- 1Department of Biological Sciences, University of Notre Dame, Notre Dame, IN, United States
- 2Department of Entomology, Virginia Tech, Blacksburg, VA, United States
- 3Cary Institute of Ecosystem Studies, Millbrook, NY, United States
- 4Department of Biology, Loyola University, Chicago, Chicago, IL, United States
Headwaters suffer from reduced leaf and wood inputs and retention capacity from historical land actions like watershed logging and agriculture. When in-stream wood is reduced, stream retention capacity declines and subsequent changes in streamwater flow-paths and patterns of deposition alter decomposition and primary production that influence secondary invertebrate production via modified habitat and resources. Wood additions are commonly used as stream restoration tools for habitat improvements that can restore or strengthen food web connections; however, changes in carbon (C) flow through food webs are rarely measured because of time and expense. We quantified allochthonous and autochthonous C flow through aquatic macroinvertebrate communities 1 year before and 2 years after an experimental addition of large wood, compared to macroinvertebrates in an upstream control, in a temperate headwater stream. We predicted wood additions increase macroinvertebrate consumption and assimilation of allochthonous and autochthonous C through retention of leaves and altered flow-paths that expose more gravel and cobble for periphyton colonization. Macroinvertebrate allochthonous C assimilation tended to increase in years with greater organic matter retention and autochthonous C increased with more exposed gravel and cobble across seasons and between reaches. While the effect of wood addition on C flow through the macroinvertebrate community was minimal, it increased by ∼20% relative to the control from an increase in production and C assimilation of common mayfly and caddisfly scrapers, Baetis and Glossossoma. Because the amount of organic matter retained and coarse substrate exposed corresponded with C form and amount consumed, restoration of large wood has the potential to increase organic matter C trophic transfer.
Introduction
Freshwater ecosystems cover only a small fraction of Earth’s surface but receive at least 1.9 Pg carbon yr–1 from terrestrial ecosystems. At least 50% of these carbon (C) inputs are stored in or emitted from freshwaters (Cole et al., 2007). Consequently, cross-ecosystem terrestrial-aquatic exchanges are essential for understanding global C cycling (Cole et al., 2007). Stream food webs are strongly influenced by leaf-litter and wood from adjacent riparian areas. Changes in riparian detrital quality and quantity dictate aquatic biological community structure, organismal growth, and organismal lifecycle completion rates, described as organismal performance (Wallace et al., 1997b). Collectively, this community performance governs aquatic ecosystem functions like secondary production and decomposition (Webster et al., 1997). In turn, in-stream secondary production and decomposition govern the capacity of small streams to transfer and transport C that subsidizes downstream and riparian communities.
Human actions in forested watersheds can change the timing or amount of allochthonous and autochthonous C that affect the organisms available to consume and transfer C (Cummins et al., 1989). For example, greater retention of allochthonous material in streams stimulate growth of fungi and bacteria, and organic matter consumption by macroinvertebrates (Richardson, 1991; Negishi and Richardson, 2003; Tiegs et al., 2008), increasing the relative amount of allochthonous versus autochthonous C transferred through the stream food web (Rosemond et al., 1993). However, increases in water velocity can increase exposure of large substrates subsequently covered in sand and stimulate periphyton growth (Kail, 2002), increasing the relative contribution of autochthonous C to secondary consumers like macroinvertebrates (McNeely et al., 2007). Large wood results in both organic matter retention and substrate sorting via modified flow paths. Therefore, adding wood to streams can change available habitat and food resources for aquatic biota. The concurrent increase in autochthonous and allochthonous C in low-production, forested streams could lead to an overall increase in C contributions to secondary macroinvertebrate production, resulting in greater trophic transfer and more trophic linkages.
Attempts to restore headwater streams through a bottom-up organic matter addition (e.g., addition of large wood) provide stability and greater resource availability that could also alter the relative terrestrial- and instream-derived C eaten and assimilated by aquatic heterotrophs (e.g., Rosi-Marshall and Wallace, 2002). Forested headwater streams have historically been considered to be strongly influenced by the volume and timing of terrestrially derived allochthonous material delivered to the stream (Polis and Strong, 1996; Wallace et al., 1997b; Moore et al., 2004). However, algae can also be seasonally important in many temperate streams (Finlay, 2001; Hall et al., 2001). The alternating increase of algae in winter when the canopy is more open and leaf litter inputs retained in autumn may act to stabilize the food web when resources may otherwise be scarce (Power et al., 1988; Polis and Strong, 1996; Moore et al., 2004; Guo et al., 2016). We are unaware of any studies that have quantified allochthonous C (i.e., terrestrially derived) versus autochthonous C (algal-derived) following in-stream wood addition.
Ecosystem processes that restorations aim to affect include rates of nutrient uptake (Sudduth et al., 2011), organic matter retention, and decomposition (Lepori et al., 2005; Frainer et al., 2017). These functional metrics are especially useful in restoration studies because they reveal how changes in physical structure may influence the rate, and pathway of energy or elements flowing through an ecosystem. Stable isotopes can be used to identify the source and amount of C assimilated by a target community and are increasingly used in restorations (for example, Fry, 2002; Kennedy et al., 2005; Lepori et al., 2006). For example, Lepori et al. (2006) added boulders to several streams in Sweden that increased detrital retention, but did not result in consumers δ13C more similar to the retained detritus.
Still, stable isotopes may identify food source assimilated and can be used in combination with measures of community structure and secondary production to develop quantitative food webs (e.g., Rosi-Marshall and Wallace, 2002). Quantitative food webs can reveal consumer-level controls on ecological processes and illustrate changes in trophic structure (Rosi-Marshall and Wallace, 2002), community assemblage, and nutrient flow (Cross et al., 2007)following ecosystem restoration. Tracking pathways of energy flow through the food web integrates changes in food resource assimilation, community structure, survival, and production (Benke and Wallace, 2011). Carbon flow measurements are commonly used to test ecological theory (e.g., Cross et al., 2007), but have not been used to assess restorations.
