et al. (2012) found that woodpecker nestlings have little influence on the concentration of CO2 inside nests until they reach 15 days of age and become more active within the nests.
To our knowledge, this study is the first to analyze the concentration of CH4 in nest boxes throughout the nestling period. In contrast to our results for CO2, we observed a lower concentration of CH4 and a significantly heavier isotopic signal [δ13CH4(‰)] inside nest boxes compared with forest air. This difference was evident even in the nests without nestlings, indicating that methane-oxidizing bacteria (MOB), which are thought to be the main consumer of CH4 (Hanson and Hanson, 1996), are active inside nest boxes from the very beginning of the breeding season. MOB activity may be more intense and slightly modified in nests due to the insulating effect of the boxes and the metabolic activity of the birds inside (probably related to the accumulation of waste and feces). Also, the negative relationship observed between CH4 concentration and hatching date indicates that MOB activity increases as the season progresses. Figure 3 shows that the progressive decrease in CH4 concentration in nest air during the nesting period is linked to the increase in δ13C-CH4 values. This result shows that the pattern of CH4 oxidization occurring in the ecosystem due to the presence of MOB in the forest soil is the same but less intense as the one observed in the nests. In other words, it is proof of intense microbial activity within nests, which may be related to bird health or the presence and/or abundance of parasites. Finally, compared with nest and forest air, the concentration of CH4 in soil air was always lower and the isotopic ratio was always lighter (see Table 1). These data are consistent with the removal of CH4 from aerobic soils by bacterial oxidization (Conrad, 1996) and the subsequent consumption of the atmospheric methane in the ecosystem.
We are unaware of any studies investigating the relationship between gasses inside nest cavities and abiotic factors (i.e., relative humidity and temperature) during the nestling period. In our study, CO2 concentration inside nest boxes was positively and significantly related to relative humidity at different nestling ages. The presence of nestlings (which increase CO2 levels) may increase transpiration, leading to an increase in relative humidity inside nests. The concentration of CH4 was also positively related to relative humidity, perhaps due to an indirect effect of humidity on bacterial activity. However, studies that experimentally test this potential effect are needed to confirm the relationships among these factors. In the case of the isotopic signals, temperature showed a negative relationship with δ13CO2(‰), whereas temperature and relative humidity were both positively related to δ13CH4(‰). Nestlings may increase the temperature inside nests, which could favor the dilution of gasses. The nature of these relationships, however, is still unclear and merit more research to be fully understood.
Our starting hypothesis was that differences in gas concentrations between nests and the forest could be an attraction cue for ectoparasites. The significant difference observed in the concentration of both CO2 and CH4 between nest and forest air support that possibility. In this respect, we expected to find a positive relationship between changes in gas concentration and ectoparasite abundance, particularly of those parasites actively seeking hosts (i.e., blackflies, biting midges, and blowflies). The positive relationship observed between biting midge abundance and CO2 concentration differences between nest and forest air at day 20 of nestling age clearly indicates that biting midges can use CO2 concentration inside nests as a cue to locate their hosts (Figure 4). We also observed a positive relationship between blackfly (Simuliidae) abundance and CH4 concentration differences between nest and forest air. That is, blackflies were more abundant in nests that had a high CH4 concentration and lower concentration differences between nest and forest air (Figure 5). A low concentration of CH4 inside nests implies a high level of bacterial activity, which could be, in some way, detrimental to blackflies. In fact, bacteria have been used to infect larvae as a means to control blackfly populations (De Barjac, 1978; Hougard and Back, 1992); however, the bacteria used in this system could be different than those growing in the nests. Further investigation of the bacterial composition of the nests would be needed to confirm this hypothesis. It is also possible that blackflies are initially attracted to hosts based on visual cues and only use host odors (e.g., ether extracts and CO2) once a host has been located (Fallis and Smith, 1964). Alternatively, Tomás and Soler (2016) have proposed that acoustic signals produced during nestling begging behavior attract blood-feeding insects to nests. We did not observe any significant relationship between blowfly abundance and gas concentration differences at any nestling age, indicating that this fly species does not use CO2 or CH4 concentration to locate their hosts, but rather relies on other attraction cues.
