- 1GIS & Spatial Ecology Laboratory, Halmos College of Natural Sciences and Oceanography, Nova Southeastern University, Fort Lauderdale, FL, United States
- 2National Centers for Coastal Ocean Science, National Oceanic and Atmospheric Administration, Silver Spring, MD, United States
- 3Florida State University Coastal and Marine Laboratory, St. Teresa, FL, United States
- 4Fish and Wildlife Research Institute, Florida Fish and Wildlife Conservation Commission, St. Petersburg, FL, United States
Florida’s west coast is a 170,000 km2 bedrock shelf (west Florida shelf, WFS) comprised of north-south discontinuous carbonate outcroppings extending more than 200 km from the intertidal zone to a depth of 200 m. These outcrops support diverse benthic communities, which contribute to a multi-billion dollar recreational and commercial fishing industry, yet only about 5% of their extent has been studied in detail. Benthic communities shift over a 6.5° geographic range, but the locations of these shifts are not well-defined. Previous studies have suggested a break in biogeographic regions at Tampa Bay, south at Cape Romano, and north at Cedar Key. The goal of this study was to map and investigate the shallow WFS marine hardbottom north and south of Tampa Bay, FL to identify differences in benthic communities and identify ecoregion boundaries. Habitat mapping yielded 295.89 km2 of hardbottom which differed in extent between Sarasota and Pasco counties. Benthic surveys tabulated 4,079 stony coral colonies of nine species and 1,918 soft corals. Stony corals were dominated by Siderastrea radians, Oculina robusta, Solenastrea hyades, and Cladocora arbuscula less than 10 cm in diameter. Distinct differences in these communities were evident from south to north. The main community shift indicated an ecoregion boundary at, or very near, the mouth of Tampa Bay. Another shift associated with the Bahamas Fracture Zone (BFZ) occurred at the Pinellas and Pasco County border. The outputs of this work provide the first detailed benthic habitat map of the area, a detailed survey of the composition of hardbottom benthic communities in the region, identify Tampa Bay as a coastal benthic biogeographic transition, and illustrate the influence of the BFZ on coastal communities. These findings illustrate a need for additional WFS benthic research and mapping to give a more comprehensive understanding of coral community biogeography in the context of future warming conditions and the potential tropicalization. Unifying seafloor mapping data, mapping new areas with high probability of hardbottom resources, and collecting benthic community data over broader scales will refine community biogeographic zonation. This is a necessary precursor to any long-term community monitoring to detect spatial shifts in communities and population modeling.
Introduction
The Florida-Bahama Platform is ∼900 km long extending from 25°N to 30°N (Paull et al., 1990). It covers a broad range of seafloor morphologies, bathymetric gradients, sediment types, benthic biological communities, hardbottom exposures, and reef structures (Hine et al., 2008; Hine and Locker, 2011). There are three southern and central Florida coastal sub-regions that exhibit distinct morphological and oceanographic characteristics from west to east: the west Florida shelf (WFS); the Florida Keys/Dry Tortugas; and the Southeast Florida Coast (Nuttle and Fletcher, 2013). The benthic communities of the Florida Keys/Dry Tortugas and the Southeast Florida Coast have been extensively investigated (Phillips et al., 1990; Hine and Locker, 2011; Walker and Gilliam, 2013; Jaap, 2015), while the WFS communities remain relatively understudied, despite their ecological and economic value (Tchounwou, 1999; Colella et al., 2008; Love et al., 2013; Saul et al., 2013). Estimates show that 50% of the WFS is exposed low relief hardbottom and that only 5% has been studied through detailed surveys (Thompson et al., 1999; Obrochta et al., 2003). These are limited to well-known reef areas (e.g., Pulley Ridge, Florida Middle Grounds, and Steamboat Lumps) or small-scale studies in nearshore habitats (Hine et al., 2003; Hine and Locker, 2011; Baumstark et al., 2016).
The WFS is defined by an expansive bedrock shelf (170,000 km2) that runs from the Florida Panhandle to the lower Florida Keys (Okey et al., 2004). It is comprised of discontinuous carbonate outcroppings that run north and south extending more than 200 km west from the intertidal zone to a depth of 200 m (Okey et al., 2004; Mallinson et al., 2014). The inner continental shelf is separated into three geologic areas: a shallow bedrock sand ridge north of Tampa Bay, a middle ebb- tidal delta, and a southern deep bedrock sand ridge south of Tampa Bay. This underlying bedrock (hardbottom) is common in carbonate and siliclastic marine environments worldwide and consists of ledges that support diverse benthic communities (Hallock et al., 2010; Locker et al., 2016), which contribute to a multi-billion dollar recreational and commercial fishing industry (Okey et al., 2004; Colella et al., 2008; Lirman, 2013).
These hardbottom habitats are shallow (<20 m), with generally turbid waters, and support low diversity mixtures of ahermatypic eurytopic taxa of stony corals (Cladocora arbuscula, Siderastrea spp., and Oculina spp.), octocorals, macroalgae, and sponges (Jaap, 1984; Phillips et al., 1990; Walker et al., 2008; Lirman, 2013). They are classified as essential fish habitat by the NOAA Fisheries serving as nursery and foraging grounds for economically and commercially important species (e.g., grouper, gray snapper, and hogfish) (Jaap, 1984; Simon and Mahadevan, 1985; Rice and Hunter, 1992; Thompson et al., 1999; Saul et al., 2013; Coleman et al., 2014; Jaap et al., 2014).
West Florida shelf benthic communities tolerate many local stressors that affect the distribution of marine species at various spatial and temporal scales and make corals more susceptible to paling, bleaching, and disease (Dupont and Coy, 2008; Anderson, 2009; Asis et al., 2017). A few of the common stressors are water temperature, hurricane and storm surges, river discharge, and harmful algal blooms (HABs).
Coastal water temperatures on the WFS vary from 18 to 30°C, but can drop below 10°C (Mallinson et al., 2014; Locker et al., 2016; Klaus et al., 2017), which can increase stress and cause mortality events (Klaus et al., 2017; Overstreet and Hawkins, 2017).
The WFS is typically a low energy environment with tidal ranges <1 m, however, it is frequently affected by hurricane and tropical storm surges (Hallock et al., 2010; Locker et al., 2016). Hurricanes and storm surges can cause large shifts in sediment that can smother the local biota including corals, gorgonians and sponges (Anderson, 2009), or expose areas previously covered in sediment. These natural events lead to spatial shifts in distribution and succession of benthic communities and fragmentation of benthic habitats (Briones, 2004).
Proximity of benthic communities to rivers, estuaries, and bays influences abundance, biomass, and community composition of the benthos by many environmental factors including salinity, turbidity, temperature, and nutrients (Briones, 2004). Excessive flow of nutrients can arise from major river systems and impact the water quality which can exacerbate HABs (Briones, 2004; Colella et al., 2008) and may cause large-scale community shifts depending on their intensity and frequency (Overstreet and Hawkins, 2017). Over the last few decades, HABs have affected coastal communities across increasing geographic areas, threatening the health of humans and causing large-scale mortality of marine organisms, including benthic communities (Colella et al., 2008; Anderson, 2009). The nearshore oligotrophic waters of the WFS are defined by a long residence time increasing their susceptibility to stratification that can favor development of phytoplankton blooms (Nuttle and Fletcher, 2013; Weisberg et al., 2016). More than 40 species of toxic microalgae live naturally in these waters at low concentrations. While red tides or algal blooms are a natural phenomenon, they can be exacerbated by human activities, pollution, and heavy outflow of major river systems into the Gulf (Anderson, 2009; Overstreet and Hawkins, 2017). The most common HABs in the Gulf of Mexico consist of the dinoflagellate Karenia brevis, and have occurred nearly annually on the west Florida coast since the mid-1980s. When concentrations of K. brevis are high, brevetoxin, a compound within the dinoflagellate, can cause massive fish kills, paralytic shellfish poisoning, and if persistent, large-scale mortality of marine organisms (Colella et al., 2008).
There is a climate gradient along the Florida peninsula ranging from warm-temperate in the north to sub-tropical in the south, causing evident latitudinal changes in marine hardbottom communities (Engle and Summers, 2000; Spalding et al., 2007; Walker et al., 2008; Canning-Clode, 2009; Walker and Gilliam, 2013; Toonen et al., 2016). Three marine biogeographic ecoregions have been identified along Florida’s coast as described in “Marine Ecoregions of the World”; a hierarchical system based on taxonomic configurations, influenced by evolutionary history, patterns of dispersal, and isolation (Spalding et al., 2007) (Figure 1). This volume identified a split between the Northern Gulf of Mexico and Floridian ecoregions just north of Tampa Bay. Similarly, the Environmental Monitoring and Assessment Program for Estuaries (EMAP-E) used climate and ocean currents to delineate Florida into Carolinian (Virginia to St. Lucie Inlet, FL, United States), West Indian (St. Lucie/Jupiter Inlet to Tampa Bay, FL, United States), and Louisianian provinces (Tampa Bay, FL, United States through the Texas border) (Engle and Summers, 2000). The West Indian and Louisianian provinces split the northern Gulf of Mexico inhabiting warm-temperate waters just north of Tampa Bay, FL from the more tropical waters of the southern WFS (Engle and Summers, 2000).
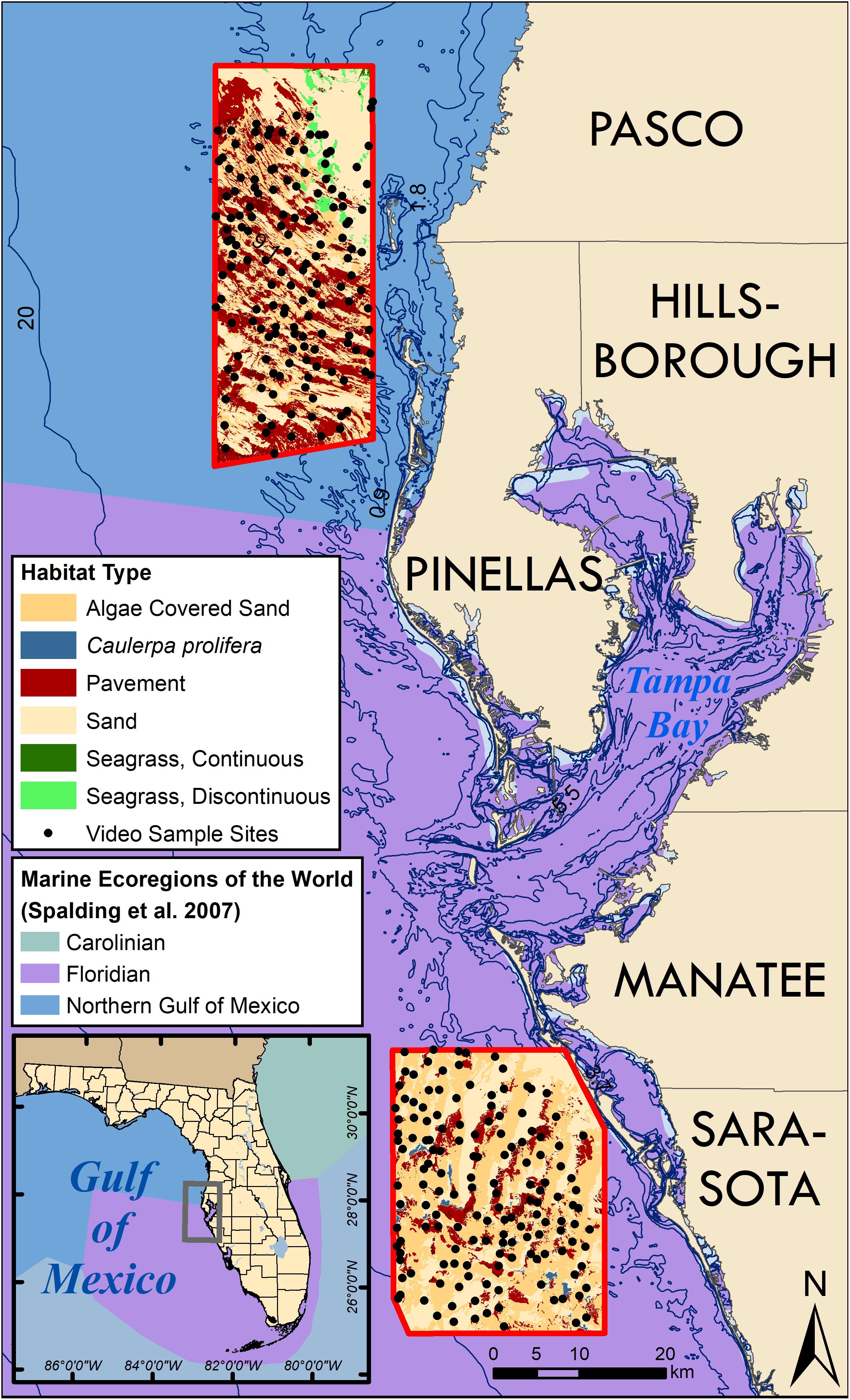
Figure 1. A map of west coastal Florida near Tampa Bay illustrating the benthic habitat maps (bordered in red) where groundtruthing video was collected to confirm habitat type (black dots). Adjacent counties are labeled. Ocean colors on main map and inset (lower left) represent the Marine ecosystems of the World from Spalding et al. (2007). The transition between Florida and Northern Gulf of Mexico is located just north of Tampa Bay.