We quantified allochthonous and autochthonous C assimilation in macroinvertebrates before and after experimental wood addition in a Michigan headwater stream using natural abundance of C isotopes for macroinvertebrates and their food resources. Then we combined estimates of C assimilation with secondary production (Entrekin et al., 2009) to calculate trophic basis of production (Benke and Wallace, 1997). Finally, by using assumed assimilation efficiencies, we were able to back-calculate the amount of allochthonous and autochthonous C consumed (expressed as a rate) and compare that C flow with the amount available. Our previous work in this stream showed that total macroinvertebrate secondary production was low before wood addition, but increased by ∼25% 2 years after wood addition, resulting in a statistically significant increase in invertebrate biomass and greater secondary invertebrate production (Entrekin et al., 2009). Here, we sought to quantify how assimilation, trophic basis of production and C allochthonous and autochthonous C flowing through the macroinvertebrate food web changed after wood was added. We predicted wood addition would increase consumption and assimilation of allochthonous and autochthonous C by macroinvertebrates through an increased retention of leaf litter, and the exposure of sand-covered large inorganic substrates for periphyton colonization.
Study Site
State Creek is a 1st-order stream draining 3.9 km2 in the Ottawa National Forest in the Ontonagon River basin in the Upper Peninsula of Michigan, United States (46° 28′N, 89° 1′W). We began sampling monthly in May 2003, 1 year before wood addition (Y0) and continued for 1 (Y1) and 2 (Y2) years after wood addition in both the wood-added (treatment) and the upstream control reach. Treatment and control reaches were separated by a 50 m distance to promote some independence between sites, while reducing differences associated with longitudinal changes. We measured no difference in the amount of in-stream wood between the control and treatment reaches prior to the wood addition (Entrekin et al., 2007). The stream section we studied had 90% canopy cover during spring and summer and 75% canopy cover in autumn and winter, an average bank-full width of 2.4 m in the control reach and 2.5 meters in the treatment reach, an average water depth at base flow of 13 cm in the control reach and 12 cm in the treatment reach, and an average discharge in the control reach of 64 and 67 L s–1 in the treatment reach (for more details see Entrekin et al., 2007; Hoellein et al., 2009). The stream flows through a managed, second-growth forest with intact, but young, riparian vegetation in a catchment of 95% deciduous forest that was last logged in 1967. Riparian trees include Populus tremuloides Michx (trembling aspen), Acer rubrum L. (red maple), Acer saccharum Marsh. (sugar maple), Betula papyrifera Marsh. (paper birch), Tsuga canadensis L. (hemlock), Pinus alba L. (white pine), with a thick understory of Alnus serrulata Alt. (tag alder). The study stream had low in-stream large wood density (13 pieces/100 m stream length) and low storage of coarse benthic organic matter [annual average of 146 ± 70 (SE) g AFDM m–2] before wood addition (Cordova et al., 2007; Entrekin et al., 2007) from a history of region-wide logging and shale mining (Webster et al., 2008).
In May 2004, we added 25 logs (each 2.5 m long × 0.5-m diameter) of big tooth aspen P. grandidentata Michx., purchased from a nearby tree farm, haphazardly to a 100-m stream reach, while maintaining a 100 m upstream control reach. After 2 years in the stream, 15 of the 25 logs moved. Most of the added logs moved less than two meters with one moving the farthest at 18 m (G. Lamberti unpublished data). None of the added wood moved out of the study reaches. We did measure an increase in the amount of organic matter retained and more exposed cobble from a localized increase in water velocity caused by the added logs (Entrekin et al., 2008).
Materials and Methods
Habitat Characteristics
Standing crops of coarse (CBOM) and fine (FBOM) benthic organic matter were measured from five 804-cm2 benthic cores sampled in each reach on each sampling date; CBOM was separated from FBOM using a 1-mm sieve. After CBOM was removed from the corer, a FBOM slurry was made by stirring the sediment in the core and then subsampled using a 160 mL specimen container. Subsamples were stored on ice until processing. In the laboratory, samples for CBOM were dried at 60°C, sorted by organic matter type (leaves, moss, and wood), and weighed. A subsample of each organic matter type was then combusted at 550°C, and reweighed to determine ash-free dry mass (AFDM; Benfield 2006). For FBOM, subsamples were filtered onto glass fiber filters (GF/F), dried at 60°C, weighed, combusted at 550°C, and reweighed for AFDM.
On each sampling date, we also measured discharge and velocity from the dilution of a conservative tracer during concurrent measurements of whole-stream nutrient uptake rates (Hoellein et al., 2007). Water temperature was recorded at the bottom of each stream reach hourly from May 2003–May 2006 using HOBO® data loggers (Onset Computer Corporation, Bourne, MA, United States). We also surveyed benthic habitat using transects spaced every 5 m (perpendicular to flow) in both reaches in May and August of 2003 (before wood addition) and in May, July, and November 2004–2006 (after wood addition). Sediments were categorized using the Wentworth scale (Minshall and Rugenski, 2006). Inorganic sediments were classified as boulders, gravel and cobble, and sand, while organic substrates were moss, CBOM (>1 mm), silt, small wood (<10 cm), and large wood (>10 cm). Measurements were recorded every 20 cm across the channel. We calculated percent cover for each substrate at each transect-scale, and as the mean of each category across all transects combined (i.e., reach-scale).
Measuring δ13C Natural Abundance Signature
We measured δ13C values for the most productive taxa that collectively represented ∼90% of the total macroinvertebrate community production (Entrekin et al., 2009). Macroinvertebrate abundance, community composition, and secondary production were measured from monthly (none collected in January) Hess (32 cm diameter, 250 μm-mesh) samples (5 per stream reach) in the treatment and control reaches for the 5-year study period. For secondary production, size-frequency histograms were developed for each taxon and corrected using cohort production intervals. For rare taxa, we used either production to biomass ratios we developed or published values (Entrekin et al., 2009). We then used a sub-set of those individuals for stable isotope analysis. Samples were preserved in 6–8% formalin, which we note may result in a 1–1.65‰ systematic depletion of δ13C across taxa (Sarakinos et al., 2002; Bicknell et al., 2011). However, a comparison of δ13C differences between frozen and formalin-preserved samples for four dominant taxa in one season showed no consistent change (S. Entrekin, unpublished data). Despite some inorganic C, we did not acidify samples because inorganic C was low and acidifying can lead to fractionation (Schlacher and Connolly, 2014). To measure C stable isotopes of macroinvertebrates, we selected late instar taxa from March (late winter), May (spring), June or July (summer), and November (autumn) for the three study years to represent possible seasonal changes in taxa. Thus, our macroinvertebrate sampling incorporated seasonal variation in macroinvertebrate diet as well as any potential changes due to the wood addition. For each stable isotope measurement, the number of individuals representing a single taxon varied based on an individual’s mass (i.e., 2–20 individuals). When possible, we used individuals from at least three different replicate Hess cores that were taken haphazardly along each 100-m reach to incorporate reach-scale variation and expressed as averages with standard error (see Supplementary Appendix 1). Finally, we measured the δ13C signature of macroinvertebrate food resources including conditioned leaves (i.e., leaf litter colonized by bacteria and fungi) and algae from grab samples in the selected months. Conditioned leaves were collected along each stream reach from State Creek in spring, summer, autumn, and winter and frozen until analysis. Periphyton samples were also collected seasonally by scraping multiple rocks throughout the stream reach.
