- 1Allen Discovery Center, Tufts University, Medford, MA, United States
- 2Department of Biology, Duke University, Durham, NC, United States
The nymphalid groundplan (NGP) has proven to be extraordinarily useful in the study of butterfly color patterns because it allows for the identification of homologous elements across species. It has long been thought that the NGP is broadly applicable to all Lepidoptera, implying that the characters which make-up butterfly color patterns are homologous to those found in the moths. However, this conjecture remains mostly untested. We analyzed the wing patterns of the hyper-diverse arctiid tiger moths, which are represented worldwide by approximately 11,000 species, and found that the color patterns of these animals can be parsed into a limited toolkit of homologous characters. Some of the pattern elements identified, such as the basal and central symmetry system, are present on the NGP, but their morphology is often quite different from what is seen in the butterflies. The border ocelli of the NGP appear to be absent altogether in the Arctiidae, and conversely, two distal pattern element we term the “van Bemmelen” and “terminal” bands are present in the color patterns of many arctiids, but are not represented on the NGP. In light of the observed differences, we derive a new theoretical groundplan based on the original NGP that we refer to as the “arctiid archetype.” This model provides a comprehensive framework for understanding how the wing patterns of these animals develop, and yields novel insight into their evolutionary history.
Introduction
The wing patterns of butterflies have proven to be a uniquely tractable system for research at the interface of evolution and development (Nijhout, 1991; Beldade and Brakefield, 2002; Jiggins, 2017). Unlike the stripes of zebras (Jonathan, 1977; Caro et al., 2014) or the networks of spots found on the coats of leopards (Allen et al., 2011), butterfly color patterns are composed of a limited number of homologous characters that are comparable to better known systems of homologs, such as the bones of pentadactyl limbs (Wagner, 2014). A theoretical archetype known as the nymphalid groundplan (NGP) (Nijhout, 1991) allows for the identification of these homologous characters within and across species, making it possible to precisely describe the results of experiments designed to interfere with pattern development (Monteiro et al., 2006; Martin and Reed, 2014; Mazo-Vargas et al., 2017), and identify large-scale trends in pattern evolution (Monteiro, 2008). Since its rediscovery in the 1970s (Nijhout, 1978), the NGP has directly stimulated many of the advances that have been made in our understanding of butterfly color patterns.
Although we now know a great deal about butterfly wing patterning, our knowledge of how the patterns of moths develop and evolve is comparatively limited. Researchers have often speculated that nymphalid groundplan homologs must be present in the color patterns of these animals, but attempts to test this conjecture empirically have been few and far between (Schachat and Brown, 2015, 2016; Schachat and Goldstein, 2018; Gawne and Nijhout, 2019). The aim of this paper is to broaden our understanding of lepidopteran wing patterning by determining whether the dorsal forewing patterns of the hyper-diverse arctiid “tiger moths” [Lepidoptera, Erebidae (Arctiidae)]1 can be decomposed into an underlying system of characters that are homologous to elements found on the nymphalid groundplan. The arctiids exhibit an enormous amount of pattern diversity both within and across species, which makes them interesting from an evolutionary and developmental point of view. A previous study has shown that it is possible to locate NGP characters such as the basal symmetry system, central symmetry system, and discal spot in the patterns of the tiger moth Utetheisa ornatrix (Gawne and Nijhout, 2019), but no attempt has yet been made to assess the generality of this finding through a large-scale study of the Arctiidae.
An analysis of specimens in museum collections suggested that the nymphalid groundplan homologs identified in U. ornatrix are widespread among the arctiids, and confirmed that tiger moth color patterns often contain two unique characters that are not depicted on modern renditions of the groundplan (Gawne and Nijhout, 2019). The fact that the arctiids deviate from the NGP in this way suggests that research on wing patterning in this subfamily will necessitate a return to earlier versions of the model, which included supernumerary characters that are primarily found in the moths (Gawne and Nijhout, 2019). To help stimulate research on arctiid color patterns, we introduce a modified version of the original groundplan (Schwanwitsch, 1924; Süffert, 1927) that we refer to as the arctiid archetype or “arctiitype.” This theoretical model has the potential to motivate novel experimental studies of the arctiids because it allows us to identify homologies, and make sense of the diversity observed their wing patterns.
We suggest that the dorsal forewing patterns of the arctiids are constructed from a maximum of five primary characters: a basal and central symmetry system, along with a discal spot, and two external characters we have termed the “van Bemmelen,” and “terminal” bands. Similar to what previous researchers have noted about NGP homologs in the butterflies (Nijhout, 1991; Otaki, 2012), there is no expectation that the entire suite of characters shown on arctiitype will be present in every species or individual. Rather, our hypothesis is that no tiger moth wing patterns contain supernumerary primary elements that are not depicted on the arctiid archetype. The combination, alteration, and interaction of the five archetypical characters, along with the occasional inclusion of “detailed” elements such as venous stripes and stochastic melanization (Nijhout, 1991) is sufficient to generate the vast array of color patterns observed in the tiger moths. Thus, in delineating the homologies of arctiid wing patterns, we have identified the morphological toolkit these animals have employed and redeployed to move through morphospace over the course of their evolutionary history.
Methods
To survey the diversity of tiger moth dorsal forewing patterns, we photographed over 1,100 mounted specimens belonging to an estimated 100 different species. These animals were found in the collections of the Smithsonian National Museum of Natural History, the Vienna Museum of Natural History, and the Hungarian Museum of Natural History. Specimens were photographed with either a Nikon Coolpix L340 (20.2 megapixels) or a Nikon D-5300 (24.1 megapixels) digital camera, using overhead florescent lighting and a scale bar. The resulting images provided the basis for the figures presented in this paper. Photoshop (Adobe Inc.) was used to edit these images, allowing for easier analysis of the macro-structure of the dorsal forewing pattern, and on-screen/in-print readability. The final, fully assembled figures were produced in Photoshop and PowerPoint (Microsoft Corp.). When relevant, left forewings were transformed via a horizontal flip to ensure a common orientation of the samples. The antennae have been drawn in on all full specimens shown (Figures 2, 3, 9).
A simple visual analysis of the photographic dataset revealed that arctiid moths show an enormous amount of variation in patterning both within (Figure 1) and across species (Figure 2). To help systematize the observed interspecific differences in phenotype, we attempted to identify recurring types of dorsal forewing patterning (Figure 2), and describe the varieties of non-homologous detailed elements (Nijhout, 1991) that occur in the photographed specimens (Figure 3). Detailed elements are common in arctiid color patterns, and similar to what has been documented in the butterflies (Nijhout, 1991), it appears that these features can sometime become the dominant component of the pattern.
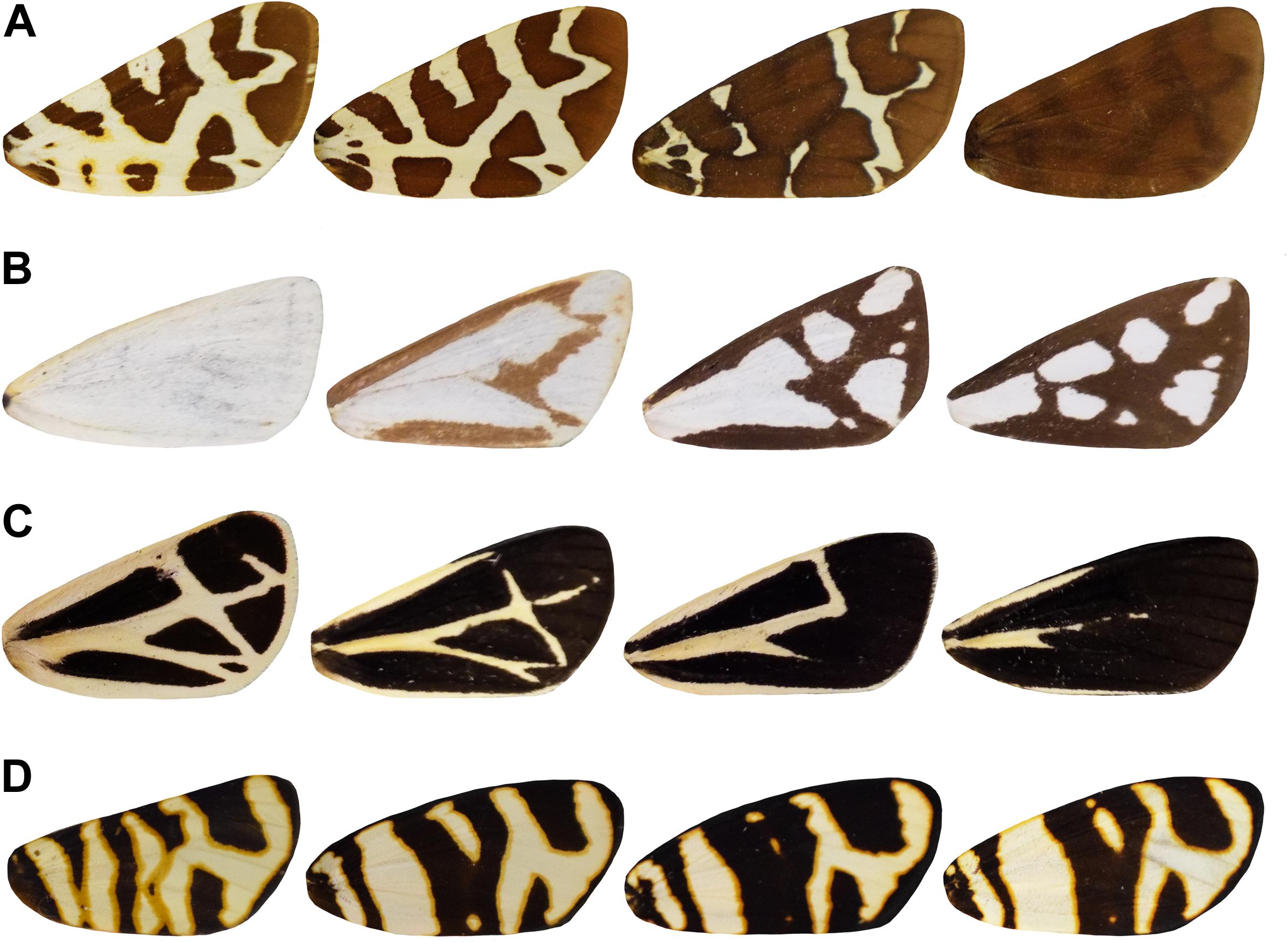
Figure 1. Intraspecific variation in arctiid dorsal forewing patterning. Many arctiid species show extremely high levels of intraspecific phenotypic variation, with various pattern elements being significantly expanded or reduced in size, and even merged with adjoining elements. Examination of large numbers of specimens found in museum collections reveals that this variation is often continuous. Row (A): Arctia caja; row (B): Haploa confusa; row (C): Apantesis nais; row (D): Eucharia festiva.