However, there is inconsistency as to where biogeographic transitions are located. Many coastal and shallow habitats base these boundaries on taxonomic distributions and percent endemism within a geographic boundary (Briggs and Bowen, 2012). Boundaries are often fuzzy and vary with different seasonal temperatures and are typically defined by fish distributions due to unavailable or incomplete benthic community data (Engle and Summers, 2000; Toonen et al., 2016). On the WFS, benthic communities shift over a 6.5° geographic range from the Florida Keys to northern Florida (Hine and Locker, 2011), but the location of these shifts are not quantified or well-defined. Previous studies have suggested a break in biogeographic regions at Tampa Bay, south at Cape Romano, and north at Cedar Key (Engle and Summers, 2000; Toonen et al., 2016). Other studies suggest that the west central coast resides in a second part of the Carolinian province (Briggs and Bowen, 2012; Toonen et al., 2016). These are important to identify now more than ever. As the climate continues to warm, many have proposed the poleward tropicalization of temperate environments (Verges et al., 2014) and in some cases, these are occurring now (Yamano et al., 2011). Community biogeographic baseline data are essential in order to detect tropicalization effects.
Benthic habitat maps can be used to define reef community biogeography (Walker, 2012; Fisco, 2016; Ames, 2017). Habitat mapping of the Florida Reef Tract (FRT) on Florida’s Atlantic coast has provided accurate maps and habitat characterization for 955 km2 of shallow (<40 m) seafloor. Quantitative analyses of the community and habitat data supported the designation of seven distinct ecoregions over 10’s of km spatial scale identifying biogeographic boundaries in southeast Florida (Walker, 2012; Walker and Gilliam, 2013; Walker and Klug, 2014). There was an overall reduction in community diversity from south to the north changing significantly at the Bahamas Fracture Zone (BFZ) that functions as a divide of sub-tropical and temperate waters on the SE FRT (Walker and Gilliam, 2013). Habitat mapping on the WFS could be used similarly if it existed.
While latitudinal variations in benthic habitats (Walker, 2012), benthic communities (Walker and Gilliam, 2013; Klug, 2015), and reef fish (Fisco, 2016; Ames, 2017) support the definition of separate coral reef ecosystem ecoregions on the FRT, the WFS remains understudied and benthic marine community spatial differences have yet to be quantitatively investigated. Thus, the goal of this study was to map and investigate the WFS marine hardbottom north (Pasco and Pinellas counties) and south (Sarasota County) of Tampa Bay, FL to identify differences in benthic communities and the potential for ecoregion categorization. We identify latitudinal changes in the hardbottom community composition and where those changes occur. Our objectives were to (1) construct a benthic habitat map of approximately 1,200 km2 of shallow-water seafloor north and south of Tampa Bay, (2) collect quantitative survey data to characterize hardbottom benthic communities across the map, and (3) investigate coastal benthic community spatial patterns in a biogeographic context. The outputs of this work provide the first detailed benthic habitat map of the area, a detailed survey of the composition of hardbottom benthic communities in the region, identify Tampa Bay as a coastal benthic biogeographic transition, and illustrate the influence of the BFZ on coastal communities.
Materials and Methods
Benthic Habitat Mapping
Archived WorldView-2 (WV2) and QuickBird (QB) source imagery taken between December 2nd, 2011 and June 27th, 2016 of optically shallow water areas (0–20 m) were used for benthic habitat mapping. Study areas (Figure 1) were chosen based on locations where archived satellite imagery was suitable for visual image interpretation (mapping). Detailed habitat mapping of the imagery was accomplished by visual interpretation at a 1:3,000 scale with a minimum mapping unit of 0.4 ha (1 acre) following similar methodologies used by other regional mapping efforts (Walker et al., 2008; Walker and Gilliam, 2013). Features were classified to the Florida Unified Reef Map following the Federal Coastal and Marine Ecological Classification Standard (CMECS). CMECS was used for habitat categorization and was modified where necessary to better define habitats. Groundtruth video data were collected at 258 locations throughout the study area in August 2016 and March 2017 using drop cameras to visualize and determine habitat type in each location. The video data were used to inform the image interpretation process in GIS.
Community Composition Data Collection
Mapped hardbottom habitats were sampled to determine benthic community composition and its present condition. Twenty-nine haphazardly chosen, shallow-water (<20 m) hardbottom quantitative survey sites (Figures 2, 3) were visited over a 6-day period between July 17 and 22, 2017; 15 in the northern mapped area and nine in the south. The mapping attempted to distinguish high biological covered pavement by delineating the darkest portions of the habitat in the imagery separately, assuming the dark coloration was from denser cover. Thus, one pavement and one pavement-high cover (biological) site were haphazardly selected near randomly chosen map locations, utilizing a random design but also allowed comparison between the two cover types at each location.
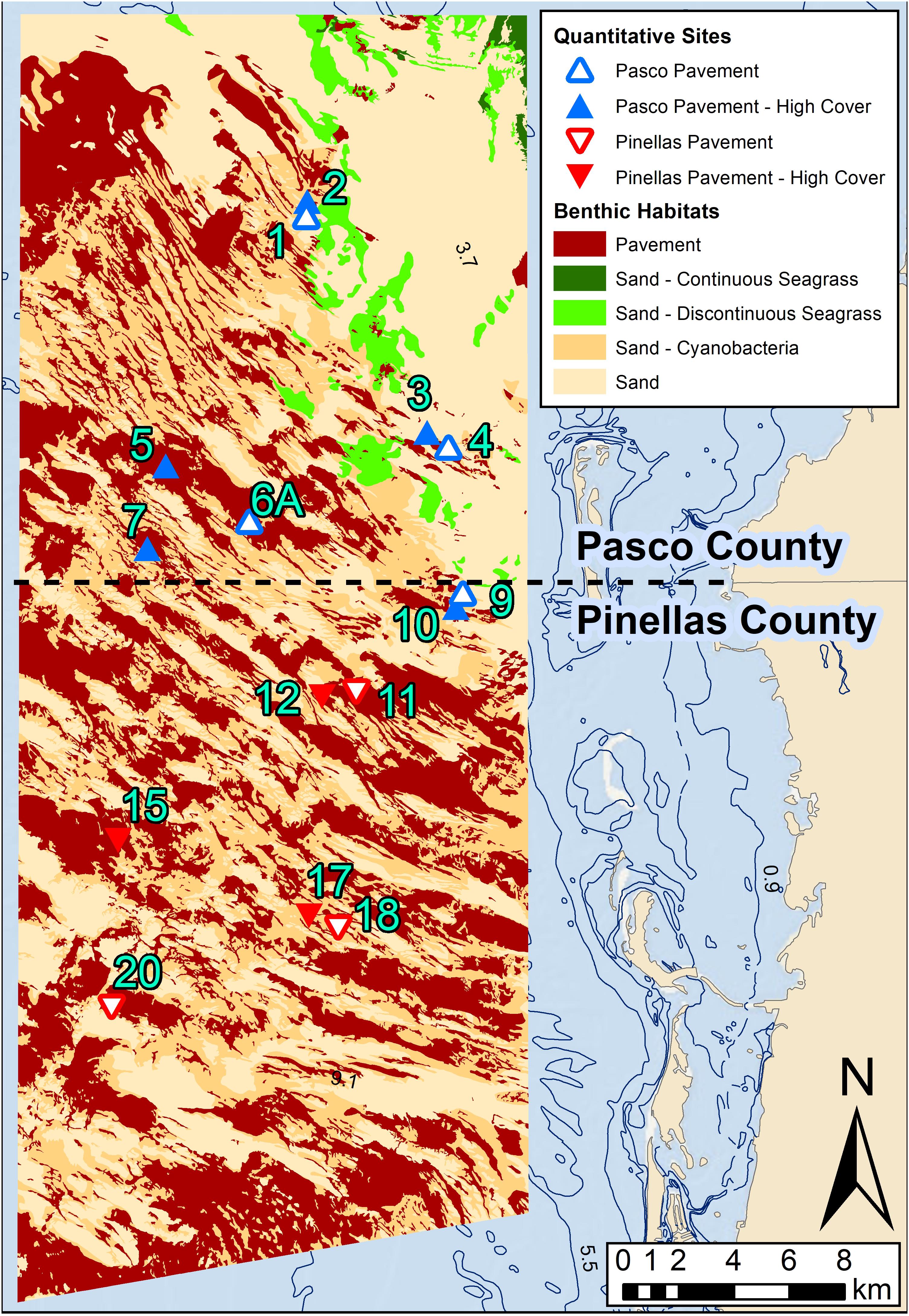
Figure 2. The northern mapped area off Pasco and Pinellas counties. The quantitative sampling site locations are coded by County and habitat cover that match the legend in the MDS plot (Figure 4). Analyses showed no significant dissimilarities between pavement and pavement-high cover so these habitats were combined to pavement in the map.