Macroinvertebrate taxa and food resources were dried at 60°C, ground to a fine powder, and analyzed on a Finnigan Delta Plus Stable Isotope Mass Spectrometer. Stable isotope values were expressed in δ notation as the difference in parts per thousand (‰) from a standard (PeeDee Belemnite), using the calculation: δ13C = (Rsample/Rstandard−1) ∗ 1000; where R is 13C/12C. Our attempts at silica separation (Hamilton et al., 2005) were unsuccessful in isolating low amounts of algae (mostly diatoms) from periphyton biofilm scrapings. Therefore, we used the δ13C value of a known grazer, the caddisfly Glossosoma sp., as the presumed proxy for periphyton, as has been done in other studies (e.g., Finlay, 2001). Glossosoma gut contents were examined each season to verify the presence of algae; however, small amounts of allochthonous C may have been assimilated that would result in an underestimate of the contribution of autochthonous C (to the invertebrate community diet).
Our first goal was to partition allochthonous (i.e., leaf litter) from autochthonous (i.e., in-stream algae) C assimilation by macroinvertebrates as indicated by δ13C signatures using a two-source mixing model rather than using gut content analysis. Therefore, no other food resources were used in the mixing model because leaf litter and epilithic algae (or periphyton) represented the end members (allochthonous versus autochthonous food resources) in this food web. We used the model IsoSource and IsoError from the Environmental Protection Agency1, which has the advantage of accounting for replicate sample variation (Phillips and Gregg, 2001). The δ13C average of conditioned leaf litter was −29.98 ± 0.01‰, and δ13C of algal signature of Glossosoma sp. was 36.94 ± 0.2‰ (upper confidence interval = 1 and lower confidence interval = 0.97) across our sample period, indicating separation of the two C sources. δ13C signatures were not corrected for C fractionation rates as they are likely low and unpredictable (McCutchan et al., 2003).
Relative Allochthonous and Autochthonous C Assimilation and Their Contribution to Production
Then, we used a modified method to quantify the trophic basis of production described by Benke and Wallace (1997, 2011) where the proportional contribution of allochthonous and autochthonous C to assimilation for each taxon, as indicated by stable isotopes, was multiplied by the total production of that taxon to determine the contribution to secondary invertebrate production or the trophic basis of production. The contribution of allochthonous and autochthonous C to production was then calculated for each dominant macroinvertebrate taxa and summarized for each functional feeding group (FFG). The advantage of this method was that assumptions about assimilation efficiency were not needed because natural abundance of isotopes reflect assimilation. However, we were not able to estimate total amount of resources consumed. Therefore, to quantify how much autochthonous and allochthonous food was consumed (i.e., in units of AFDM per m–2 time–1), we divided secondary production by published assimilation efficiencies for detritus and algae and then multiplied that by published net production efficiency (Benke and Wallace, 1997; Hall and Meyer, 1998; Rosi-Marshall and Wallace, 2002) to get autochthonous and allochthonous C consumption (Benke and Wallace, 1997):
Algal or detrital C consumption = (secondary production/assimilation efficiency) ∗net production efficiency where secondary production is expressed as mg dry mass per m–2 yr–1, assimilation efficiency is a proportion (detritus = 0.1 or algae = 0.3), and net production efficiency is a proportion (NPE = 0.5).
Statistical Analyses
We used a Before-After-Control-Impact Analysis of Variance (BACI-ANOVA) (Stewart-Oaten et al., 1986; Underwood, 1992) with a Tukey post hoc when significance at α = 0.05 was met to test for changes in δ13C and the contribution of allochthonous versus autochthonous C for the macroinvertebrate community, functional feeding groups, and for individual taxa. Pearson product moment correlations were used to explore the relationship among δ13C values and environmental parameters that may contribute to variation across stream reaches through time (e.g., organic matter standing crops and % substrate cover). All data were tested for conformance to homogeneity of variance using Levene’s test and assumptions of ANOVA using Kolmogorov-Smirnov tests, and transformed when assumptions of normality were not met. All analyses were performed with SAS software (v. 8.02, SAS Institute Inc., Cary, NC, United States).
The amount of C consumed, assimilated and supporting secondary production (i.e., flux or flow) were not replicated measurements; therefore, we did not conduct statistics, but we present and describe the effect size as the difference between the treatment and control reaches before and after wood addition.
Results
Changes in δ13C Signatures After Wood Addition
δ13C ranged from ∼−25‰ to −37‰ across all macroinvertebrate taxa (Supplementary Appendix 1). We were surprised that overall average macroinvertebrate δ13C remained mostly unchanged following wood addition (Figure 1). Before wood addition, the mean (±1 standard error) δ13C signature across all macroinvertebrate taxa differed by ∼1‰ between the control and treatment reaches (Figure 1 and Table 1). The pattern remained after wood addition, and there was no difference in community-level δ13C between the treatment and control reach in Y1 (F3,12 = 0.42, P = 0.74) or Y2 (F3,12 = 0.23, P = 0.87) (Table 1). Similarly, there was no difference in functional feeding group and taxa-specific δ13C between reaches after wood addition in Y1 or Y2 (Table 1).