We observed some correlation between differences in gas concentration and mite abundance. In contrast to other ectoparasites, mites must be transported to new nests by way of adult birds, for instance, when they visit old nests or cavities containing mites (Proctor and Owens, 2000). In some cases, mites can also reach a new nest by phoresy on other arthropods (Marshall, 1981, p. 274). Thus, it is possible that some mites reached the nests via other arthropods that use CO2 concentration as an attraction cue. Alternatively, the positive relationship between CO2 differences and mite abundance at day 3 of nestling age may be associated with brood size: a high number of nestlings (and hence higher CO2 production) may be needed at the beginning of nestling development to enable mite population growth within nests. Mites go through several life cycle stages before reaching adulthood. In this context and given their short life span, mite population growth might depend more on the initial number of nestlings rather than on nestling age. Another possibility is that mites may have disturbed brooding females at the beginning of the nestling period, causing them to produce more CO2, thus accounting for the positive relationship between mite abundance and CO2 concentration at day 3 of nestling age. The positive relationship between mite abundance and CH4 concentration differences at the end of the nestling period (day 20) might be related to bacterial activity, as suggested in the case of blackflies. The negative relationship observed between brood size and flea abundance at days 13 and 20 of nestling age and between brood size and mite abundance at day 20 could be due to the fact that some of the nestlings at those ages abandoned some of the more infested nests (see e.g., Berggren, 2005).
Temperature and humidity are important factors for ectoparasite development. For example, several studies have shown that a high humidity environment can favor an increase in the number of ectoparasites (Heeb et al., 2000; Moyer et al., 2002). The positive relationship between relative humidity and larval flea abundance inside nests could be explained by the importance of humidity for their development. Indeed, an experimental increase in temperature inside blue tit nest boxes leads to a reduction in relative humidity and in the number of blowfly pupae in nests (Castaño-Vázquez et al., 2018).
Blowfly pupal abundance was negatively and significantly related to the condition of blue tit females. Tomás et al. (2005) also found a negative effect of blowfly pupae on the mass of blue tit females in the same study area. This relationship is indicative of an extra effort made by females trying to compensate for the effect of blowflies on nestlings, supporting the idea that females pay part of the cost of parasitism on nestlings (Tripet and Richner, 1997; Bouslama et al., 2002).
Differences between the atmospheric gas composition of nest boxes and the surrounding forest appear to be a cue, among other potential ones, used by, at least, biting midges to locate hosts. On the basis of our results, we cannot conclude that gas concentration differences act as an attraction cue for the other parasites, which may use other cues. Clearly, more studies are needed to better understand the relationships among nest cavity environment, hosts, parasites and even bacteria living in nests. In this respect, studies that analyze gas composition, and its variation, inside nest boxes would provide key insights on the host–parasite relationships of these organisms.
Data Availability Statement
The datasets generated for this study are available on request to the corresponding author.
Ethics Statement
The animal study was reviewed and approved by Dirección General de Agricultura, Ganaderia y Alimentación, Comunidad de Madrid.
Author Contributions
FC-V and SM conducted the field-work and parasite analyses. SC and SS-M conducted the analyses of gasses. All authors contribute to the design of the research and analyses of data as well as writing of MS.
Funding
We thank the National Museum of Natural Sciences (MNCN) for providing facilities for this research. Moreover, this study is a contribution to the research developed at the ‘El Ventorrillo’ field station. This study was funded by the project CGL2015-67789-C2-1-P, PGC2018-097426-B-C21, and CGL2016-78318-C2-1-R (MINECO/MICINN/FEDER). The funders had no role in the study design, data collection and analysis, decision to publish, or preparation of the manuscript. The Junta de Castilla y León authorized the ringing and handling of birds. We acknowledge support of the publication fee by the CSIC Open Access Publication Support Initiative through its Unit of Information Resources for Research (URICI). A previous version of this manuscript was released as a pre-print at bioRxiv (Castaño-Vázquez et al., 2019).