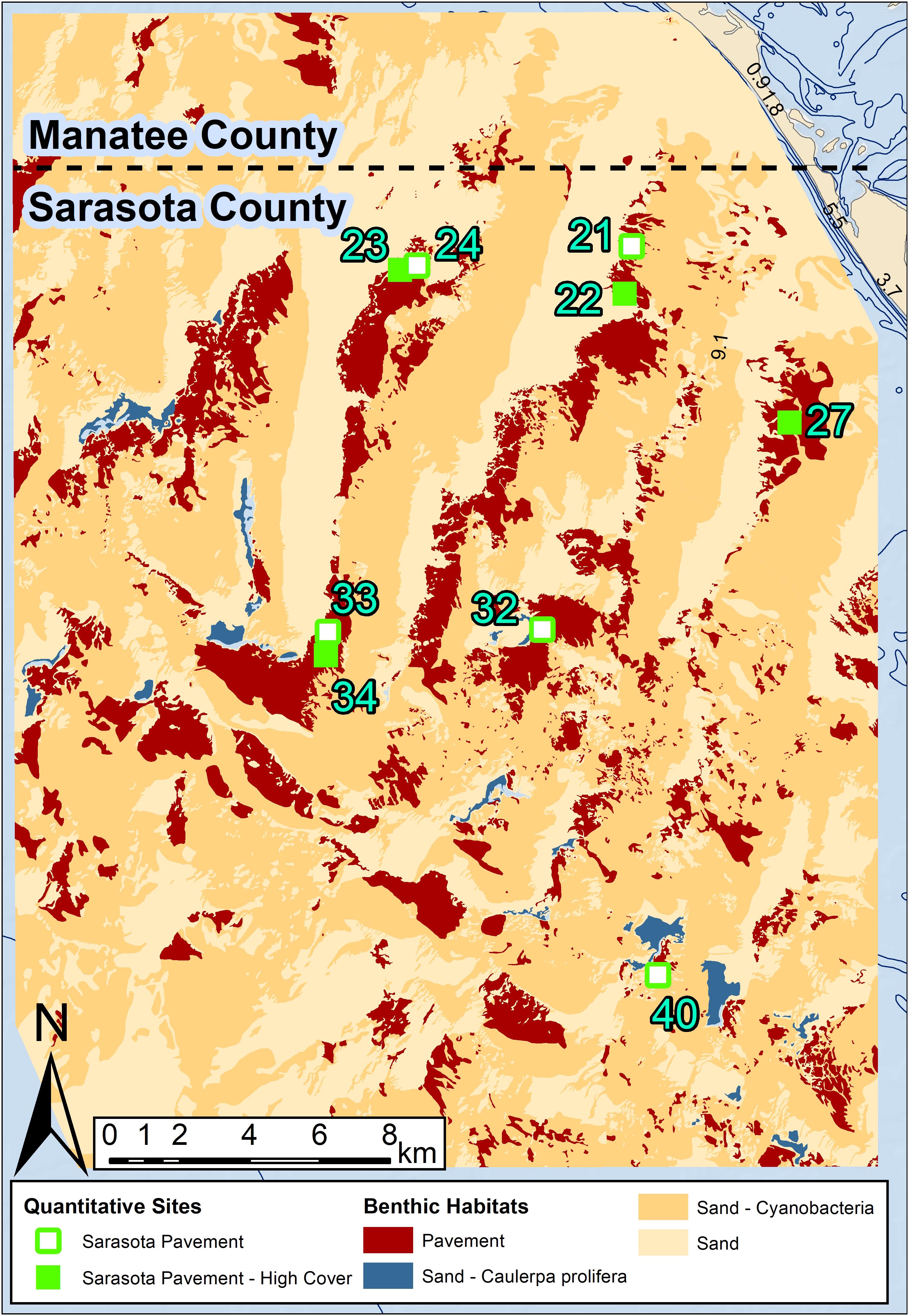
Figure 3. The southern mapped area off Sarasota County. The quantitative sampling site locations coded are coded by County and habitat cover that match the legend in the MDS plot (Figure 4). Analyses showed no significant dissimilarities between pavement and pavement-high cover so these habitats were combined to pavement in the map.
At each site, four non-overlapping 20 m transect tapes parallel to each other were extended without crossing into a different habitat. Photographs were collected 52 cm above the surface at 1-m intervals along the length of each transect. Simultaneously, a 1-m belt survey was conducted along 15 m of the two middle transects (30 m2 total) to document coral demographic and condition data. Along each of the middle transects, scleractinian corals greater than 4 cm were identified, counted, and measured (max length, width, and height) to calculate density. Gorgonian coral density was binned into height size classes (4–10, 11–25, 26–50, 50+ cm) and by individual morpho-type (Rod, Plume, and Fan). Percent mortality and the presence and severity of bleaching and disease were documented for each coral surveyed. Bleaching and disease prevalence were tabulated by the number of affected colonies divided by the total number of colonies.
Percent cover was estimated from the transect photographs using Coral Point Count with excel extensions (CPCe) software (Kohler and Gill, 2006). Twenty randomly placed points were assigned on each image where the substrate and organisms were identified to functional groups using a specially designed source code with known shallow WFS species. Algae were identified to their most general taxonomic level (i.e., Red algae, Brown Algae, and Green Algae), and corals were identified to their lowest taxonomic level (i.e., family and species).
Statistical analyses of the benthic community data included non-parametric analysis of variance (ANOVA) to examine differences in major functional group cover, abundances, species richness, number of diseased colonies, percent live tissue, and percent of recent dead. Non-metric, multidimensional scaling (MDS) plots were constructed using Bray-Curtis similarity indices to examine differences in community structure (PRIMER v6). An analysis of similarity (ANOSIM) test was used to identify differences in community structure, and similarity percentage (SIMPER) analysis was used to identify those species most responsible for the differences seen among different factor groups. All data underwent a thorough process of quality assurance and quality control before and after being compiled and statistically analyzed. Datasheets were reviewed for consistency and clarification. Any outlier species identifications or abundance/sizes etc. were reviewed and changed when appropriate.
Results
Satellite Image Mapping
A total mapped area of 1,263.34 km2 total seafloor indicated 23.4% (295.89 km2) of the surveyed cover was pavement and 76.6% (967.45 km2) was sand. Pavement was originally divided into two categories pavement, and pavement-high cover (biological), but the similarity between pavement and pavement-high cover sites suggested that the map did not distinguish pavement by cover very well. Therefore, we combined these classes into one class called pavement. Sand was categorized into several categories based on biological cover. Of the total mapped area, 40% (505.51 km2) was sand covered with cyanobacteria, while 34.6% (437.25 km2) consisted of bare sand, 0.4% (4.56 km2) was sand with Caulerpa prolifera, and 1.6% (20.13 km2) was sand with seagrass (Table 1).
Habitat distribution differed between the north and south study areas (Table 1). The northern study area contained 72.9% (215.77 km2) of the total pavement, 100% (20.13 km2) of continuous and discontinuous seagrass, and 37.3% (188.56 km2) of sand-cyanobacteria (Figure 2). The southern study area contained 27.1% (80.12 km2) of the pavement, 100% (4.56 km2) of the Ca. prolifera, and 62.7% (316.95 km2) of the sand-cyanobacteria (Figure 3).
The habitats also varied within each mapped area (Table 1). The northern area was comprised of 33.5% pavement, 34% (218.77 km2) sand, 3.1% continuous and discontinuous seagrass, and 29.3% sand-cyanobacteria. The southern area was comprised of 12.9% pavement, 35.2% (218.48 km2) sand, 0.7% sand-Ca. prolifera, and 51.1% sand-cyanobacteria.
Benthic Communities
Stony Corals
Florida’s west coast hardbottom shelf contains a vast and dense community of ahermatypic stony corals, albeit less diverse than further south. A total of 4,079 stony coral colonies were identified and measured in this study (Table 2). Ten species were identified, however, four species [Siderastrea spp. (42.46%), Oculina robusta (28.61%), Solenastrea hyades (21.52%), and Cl. arbuscula (6.33%)] comprised 99.92% of the total stony corals measured in this study. Total mean density of stony corals (colony m–2) was high, 11.56 corals m–2, compared to a mean density of 6.68 ± 0.35 colonies m–2 on the SE FRT (Gilliam et al., 2017). Sarasota County had the highest mean density of corals (18.4 m–2), where Pasco County had the lowest mean density (5.18 m–2) and Pinellas County had a moderate mean density (11.42 m–2). Siderastrea spp. had the highest density (4.90 m–2) followed by O. robusta (3.30 m–2), So. hyades (2.48 m–2), and Cl. arbuscula (0.73 m–2) (Table 2).
No large tropical reef-building corals were found in this study. Stony corals were found in very high densities but consisted of small individuals (<40 cm). Seventy-three percent of coral colonies were less than 10 cm long and 80% of these corals were less than 10 cm tall. There was a mean coral size in Sarasota County of 9.12 cm (± 0.32 SE) length, 7.83 cm (± 0.85 SE) width, and 7.98 cm (± 1.16 SE) height. The mean coral size in Pinellas County was 5.42 cm (± 0.14 SE) length, 3.92 cm (± 0.14 SE) width, and 3.75 cm (± 0.16 SE) height. The mean coral size in Pasco County was 7.95 cm (± 0.51 SE) length, 6.54 cm (± 0.47 SE) width, and 5.93 cm (± 0.35 SE) height. The fire coral species Millepora complanata had the largest length, width, and height of the species surveyed (>30 cm) due to its encrusting nature.
Out of the ten coral species documented, only four species (Siderastrea spp., O. robusta, So. hyades, and Cl. arbuscula) showed signs of disease, paling, partial bleaching, or bleaching, totaling 838 of 4,079 individuals (Table 3). This equated to 19% of the corals exhibiting at least some stress condition, however this varied dramatically by species. O. robusta had a 51.8% bleaching prevalence (combining partially and totally bleached) whereas 17.1% of So. hyades, 2.3% of Cl. arbuscula, and 0.8% of Siderastrea spp. were bleached. Over 85% of bleaching occurred on O. robusta (586) and So. hyades (129). These two-species had the highest amount of paling, partial bleaching, and bleaching documented. Total disease prevalence was extremely low (0.1%) and was documented in only two species, Siderastrea spp. (0.23%) and So. hyades (0.23%). The type of disease was not identified or confirmed.

Table 3. Condition (bleaching and disease) of the stony corals observed in the benthic quadrat surveys with bleaching and disease prevalence by species.
Gorgonians
Gorgonians were very abundant, with a total count of 1,918 colonies (Table 4). Total mean density of gorgonians was 5.43 ± 0.46 SE m–2. Sarasota County had the highest mean density of gorgonians (12.13 ± 7.1 SE m–2), Pasco County had the second highest mean density of gorgonians (2.01 ± 0.42 SE m–2), and Pinellas had the lowest mean density of gorgonians (0.12 ± 0.21 SE m–2). The dominant morpho-type was rods, which comprised 67.2% of the gorgonians counted. These were dominated by two size classes; Rods 11–25 cm and Rods 26–50 cm. Plumes comprised 32.8% of the gorgonian individuals recorded and were dominated by Plume 26–50 cm and Plume 50+ cm size classes. Only one Fan 26–50 cm was recorded. No bleaching or diseased gorgonians were found.
Community Spatial Patterns
Region
The community analyses suggest that the benthic communities in the south (Sarasota County) were very different from the north sites (Pinellas and Pasco counties). The average dissimilarity between the Northern and Southern sites was 53.16% and an ANOSIM test was significant (p = 0.1, R = 0.431). Differences between regions were driven by stony coral and gorgonian metrics. Mean density of So. hyades (N: 0.80 ± 0.19 SE, S: 5.21 ± 0.94 SE) and Siderastrea spp. (N: 3.20 ± 0.67 SE, S: 7.84 ± 1.47 SE) were significantly different between Northern and Southern sites (SHYA: ANOVA, p = 0.001; SIDspp: ANOVA, p = 0.006).