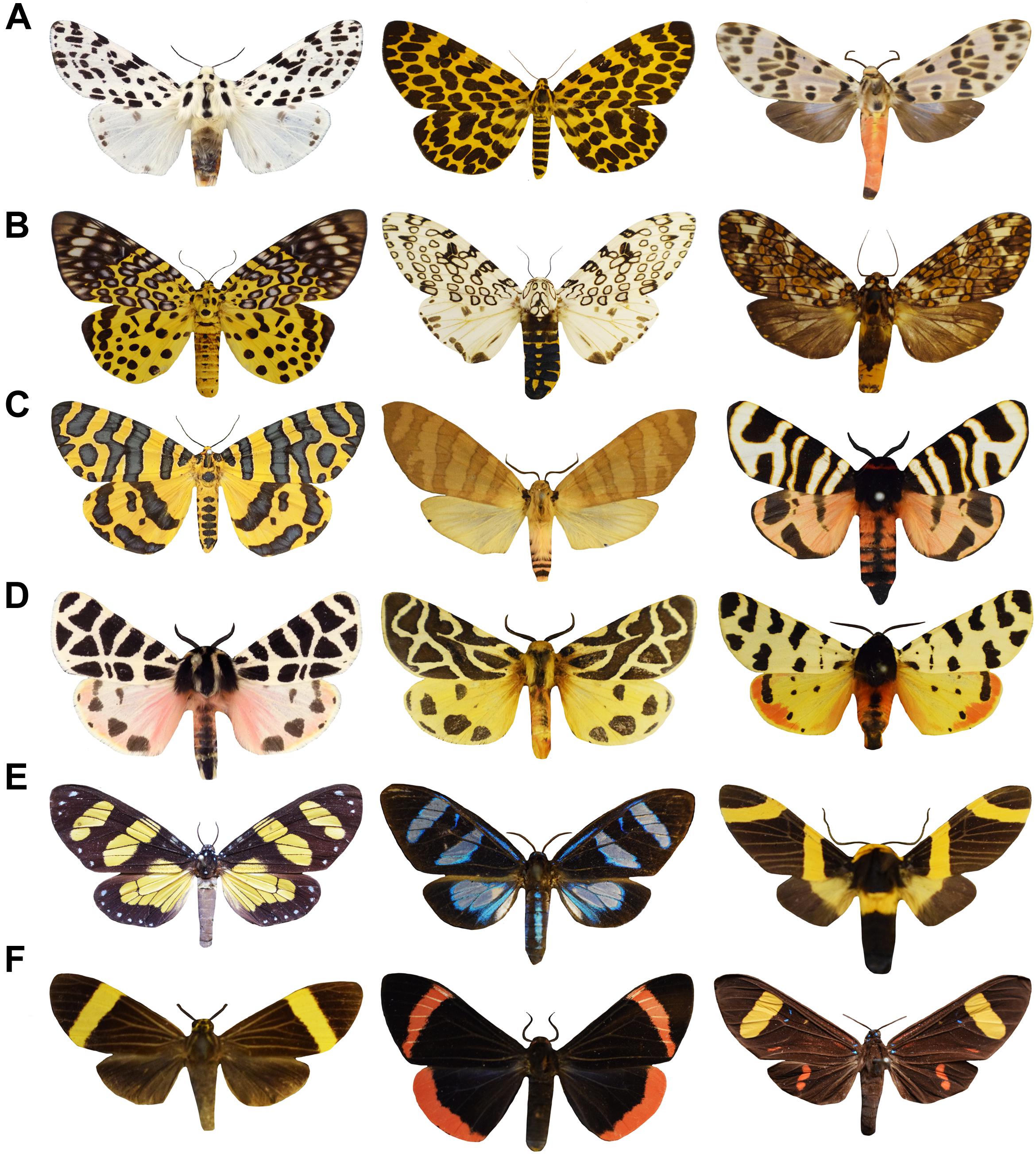
Figure 2. The primary types of arctiid dorsal forewing patterning. Animals shown in row (A) exhibit single spot phenotypes (left: Hypercompe permaculata; middle: Calpenia monilifera; right: Delphyre roseiceps). In these patterns, the homologous characters are composed of semi-discrete spotted elements that are one solid color. The specimens presented in row (B) exhibit concentric spot phenotypes (left: Sebastia argus; middle: Ecpantheria scribonia; right: Phaegoptera granifera) in which the inner focus of the spots is a different color than the surrounding halo region. As illustrated by the animals present in row (C), these spotted elements can fuse together to form mostly continuous bands that run from the wing’s leading anterior edge to its trailing posterior margin (left: Callimorpha bellatrix; middle: Elysius meridionalis; right: Eucharia festiva). The specimens in row (D) (left: Apantesis nevadensis; middle: Preparctia romanovi; right: Apantesis tigrina) exhibit more discontinuous banded patterns characterized by the presence of numerous breaks and distortions that are not as pronounced in patterns with continuous bands. Row (E) shows specimens with triple bold patterns (left: Dysschema tiresias; middle: Agyrtidia uranophila; right: Automolis angulosa). Triple bold patterns are predicted to result from the fusion of banded elements along the wing’s distal-proximal axis. Patterns of this type are characterized by three mostly contiguous regions (often melanic in color) of fused primary characters separated by contrasting regions of background coloration. Finally, the species depicted in row (F) display double bold patterns brought about through additional distal-proximal fusions of banded pattern elements (left: Napata jynx; middle: Coreura fida; right: Histoea proserpina). Animals showing this phenotype possess two fused regions (often melanic in color) separated by a single patch of background coloration. The phenotypic classes described in (A–F) are continuous, rather than discrete, and specimens belonging to the same species often exhibit distinct types of dorsal forewing patterning.
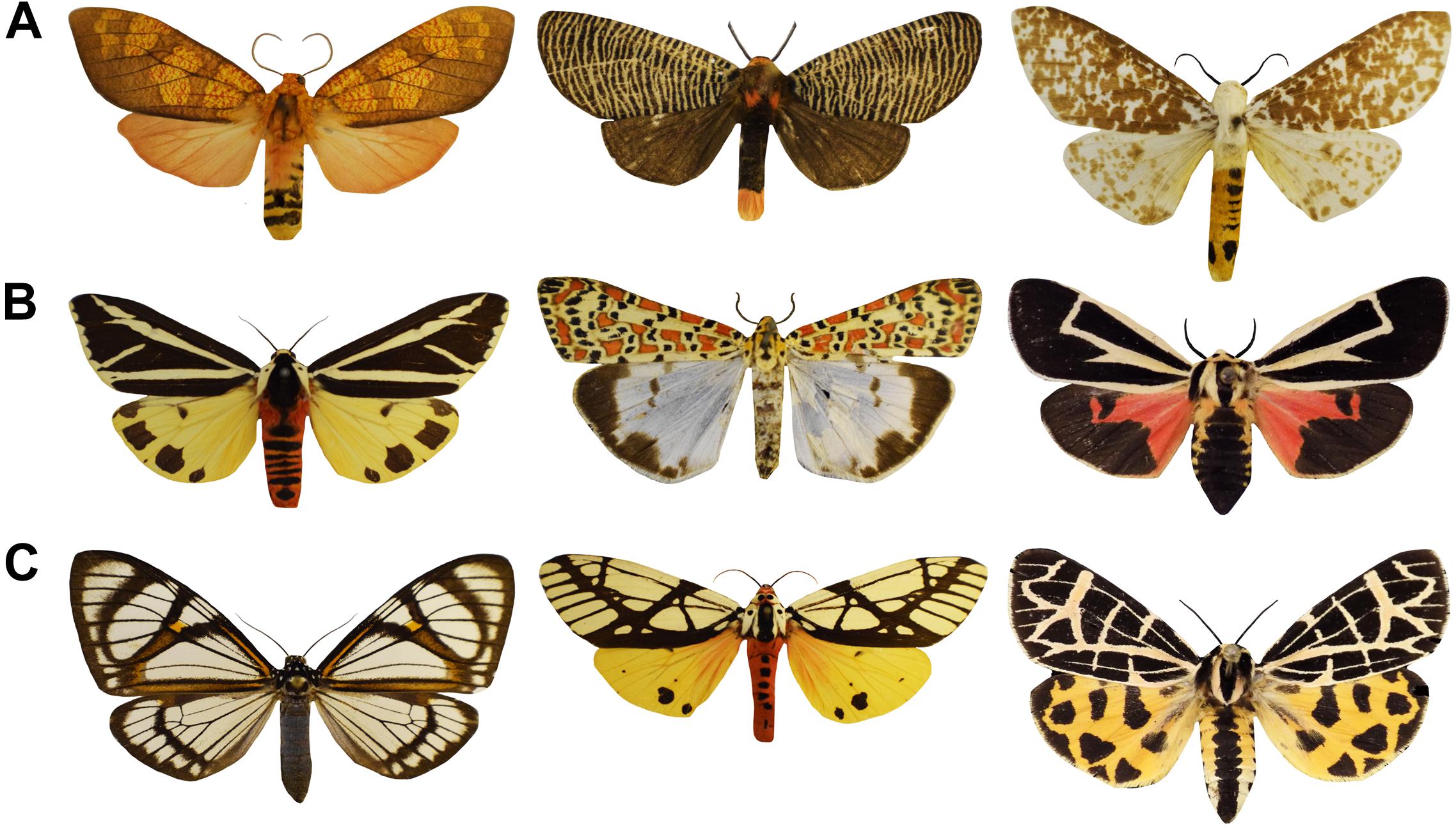
Figure 3. Varieties of detailed elements found in arctiid dorsal forewing patterns. Detailed pattern elements often appear in conjunction with the homologous pattern elements, similar to what has been documented in the butterflies. Animals shown in row (A) exhibit disruptive details, which include Turing-like patterns that serve as background coloration, and stochastic melanization that is overlaid on top of the primary pattern elements (left: Elysius conspersus; middle: Graphelysia strigilcata; right: Spilosoma aspersa). Animals depicted in row (B) possess dorsal forewing patterns that include filled wing cells. This detailed feature occurs when an intervenous region such as the discal cell becomes completely pigmented, creating a structure that closely outlines the focal venous compartment (left: Areas imperialis; middle: Utetheisa pulchella; right: Apantesis nais). The specimens shown in row (C) exhibit venous striping, which partially or completely outlines the wing’s veins (left: Eucyane phanoptoides; middle: Pericallia obliquifascia; right: Apantesis ornata).