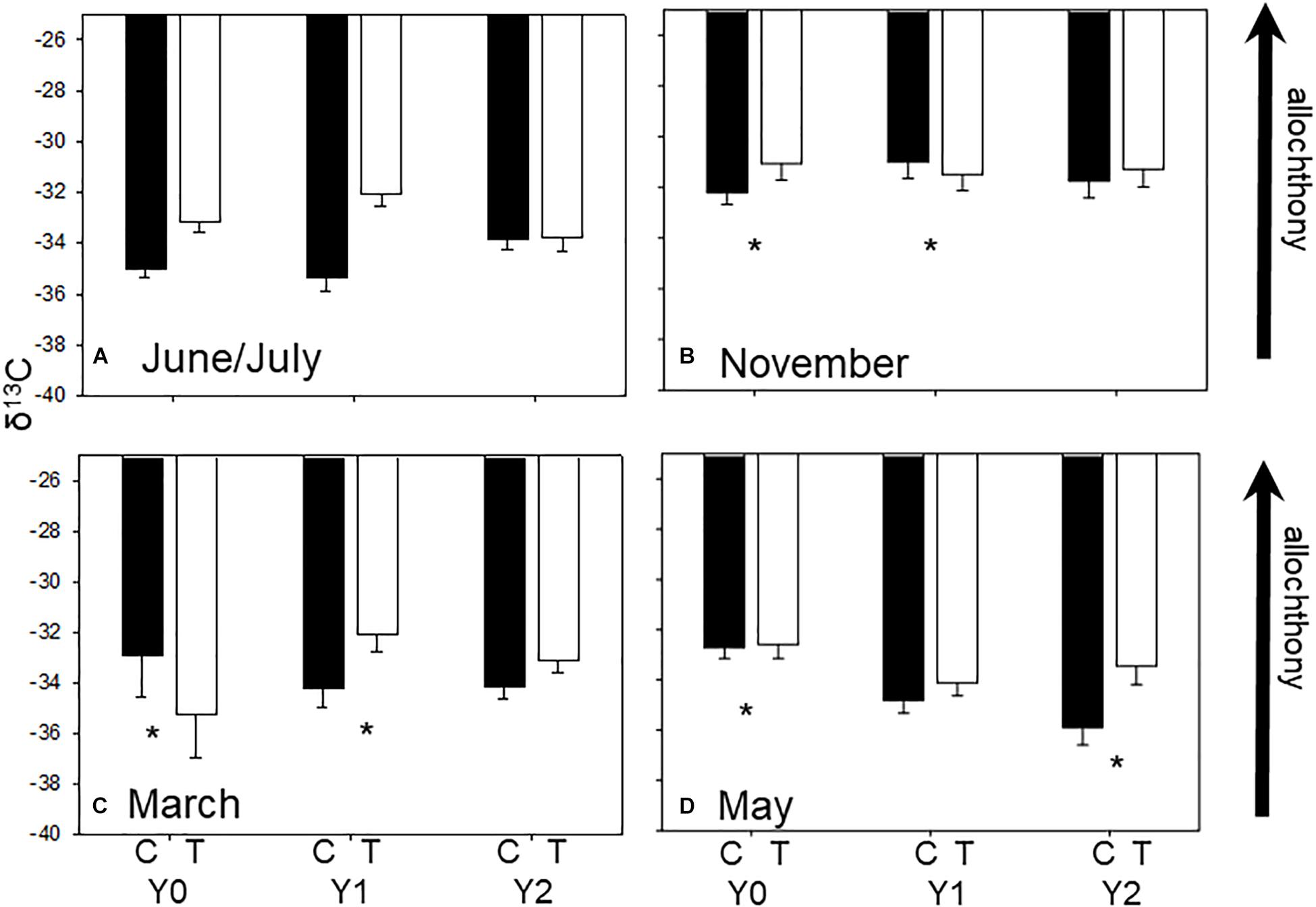
Figure 1. Average (±SE) seasonal δ13C values in each stream reach 1 year before (Y0) and 1 (Y1) and 2 years (Y2) after wood addition in control (C, light bars) and treatment (T, dark bars) in State Creek (Michigan). Dashed lines are average leaf litter δ13C values. * Indicates a significant interaction between control and treatment reaches using ANOVA. (A) Are average seasonal δ13C values from June/July, (B) are average seasonal δ13C values from November, (C) are average seasonal δ13C values from March and (D) are average seasonal δ13C values from May.
Seasonal Changes in δ13C Signatures
Macroinvertebrate δ13C signatures reflected seasonal variation in resources as expected in a temperate headwater stream with signatures closer to leaf-litter in autumn (Supplementary Appendix 1 and Figure 1B) and farther from leaf-litter in March (Figure 1C) when canopy is more open. Community mean δ13C values were different among seasons (F3,8 = 13.54, P < 0.001) with greater δ13C value of −31.79‰ (i.e., closer to leaf litter signature) in autumn (November) and a lower ∼−33.5‰δ13C in winter, spring, and summer (i.e., farther from leaf litter signature) (Figure 1, Tukey’s test P < 0.001).
The δ13C signatures of many individual taxa responded to wood addition differently depending upon taxonomic identity and season, although not necessarily in a predictable manner (Supplementary Appendix 1 and Table 1). For example, Tipula, an obligate shredder, tended to have δ13C closer to leaf litter in the treatment reach relative to the control in Y2 compared to their difference in Y0. A dominant filterer, Simulium, had lower δ13C in the treatment reach in May of Y2 compared to the difference between the control and treatment reach in Y0 (Supplementary Appendix 1, F3,4 = 77.77, p < 0.001). In addition, the predatory stonefly, Isogenoides, had greater δ13C in the treatment reach in autumn of Y1 compared to the difference between the control and treatment reach in Y0 (Table 1, F3,5 = 11.7, p = 0.01). Baetis, among the most productive facultative scraper and gatherers, had greater δ13C in the treatment reach in Y2 winter compared to the difference between the control and treatment reach in Y0 (Supplementary Appendix 1, F3,7 = 18.6, p = 0.001).
Variation in Macroinvertebrate Functional Feeding Group C Assimilation Related to Substrate Composition and Organic Matter Standing Stocks
We correlated allochthonous C assimilation of average community and functional groups with substrate type to identify environmental factors influencing variation across stream reaches and season (Figure 2). The overall % allochthonous C assimilation at the community level was not explained by any one variable, although variation between stream reaches was related to the proportion of sand making up the stream bottom (Figure 2A). The relative amount of allochthonous C assimilated by macroinvertebrate FFGs across season and reaches was mostly correlated to differences in available coarse substrate and organic matter standing crop. The overall proportion of allochthonous C assimilation by shredders, filterers, and gatherers was positively related to the mean annual coarse organic matter standing crop (Figures 2B,C,E). In contrast, scrapers, that feed mostly on inorganic substrates, ranged from less than 1 to 15% allochthonous C assimilation. The proportion of terrestrially derived C assimilated by predators closely tracked gatherer assimilation of allochthonous C (Figure 2F).