Regional differences between gorgonian morpho-type and colony size classes were observed. Mean density of Rod morpho-type was significantly different between the size class 4–10 cm (N: 0.12 ± 0.03 SE, S: 0.85 ± 0.48 SE) (ANOVA, p-value = 0.027) and 50+ cm (N: 0.09 ± 0.02, S: 1.33 ± 1.07 SE) (ANOVA, p = 0.009). Plume morpho-type mean density was significantly different between regions for size classes 4–10 cm (ANOVA, p < 0.001) and 11–25 (ANOVA, p < 0.001). South sites had a significantly higher mean density of Plume 26–50 cm (1.47 ± 1.19 SE) than the North (0.01 ± 0.01 SE) (ANOVA, p = 0.003).
There were few significant differences in percent cover of biota between the North and South. Hardbottom veneered with sediment cover was higher in the north (N: 43.84%; S: 20.65%) (ANOVA, p = 0.009) and exposed Hardbottom cover was higher in the south (N: 19.27%; S: 39.53%) (ANOVA, p = 0.023). Brown Algae cover was significantly higher in the north (N: 10.97%; S: 2.25%) (ANOVA, p < 0.001) and Plume gorgonians (N: 0.24%; S: 3.30%) were significantly higher in the south (ANOVA, p = 0.013).
County
Multivariate analyses of density data (stony coral species and gorgonian morpho-type by size class) showed distinct spatial patterns among counties (Figure 4). The northern most sites in Pasco County (blue triangles in Figure 4) were 64.7% similar, Pinellas County (red triangles) sites were 65.6% similar, and the southern Sarasota sites (green squares) were 54.1% similar; all clustering in groups and in spatial order. Pasco and Pinellas had an average dissimilarity of 42.5%. Pasco and Sarasota had an average dissimilarity of 54.5%. Pinellas and Sarasota sites had an average dissimilarity of 51.2%. An ANOSIM statistically supported the County differences in benthic community density with the strongest difference between Pasco and Sarasota sites (R = 0.512). A 3-dimensional bootstrap averages plot clearly illustrates the statistical dissimilarity in coral densities on pavement sites between counties (Figure 5).
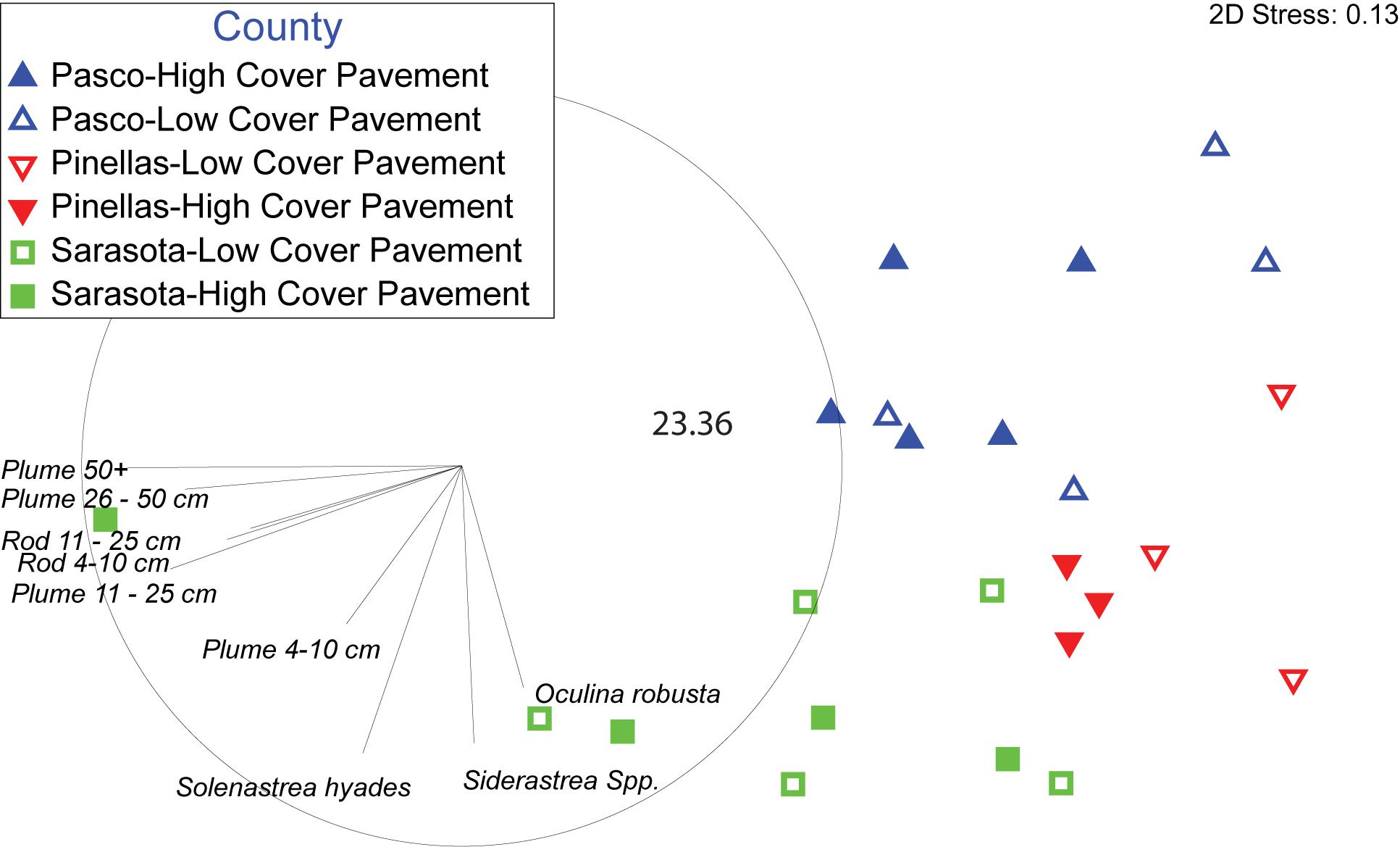
Figure 4. Non-metric multidimensional scaling plot of Bray-Curtis similarities of coral species and gorgonian morpho-type by size class densities by site. Sites are coded by County and initial map cover categories. Map cover categories were combined to pavement due to a lack of dissimilarity between high and low cover sites. The inside circle represents a Pearson correlation of the direction of influence different taxa had on the plot.
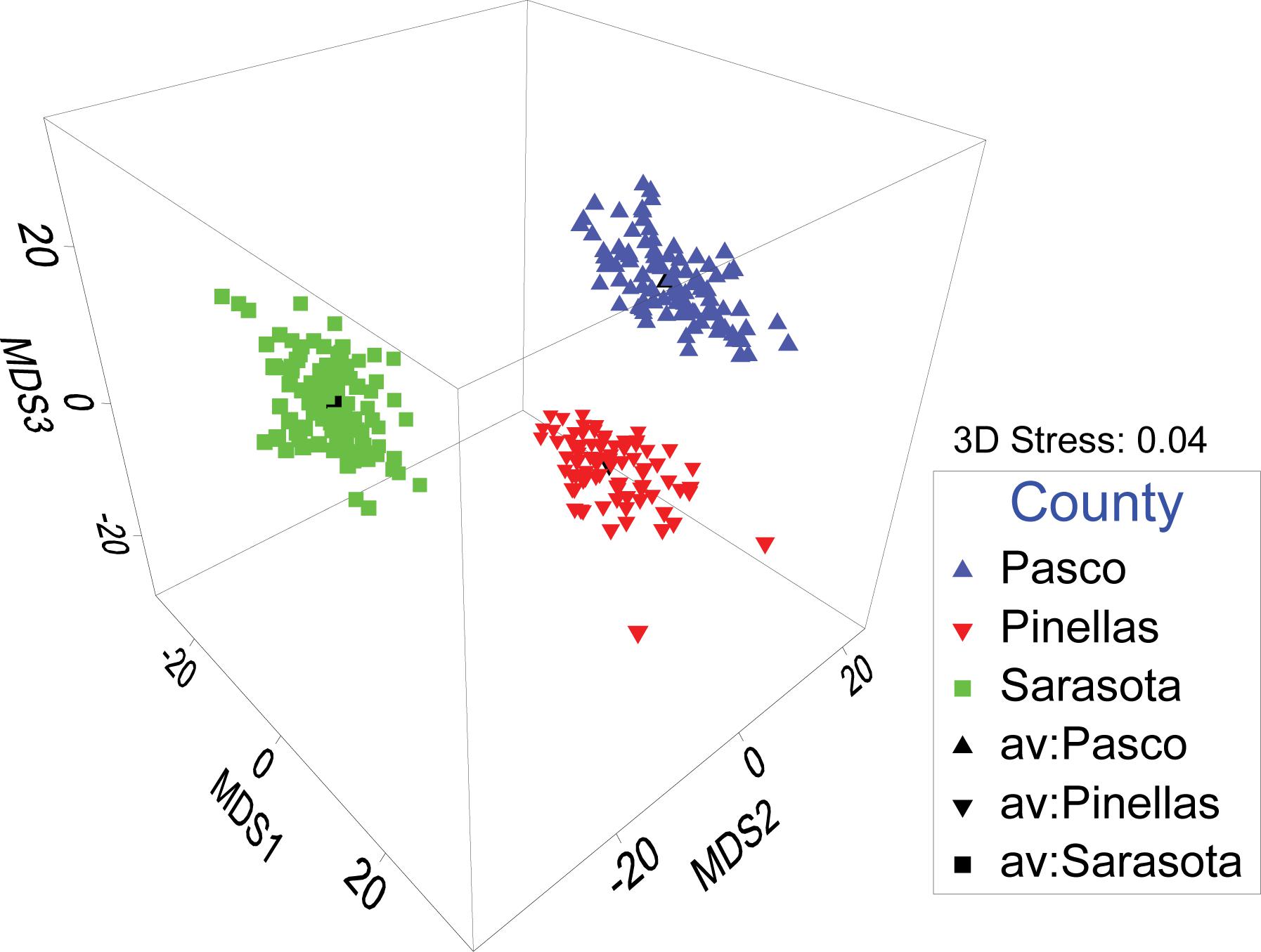
Figure 5. A 3-dimensional bootstrap averages plot of all site organism density categorized by County. Separate distinct clusters indicate each county has distinct benthic coral and gorgonian communities.
A Pearson correlation overlaying the MDS showed the major contributors of the spatial patterns in the data (Figure 4). Mean stony coral density increased significantly by County from north to south with the lowest in Pasco County sites (5.18 ± 0.23 SE m–2), then Pinellas County sites (11.42 ± 0.60 SE m–2), and Sarasota County sites (18.4 ± 0.86 SE m–2) (Figure 6). Differences were mostly driven by Siderastrea spp., O. robusta, and So. hyades (Figure 7). The mean density of Siderastrea spp. was 1.76 (± 0.38 SE m–2) in Pasco sites, 5.35 (± 1.10 SE m–2) in Pinellas, and 7.84 (± 1.50 SE m–2) in Sarasota sites (ANOVA, p-value = 0.012). The mean density of So. hyades was 0.67 (± 0.16 SE m–2) in Pasco sites, 1.00 (± 040 SE m–2) in Pinellas sites, and 5.21 (± 0.94 SE m–2) in Sarasota sites (ANOVA, p-value = 0.005). Sarasota County sites had the highest densities of all stony corals except Cl. arbuscula, which was higher in both Pasco and Pinellas sites.