To test this hypothesis, we artificially selected for intensified venous striping in Utetheisa ornatrix (Figure 4). This experiment began with a large, previously established laboratory colony that contained animals with a diverse array of pattern morphologies (Gawne and Nijhout, 2019). Animals were reared under controlled day/night, temperature, and nutritional conditions that have previously been described (Gawne and Nijhout, 2019). Individuals with white stripes that outlined the contours of the veins were known to appear in the colony. To determine whether the intensity of venous striping could be increased through artificial selection, we separated a population of approximately 10–20 animals with white stripes from the main colony and allowed them to mate. Some of the animals resulting from these initial pairings displayed slightly intensified venous striping. These individuals were then put back into the experimental colony, and allowed to mate with one another, and any remaining animals from the parental generation. This selection regime was continued for two additional generations. During the four generations over which the experiment was carried out, we periodically sampled animals with more pronounced venous striping to document their wing patterns. The forewings of these animals were removed, and mounted between two microscope slides. These prepared specimens were then photographed using an Olympus SZX16 stereomicroscope equipped with an Olympus DP71 camera. The resulting images were subsequently edited in Photoshop to prepare them for use in the figures, similar to our previous work on U. ornatrix (Gawne and Nijhout, 2019).
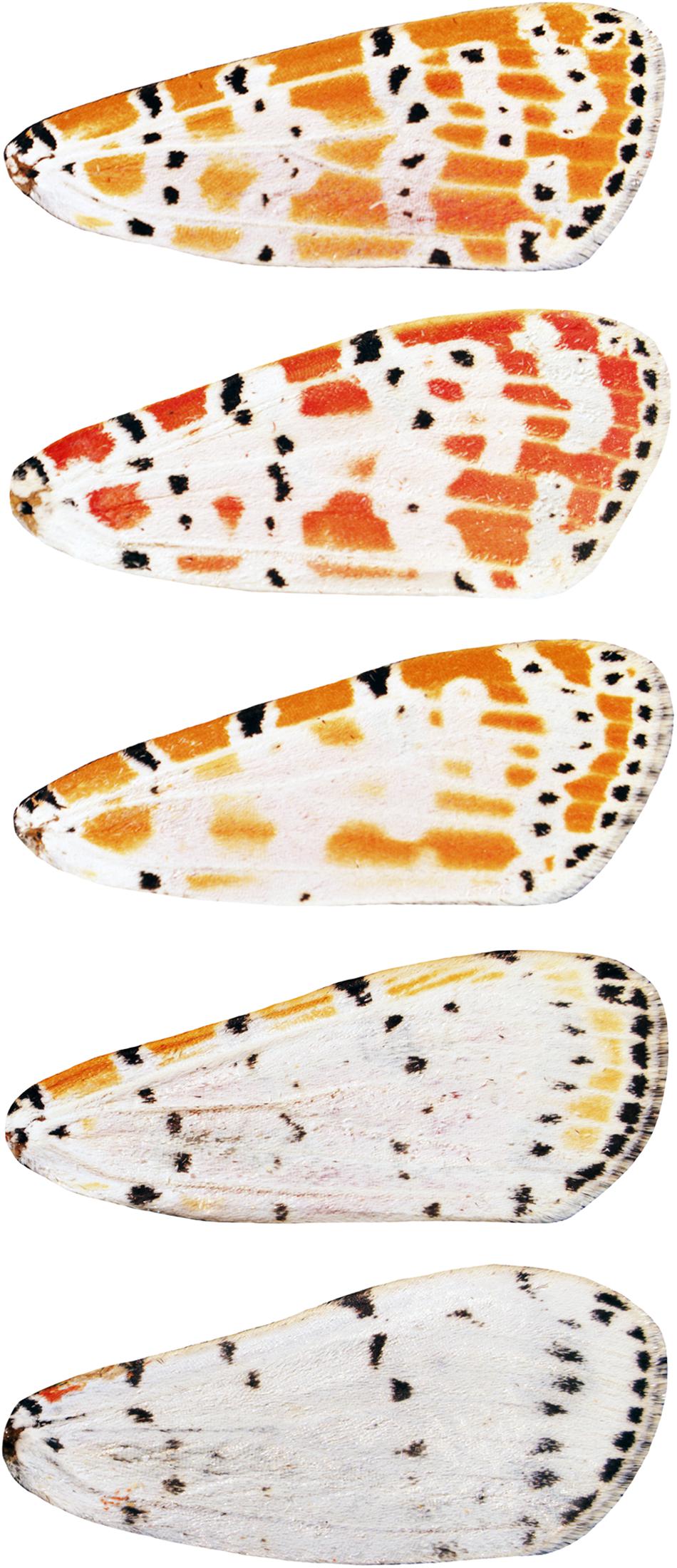
Figure 4. Selection for intensified venous striping in Utetheisa ornatrix. Over the course of four generations, we artificially selected for more pronounced venous stripes in U. ornatrix. The animals initially selected for use in the experiment had modest white stripes that did little more than outline the contours of the veins, similar to those shown at the top of the figure. The offspring of these animals sometimes showed more pronounced venous striping that began to invade the intervenous regions of the wing. These animals were allowed to mate with one another, and any animals remaining from the previous generation. We continued to apply this directional selective pressure for two additional generations, resulting in a further intensification of the venous striping in some animals. The selection regime occasionally produced animals that were almost pure white overall (bottom), with few of the primary black spotted elements remaining. This experiment reveals that details such as venous stripes have the ability to become the most prominent feature of the pattern. Some of the animals shown are also depicted in Figure 1 of Gawne and Nijhout (2019).
The identification of morphological types provides insight into the parameters of arctiid pattern morphospace, and allows for more nuanced analyses designed to locate specific homologous characters. Based on our earlier work with U. ornatrix (Gawne and Nijhout, 2019), and an examination of the positioning of elements in the specimens used for this study, we hypothesized that tiger moth color patterns are composed of a maximum of five homologous characters, three of which are found in the modern rendition of the NGP (Nijhout, 1991, 2001; Otaki, 2012). Accordingly, we developed an arctiid archetype that depicts what appear to be the primary homologous characters found in the dorsal forewing patterns of the tiger moths (Figure 5). The arctiid archetype is a hypothesis that purports to identify the complete morphological toolkit the arctiid moths have used to generate their phenotypic diversity. Like the nymphalid groundplan, it omits detailed pattern elements (Nijhout, 1991; Otaki, 2012) such as venous stripes and stochastic peppering, and instead shows only a series of five homologous pattern elements. Following the lead of early butterfly color pattern researchers (Schwanwitsch, 1924; Süffert, 1927), we have attempted to demonstrate the plausibility of the archetype using comparative morphological mapping. An analysis of the inter- and intra-specific variation documented in museum collections allows for inferences to be drawn about the location of the homologous pattern elements in the six most common types of arctiid wing patterns (Figure 6).
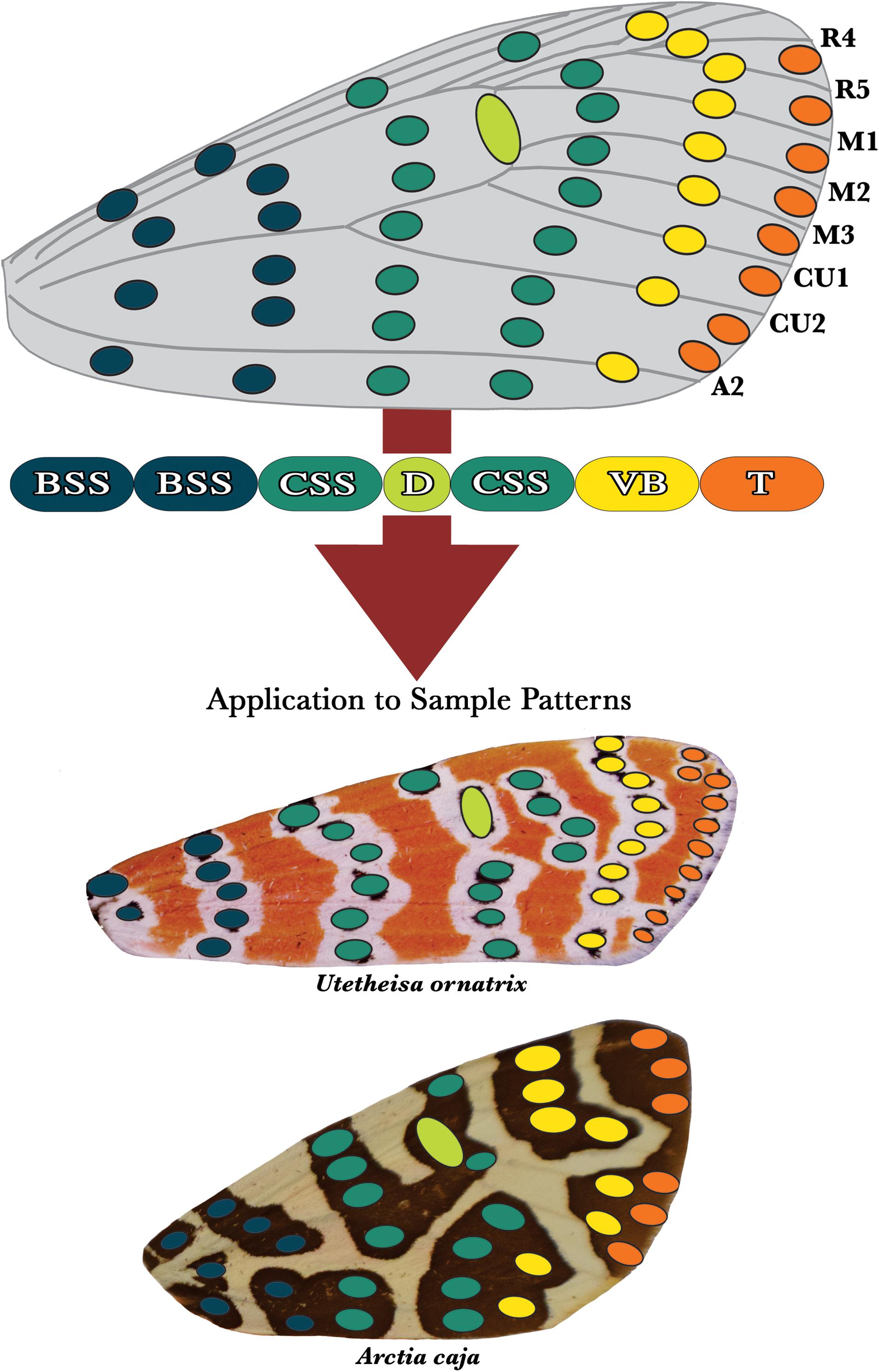
Figure 5. The arctiid archetype. We hypothesize that arctiid moth dorsal forewing patterns are fundamentally composed of serially repeated spotted elements arranged in columns that run anterior-posterior across the wing’s surface. The development of certain columns of spots is predicted to be highly correlated, leading to the formation of a series of five individuated characters: the (1) basal symmetry system (BSS), (2) central symmetry system (CSS), (3) discal spot (D), (4) van Bemmelen band (VB), and (5) terminal band (T). As with the butterflies, the two columns nearest to the wing hinge are hypothesized to constitute the basal symmetry system (blue). The central symmetry system (green) is composed of two columns of spots that are positioned immediately distal to the BSS. The columns of spots that compose this character lie on either side of the discal spot (lime), which is located directly on top of the discalis cross-vein. The van Bemmelen band (yellow) has no counterpart in the dorsal forewing patterns of the butterflies, and could be unique to the arctiids. The terminal band (orange) is located on the wing’s distal margin, and consists of a series of spots positioned directly between the vein termini. At the bottom of the figure, the arctiitype has been applied to the patterns of two sample tiger moth species, Utetheisa ornatrix and Arctia caja.