Conflict of Interest
The authors declare that the research was conducted in the absence of any commercial or financial relationships that could be construed as a potential conflict of interest.
Footnotes
References
Amundson, R., Stern, L., Baisden, T., and Wang, Y. (1998). The isotopic composition of soil and soil-respired CO2. Geoderma 82, 83–114. doi: 10.1016/s0016-7061(97)00098-0
Berggren, Å (2005). Effect of the blood-sucking mite Ornithonyssus bursa on chick growth and fledging age in the North Island robin. N. Z. J. Ecol. 29, 243–250.
Bogner, F. (1992). Response properties of CO2-sensitive receptors in tsetse flies (Diptera: Glossina palpalis). Physiol. Entomol. 17, 19–24. doi: 10.1111/j.1365-3032.1992.tb00985.x
Bouslama, Z., Lambrechts, M. M., Ziane, N., Djenidi, R., and Chabi, Y. (2002). The effect of nest ectoparasites on parental provisioning in a North-African population of the Blue Tit Parus caeruleus. Ibis 144, 73–78. doi: 10.1046/j.1474-919X.2002.00070
Castaño-Vázquez, F., Martínez, J., Merino, S., and Lozano, M. (2018). Experimental manipulation of temperature reduce ectoparasites in nests of blue tits Cyanistes caeruleus. J. Avian Biol. 49:e01695. doi: 10.1111/jav.01695
Castaño-Vázquez, F., Merino, S., Cuezva, S., and Sánchez-Moral, S. (2019). Microclimate, CO2 and CH4 concentration on Blue tits (Cyanistes caeruleus) nests: effects of brood size, nestling age and on ectoparasites. bioRxiv
Cerling, T. E., Solomon, D. K., Quade, J., and Bowman, J. R. (1991). On the isotopic composition of carbon in soil carbon-dioxide. Geochim. Cosmochim. Acta 55, 3403–3405. doi: 10.1016/0016-7037(91)90498-T
Conrad, R. (1996). Soil microorganisms as controllers of atmospheric trace gases (H2, CO, CH4, OCS, N2O, and NO). Microbiol. Rev. 60, 609–640. doi: 10.1128/mmbr.60.4.609-640.1996
Crosson, E. R. (2008). A cavity ring-down analyzer for measuring atmospheric levels of methane, carbon dioxide, and water vapor. Appl. Phys. 92, 403–408. doi: 10.1007/s00340-008-3135-y
Crutzen, P. J., Aselmann, I., and Seiler, W. (1986). Methane production by domestic animals, wild ruminants, other herbivorous fauna, and humans. Tellus B. 38, 271–284. doi: 10.3402/tellusb.v38i3-4.15135
De Barjac, H. (1978). Une nouvelle variété de Bacillus thuringiensistrès toxique pour les moustiques: B. thuringiensis var israelensis sérotype H14. C. R. Acad. Sci. 286, 797–800.