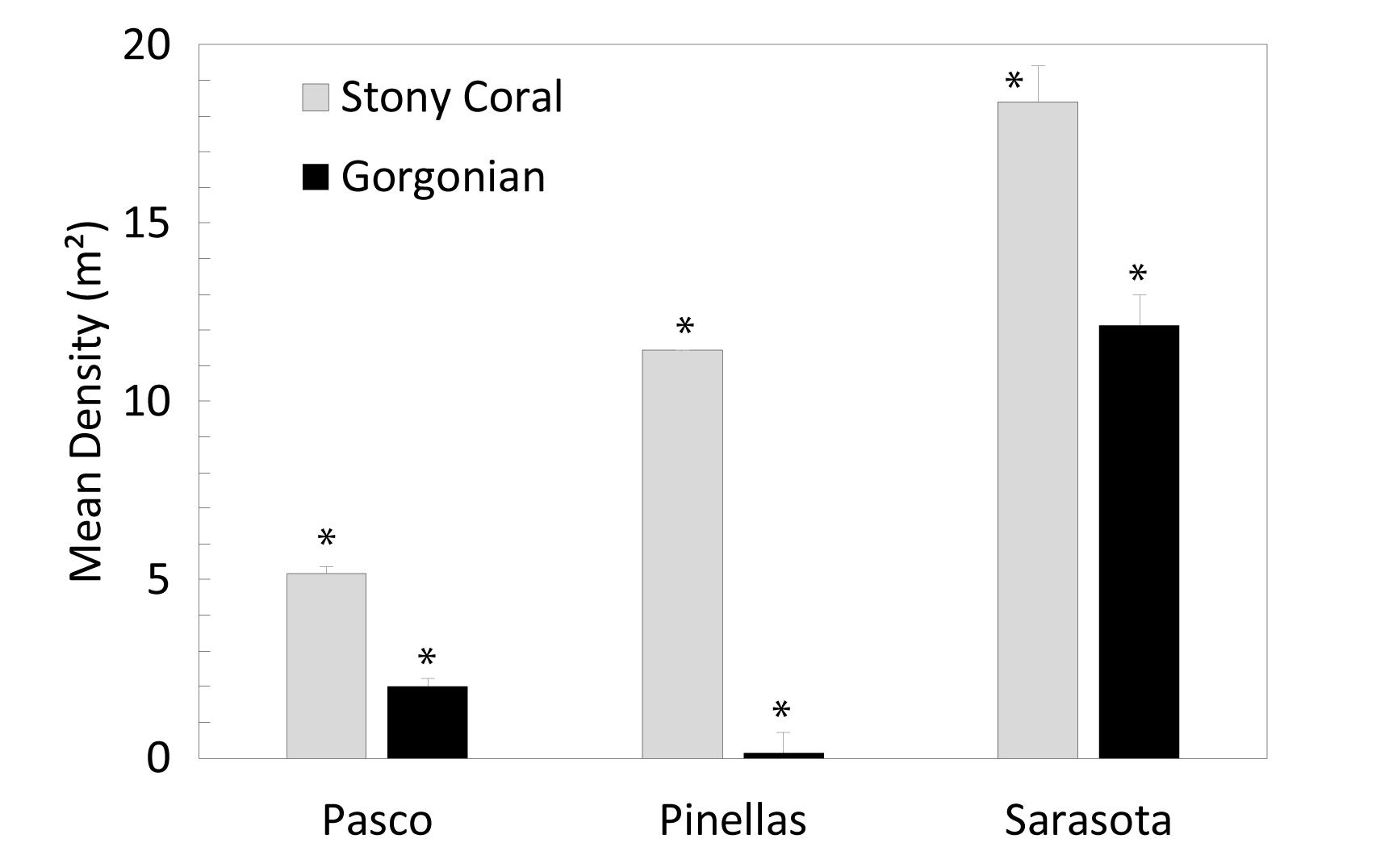
Figure 6. Mean (±SE) stony coral density and gorgonian density (m–2) differences between counties. Asterisks represent significant differences between county.
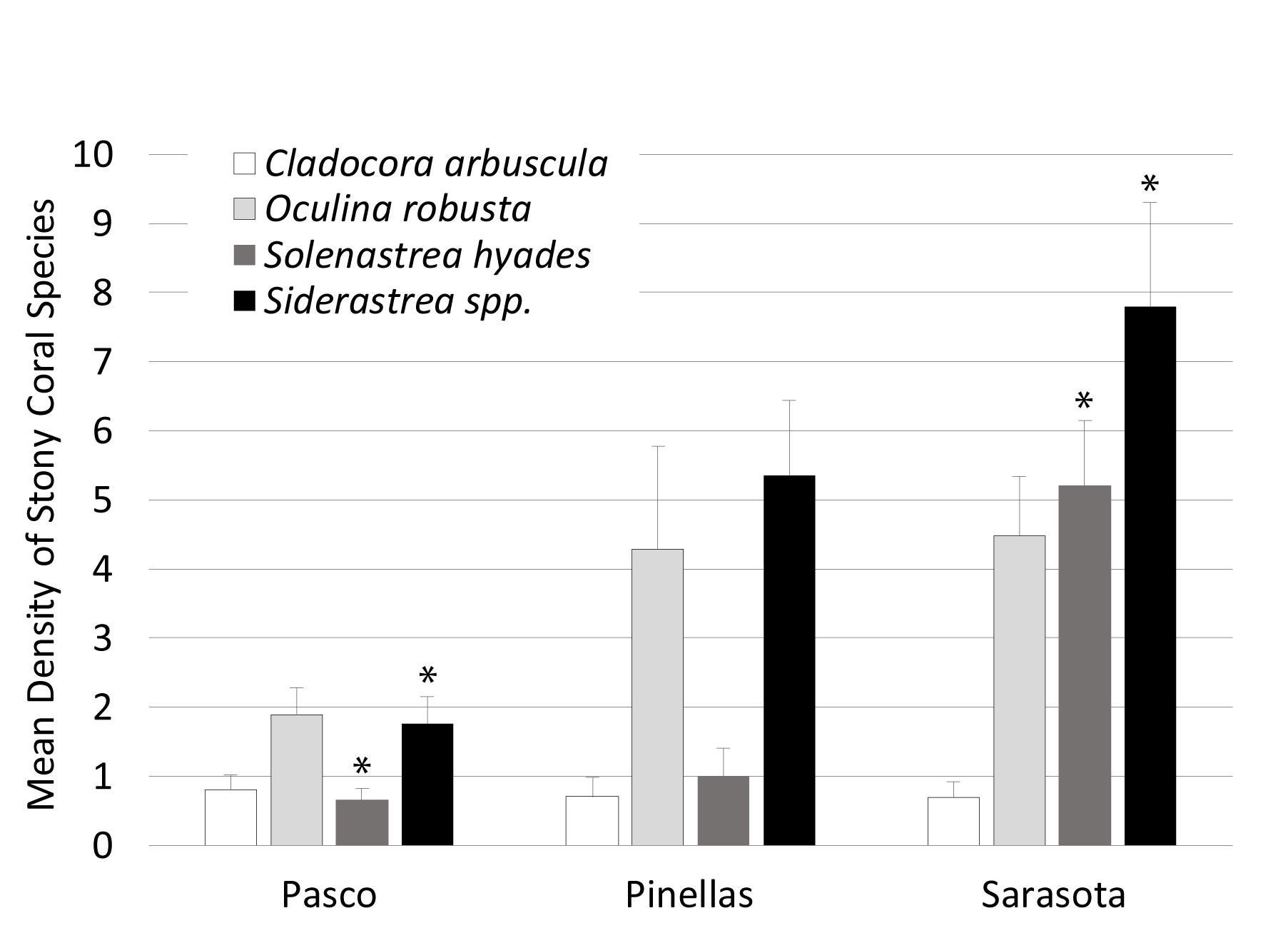
Figure 7. Mean (±SE) stony coral species density differences between counties. Asterisks represent significant differences between county.
Gorgonian densities varied between counties as well (Figure 6). Sarasota had the highest total mean gorgonian density (12.13 ± 1.00 SE m–2) (ANOVA, p = 0.006). Pasco had the second highest mean density (2.01 ± 0.18 SE m–2) and Pinellas had the lowest (0.13 ± 0.02 SE m–2). Gorgonian density in Sarasota was comparable to the mean density in the FL Keys (12.96 ± 0.59 SE colonies m–2) (Smith et al., 2011).
Most of the recorded gorgonians were greater than 10 cm (84.6%). Sarasota had significantly higher (ANOVA, p = 0.014) density of gorgonians greater than 10 cm (10.06 ± 4.1 SE m–2) than Pinellas County (0.13 ± 0.05 SE m–2) and higher densities of gorgonians less than 10 cm (2.07 ± 0.05 SE m–2) than Pinellas (ANOVA, p = 0.004).
County differences in percent cover were most pronounced in Hardbottom with sand veneer, Brown Algae, and So. hyades. Mean Hardbottom with a sand veneer cover was significantly higher (ANOVA, p = 0.03) in Pasco County (45.77% ± 6.22 SE) than Sarasota County (20.65% ± 9.67 SE). Brown algae cover was significantly higher (ANOVA, p = 0.003) in Pasco (11.03% ± 2.35 SE) and Pinellas (10.88% ± 10.65 SE) than in Sarasota County (2.25% ± 0.62 SE). So. hyades cover was significantly lower (ANOVA, p = 0.005) in Pasco County (0.08% ± 0.04 SE) than Sarasota County (1.13% ± 0.30 SE).
Discussion
Analyses of shallow-water (< 20 m) habitat mapping and benthic community data near the mouth of Tampa Bay indicate a significant shift in marine benthic communities along the WFS. This change is marked by significant pole-ward decreases in stony coral density and gorgonian density and cover. The habitat mapping showed clear differences in habitat type and coverage between regions with more mapped pavement area in the northern region, however, the percent cover data indicated much of this was Hardbottom veneered with sediment. Although there was less pavement in the south, the southern sites had less hardbottom veneered with sediment, and higher coral and gorgonian density and cover. The gap in our mapping and assessments across the mouth of Tampa Bay precluded the ability to specifically identify where these changes occur. Ecoregion boundaries usually vary by the species or the habitat being investigated, and the boundaries do not always change suddenly over a short distance (Engle and Summers, 2000; Toonen et al., 2016). Some may have a broader transition where mobile and short-lived species can vary with seasons as temperature is often a lead determinant of species distributions (Topp and Hoff, 1972; Engle and Summers, 2000; Toonen et al., 2016). As global and local stressors become more prominent, this variability will add to the fuzziness of species boundaries (Briones, 2004; Spalding et al., 2007; Makowski and Keyes, 2011). Thus, this entire area may be part of a larger ecological transition zone (Figure 8). Completing the mapping and conducting assessments in this gap will help identify the best transition location.