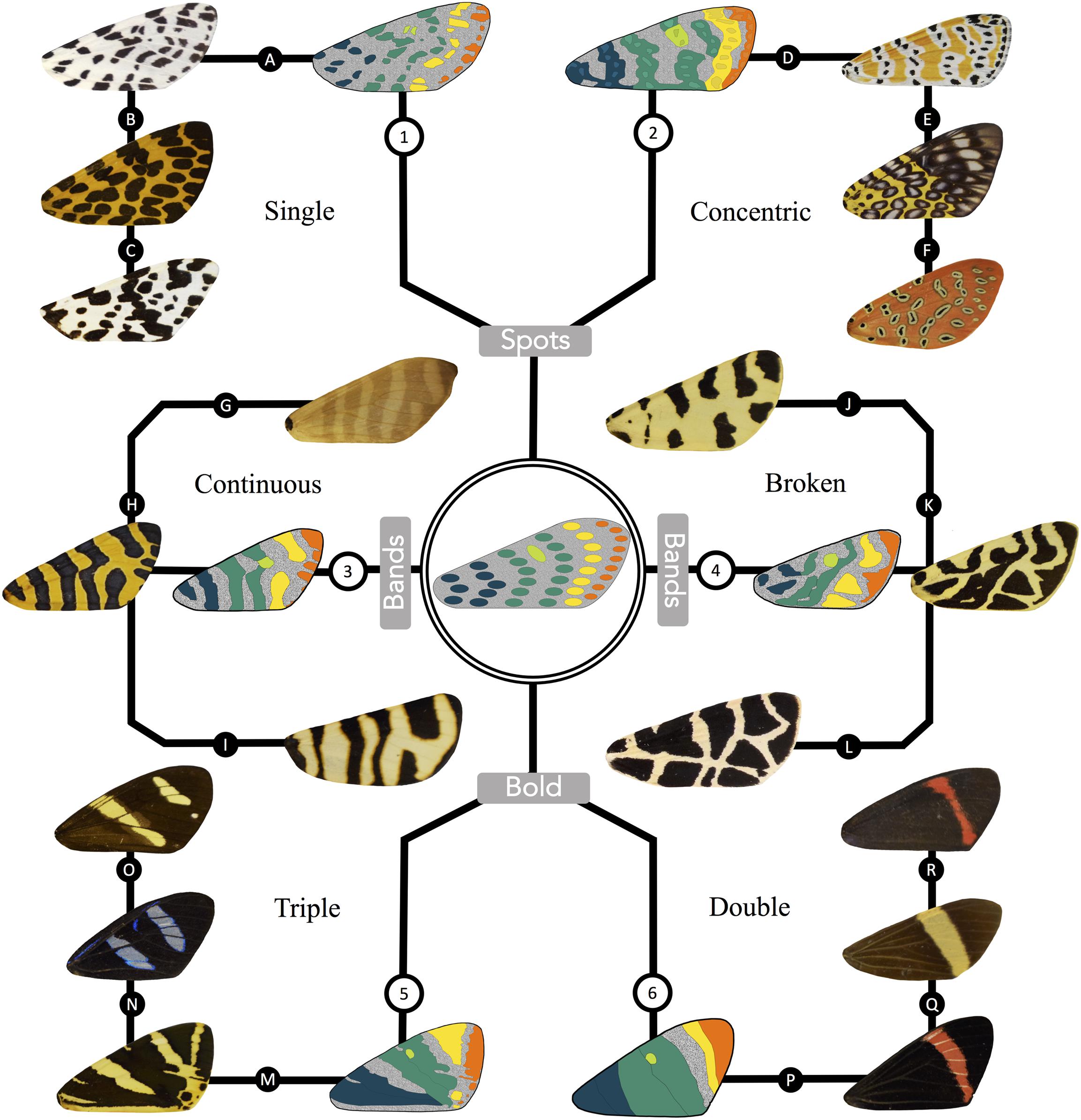
Figure 6. A derivation of the primary types of arctiid patterning from the morphologically simple archetypical characters. The arctiid archetype is depicted in the center of the figure, with six surrounding branches that are representative of the various types of patterning found in the tiger moths. The basal symmetry system, central symmetry system, discal spot, van Bemmelen band, and terminal band (colored blue, green, lime, yellow and orange, respectively) have been identified in one sample species from each of the six types. The top set of branches show animals exhibiting the (1) single spot, and (2) concentric spot phenotypes. Continuous (3) and broken band (4) phenotypes are shown on the branches to the left and right of the archetypical pattern. Bold patterns are depicted at the bottom of the figure. The animals shown in branch (5) exhibit a “triple” bold phenotype, where the primary pattern elements have fused to form three more or less continuous patches. Branch (6) shows animals exhibiting the “double” bold phenotype. In patterns of this type, fusion of the primary characters is more complete, resulting in a pair of dark-colored regions on either side of an isolated island of background coloration. Specimens from the following species are represented in the figure. Group 1: (A) Hypercompe permaculata, (B) Calpenia monilifera, (C) Eucereon coenobita; Group 2: (D) Utetheisa ornatrix, (E) Sebastia argus, (F) Argina argus; Group 3: (G) Elysius superbus, (H) Amphicallia thelwalli, (I) Eucharia festiva; Group 4: (J) Apantesis tigrina, (K) Preparctia romanovi, (L) Apantesis nevadensis; Group 5: (M) Callimorpha quadripunctaria, (N) Agyrtidia uranophila, (O) Chetone isse; Group 6: (P) Coreura fida, (Q) Napata jynx, (R) Coreura euchromioides.
Results
The arctiid moths exhibit some of the most pronounced intraspecific wing pattern variation found in the Lepidoptera (Figure 1). To give just a few examples, within a given species it is possible to identify variation in background coloration, the number of primary elements, and the overall morphological structure the pattern. Looking across species, the dorsal forewing patterns generally fall into one of three broad phenotypic classes: spotted, banded, and bold (Figure 2). In species with spotted patterns, the intervenous elements that compose the presumptive homologous characters take the form of a highly discretized series of spots arranged in columns that run anterior-posterior across the wing. Spotted patterns can be further decomposed into two sub-types: (a) “single spot” phenotypes where all spots are homogenous in their coloration, and (b) “concentric spot” phenotypes, in which a large halo-like ring surrounds a spot of a different color.
As with the spotted patterns, banded phenotypes can be separated into two varieties. In species that exhibit “continuous bands,” a series of semi-parallel stripes run mostly uninterrupted across the wing’s surface in an anterior-posterior direction. In the “broken band” sub-type, the columnar banded patterns exhibit more regular or pronounced interruptions, especially along the CU2 vein. Bold patterns are the third class of phenotypes found in the arctiids. In most instances, this type of patterning appears to result from the fusion of characters, predominantly along the wing’s proximo-distal axis. Bold phenotypes are characterized by the presence of contiguous melanic regions that are interspersed with smaller islands of background coloration (Nijhout and Wray, 1988; Nijhout, 1991). For comparative purposes, we have divided bold patterns into the sub-classes “double” and “triple,” which are differentiated by the number of fused melanic regions present on the wing.
As shown in Figure 3, detailed elements such as venous striping, filled wing cells, ripples, and stochastic peppering can also be found in tiger moth wing patterns. Details are often overlaid on top of the primary pattern elements, but can also become the dominant feature of the pattern. Our artificial selection experiment with U. ornatrix (Figure 4) revealed that the venous striping which initially did little more than outline the contours of the wing veins could be intensified through the application of directional selective pressure. In no more than four generations, we were able to produce animals in which the formerly modest venous stripes expanded into the intervenous regions. The fact that details such as venous stripes can be upregulated in this way is important because it raises the possibility that some of the bold patterns observed in the tiger moths might be produced by the expansion of these elements, rather than the fusion of spots or bands that comprise the primary characters. This hypothesis is reinforced by an examination of other arctiid species (Figure 7).