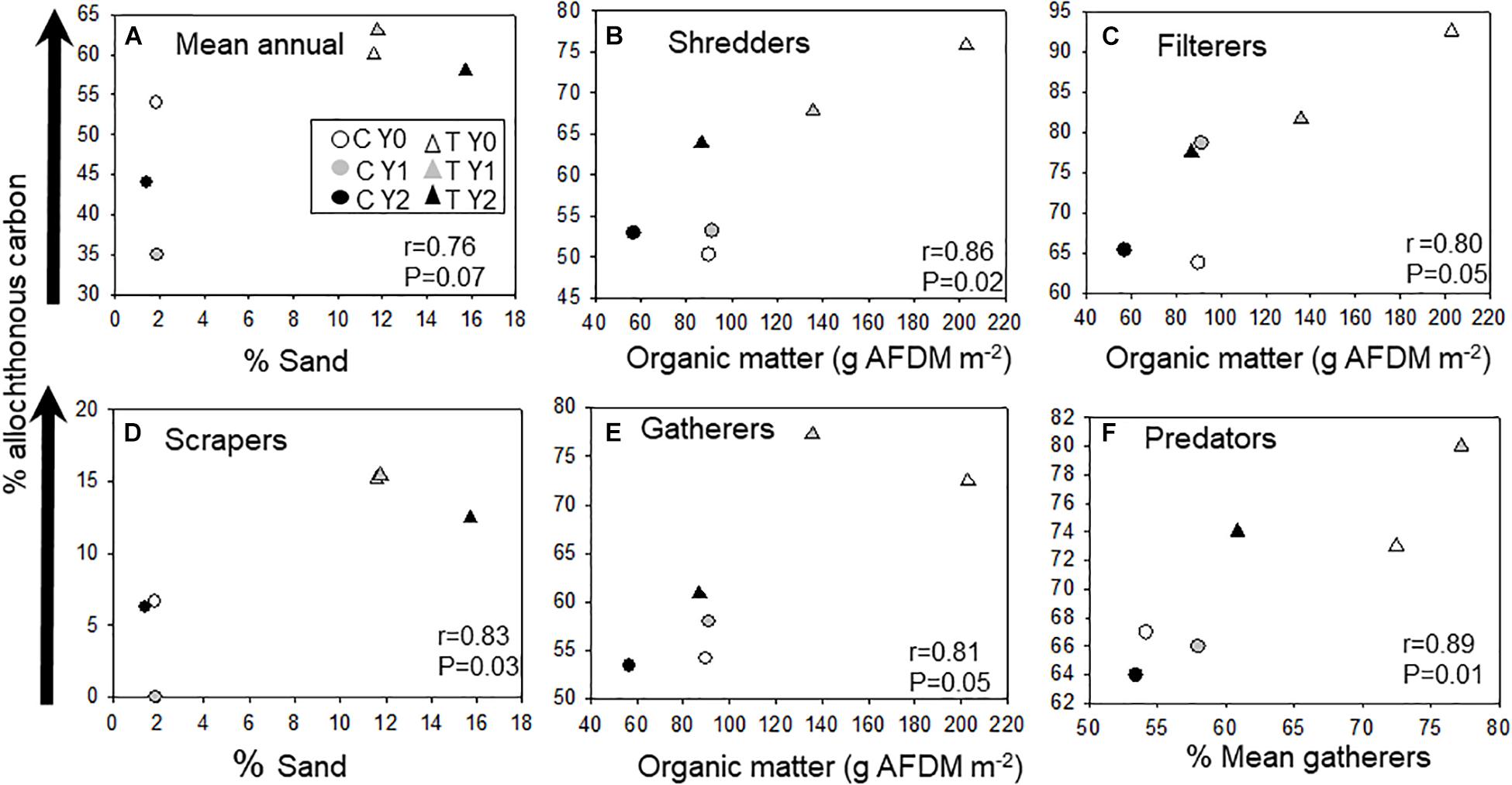
Figure 2. Pearson product moment correlations between (A) annual average macroinvertebrate community δ13C values, (B) macroinvertebrate shredders, (C) filterers, (D) scrapers, (E) filterers, and (F) predators and environmental variables. Control (C) upstream reach without wood addition and treatment (T) with wood added. Arrow indicates a gradient of the proportional increase in allochthonous C assimilation. Organic matter is coarse benthic organic matter fractions > 1 mm.
All Functional Feeding Groups Consumed Allochthonous and Autochthonous C Sources, but FFGs Consumed Different Amounts of the C Sources
Allochthonous and autochthonous-based C contributed roughly equal parts to macroinvertebrate production in the control and treatment reaches, but with little change following wood addition. Annual contribution of allochthonous- C ranged from 35 to 44% in the control reach and 41–56% in the treatment reach over the 3-year study (Figure 3A). The origin of C assimilated by FFGs differed among groups as expected. Autochthonous-based C contributed the most to scraper production, ranging from 70 to 100%. However, allochthonous-based C contribution decreased in scrapers in Y1 relative to the difference between the control and treatment in Y0 (Figure 3B). For shredders, contribution of allochthonous C ranged from 50 to 90%, varying among years, reach, and taxa (Figure 3C and Supplementary Appendix 1). Contribution of allochthonous C to gatherer production increased in Y1 in the treatment reach relative to the difference between the control and treatment in Y0, ranging from 58 to 66% in the control reach and 72 to 88% in the treatment reach (Figure 3D). Allochthonous C contribution to filterer production increased in both reaches in Y1 and declined back to pre-wood addition values in Y2 (60–90%; Figure 3E). Allochthonous C contribution to predator production ranged from 62 to 77% and changed very little following the addition of wood (Figure 3F).