Dube, W. C., Hund, A. K., Turbek, S. P., and Safran, R. J. (2018). Microclimate and host body condition influence mite population growth in a wild bird-ectoparasite system. Int. J. Parasitol. Parasites Wildl. 7, 301–318. doi: 10.1016/j.ijppaw.2018.07.007
Fallis, A. M., and Smith, S. M. (1964). Ether extracts from birds and CO2 as attractants for some ornithophilic simuliids. Can. J. Zool. 42, 723–730. doi: 10.1139/z64-069
Fernandez-Cortes, A., Cuezva, S., Alvarez-Gallego, M., Garcia-Anton, E., Pla, C., Benavente, D., et al. (2015). Subterranean atmospheres may act as daily methane sinks. Nat. Commun. 6:7003. doi: 10.1038/ncomms8003
Fernandez-Cortes, A., Perez-Lopez, R., Cuezva, S., Calaforra, J. M., Cañaveras, J. C., and Sanchez-Moral, S. (2018). Geochemical fingerprinting of rising deep endogenous gases in an active hypogenic karst system. Geofluids 2018:19. doi: 10.1155/2018/4934520
Garcia-Anton, E., Cuezva, S., Fernandez-Cortes, A., Alvarez-Gallego, M., Pla, C., Benavente, D., et al. (2017). Abiotic and seasonal control of soil-produced CO2 efflux in karstic ecosystems located in Oceanic and Mediterranean climates. Atmos. Environ. 164, 31–49. doi: 10.1016/j.atmosenv.2017.05.036
Garcia-Anton, E., Cuezva, S., Fernandez-Cortes, A., Benavente, D., and Sanchez-Moral, S. (2014). Main drivers of diffusive and advective processes of CO2-gas exchange between a shallow vadose zone and the atmosphere. Int. J. Greenh. Gas Con. 21, 113–129. doi: 10.1016/j.ijggc.2013.12.006
GatesNotes. (2014). Mosquito Week. The Deadliest Animal in the World. Available online at: https://www.gatesnotes.com/Health/Most-Lethal-Animal-Mosquito-week?WT.mc_id=00_00_00_share_em [Accessed 29 April 2020]
Grant, A. J., and Kline, D. L. (2003). Electrophysiological responses from Culicoides (Diptera: Ceratopogonidae) to stimulation with carbon dioxide. J. Med. Entomol. 40, 284–292. doi: 10.1603/0022-2585-40.3.284
Grant, A. J., Wigton, B. E., Aghajanian, J. G., and O’Connell, R. J. (1995). Electrophysiological responses of receptor neurons in mosquito maxillary palp sensilla to carbon dioxide. J. Comp. Physiol. A 177, 389–396.
Hanson, R. S., and Hanson, T. E. (1996). Methanotrophic bacteria. Microbiol. Rev. 60, 439–471. doi: 10.1128/mmbr.60.2.439-471.1996
Heeb, P., Kölliker, M., and Richner, H. (2000). Bird-ectoparasite interactions, nest humidity, and ectoparasite community structure. Ecology 81, 958–996. doi: 10.2307/17717010
Hougard, J. M., and Back, C. (1992). Perspectives on the bacteriological control of vectors in the tropics. Parasitol. Today 8, 364–366. doi: 10.1016/0169-4758(92)90168-2
Lamson, G. H. Jr., and Edmond, H. D. (1914). Carbon dioxide in incubation. Bull. Storrs. Agric. Exp. Station 76, 219–257.
Lehane, M. J. (2005). The Biology of Blood-Sucking in Insects, 2nd Edn. Cambridge: Cambridge University Press, 337.
Macfie, J. W. S., and Thomson, J. G. (1929). A trypanosome of the canary (Serinus canarius, Koch). Trans. R. Soc. Trop. Med. Hyg. 23, 185–190.
Mainwaring, M. C. (2017). Causes and consequences of intraspecific variation in nesting behaviors: insights from blue tits and great tits. Front. Ecol. Evol. 5:39. doi: 10.3389/fevo.2017.00039
Marquardt, W. C., Demaree, R. S., and Grieve, R. B. (2000). Parasitology and Vector Biology, 2nd Edn. San Diego, CA: Harcourt Academic Press, 702.
Martínez-de la Puente, J., Martínez, J., Rivero de Aguilar, J., Herrero, J., and Merino, S. (2011). On the specificity of avian blood parasites: revealing specific and generalist relationships between haemosporidians and biting midges. Mol. Ecol. 20, 3275–3287. doi: 10.1111/j.1365-294X.2011.05136.x
Martínez–de la Puente, J., Merino, S., Tomás, G., Moreno, J., Morales, J., Lobato, E., et al. (2010). The blood parasite Haemoproteus reduces survival in a wild bird: a medication experiment. Biol. Lett. 6, 663–665. doi: 10.1098/rsbl.2010.0046
Maseyk, K., Wingate, L., Seibt, U., Ghashghaie, J., Bathellier, C., Almeida, P., et al. (2009). Biotic and abiotic factors affecting the delta C-13 of soil respired CO2 in a Mediterranean oak woodland. Isot. Environ. Health Stud. 45, 343–359. doi: 10.1080/10256010903388212
Maziarz, M. (2019). Breeding birds actively modify the initial microclimate of occupied tree cavities. Int. J. Biometeorol. 63, 247–257. doi: 10.1007/s00484-018-01658-x
Mboera, L., and Takken, W. (1997). Carbon dioxide chemotropism in mosquitoes (Diptera: Culicidae) and its potential in vector surveillance and management programmes. Rev. Med. Vet. Entomol. 85, 355–368.