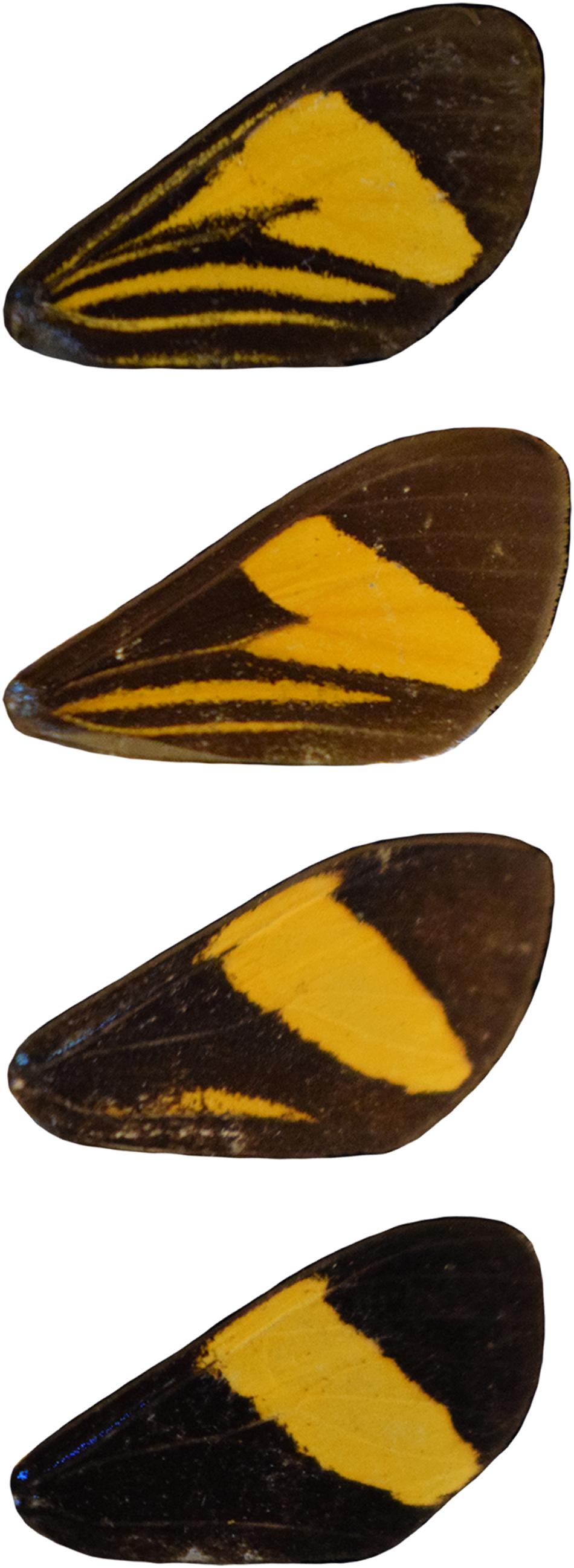
Figure 7. Bold patterns can be produced through the intensification of venous striping. The scheme depicted in Figure 6 suggests that bold patterns are produced from the distal-proximal fusion of primary elements. However, examination of specimens found in museum collections seems to reveal that an alternative mechanism of bold pattern development also exists. For example, in the genus Callopela, it appears that bold patterns can be produced through an intensification of venous striping, which has the ability expand into the intervenous regions and produce continuous patches of melanic coloration. Specimens such as those shown exhibit venous striping which varies continuously in intensity, and a gives rise to a corresponding continuum of overall pattern “boldness” (Top to bottom: Callopepla inachia; Callopepla grandis; Callopepla similis; Callopepla similis).
It appears that the developmental processes responsible for generating venous stripes in the tiger moths are at least partially independent of the mechanisms that give rise to other pattern components. However, very little is known about the specific developmental pathways that produce the detailed elements of any lepidopteran color pattern, and as such we cannot be certain that the same ontogenetic processes are responsible for their formation in every individual or species. Because of this, we suggest that details must be treated as phenotypic analogies until their development is more thoroughly understood. This implies that the melanic peppering, venous striping, ripple patterns, etc. observed in one arctiid species should not be assumed to be homologous to similar details found in the patterns of other species (Nijhout, 1991).
The arctiid archetype is visualized in Figure 5. Like the nymphalid groundplan, this archetype has been derived from an analysis of intraspecific pattern variation, and interspecific pattern diversity. We suggest that the dorsal forewing patterns of the arctiids are produced from a morphological toolkit that consists of no more than five primary characters: (1) a basal symmetry system, (2) a central symmetry system, (3) a discal spot, and two distinct marginal characters we term the (4) van Bemmelen and (5) terminal bands. The basal symmetry system shown on the arctiitype consists of two columns of spots that run side-by-side near the wing hinge. Similarly, the central symmetry system is composed of two columns of spots that lie on either side of the discal spot. As with the NGP (Nijhout, 1991, 2001), the discal spot depicted on the arctiid archetype is a relatively simple character that is always located on the discalis cross-vein. The van Bemmelen band is a unique trait that has no counterpart on the modern version of nymphalid groundplan. It is located immediately adjacent to the central symmetry system’s distal band. This character is composed of smaller spotted elements (sometimes merged into a band-like column) that can be located directly on top of the wing veins (Gawne and Nijhout, 2019). The final character shown on the arctiid archetype is the terminal band, which generally consists of a series of semi-discrete spots that are positioned between the vein termini along the wing’s distal margin.
The basal and central symmetry systems shown on the arctiitype are likely homologs of their counterparts on the nymphalid groundplan (Gawne and Nijhout, 2019). However, the symmetry systems of the arctiitype differ from those of the NGP in that they are represented as discrete columns of spots, as opposed to fused bands (Gawne and Nijhout, 2019). This has been done to indicate that spotted elements produced by presumptive morphogenetic point sources are the most basic compositional unit of arctiid wing patterns. The banded and bold patterns found in the arctiids are emergent structures that result from the fusion of these smaller elements, as occurs, e.g., in the Heliconius butterflies (Nijhout and Wray, 1988).
In Figure 6, we have used the arctiitype to preliminarily identify homologous pattern elements in the six major classes of tiger moth wing patterns. The archetype itself is shown in the center of this figure, and the pattern types (1) single spot, (2) concentric spot, (3) continuous band, (4) broken band, (5) triple bold, and (6) double bold are shown around its periphery. For each of these classes, we have illustrated the symmetry systems and other primary elements for the first specimen shown in the series. The presumptive pattern homologs have been color-coded to match the appropriate characters in the arctiitype, i.e., the basal symmetry system is shown in blue, the central symmetry system has been colored green, the discal spot is lime, the van Bemmelen band is yellow, and the terminal band is depicted in orange. Immediately adjacent to the stylized graphical figure shown for each type is a photograph of the focal wing it is based on, followed by two additional samples which belong to the same phenotypic class. As shown in Figure 6, the arctiitype can be applied to a diverse array of tiger moth wing patterns in order to identify presumptive pattern homologs within and across species. The fact that the archetype can be used to identify homologous characters enables us to tame what initially appears to a bewildering amount of pattern diversity, and this in turn allows us to ask novel questions about how the dorsal forewing patterns of the arctiids develop and evolve.
Discussion
The arctiid archetype described in the preceding sections is remarkably similar to a scheme proposed by the Dutch biologist van Bemmelen (1918). Van Bemmelen’s paper represents an important intellectual achievement, as it constitutes one of the first attempts to identify pattern homologies within the Lepidoptera, and subsequently use the presence of these characters to describe and systematize interspecific phenotypic diversity. Unfortunately, his groundbreaking study was almost immediately lost to history (but see Hegna, 2013; Hegna and Mappes, 2014). Within a decade of Van Bemmelen’s work, Schwanwitsch (1924) and Süffert (1927) independently published the comparative analysis of butterfly color patterns that eventually became encapsulated as the nymphalid groundplan (Nijhout, 1991). Although Schwanwitsch showed that his groundplan was also broadly applicable to the moths, neither he nor Süffert seems to have paid much attention to Van Bemmelen’s model, noting it merely as one of several prior works that pointed out the significance of crossbands as a fundamental organizing principle of lepidopteran color patterns. A decade later, Sokolow (1936) published a lengthy study of the evolution of color patterns in the Arctiidae, but his work was based on that of Schwanwitsch, and gave only a glancing nod to van Bemmelen’s study. Sokolow’s analysis was largely focused on what we have referred to as the “bold” patterns, and used Schwanwitch’s coding nomenclature. Although we only became aware of van Bemmelen’s work recently, we had independently arrived at many of the same conclusions that he reached about the morphological structure of tiger moth dorsal forewing patterns [cf. (Nijhout, 1991) on the parallel and independent development of the NGP by Süffert and Schwanwitsch].
At first glance, the dorsal forewing patterns found in the tiger moths are almost bafflingly diverse – some have spots, while others have roughly parallel bands, or contiguous monochromatic patches of pigmentation, and the overall structure of a pattern can be radically altered through the addition of detailed elements (Figure 8). To complicate things even further, the incomplete fusion of primary pattern elements often produces isolated regions of background coloration that can easily be mistaken for novel characters (Figure 9). Like van Bemmelen, we have attempted to systematize the pattern diversity observed in the arctiids by taking a reductionist approach which assumes that the patterns of all these animals are composed of a limited number of common compositional units: serially repeated spots arranged in columns [somewhat misleadingly referred to as “rows” by Gawne and Nijhout (2019)] that run anterior-posterior across the wing’s surface. Comparative analyses of pattern morphology using large numbers of specimens strongly suggests that the bands found in arctiid wing patterns are emergent “pseudocharacters” that arise as a byproduct when intervenous spots fuse with their anterior/posterior neighbors. Developmental studies have revealed that the intervenous spots found in lepidopteran color patterns are produced by diffusible morphogens that spread out from point sources located between the wing veins (Nijhout, 1991; French and Brakefield, 1992; Monteiro et al., 2001). This being so, banded phenotypes are predicted to be produced when: (a) the secretory cells which constitute the point sources produce a larger quantity of the morphogen, or (b) the receiving tissue becomes more sensitive to this signal, such that a lower concentration of the chemical suffices to initiate pigment synthesis in the scale cells.