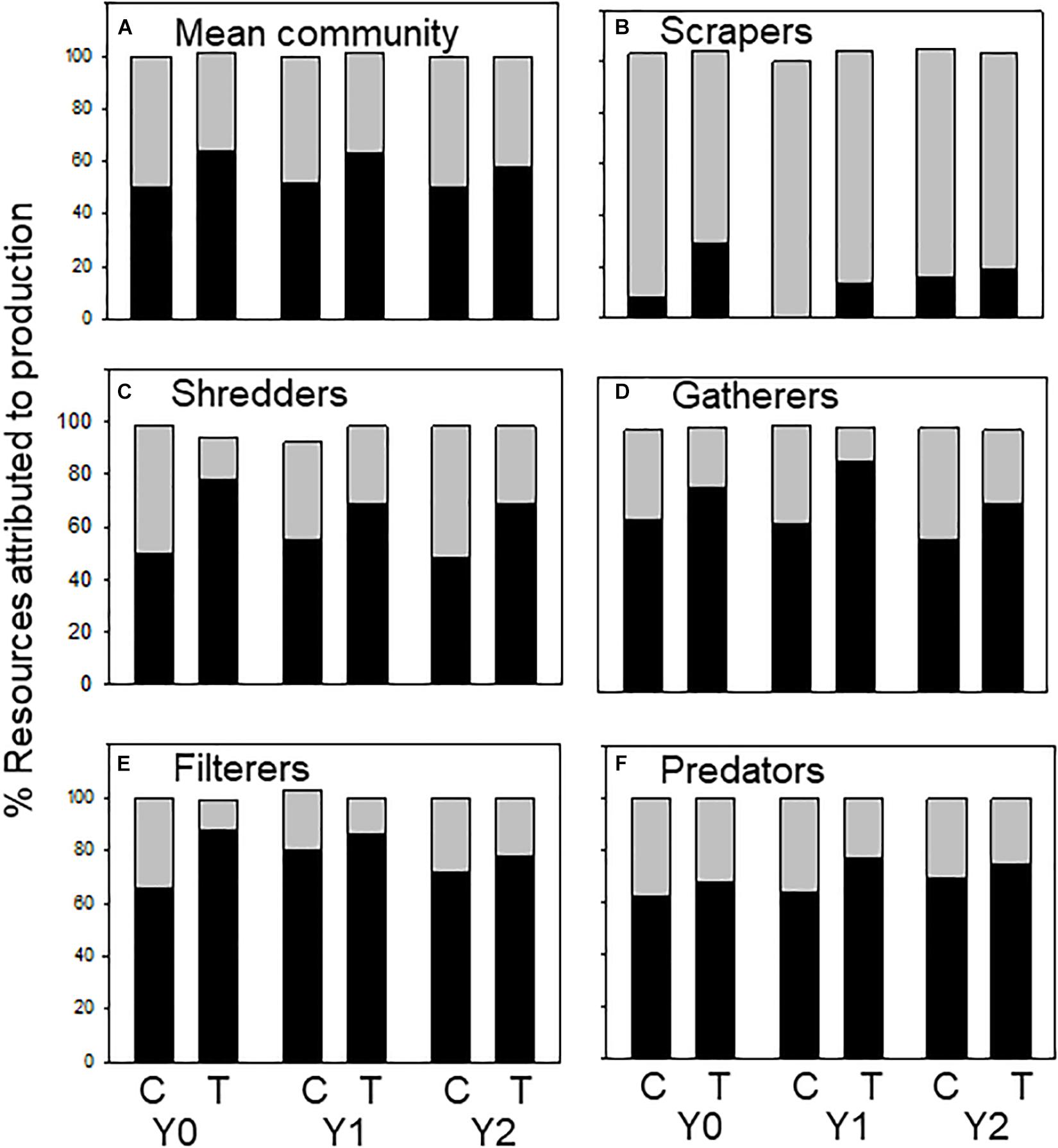
Figure 3. Percent allochthonous (dark bars) and autochthonous (light bars) C contributing to macroinvertebrate secondary production (categorized by functional feeding groups) in an upstream control C and downstream wood-added reach (treatment, T) 1 year before (Y0) and 1 (Y1) and 2 years (Y2) after wood addition. Values may not equal 100% because percentages were averaged across taxa within functional feeding groups (FFGs). (A) % resources attributed to total macroinvertebrate production, (B) % resources attributed to scraper production, (C) % resources attributed to shredder production, (D) % resources attributed to gatherer production, (E) % resources attributed to filterer production, and (F) % resources attributed to predator production.
Actual Allochthonous-Based and Autochthonous-Based C Contributing to Macroinvertebrate Production
In the control reach, allochthonous and autochthonous production declined each year of the study. In contrast, allochthonous and autochthonous -based production in the treatment reach was identical in Y0 and Y2 but lower in Y1 (Table 2 and Figure 4A). All FFG secondary production declined in Y1, while scraper and shredder production increased beyond Y0 values. Autochthonous-based scraper production was lowest in the treatment reach in Y1 and greatest in the treatment reach in Y2 (Figure 4B). Changes in scraper production in Y2 of the treatment reach were driven by an increase in mayfly Baetis production and C assimilation changed from mostly autochthonous to more allochthonous (Table 2). Allochthonous based shredder