McPhatter, L., and Gerry, A. C. (2017). Effect of CO2 concentration on mosquito collection rate using odor-baited suction traps. J. Vect. Ecol. 42, 44–50. doi: 10.1111/jvec.12238
Merino, S., Moreno, J., Sanz, J. J., and Arriero, E. (2000). Are avian blood parasites patho- genic in the wild? A medication experiment in blue tits (Cyanistes caeruleus). Proc. R. Soc. Lond. B 267, 2507–2510. doi: 10.1098/rspb.2000.1312
Merino, S., and Potti, J. (1995). Mites and blowflies decrease growth and survival in nestling pied flycatchers. Oikos 73, 95–103. doi: 10.2307/354730
Mersten-Katz, C., Barnea, A., Yom-Tov, Y., and Ar, A. (2012). The woodpecker’s cavity microenvironment: advantageous or restrict? Avian Biol. Res. 5, 227–237. doi: 10.3184/174751912X13530894822224
Mondain-Monval, T., and Sharp, S. P. (2018). Burrow depth, carbon dioxide and reproductive success in Sand Martins Riparia riparia. Bird Study 65, 123–131. doi: 10.1080/00063657.2018.1438363
Morganti, M., Rubolini, D., Caprioli, M., Saino, N., and Ambrosini, R. (2017). Rainfall, but no temperature, negatively affects the growth of blue tit (Cyanistes caeruleus) nestlings. Bird Study 64, 159–167. doi: 10.180/00063657.2017.1309006
Moyer, R. B., Drown, M. D., and Clayton, H. D. (2002). Low humidity reduces ectoparasite pressure: implications for host life history evolution. Oikos 2, 223–228. doi: 10.1034/j.1600-0706.2002.970208.x
Pereyra, M. E., and Morton, M. L. (2001). Nestling growth and thermoregulatory development in subalpine dusky flycatchers. Auk 118, 116–136. doi: 10.1093/auk/118.1.116
Peterson, B. J., and Fry, B. (1987). Stable isotopes in ecosystem studies. Ann. Rev. Ecol. Syst. 18, 293–320. doi: 10.1146/annurev.es.18.110187.001453
Proctor, H., and Owens, I. (2000). Mites and birds: diversity, parasitism and coevolution. Trends Ecol. Evol. 15, 358–364. doi: 10.1016/s0169-5347(00)01924-8
Sanz, J. J. (2002). Climate change and breeding parameters of great and blue tits throughout the Western Palaearctic. Glob. Change Biol. 8, 409–422. doi: 10.1046/j.1365-2486.2002.00496.x
Takken, W., and Knols, B. G. J. (1999). Odor-mediated behavior of afrotropical malaria mosquitoes. Annu. Rev. Entomol 44, 131–157. doi: 10.1146/annurev.ento.44.1.131
Tomás, G., Merino, S., Martínez, J., Moreno, J., and Sanz, J. J. (2005). Stress protein levels and blood parasite infection in blue tits (Parus caeruleus): a medication field experiment. Ann. Zool. Fennici. 42, 45–56.