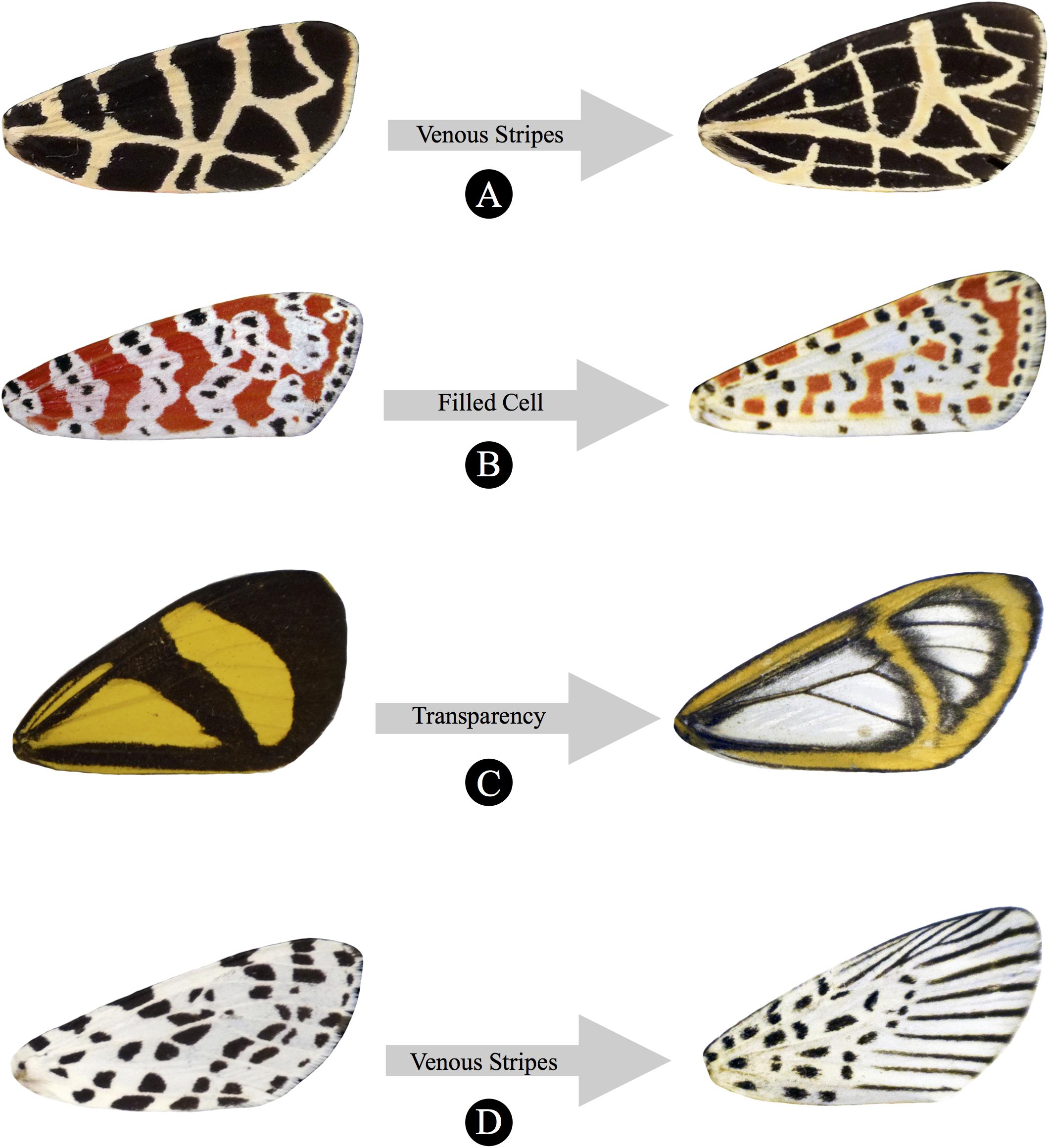
Figure 8. The diversity observed in tiger moth wing patterns is not attributable solely to changes in the size, shape, and position of the five archetypical characters. Detailed elements can be added to dorsal forewing patterns, and their inclusion often radically changes its appearance. Row (A) shows how the inclusion of overlaid venous stripes can alter pattern morphology (left: Apantesis nevadensis; right: Apantesis ornata). Row (B) illustrates how a filled discal wing cell can significantly alter a color pattern’s appearance (left: Utetheisa ornatrix; right: Utetheisa pulchella). As shown in row (C), the replacement of various pattern features with transparency can be a source of pattern diversity (left: Ephestris melaxantha; right: Hyalurga fenestra). With H. fenestra, the black primary characters have become transparent, but in some wasp-mimicking species, it appears that the background coloration has been modified to become transparent. Finally, the animals shown in row (D), demonstrate that the addition of venous stripes can change the overall structure and appearance of the pattern (left: Hypercompe permaculata; right: Agrisius guttivitta).
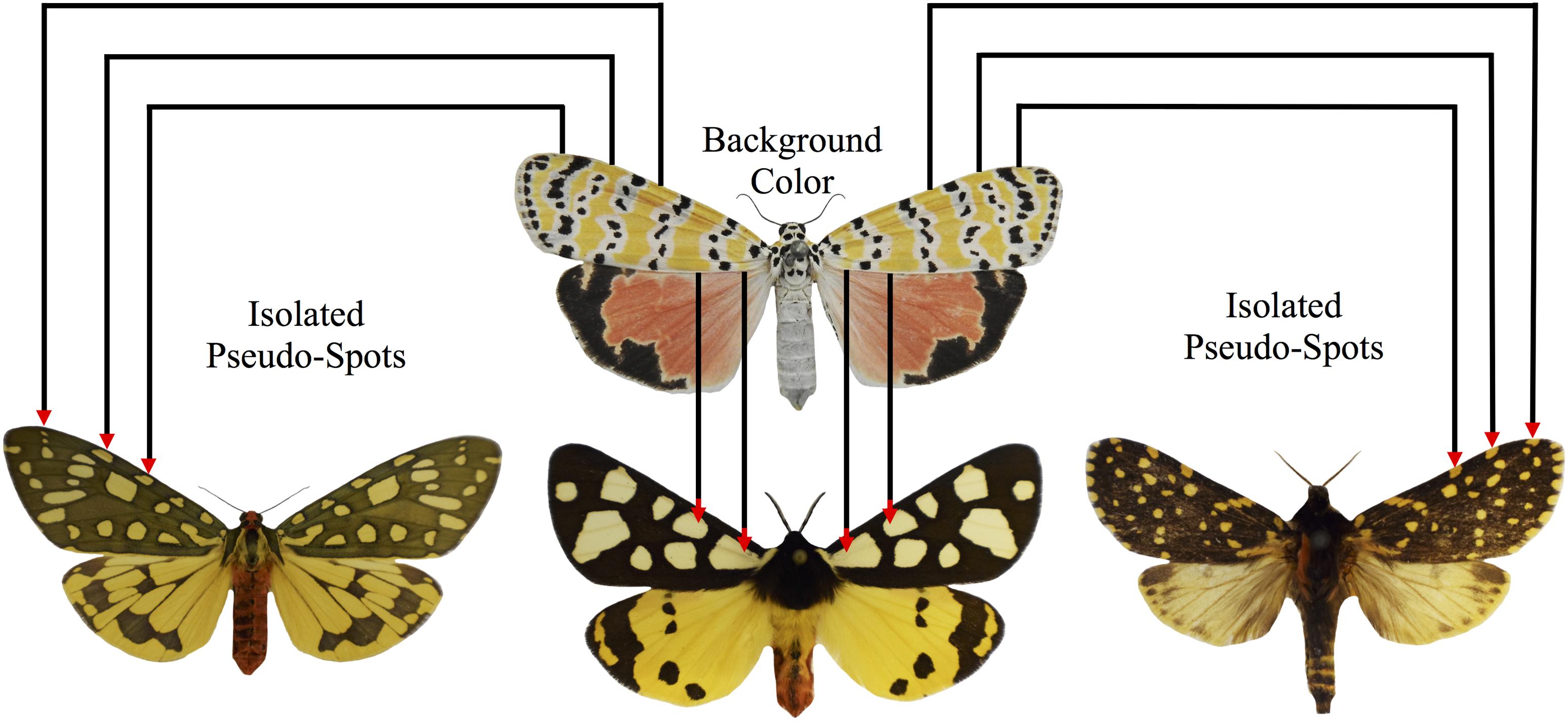
Figure 9. “Pesudospots” are islands of background coloration formed through the incomplete fusion of primary pattern elements. In some species of tiger moths, it is possible to identify apparent spotted elements that are not depicted on the arctiitype. We predict that these are not true developmentally individuated characters, but instead, are byproducts that arise accidentally through the incomplete fusion of the primary pattern elements. A sample specimen of U. ornatrix displaying broad patches of orange background coloration that separate the various primary pattern elements is shown at the top of the figure. In the bottom row (left: Aglaomorpha plagiata; middle: Arctia villica.; right: Phaegoptera aurogutta), the corresponding patches of background coloration have been transformed into pseudospots due to increases in the size of the primary pattern elements, the inclusion of venous stripe details, and changes in overall wing geometry.
We hypothesize that bold patterns arise in a similar manner. The continuous melanic regions observed in patterns of this type are produced when multiple columns of spots belonging to either the same or different systems, fuse with one another in a proximo-distal direction. Patterns of this type could be formed through changes in the way morphogens are secreted and received, but in addition, we predict that their development is dependent upon alterations to the wing’s overall shape. Venous junction points on the wing provide positional information which functions to “anchor” the primary characters to one region of the wing, rather than another. If the distance between the junction points is reduced, then all else being equal with the morphogen point sources, we would expect the characters to fuse in a distal-proximal direction, and produce a bold pattern. Experimental studies of the relevant morphogens, and quantitative analyses of wing shape will be needed to confirm the validity of this hypothesis, which is readily testable using existing molecular, statistical, and image processing tools.
Like van Bemmelen, we believe that the identification of large-scale trends in tiger moth patterning is heavily dependent upon our ability to draw a distinction between the primary pattern elements depicted in Figure 5, and the remaining portion of the wing that is now commonly referred to as “background coloration” (Nijhout, 1991). The separation of ground and foreground is essential (Figure 9) because it enables researchers to communicate using a common language, and allows for experimental reproducibility. Failure to do this has often led to semantic difficulties, especially with bold patterns such as those found in the Heliconius butterflies [(Nijhout and Wray, 1988), but see Jiggins (2017) for an alternative interpretation]. When a distinction between ground (background coloration) and foreground (pattern) is not made, it is extremely difficult to homologize pattern elements (Nijhout and Wray, 1988), and perhaps more importantly, there is a tendency to treat isolated patches of background color, e.g., the “Belem spot” and “Ecuador triangle” in Heliconius, as individuated characters (Nijhout et al., 1990; Brakefield and French, 1993; Morris et al., 2019) [see Van Valen (1982) for discussion of an analogous problem in comparative dental morphology]. In the tiger moths, the background coloration is often a bright color such as red, orange or yellow, and the primary pattern elements tend to be black, brown, or white. As the literature on tiger moth color patterns grows, it will be essential for researchers to agree on a common terminology, and then consistently stick with the conventional designations in order to avoid unproductive disagreements pertaining to the assignment of homologies.