production was also lowest in Y1 and greatest in the treatment reach in Y2 from an increase in Tipula production (Figure 4C). Other FFGs did not show evidence of a treatment effect. Gatherer production was consistent across years in the control reach, but declined in the treatment reach, while filterer production declined across years in both reaches (Figure 4E). Finally, overall predator production and the ratio of allochthonous and autochthonous-based C contributions to production changed little across dates and reaches (Figure 4F).
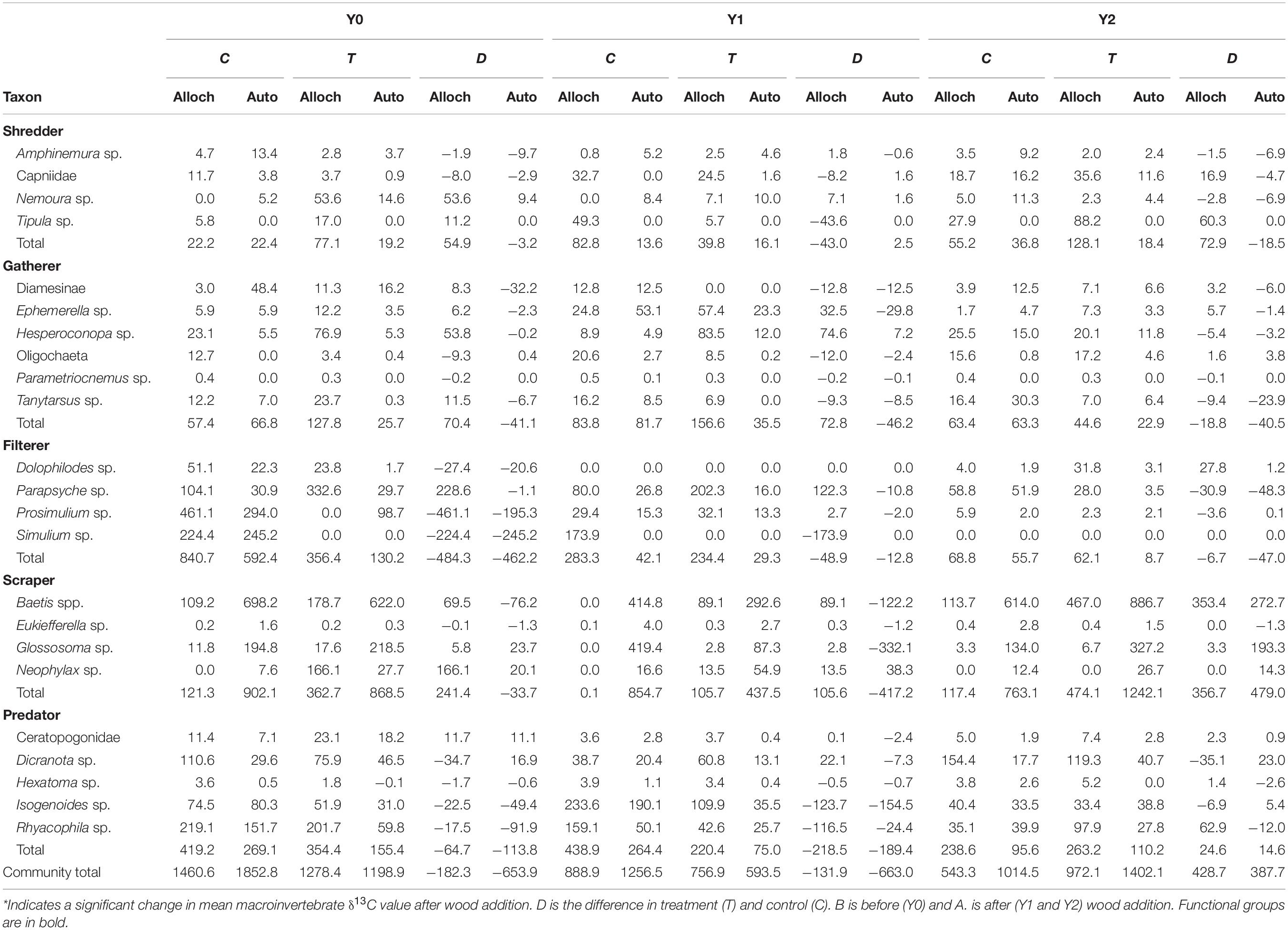
Table 2. Total allochthonous (alloch) or autochthonous (auto) carbon based production (mg AFDM m–2 y–1) of each taxon calculated as production multiplied by the proportion of alloch or auto carbon source assimilated (estimated from δ13C values).
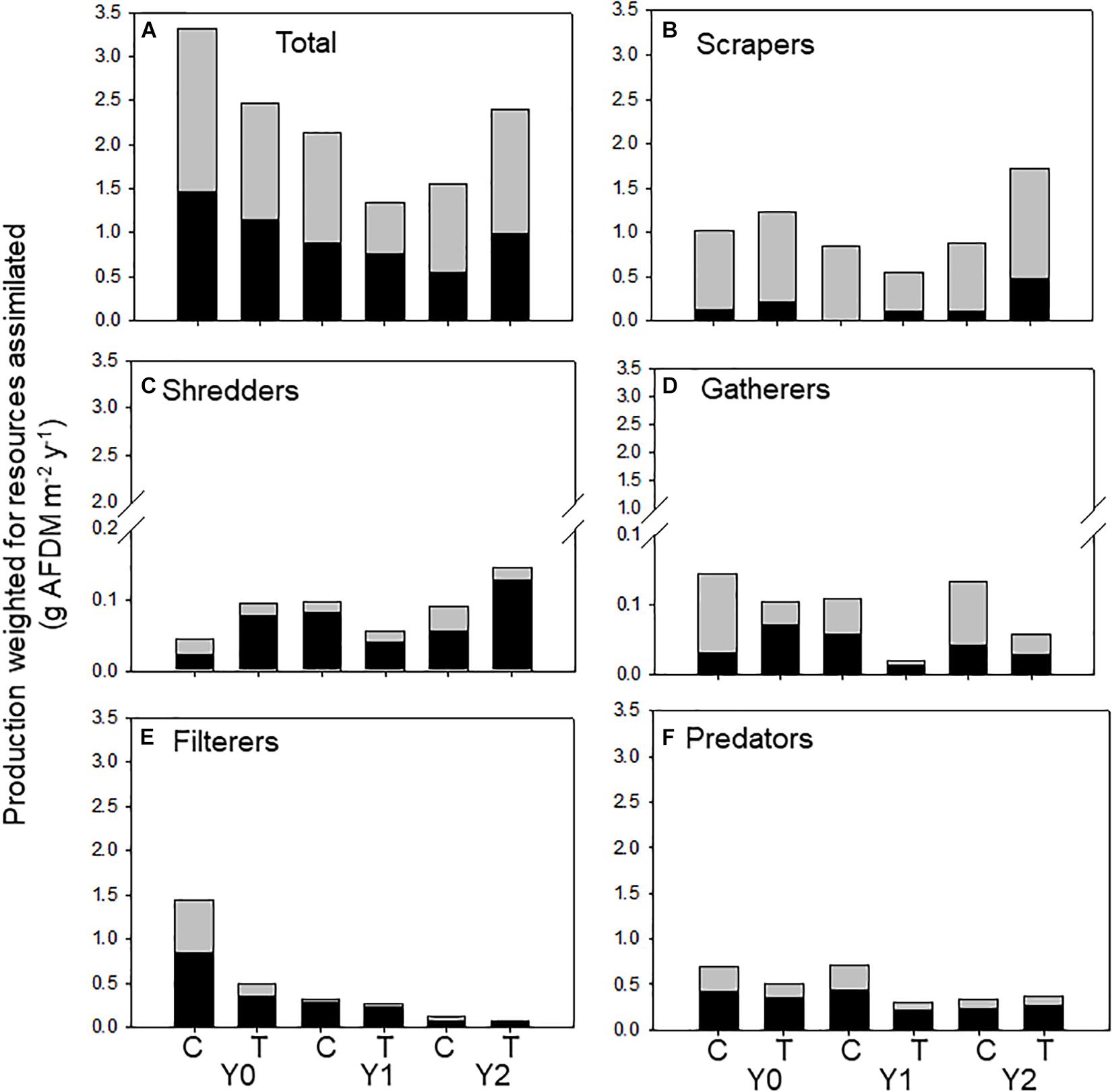
Figure 4. Total macroinvertebrate secondary production supported by allochthonous (dark bars) and autochthonous (light bars) food resources assimilated by macroinvertebrates (categorized by functional feeding groups) in an upstream control C and downstream wood-added reach (treatment, T) 1 year before (Y0) and 1 (Y1) and 2 years (Y2) after wood addition. (A) Is total macroinvertebrate production weighted for resource assimilation, (B) is scraper production weighted for resource assimilation, (C) is shredder production weighted for resource assimilation, (D) is gatherer production weighted for resource assimilation, (E) is filterer production weighted for resource assimilation, and (F) is predator production weighted for resource assimilation.