Tomás, G., Merino, S., Martínez-de la Puente, J., Moreno, J., Morales, J., and Lobato, E. (2008). A simple trapping method to estimate abundances of blood-sucking flying insects in avian nests. Anim. Behav. 75, 723–729. doi: 10.1016/j.anbehav.2007.08.018
Tomás, G., Merino, S., Moreno, J., and Morales, J. (2007). Consequences of nest reuse for parasite burden and female health and condition in blue tits, Cyanistes caeruleus. Anim. Behav. 73, 805–814. doi: 10.1016/j.anbehav.2006.06.016
Tomás, G., Merino, S., Moreno, J., Sanz, J. J., Morales, J., and García-Fraile, S. (2006). Nest weight and female health in the blue tit (Cyanistes caeruleus). Auk 123, 1013–1021. doi: 10.2307/25150216
Tomás, G., and Soler, J. J. (2016). Begging and ectoparasite attraction. Anim. Behav. 113, 93–98. doi: 10.1016/j.anbehav.2015.12.026
Tripet, F., and Richner, H. (1997). Host responses to ectoparasites: food compensation by parent blue tits. Oikos 78, 557–561. doi: 10.2307/3545617
Valkiunas, G. (2005). Avian Malaria Parasites and other Haemosporidia. Boca Raton, FL: CRC Press, 1932.
Visser, G. H. (1998). “Development of temperature regulation,” in Avian Growth and Development: Evolution within the Altricial–Precocial Spectrum, eds J. M. Starck and R. E. Ricklefs (New York, NY: Oxford University Press), 117–156.
Walsberg, G. E. (1980). The gaseous microclimate of the avian nest during incubation. Am. Zool. 20, 363–372. doi: 10.1093/icb/20.2.363
Wangensteen, O. D., Wilson, D., and Rahn, H. (1971). Diffusion of gases across the shell of the hen’s egg. Respir. Physiol. 11, 16–30. doi: 10.1016/0034-5687(70)90099-x
Werner, C., Unger, S., Pereira, J. S., Maia, R., Kurz-Besson, C., David, T. S., et al. (2006). Importance in short-term dynamics in carbon isotope ratios of ecosystem respiration (δ13CR) in a Mediterranean oak woodland and linkage to environmental factors. New Phytol. 172, 330–346. doi: 10.1111/j.1469-8137.2006.01836.x
White, F. N., Bartholomew, G. A., and Kinney, J. L. (1978). Physiological and ecological correlates of tunnel nesting in the European bee-eater, Merops apiaster. Physiol. Zool. 51, 140–154. doi: 10.1086/physzool.51.2.30157862
Wickler, S. J., and Marsh, R. L. (1981). Effects of nestling age and burrow depth on CO2 and concentrations in the burrows of Bank Swallows (Riparia riparia). Physiol. Zool. 54, 132–136. doi: 10.1086/physzool.54.1.30155811
Wright, R. H., and Kellogg, F. E. (1962). Response of Aedes aegypti to moist convection currents. Nature 194, 402–403. doi: 10.1038/194402a0
Keywords: CH4, CO2, ectoparasites, gasses, nesting period, relative humidity
Citation: Castaño-Vázquez F, Merino S, Cuezva S and Sánchez-Moral S (2020) Nest Gasses as a Potential Attraction Cue for Biting Flying Insects and Other Ectoparasites of Cavity Nesting Birds. Front. Ecol. Evol. 8:258. doi: 10.3389/fevo.2020.00258
Received: 12 February 2020; Accepted: 14 July 2020;
Published: 05 August 2020.
Edited by:
Laura Gangoso, Consejo Superior de Investigaciones Científicas (CSIC), SpainReviewed by:
Alejandro Cantarero, University of Turku, FinlandGustavo Tomás, Estación Experimental de Zonas Áridas (EEZA), Spain
Copyright © 2020 Castaño-Vázquez, Merino, Cuezva and Sánchez-Moral. This is an open-access article distributed under the terms of the Creative Commons Attribution License (CC BY). The use, distribution or reproduction in other forums is permitted, provided the original author(s) and the copyright owner(s) are credited and that the original publication in this journal is cited, in accordance with accepted academic practice. No use, distribution or reproduction is permitted which does not comply with these terms.
*Correspondence: Santiago Merino, c2FudGlhZ29tQG1uY24uY3NpYy5lcw==