Although we find ourselves to be in agreement with van Bemmelen on many issues, there is at least one important difference between his system and the archetype we have proposed. According to van Bemmelen, “the color-pattern of Arctiidae [can] be deduced from an ancestral fundamental form, in which a light ground is divided into seven fields by a corresponding number of transverse rows of dark spots” (van Bemmelen, 1918, p. 8). The pattern depicted on the arctiitype (Figure 5) is composed of seven columns of spots, and thus it would appear that we are in agreement with him on this issue. However, our analysis assumes that the discal spot is a unique character, whereas van Bemmelen combined this element with the central symmetry system, and counted certain discontinuous pattern elements twice. In many tiger moths, the distal portion of the central symmetry system is broken and “pierellized” (Nijhout, 1991) posterior to the cell formed by the M3 and CU1 veins, giving the impression that this unified character is actually two separate elements. A previously published study (Gawne and Nijhout, 2019) speaks against such an interpretation. Our comparative studies of tiger moth color patterns suggest that they composed of five primary characters which, in their more discretized states, divide the wing into a total of seven “fields”: (i) two columns that comprise the basal symmetry system, (ii) two columns that comprise the central symmetry system, (iii) a discal spot, and a pair of distal elements that we have designated the (iv) van Bemmelen, and (v) terminal bands.
As mentioned earlier, the basal symmetry system, central symmetry system, and discal spot are likely homologs of similarly designated pattern elements that have been identified in the butterflies (Nijhout, 1991), and other groups of moths (Schwanwitsch, 1956). This judgment is based on the spatial positioning of these elements on the wing—the basal symmetry system is located near the wing hinge, the discal spot is positioned on the discalis vein, and the central symmetry system lies on either side of the discal spot—and some relatively uncontroversial assumptions about the conservation of developmental mechanisms. That being said, there is no denying that the symmetry systems found in the arctiids are visually distinct from those present in the butterflies. As described above, the basal and central symmetry systems of tiger moth color patterns are likely produced by, and often manifest phenotypically as serially repeated spots. Conversely, in the butterflies these characters tend to take the form of continuous bi-colored bands that are mirror images of one another (Nijhout, 1991; Otaki, 2012). These phenotypic differences are significant, but do not in themselves provide evidence that the characters in question are non-homologous.
What matters in the ascription of homology are shared developmental mechanisms (Roth, 1984; Wagner, 2014), and like the pattern elements found in the tiger moths, butterfly symmetry systems are known to be produced by serially repeated intervenous morphogen point sources. We hypothesize that the gene regulatory networks that produce correlations in the development of the spots which jointly compose the basal symmetry system, central symmetry system, and discal spot are implicated in the formation of these characters in both the butterflies and tiger moths. Under such circumstances, these pattern elements would be homologs. To put the situation in slightly different terms, we are suggesting that the central symmetry system found in a tiger moth such as U. ornatrix and a focal species of butterfly represent different character states of the same underlying character identity (Wagner, 2014, 2016). Given that we currently have very little data on color pattern development in the tiger moths, it is difficult to make any sort of conclusive homology assignments, but the morphology and positioning of the various elements on the wing is highly suggestive, and we expect that molecular studies will eventually vindicate our claims of homology.
Although the tiger moths and butterflies have several common pattern elements, they also differ in at least two respects. Specifically, arctiid color patterns: (a) lack both border ocelli and parafocal elements, and (b) contain two unique elements not found in the patterns of the butterflies, i.e., the van Bemmelen band and the terminal band. The absence of the border ocelli is hardly surprising – these characters have never been documented outside of the Rhopalocera, and likely emerged as an evolutionary novelty within this clade (Oliver et al., 2014). Similarly, given that the parafocal elements are thought to develop in conjunction with the border ocelli (Nijhout, 1991, 2017), the absence of the former characters is also predictable. What makes tiger moth color patterns interesting is not the absence of these elements, but the fact that they appear to have characters that are not present in the butterflies. The van Bemmelen band is of special interest developmentally because in species such as U. ornatrix, the spots that compose this character are located directly on top of the wing veins. In the butterflies, details such as venous stripes are positioned on the veins, but all of the primary characters except the discal spot develop exclusively in the interstitial region between the wing veins. It is possible that in the tiger moths the column of spots we have referred to as the van Bemmelen band (a primary character) is actually a series of truncated venous stripes (a detailed element). However, the fact that U. ornatrix and other species are known to develop non-truncated venous stripes (Figure 4) which sometimes occur in conjunction with the van Bemmelen band’s co-located spots seems to speak against such an interpretation.
Additionally, there are species such as Hypercompe permaculata (Figure 2, Row A, left specimen) in which the apparent van Bemmelen band is composed of spots that are positioned between the wing veins. With this species, none of the primary pattern elements other than the discal spot are located on top of the veins. This could mean that the location of the spots comprising the van Bemmelen band is indeterminate, i.e., they have the potential to exist either on or between the wing veins. However, we believe that an alternative explanation is more plausible. In the butterflies, intervenous pattern elements are known to break apart, and disassociate from one another (Nijhout, 1991). This can mistakenly give the impression that a particular species or individual possesses a novel character(s). Knowing that this is possible in the Rhopalocera, we suggest that H. permaculata and similar species could lack a van Bemmelen band. Under such circumstances, the intervenous spots positioned where this structure would normally be are dissociated portions of either the distal band of the central symmetry system, or the neighboring terminal band. These, and other peculiarities associated with the van Bemmelen band are intriguing puzzles that represent an opportunity for future research.
One important issue that has not yet been discussed is the relationship between the arctiid archetype and the nymphalid groundplan. As mentioned earlier, the NGP is a conceptual tool that allows us to make sense of the pattern diversity observed in lepidopteran color patterns. When Schwanwitsch (1924) and Süffert (1927) were originally developing this model, they looked at a large number of nymphalid butterflies, and identified every novel pattern element they could find in this family. Each of these characters was subsequently depicted on the groundplan in order to allow for the identification of homologous elements across the Nymphalidae. Later, Schwanwitch (Schwanwitsch, 1956) would add additional characters to the NGP in order to make it more broadly applicable to other species of butterflies and moths, including the arctiids. When the NGP was resurrected in the 1970s, it was primarily in the context of developmental research of butterfly color patterns (Nijhout, 1991; Otaki, 2012) and accordingly, the archetype was modified yet again. This time, a number of elements found in the moths were removed, and new features such as the marginal and sub-marginal bands were added (Nijhout, 1991; Otaki, 2012).
The point in recounting all of this is to emphasize that the NGP is a theoretical construct that we are free to update in light of emerging data, or alter as we see fit in order to suit the needs of a particular research program. In its modern form, the NGP has been streamlined to allow for ease of use in the context of evolutionary and developmental studies of butterfly color patterns (Nijhout, 1991; Gawne and Nijhout, 2019). One way to make the NGP applicable to tiger moth wing patterns is to return to an earlier incarnation of the model which included the characters that Schwanwitsch (1956) referred to as “umbrae” and “externae.” These pattern elements appear to correspond to the van Bemmelen and terminal bands described above, and it is worth emphasizing again that researchers such as Sokolow (1936) successfully applied an early version of the NGP to the arctiids. However, in order to avoid unnecessary confusion caused by the surplus of characters on this rendition of the groundplan, it seems more prudent to proceed by crafting a new model that depicts all and only those characters that are present in the dorsal forewing patterns of the tiger moths. The arctiitype fits this description, and allows for the identification of homologous pattern elements in the roughly 11,000 existing species of arctiids (Weller et al., 2009).
Before concluding, it is important to emphasize that the arctiid archetype we have presented is much more than a tool for understanding the morphological structure of arctiid wing patterns. To date, the vast majority of research on color pattern development has focused on the butterflies, and few would dispute that this emphasis has made it difficult to construct truly generalized ontogenetic models that are applicable to the Lepidoptera as a whole. The arctiid archetype has the potential to help remedy this situation by allowing us to accurately describe and quantify the results of experimental studies designed to interfere with the mechanisms that drive pattern development in these animals. Ecological and evolutionary research on arctiids such as U. ornatrix (Conner et al., 1981; Dussourd et al., 1988; LaMunyon and Eisner, 1993; Egan et al., 2016) and the wood tiger Arctia plantaginis (Nokelainen et al., 2012, 2014; Henze et al., 2018; Rojas et al., 2019) has been ongoing for decades, and the associated literature touches on a number of fundamentally important theoretical issues in evolutionary biology, including aposematic signaling, mimetic resemblance, and sexual selection. Given that both U. ornatrix and A. planaginis can be reared in the lab, an opportunity exists for developmental biologists to provide ontogenetic context for these innovative evolutionary studies. Moreover, since the arctiids are so species rich, new model systems chosen specifically for their heuristic value in developmental contexts are undoubtedly waiting to be introduced. Tiger moths are widely revered as aesthetic objects, but they are much more than visually striking curiosities. These animals are a scientific resource that remains almost completely untapped.
Data Availability Statement
The datasets generated for this study are available on request to the corresponding author.
Author Contributions
RG and HN jointly conceived of the ideas presented, and contributed equally to the final manuscript.
Funding
This work was supported by the grant IOS-1557341 from the National Science Foundation.
Conflict of Interest
The authors declare that the research was conducted in the absence of any commercial or financial relationships that could be construed as a potential conflict of interest.
Acknowledgments
We thank Jameson Clarke, Laura Grunert, and Ken McKenna for their input and assistance during various stages of this project. Audiences at the National Center for Biological Sciences (NCBS) and the University of Jyväskylä provided stimulating feedback and helpful criticisms of the ideas presented. Comments from two anonymous Frontiers reviewers significantly improved the quality of the final manuscript. We are grateful to the curators and staff at the Smithsonian National Museum of Natural History, the Vienna Museum of Natural History, and the Hungarian Museum of Natural History for allowing us to use their collections. Much of this research was completed during a postdoctoral fellowship at the Konrad Lorenz Institute for Evolution and Cognition Research awarded to RG, who adds special thanks to the KLI staff/fellows and Katharina Aigner for their support during the final months of his stay.
Footnotes
- ^ We are aware that the family Arctiidae has recently been reclassified as the sub-family Arctiinae (Zahiri et al., 2012). However, this re-classification has not yet become universally adopted and the new terminology is largely unknown to researchers in evolutionary and developmental biology. For this reason, we have chosen to retain terms such as “Arctiidae” and “arctiid,” but in doing so we are not intending to make any specific taxonomic claims.