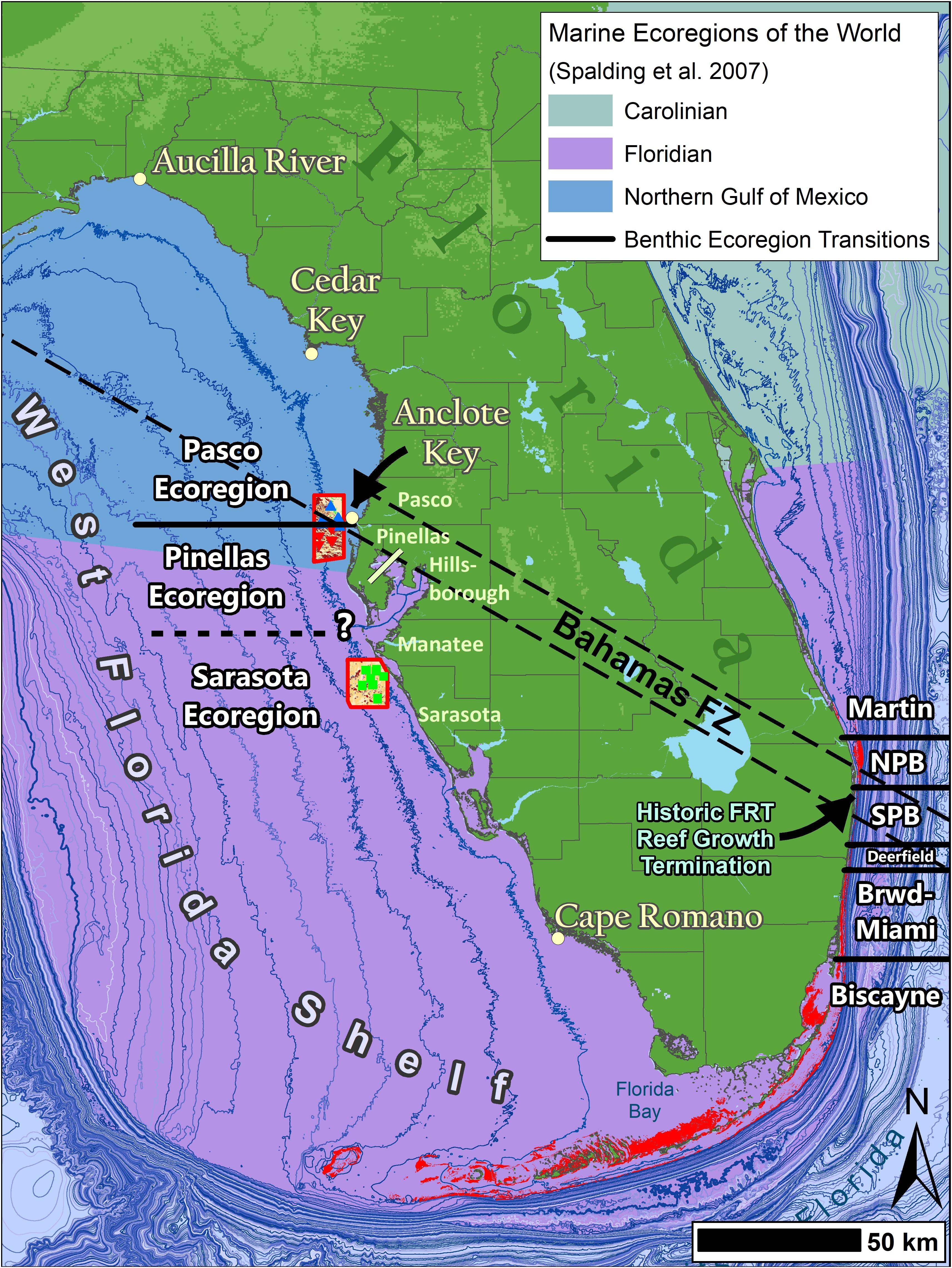
Figure 8. A map of Florida coastal benthic ecoregions overlaying the Marine Ecoregions of the World (Spalding et al., 2007) and the approximate location of the Bahamas Fracture Zone (BFZ) taken from Klitgord et al. (1984). The east coast illustrates the regions from Walker and Gilliam (2013). The west coast are results from this study. There are benthic community shifts along both coasts at the BFZ. On the west coast there is one near the Pinellas and Pasco Countyline and a more pronounced one somewhere between Pinellas and Sarasota counties near Tampa Bay.
Temperature is one of the most important factors affecting WFS coral distributions and ranges as well as a limiting factor for gorgonian and algal cover (Hedgpeth, 1954; Engle and Summers, 1999; Colella et al., 2008; Anderson, 2009; Hale, 2010; Mallinson et al., 2014; Jaap, 2015; Locker et al., 2016; Klaus et al., 2017). The southern WFS is warmer and subjected to less seasonal variability (Engle and Summers, 2000) and studies around Tampa Bay show distinct changes in water movement that latitudinally affect water temperatures (Hine and Locker, 2011; Jaap, 2015; Weisberg et al., 2016). Smith (1954) identified the 21°C isotherm in January, located at the Pinellas County line just north of Tampa Bay as stated by Fuglister’s (1947) atlas of oceanic temperatures, to be the northern limit of coral reefs in the Gulf (Glynn, 1973). The minimum average monthly sea surface temperatures show tight isotherms changing from 20.5 to 18.3°C between Tampa Bay to Hernando Beach. Work (1969) described this area Anclote Key to Aucilla River (north of the 21°C isotherm) as an “extraordinary faunal area” due to the “paradoxically richer number of both Carolinian and West Indian species.” Cladocora, Manicina, lsophyllia, Scolymia, Siderastrea, and Oculina corals are common throughout the area (Work, 1969). With the exception of lsophyllia and Scolymia, his description matches our results throughout our mapped area, indicating that perhaps this extraordinary fauna extends further south than previously realized.
Interestingly, the location of Fuglister’s (1947) tight minimum isotherms on the WFS is also where the BFZ crosses north Tampa Bay (Figure 8) (Klitgord et al., 1984). The BFZ is an important feature in the Gulf of Mexico that extends northwest to southeast beneath central peninsular Florida separating Florida’s basement rocks (Hine et al., 2008). This zone creates coastal morphologies that influence ecological changes (Klitgord et al., 1984; Walker, 2012; Walker and Gilliam, 2013). On Florida’s East coast, the BFZ is associated with significant changes in benthic and reef fish communities and benthic habitat extent and type (Walker, 2012; Walker and Gilliam, 2013; Fisco, 2016; Ames, 2017). This is the northern range of many sub-tropical flora and fauna on the east coast. To the north of this feature, the shelf expands west and allows the Florida Current to meander creating periodic cold-water upwelling (Walker and Gilliam, 2013), which limits sub-tropical biota. There are significant reef fish and hardbottom community differences north and south of this area (Briggs, 1974; Engle and Summers, 1999; Walker, 2012; Klug, 2015; Fisco, 2016; Ames, 2017) and it separates the EMAP-E West Indian and Carolinian provinces. Thus, it is likely not just a coincidence that the BFZ is a major community transition point on the WFS.
Our results support the Marine Ecoregions of the World and the EMAP-E WFS biogeographic province that illustrate a transition just north of Tampa Bay, FL at Indian Rocks Beach (Hutchins, 1947; Ekman, 1953; Hall, 1964; Gosner, 1971; Briggs, 1974; Spalding et al., 2007). The spatial limits of our work did not allow us to identify the specific biogeographic province transition location between Pinellas and Sarasota or evaluate other ecoregions south at Cape Romano or north at Cedar Key (Engle and Summers, 2000; Toonen et al., 2016). However, our study spanned an ecoregion boundary at Anclote Key where the benthic communities are dissimilar between Pinellas and Pasco counties. This is not surprising given the tighter isotherms of minimum temperatures north of Tampa Bay (Fuglister, 1947).
Similar to Florida’s east coast, there are likely other benthic and reef fish ecoregions along the WFS. Coomans (1962) suggested that WFS faunal breaks occur based on annual surface isotherms of 25°. Previously proposed faunal boundaries of flatfishes include Apalachicola, Cedar Key, Anclote Key, Tampa Bay, Charlotte Harbor, Cape Romano, or Cape Sable (Topp and Hoff, 1972). West Florida shelf flatfish distributions were arbitrarily separated at Tampa Bay because the transition between areas is gradual with some species occurring irregularly and seasonally (Topp and Hoff, 1972). Hedgpeth (1954) and Briggs (1958) identified the southern WFS as one of the four major bottom communities of the Gulf called, “sponge grounds.” Fifty-three scleractinian species have been found in this area (Phillips et al., 1990), however, apart from the very southern extent, conditions on the WFS are unfavorable for coral reef development (Jaap, 2015). Most of the West Florida shelf supports low diversity of mostly warm-temperate ahermatypic eurytopic taxa (Jaap, 1984, 2015; Phillips et al., 1990; Lirman, 2013). There is a loss in species richness and major differences in stony coral demographics from southern to northern latitudes (Jaap, 2015; this study). Ten stony coral species were documented in this study with four dominant species that are tolerant of cold-water temperatures: Siderastrea spp., O. robusta, Cl. arbuscula, and So. hyades (Jaap, 1984; Phillips et al., 1990; Lirman, 2013).
Alcyonarian fauna also changes significantly along the west Florida shelf. Antillogorgia acerosa, A. americana, and Pterogorgia anceps are part of a decimated West Indian assemblage that occurs some distance up the west coast of Florida (Bayer, 1954). This group is merged with and then replaced by a distinctly temperate fauna dominated with Leptogorgia virgulata and L. setacea (both of which are referable, Bayer, 1952 to Verrill’s genus Eugorgia), and Muricea pendula (Bayer, 1954). This assemblage matches that of the Carolina coast (Bayer, 1954). The locations of these changes have not been identified, however, our study found distinct differences in gorgonian densities and sizes north and south of Tampa Bay.
Wide temperature variations can cause significant ecological change in the community structure (Lugo-Fernández et al., 2001; Jaap, 2015). Extended periods of warm or cold temperatures are likely to cause hermatypic coral bleaching, a stress response that can lead to mortality. Our observations were made in August in very warm water (>30°C, Rutgers Coastal Ocean Observation Lab). Three sites were not surveyed due to thick green water with zero visibility at depth. We found that 19% of all corals were at least partially bleached, the majority from two species: O. robusta (51.8%) and So. hyades (17.1%). Oculina corals are not obligate zooxanthellate corals so it is unclear how their bleaching effects their physiology, however, they are known to be dominated by Symbiodinium type B1 in the northern Gulf of Mexico (Leydet and Hellberg, 2016), which is a clade that usually dominates in temperate environments (Thornhill et al., 2008) and is more thermally sensitive than type B2 (McGinty et al., 2012). Symbiodinium type was unknown in our study, however, it is possible that the warm temperatures caused the release of a clade adapted to the normally cooler temperatures.
Although much bleaching was found, we found no disease or evidence of the stony coral tissue loss disease outbreak that was affecting over 60% of the colonies in its wake (Precht et al., 2016; Walton et al., 2018). The dynamics of coral bleaching, colony recovery and colony mortality are not known for the WFS, however, the small colony sizes indicate these colonies do not survive much more than a decade.
Local bay-specific factors (i.e., surface area or anthropogenic factors) can also affect the distribution of benthic communities (Eidens et al., 2015). Jaap (2015) identified discontinuous and patchy scleractinian corals where large estuaries and bays (such as Tampa Bay) influence salinity altering community structure. The WFS is prone to frequent, significant impact from HAB impacting the water quality and light transmission (Colella et al., 2008; Anderson, 2009). However, prior to 2005, there was only one documented case of a benthic mortality event off Pinellas and Sarasota resulting in benthic die-offs but limited stony coral mortality. The recovery process for the benthic fauna from that event is slow and continues today (Smith, 1975; Colella et al., 2008).