References
Allen, W. L., Cuthill, I. C., Scott-Samuel, N. E., and Baddeley, R. (2011). Why the leopard got its spots: relating pattern development to ecology in felids. Proc. R. Soc. B Biol. Sci. 278, 1373–1380. doi: 10.1098/rspb.2010.1734
Beldade, P., and Brakefield, P. M. (2002). The genetics and evo–devo of butterfly wing patterns. Nat. Rev. Genet. 3, 442–452. doi: 10.1038/nrg818
Caro, T., Izzo, A., Reiner, R. C., Walker, H., and Stankowich, T. (2014). The function of zebra stripes. Nat. Commun. 5, 1–10.
Conner, W. E., Eisner, T., Vander Meer, R. K., Guerrero, A., and Meinwald, J. (1981). Precopulatory sexual interaction in an arctiid moth (Utetheisa ornatrix): role of a pheromone derived from dietary alkaloids. Behav. Ecol. Sociobiol. 9, 227–235. doi: 10.1007/bf00302942
Dussourd, D. E., Ubik, K., Harvis, C., Resch, J., Meinwald, J., and Eisner, T. (1988). Biparental defensive endowment of eggs with acquired plant alkaloid in the moth Utetheisa ornatrix. Proc. Natl. Acad. Sci. U.S.A. 85, 5992–5996. doi: 10.1073/pnas.85.16.5992
Egan, A. L., Hook, K. A., Reeve, H. K., and Iyengar, V. K. (2016). Polyandrous females provide sons with more competitive sperm: support for the sexy-sperm hypothesis in the rattlebox moth (Utetheisa ornatrix). Evolution 70, 72–81. doi: 10.1111/evo.12829
French, V., and Brakefield, P. M. (1992). The development of eyespot patterns on butterfly wings: morphogen sources or sinks? Development 116, 103–109.
Gawne, R., and Nijhout, H. F. (2019). Expanding the nymphalid groundplan’s domain of applicability: pattern homologies in an arctiid moth (Utetheisa ornatrix). Biol. J. Linn. Soc. 126, 912–924. doi: 10.1093/biolinnean/bly193
Hegna, R. (2013). Geographic Variation in the Warning Signals of the Wood Tiger Moth (Parasemia plantaginis; Arctiidae). Jyväskylän: University of Jyväskylä.
Hegna, R. H., and Mappes, J. (2014). Influences of geographic differentiation in the forewing warning signal of the wood tiger moth in Alaska. Evol. Ecol. 28, 1003–1017. doi: 10.1007/s10682-014-9734-7
Henze, M. J., Lind, O., Mappes, J., Rojas, B., and Kelber, A. (2018). An aposematic colour-polymorphic moth seen through the eyes of conspecifics and predators–Sensitivity and colour discrimination in a tiger moth. Funct. Ecol. 32, 1797–1809. doi: 10.1111/1365-2435.13100
Jiggins, C. D. (2017). The Ecology and Evolution of Heliconius Butterflies. Oxford: Oxford University Press.
Jonathan, B. B. (1977). A unity underlying the different zebra striping patterns. J. Zool. 183, 527–539. doi: 10.1111/j.1469-7998.1977.tb04204.x
LaMunyon, C. W., and Eisner, T. (1993). Postcopulatory sexual selection in an arctiid moth (Utetheisa ornatrix). Proc. Natl. Acad. Sci. U.S.A. 90, 4689–4692. doi: 10.1073/pnas.90.10.4689
Martin, A., and Reed, R. D. (2014). Wnt signaling underlies evolution and development of the butterfly wing pattern symmetry systems. Dev. Biol. 395, 367–378. doi: 10.1016/j.ydbio.2014.08.031
Mazo-Vargas, A., Concha, C., Livraghi, L., Massardo, D., Wallbank, R. W., Zhang, L., et al. (2017). Macroevolutionary shifts of WntA function potentiate butterfly wing-pattern diversity. Proc. Natl. Acad. Sci. U.S.A. 114, 10701–10706. doi: 10.1073/pnas.1708149114
Monteiro, A. (2008). Alternative models for the evolution of eyespots and of serial homology on lepidopteran wings. Bioessays 30, 358–366. doi: 10.1002/bies.20733
Monteiro, A., French, V., Smit, G., Brakefield, P. M., and Metz, J. A. (2001). Butterfly eyespot patterns: evidence for specification by a morphogen diffusion gradient. Acta Biotheor. 49, 77–88.
Monteiro, A., Glaser, G., Stockslager, S., Glansdorp, N., and Ramos, D. (2006). Comparative insights into questions of lepidopteran wing pattern homology. BMC Dev. Biol. 6:52. doi: 10.1186/1471-213X-6-52
Morris, J., Navarro, N., Rastas, P., Rawlins, L. D., Sammy, J., Mallet, J., et al. (2019). The genetic architecture of adaptation: convergence and pleiotropy in Heliconius wing pattern evolution. Heredity 123, 138–152. doi: 10.1038/s41437-018-0180-0
Nijhout, H. F. (1978). Wing pattern formation in Lepidoptera: a model. J. Exp. Zool. 206, 119–136. doi: 10.1002/jez.1402060202
Nijhout, H. F. (1991). The Development and Evolution of Butterfly Wing Patterns. Washington, DC: Smithsonian Institution.
Nijhout, H. F. (2001). Elements of butterfly wing patterns. J. Exp. Zool. 291, 213–225. doi: 10.1002/jez.1099
Nijhout, H. F. (2017). “The common developmental origin of eyespots and parafocal elements and a new model mechanism for color pattern formation,” in Diversity and Evolution of Butterfly Wing Patterns, eds T. Sekimura and H. Nijhout (Singapore: Springer), 3–19. doi: 10.1007/978-981-10-4956-9_1
Nijhout, H. F., and Wray, G. A. (1988). Homologies in the colour patterns of the genus Heliconius (Lepidoptera: Nymphalidae). Biol. J. Linn. Soc. 33, 345–365. doi: 10.1111/j.1095-8312.1988.tb00449.x
Nijhout, H. F., Wray, G. A., and Gilbert, L. E. (1990). An analysis of the phenotypic effects of certain colour pattern genes in Heliconius (Lepidoptera: Nymphalidae). Biol. J. Linn. Soc. 40, 357–372. doi: 10.1111/j.1095-8312.1990.tb00545.x
Nokelainen, O., Hegna, R. H., Reudler, J. H., Lindstedt, C., and Mappes, J. (2012). Trade-off between warning signal efficacy and mating success in the wood tiger moth. Proc. R. Soc. B Biol. Sci. 279, 257–265. doi: 10.1098/rspb.2011.0880
Nokelainen, O., Valkonen, J., Lindstedt, C., and Mappes, J. (2014). Changes in predator community structure shifts the efficacy of two warning signals in Arctiid moths. J. Anim. Ecol. 83, 598–605. doi: 10.1111/1365-2656.12169
Oliver, J. C., Beaulieu, J. M., Gall, L. F., Piel, W. H., and Monteiro, A. (2014). Nymphalid eyespot serial homologues originate as a few individualized modules. Proc. R. Soc. B Biol. Sci. 281:20133262. doi: 10.1098/rspb.2013.3262
Otaki, J. M. (2012). Color pattern analysis of nymphalid butterfly wings: revision of the nymphalid groundplan. Zool. Sci. 29, 568–576. doi: 10.2108/zsj.29.568
Rojas, B., Mappes, J., and Burdfield-Steel, E. (2019). Multiple modalities in insect warning displays have additive effects against wild avian predators. Behav. Ecol. Sociobiol. 73:37.
Schachat, S. R., and Brown, R. L. (2015). Color pattern on the forewing of Micropterix (Lepidoptera: Micropterigidae): insights into the evolution of wing pattern and wing venation in moths. PLoS One 10:e0139972. doi: 10.1371/journal.pone.0139972
Schachat, S. R., and Brown, R. L. (2016). Forewing color pattern in Micropterigidae (Insecta: Lepidoptera): homologies between contrast boundaries, and a revised hypothesis for the origin of symmetry systems. BMC Evol. Biol. 16:116. doi: 10.1186/s12862-016-0687-z
Schachat, S. R., and Goldstein, P. Z. (2018). Acronictinae (Lepidoptera: Macroheterocera: noctuidae) demonstrate the variable role of wing venation in the evolution of the nymphalid groundplan. Insect Syst. Divers. 2:4.
Schwanwitsch, B. (1924). “21. On the ground-plan of wing-pattern in nymphalids and certain other families of the rhopaloeerous lepidoptera,” in Proceedings of the Zoological Society of London, (London: Wiley Online Library).
van Bemmelen, J. (1918). The wing-marking of Arctiidae. Koninklijke Nederlandse Akademie van Weteschappen Proc. Ser. B Phys. Sci. 20, 849–860.
Van Valen, L. M. (1982). Homology and causes. J. Morphol. 173, 305–312. doi: 10.1002/jmor.1051730307
Wagner, G. P. (2014). Homology, Genes, and Evolutionary Innovation. Princeton, NJ: Princeton university press.
Wagner, G. P. (2016). What is homology thinking and what is it for? J. Exp. Zool. B Mol. Dev. Evol. 326, 3–8. doi: 10.1002/jez.b.22656
Weller, S., DaCosta, M., Simmons, R., Dittmar, K., and Whiting, M. (2009). “Evolution and taxonomic confusion in Arctiidae,” in Tiger Moths and Woolly Bears, Behavior, Ecology, and Evolution of the Arctiidae, ed. W. E. Conner (New York, NY: Oxford University Press), 11–30.
Keywords: color pattern evolution and development, nymphalid groundplan, arctiid archetype, Arctiidae, arctiid moths, tiger moths
Citation: Gawne R and Nijhout HF (2020) The Arctiid Archetype: A New Lepidopteran Groundplan. Front. Ecol. Evol. 8:175. doi: 10.3389/fevo.2020.00175
Received: 29 February 2020; Accepted: 18 May 2020;
Published: 10 June 2020.
Edited by:
Marcus Kronforst, The University of Chicago, United StatesReviewed by:
Antonia Monteiro, National University of Singapore, SingaporeJoji M. Otaki, University of the Ryukyus, Japan
Copyright © 2020 Gawne and Nijhout. This is an open-access article distributed under the terms of the Creative Commons Attribution License (CC BY). The use, distribution or reproduction in other forums is permitted, provided the original author(s) and the copyright owner(s) are credited and that the original publication in this journal is cited, in accordance with accepted academic practice. No use, distribution or reproduction is permitted which does not comply with these terms.
*Correspondence: H. Frederik Nijhout, hfn@duke.edu