In summary, this study mapped a large expanse of hard bottom and identified a major shift in benthic communities around Tampa Bay, FL highlighting the necessity to continue mapping and benthic characterization on the WFS to understand the extent of the resources and identify other biogeographic or ecoregion boundaries. These areas serve as nursery and foraging grounds for economically and commercially important species (e.g., grouper, gray snapper, and hogfish) (Jaap, 1984; Simon and Mahadevan, 1985; Rice and Hunter, 1992; Thompson et al., 1999; Coleman et al., 2014; Jaap et al., 2014) whose populations are modeled for fisheries management (Saul et al., 2013). A detailed habitat map will facilitate more precise models of reef-associated species (Grüss et al., 2016; Grüss et al., 2017a, b). These findings also illustrate a need for additional research of the Gulf of Mexico benthic habitats to give a more comprehensive understanding of WFS marine habitat and benthic community biogeography in the context of future warming conditions and the potential tropicalization of the northern Gulf of Mexico. Undocumented changes in ecoregions and shift in transition locations may be presently occurring. We suggest unifying all presently available seafloor mapping data into spatial and categorical GIS databases, mapping new areas with high probability of hardbottom resources, and collecting benthic community data over broader scales to expand the biogeographic analyses and refine community biogeographic zonation. This is a necessary precursor to any long-term community monitoring to detect spatial shifts in communities and population modeling.
Data Availability Statement
The mapping data for this study can be found in GRIIDC at the following URL: https://data.gulfresearchinitiative.org/data/FL.x710.000:0001.
Author Contributions
BW, SK, and RB contributed conception and design of the study. BW and SK organized the database. CA created the map. CA, SE, and BW performed the statistical analysis. SE and BW wrote the first draft of the manuscript and sections of the manuscript. All authors contributed to manuscript revision, read, and approved the submitted version.
Funding
This project was paid for with federal funding from the Department of the Treasury under the Resources and Ecosystems Sustainability, Tourist Opportunities, and Revived Economies of the Gulf Coast States Act of 2012 (RESTORE Act) Subagreement No. FLRACEP 4710-1126-00-J. The statements, findings, conclusions, and recommendations are those of the author(s) and do not necessarily reflect the views of the U.S. Department of the Treasury.
Conflict of Interest
The authors declare that the research was conducted in the absence of any commercial or financial relationships that could be construed as a potential conflict of interest.
Acknowledgments
Thank you to the Florida Fish and Wildlife Research Institute who assisted in preparation and field work for this project including M. Garrett, R. Druyor, and R. Maloney.
References
Ames, C. (2017). Reef Fish Assemblage Biogeography Along the Florida Reef Tract. Master’s thesis, Nova Southeastern University, Dania Beach, FL.
Anderson, D. M. (2009). Approaches to monitoring, control and management of harmful algal blooms (HABs). Ocean Coast. Manag. 52, 342–347. doi: 10.1016/j.ocecoaman.2009.04.006
Asis, J., Berecibar, E., Claro, B., Alberto, F., Reed, D., Raimondi, P., et al. (2017). Major shifts at the range edge of marine forests: the combined effects of climate changes and limited dispersal. Sci. Rep. 7:44348. doi: 10.1038/srep44348
Baumstark, R., Duffey, R., and Pu, R. (2016). Mapping seagrass and colonized hard bottom in Springs Coast, Florida using WorldView-2 satellite imagery. Estuar. Coast. Shelf Sci. 181, 83–92. doi: 10.1016/j.ecss.2016.08.019
Bayer, F. M. (1952). New western Atlantic records of octocorals (Coelenterata: Anthozoa), with descriptions of three new species. J. Wash. Acad. Sci. 42:183.
Bayer, F. M. (1954). “Anthozoa: alcyonaria,” in Gulf of Mexico: Its Origin, Waters, and Marine Life, ed. P. S. Galtsoff (Washington D.C: United States Government Printing Office), 279–284.
Briggs, J. C. (1958). A list of Florida fishes and their distribution. Bull. Florida State Museum 2, 223–318.
Briggs, J. C., and Bowen, B. W. (2012). A realignment of marine biogeographic provinces with particular reference to fish distributions. J. Biogeogr. 39, 12–30. doi: 10.1111/j.1365-2699.2011.02613.x
Briones, E. E. (2004). “Current knowledge of benthic communities in the Gulf of Mexico. Environmental analysis of the Gulf of Mexico,” in Environmental Analysis of the Gulf of Mexico, eds K. Withers and M. Nippers (Corpus Christi, TX: Texas A&M University), 108–136.
Canning-Clode, J. (2009). “Latitudinal patterns of species richness in hard-bottom communities,” in Marine Hard Bottom Communities: Patterns Dynamics, Diversity and Change, ed. M. Wahl (Berlin: Springer), 81–87. doi: 10.1007/b76710_5
Colella, M., Richardson, B., Ruzicka, R., Johnson, D., Bertin, M., Callahan, M., et al. (2008). Assessment of Population and Community Structure of Sessile Macro Invertebrates Following a Benthic Mortality Event in the Eastern Gulf of Mexico. St. Petersburg, FL: Florida Fish and Wildlife Conservation Commission..
Coleman, F. C., Dennis, G. D., Jaap, W. C., Schmahl, G. P., Koenig, C. C., Reed, S. A., et al. (2014). NOAA CRCG 2002 Habitat Characterization of Pulley Ridge and the Florida Middle Grounds, Part 1: Status and Trends in Habitat Characterization of the Florida Middle Grounds. Silver Springs, VA: National Oceanic and Atmospheric Administration Coral Reef Conservation Program.
Coomans, H. E. (1962). The marine mollusk fauna of the Virginian area as basis for defining zoogeographic provinces. Beaufortia Ser. Misc. Pubs. 98, 83–104.
Dupont, J. M., and Coy, C. (2008). “Only the strong will survive: red tides as community-structuring forces in the eastern Gulf of Mexico,” in Diving for Science 2008. Proceedings of the American Academy of Underwater Sciences 27th Symposium, eds P. Brueggeman and N. W. Pollock (Dauphin Island, AL: AAUS), 45–52.
Eidens, C., Hauffe, T., Bayraktarov, E., Wild, C., and Wilke, T. (2015). Multi-scale Processes drive benthic community structure in upwelling-affected coral reefs. Front Mar. Sci. 2:2. doi: 10.3389/fmars.2015.00002
Engle, D. V., and Summers, J. K. (1999). Latitudinal gradients in benthic community composition in Western Atlantic estuaries. J. Biogeogr. 26, 1007–1023. doi: 10.1046/j.1365-2699.1999.00341.x
Engle, D. V., and Summers, J. K. (2000). Biogeography of benthic macroinvertebrates in estuaries along the Gulf of Mexico and western Atlantic coasts. Hydrobiologia 436, 17–33.
Fisco, D. (2016). Reef Fish Spatial Distribution and Benthic Habitat Associations on the Southeast Florida Reef Tract. Master’s thesis, Nova Southeastern University, Dania Beach, FL.
Fuglister, F. C. (1947). “Average monthly sea surface temperatures of the western north Atlantic Ocean,” in Papers in Physical Oceanography and Meteorology, Vol. 10, (Woods Hole, MA: Woods Hole Oceanography Institute), 2.
Gilliam, D. S., Walton, C. J., Brinkhuis, V., Ruzicka, R., and Hayes, N. K. (2017). Southeast Florida Coral Reef Evaluation and Monitoring Project 2015, Year 13 Executive Summary. Miami Beach, FL: Florida Department of Environmental Protection.
Glynn, P. W. (1973). Aspects of the Ecology of Coral Reefs in the Western Atlantic Region. and Geology of Coral Reefs, 2. New York, NY: Academic Press, 271–324.
Gosner, K. L. (1971). Guide to Identification of Marine and Estuarine Invertebrates. New York, NY: Wiley-Interscience.
Grüss, A., Babcock, E. A., Sagarese, S. R., Drexler, M., Chagaris, D. D., Ainsworth, C. H., et al. (2016). Improving the spatial allocation of functional group biomasses in spatially-explicit ecosystem models: insights from three Gulf of Mexico models. Bull. Mar. Sci. 92, 473–496. doi: 10.5343/bms.2016.1057
Grüss, A., Rose, K. A., Simons, J., Ainsworth, C. H., Babcock, E. A., Chagaris, D. D., et al. (2017a). Recommendations on the use of ecosystem modeling for informing ecosystem-based fisheries management and restoration outcomes in the Gulf of Mexico. Mar. Coast. Fish. 9, 281–295. doi: 10.1080/19425120.2017.1330786
Grüss, A., Thorson, J. T., Babcock, E. A., and Tarnecki, J. H. (2017b). Producing distribution maps for informing ecosystem-based fisheries management using a comprehensive survey database and spatio-temporal models. ICES J. Mar. Sci. 75, 158–177. doi: 10.1093/icesjms/fsx120
Hale, S. (2010). Biogeographical patterns of marine benthic macroinvertebrates along the Atlantic coast of the northeastern USA. Estuar. Coast. 33, 1039–1053. doi: 10.1007/s12237-010-9332-z
Hall, C. A. (1964). Shallow-water marine climates and molluscan provinces. Ecology 45, 226–234. doi: 10.2307/1933835
Hallock, P., Robbins, L. L., Larson, R. A., Beck, T., Schwing, P., Martinez-Colon, M., et al. (2010). West florida shelf: a natural laboratory for the study of ocean acidification: U.S. Geol. Survey Open File Rep. 201, 95.
Hedgpeth, J. W. (1954). “Bottom communities of the Gulf of Mexico,” in Gulf of Mexico: Its Origin, Waters, and Marine Life, ed. P. S. Galtsoff (Washington DC: United States Government Printing Office), 279–284.
Hine, A. C., Brooks, G. R., Davis, R. A. Jr., Duncan, D. S., Locker, S. D., Twichell, D. C., et al. (2003). The west-central Florida inner shelf and coastal system: a geologic conceptual overview and introduction to the special issue. Mar. Geol. 200, 1–17. doi: 10.1016/s0025-3227(03)00161-0
Hine, A. C., Halley, R. B., Locker, S. D., Jarrett, B. D., Jaap, W. C., Mallinson, D. J., et al. (2008). “Coral reefs, present and past, on the West Florida shelf and platform margin,” in Coral Reefs of the USA, eds B. M. Riegl and R. E. Dodge (Dordrecht, HL: Springer), 127–173. doi: 10.1007/978-1-4020-6847-8_4
Hine, A. C., and Locker, S. (2011). “Florida Gulf of Mexico continental shelf: great contrasts and significant transitions,” in Gulf of Mexico Origin, Water, and Biota, Volume 3, Geology, eds N. A. Buster and C. W. Holmes (Corpus Christi, TX: Texas A&M University Press), 101–127.
Hutchins, L. W. (1947). The bases for temperature zonation in geographical distribution. Ecolo. Monogr. 17, 325–335. doi: 10.2307/1948663
Jaap, W. C. (1984). Ecology of the South Florida Coral reefs: a Community Profile. FWS/OBS – 82/08. Washington, DC: U. S. Fish Wildlife Service, 138.
Jaap, W. C. (2015). Stony coral (Milleporidae and Scleractinia) communities in the eastern Gulf of Mexico: a synopsis with insights from the Hourglass collections. Bull. Mar. Sci. 91, 207–253. doi: 10.5343/bms.2014.1049
Jaap, W. C., Ross, S. W., Brooke, S., and Arnold, W. S. (2014). “Factors affecting coral reef fisheries in the eastern Gulf of Mexico,” in Interrelationships Between Corals and Fisheries, ed. S. A. Bortone (Boca Raton, FL: CRC Press), 81–110.
Klaus, J. S., Meeder, J. F., McNeill, D. F., Woodhead, J. F., and Swart, P. K. (2017). Expanded Florida reef development during the mid-Pliocene warm period. Global Planet. Change 152, 27–37. doi: 10.1016/j.gloplacha.2017.02.001
Klitgord, K. D., Popenoe, P., and Schouten, H. (1984). Florida: a jurassic transform plate boundary. J. Geophys. Res. Solid Earth 89, 7753–7772. doi: 10.1029/jb089ib09p07753
Klug, K. (2015). Cross-Shelf and Latitudinal Benthic Community Investigation in the Nearshore Habitats of the Northern Florida Reef Tract. Master’s thesis, Nova Southeastern University, Dania Beach, FL.
Kohler, K. E., and Gill, S. M. (2006). Coral point count with excel extensions (CPCe): a visual basic program for the determination of coral and substrate coverage using random point count methodology. Comput. Geosci. 32, 1259–1269. doi: 10.1016/j.cageo.2005.11.009
Leydet, K. P., and Hellberg, M. E. (2016). Discordant coral–symbiont structuring: factors shaping geographical variation of Symbiodinium communities in a facultative zooxanthellate coral genus. Oculina. Coral Reefs 35, 583–595. doi: 10.1007/s00338-016-1409-0
Lirman, D. (2013). “Benthic habitat: coral and hardbottom,” in Integrated Conceptual Ecosystem Model Development for the Southeast Florida Coastal Marine Ecosystem, eds W. K. Nuttle and P. J. Fletcher (Miami, FL: NOAA Technical Memorandum), 53–62. OAR-AOML-103 and NOS-NCCOS-163.
Locker, S. D., Reed, J. K., Farrington, S., Harter, S., Hine, A. C., and Dunn, S. (2016). Geology and biology of the “Sticky Grounds”, shelf-margin carbonate mounds, and mesophotic ecosystem in the eastern Gulf of Mexico. Cont. Shelf Res. 125, 71–87. doi: 10.1016/j.csr.2016.06.015
Love, M. S., Baldera, A., Yeung, C., and Robbins, C. (2013). The Gulf of Mexico Ecosystem: A Coastal & Marine Atlas. New Orleans, LA: Ocean Conservancy, Gulf Restoration Center.
Lugo-Fernández, A., Deslarzes, K. J. P., Price, J. M., Boland, G. S., and Morin, M. V. (2001). Inferring probable dispersal of Flower Garden Banks coral larvae (Gulf of Mexico) using observed and simulated drifter trajectories. Cont. Shelf Resour. 21, 47–67. doi: 10.1016/s0278-4343(00)00072-8
Makowski, C., and Keyes, P. (2011). Using the benthic ecological assessment for marginal reefs (BEAMR) method to quantify nearshore reef conditions in the southeast Gulf of Mexico. J. Coast. Res. 27, 428–440.
Mallinson, D., Hine, A., Naar, D., Locker, S., and Donahue, B. (2014). New perspectives on the geology and origin of the Florida Middle Ground carbonate banks, West Florida Shelf, USA. Mar. Geol. 355, 54–70. doi: 10.1016/j.margeo.2014.04.007
McGinty, E. S., Pieczonka, J., and Mydlarz, L. D. (2012). Variations in reactive oxygen release and antioxidant activity in multiple symbiodinium types in response to elevated temperature. Microb. Ecol. 64, 1000–1007. doi: 10.1007/s00248-012-0085-z
Nuttle, W. K., and Fletcher, P. (2013). “Integrated conceptual ecosystem model for the southwest florida shelf coastal marine ecosystem,” in NOAA Technical Memorandum. : OAR AOML, (Silver Spring, MA: National Oceanic and Atmospheric Administration),102.
Obrochta, S. P., Duncan, D. S., and Brooks, G. R. (2003). Hardbottom development and significance to the sediment-starved west-central Florida inner continental shelf. Mar. Geol. 200, 291–306. doi: 10.1016/s0025-3227(03)00188-9
Okey, T. A., Vargo, G. A., Mackinson, S., Vasconcellos, M., Mahmoudi, B., and Meyer, C. A. (2004). Simulating community effects of sea floor shading by plankton blooms over the West Florida Shelf. Ecol. Model. 172, 339–359. doi: 10.1016/j.ecolmodel.2003.09.015
Overstreet, R. M., and Hawkins, W. E. (2017). “Diseases and mortalities of fishes and other animals in the Gulf of Mexico,” in Habitats and Biota of the Gulf of Mexico: Before the Deepwater Horizon Oil Spill, Vol. 2, ed. C. H. Ward (New York, NY: Springer), 1589–1738. doi: 10.1007/978-1-4939-3456-0_6
Paull, C., Freeman-Lynde, R., Bralower, T., Gardemal, J., Neumann, A., D’Argenio, B., et al. (1990). Geology of the strata exposed on the Florida Escarpment. Mar. Geol. 91, 177–194. doi: 10.1016/0025-3227(90)90035-i
Phillips, N. W., Gettleson, D. A., and Spring, K. D. (1990). Benthic biological studies of the southwest Florida shelf. Am. Zool. 30, 65–75. doi: 10.1093/icb/30.1.65
Precht, W. F., Gintert, B. E., Robbart, M. L., Fura, R., and van Woesik, R. (2016). Unprecedented disease-related coral mortality in southeastern Florida. Sci. Rep. 6:31374. doi: 10.1038/srep31374
Rice, S. A., and Hunter, C. L. (1992). Effects of suspended sediment and burial on scleractinian corals from west central Florida patch reefs. Bull. Mar. Sci. 51, 429–442.
Saul, S., Walter, J. III, Die, D., Naar, D., and Donahue, B. (2013). Modeling the spatial distribution of commercially important reef fishes on the West Florida Shelf. Fish. Res. 143, 12–20. doi: 10.1016/j.fishres.2013.01.002
Simon, J., and Mahadevan, S. (1985). “Benthic macroinvertebrates of Tampa Bay,” in 1st Tampa Bay Area Scientific Information Symposium (BASIS) Proceedings, eds S. F. Treat, J. L. Simon, R. R. Lewis, and III R. L. Whitman Jr. (Minnetonka MN: Bellweather Press), 384.
Smith, F. G. W. (1954). “Gulf of Mexico Madreporaria,” in Gulf of Mexico: Its Origin, Waters, and Marine Life, ed. P. S. Galtsoff (Washington D.C: United States Government Printing Office), 291–295.
Smith, G. B. (1975). The 1971 red tide and its impact on certain reef communities in the mid-eastern Gulf of Mexico. Environ. Lett. 9, 141–152. doi: 10.1080/00139307509435843
Smith, S., Swanson, D., Chiappone, M., Miller, S., and Ault, J. (2011). Probability sampling of stony coral populations in the Florida Keys. Environ. Monit. Assess. 183, 121–138. doi: 10.1007/s10661-011-1912-2
Spalding, M. D., Fox, H. E., Allen, G. R., Davidson, N., Ferdaña, Z. A., Finlayson, M., et al. (2007). Marine ecoregions of the world: a bioregionalization of coastal and shelf areas. AIBS Bull. 57, 573–583. doi: 10.1641/b570707
Tchounwou, P. B. (1999). Climate change and its potential impacts on the Gulf Coast region of the United States. Rev. Environ. Health 14, 91–102. doi: 10.1515/reveh.1999.14.2.91
Thompson, M., Schroeder, W., Phillips, N., and Graham, B. (1999). “Ecology of live bottom habitats of the northeastern Gulf of Mexico: a community profile,” in US Department of the Interior. US Geological Survey, Biological Resources Division, USGS/BRD/CR-1999–0001, and Minerals Management Service, (New Orleans, LA: Gulf of Mexico OCS Region), 94–99. OCS Study, MMS.
Thornhill, D. J., Kemp, D. W., Bruns, B. U., Fitt, W. K., and Schmidt, G. W. (2008). Correspondence between cold tolerance and temperate biogeography in a western atlantic symbiodinium (Dinophyta) lineage. J. Phycol. 44, 1126–1135. doi: 10.1111/j.1529-8817.2008.00567.x
Toonen, R. J., Bowen, B. W., Iacchei, M., and Briggs, J. C. (2016). “Biogeography, Marine,” in Encyclopedia of Evolutionary Biology, ed. R. M. Kliman (Oxford: Academic Press), 166–178.
Topp, R. W., and Hoff, F. W. Jr. (1972). Flatfishes (Pleuronectiformes). Memoirs of the Hourglass Cruises IV(II). St. Petersburg: Florida Marine Research Institute.
Verges, A., Steinberg, P. D., Hay, M. E., Poore, A. G., Campbell, A. H., Ballesteros, E., et al. (2014). The tropicalization of temperate marine ecosystems: climate-mediated changes in herbivory and community phase shifts. Proc. R. Soc. BBiol. Sci. 281:20140846. doi: 10.1098/rspb.2014.0846
Walker, B. K. (2012). Spatial analyses of benthic habitats to define coral reef ecosystem regions and potential biogeographic boundaries along a latitudinal gradient. PLoS One 7:e30466. doi: 10.1371/journal.pone.0030466
Walker, B. K., and Gilliam, D. S. (2013). Determining the extent and characterizing coral reef habitats of the northern latitudes of the Florida Reef Tract (Martin County). PLoS One 8:e0030466.
Walker, B. K., and Klug, K. (2014). Southeast Florida Shallow-Water Habitat Mapping & Coral Reef Community Characterization. Miami, FL: Florida DEP Coral Reef Conservation Program.
Walker, B. K., Riegl, B., and Dodge, R. E. (2008). Mapping coral reef habitats in southeast Florida using a combined technique approach. J. Coast. Res. 24, 1138–1150. doi: 10.2112/06-0809.1
Walton, C. J., Hayes, N. K., and Gilliam, D. S. (2018). Impacts of a regional, multi-year, multi-species coral disease outbreak in Southeast Florida. Front. Mar. Sci. 5:323. doi: 10.3389/fmars.2018.00323
Weisberg, R. H., Zheng, L., Liu, Y., Corcoran, A. A., Lembke, C., Hu, C., et al. (2016). Karenia brevis blooms on the West Florida Shelf: a comparative study of the robust 2012 bloom and the nearly null 2013 event. Cont. Shelf Res. 120, 106–121. doi: 10.1016/j.csr.2016.03.011
Work, R. C. (1969). Systematics, ecology, and distribution of the mollusks of Los Roques, Venezuela. Bull. Mar. Sci. 19, 614–711.
Keywords: benthic habitat mapping, community characterization, biogeography, west Florida shelf, eastern Gulf of Mexico, Tampa Bay
Citation: Walker BK, Eagan S, Ames C, Brooke S, Keenan S and Baumstark R (2020) Shallow-Water Coral Communities Support the Separation of Marine Ecoregions on the West-Central Florida Gulf Coast. Front. Ecol. Evol. 8:210. doi: 10.3389/fevo.2020.00210
Received: 29 March 2020; Accepted: 08 June 2020;
Published: 26 June 2020.
Edited by:
Oana Moldovan, Emil Racovita Institute of Speleology, RomaniaReviewed by:
Juan José Morrone, National Autonomous University of Mexico, MexicoBrian Bowen, University of Hawaii, United States
Copyright © 2020 Walker, Eagan, Ames, Brooke, Keenan and Baumstark. This is an open-access article distributed under the terms of the Creative Commons Attribution License (CC BY). The use, distribution or reproduction in other forums is permitted, provided the original author(s) and the copyright owner(s) are credited and that the original publication in this journal is cited, in accordance with accepted academic practice. No use, distribution or reproduction is permitted which does not comply with these terms.
*Correspondence: Brian K. Walker, d2Fsa2VyYkBub3ZhLmVkdQ==