Consumption of Allochthonous and Autochthonous- C
We calculated the amount of allochthonous and autochthonous C consumed by macroinvertebrates from published assimilation efficiencies to document changes in the amount of each resource flowing through the macroinvertebrate food web. Because leaf litter has a lower assimilation efficiency (0.1) than algae (0.3), macroinvertebrates must consume more allochthonous -based C to support their energetic demands (Benke and Wallace, 1997). Therefore, autochthonous-based C contributed a larger proportion to production, even though autochthonous consumption was less than allochthonous (Figure 5A). All functional feeding groups consumed both C sources. However, scrapers consumed relatively more autochthonous (Figure 5B) and filterers (Figure 5C) consumed more allochthonous C. Shredders (Figure 5D) and gatherers (Figure 5E) consumed mostly allochthonous C and contributed more to overall energy flow by consuming more material to support relatively greater secondary production (Figure 5).
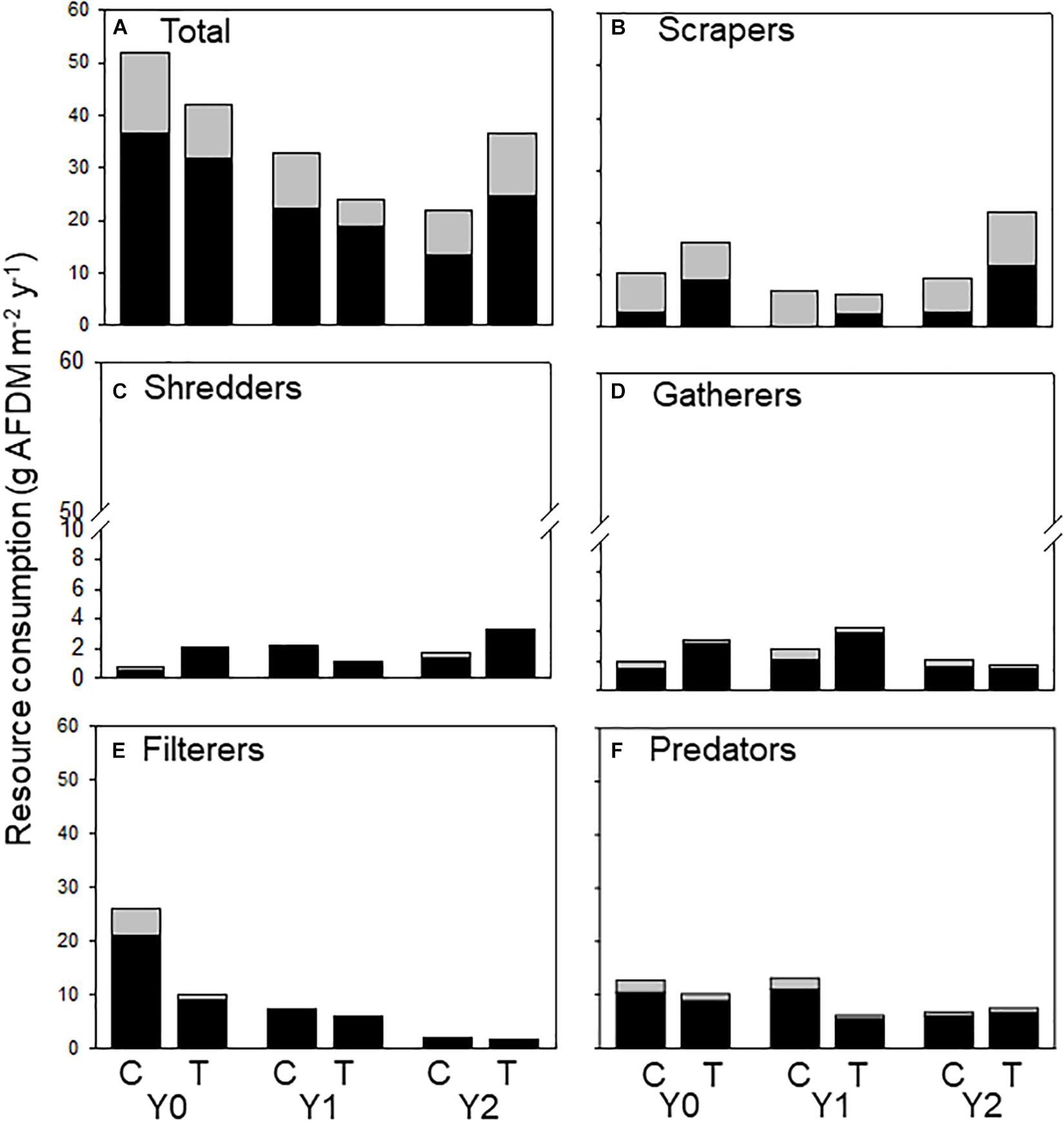
Figure 5. Allochthonous (dark bars) and autochthonous (light bars) C consumed by macroinvertebrates (categorized by functional feeding groups) in an upstream control C and downstream wood-added reach (treatment, T) 1 year before (Y0) and 1 (Y1) and 2 years (Y2) after wood addition. (A) Represents all macroinvertebrate consumption, (B) represents scraper consumption, (C) represents shredder consumption, (D) represents gatherer consumption, (E) represents filterer consumption and (F) represents predator consumption of allochthonous and authochonous carbon.
Energy Flows From Autochthonous and Allochthonous-Based C
In the control reach, the dominant energy flows remained fairly consistent among Y0, Y1, and Y2 (Table 3 and Figure 6). autochthonous C consumed by scrapers remained fairly evenly distributed between Baetis and Glossosoma in each year, with a slight increase to Baetis in Y2 (Figure 6). Allochthonous production also remained constant flowing mostly through the filtering black flies, Simulium, and to a lesser extent a net-spinning caddisfly, Parapsyche, with no changes from Y0 to Y2. In contrast, energy flow in the treatment reach showed much greater variation across years (Figure 6). Scraper basal C flow in Y0 was split between Baetis and Neophylax in the treatment reach and was predominantly autochthonous C sources. In Y1 and Y2 in the treatment reach, allochthonous C contributed the most to Baetis production in Y2 (Figure 6). For shredders, C flow through allochthonous C flow through Tipula was greater in Y2 than in Y0 or Y1.
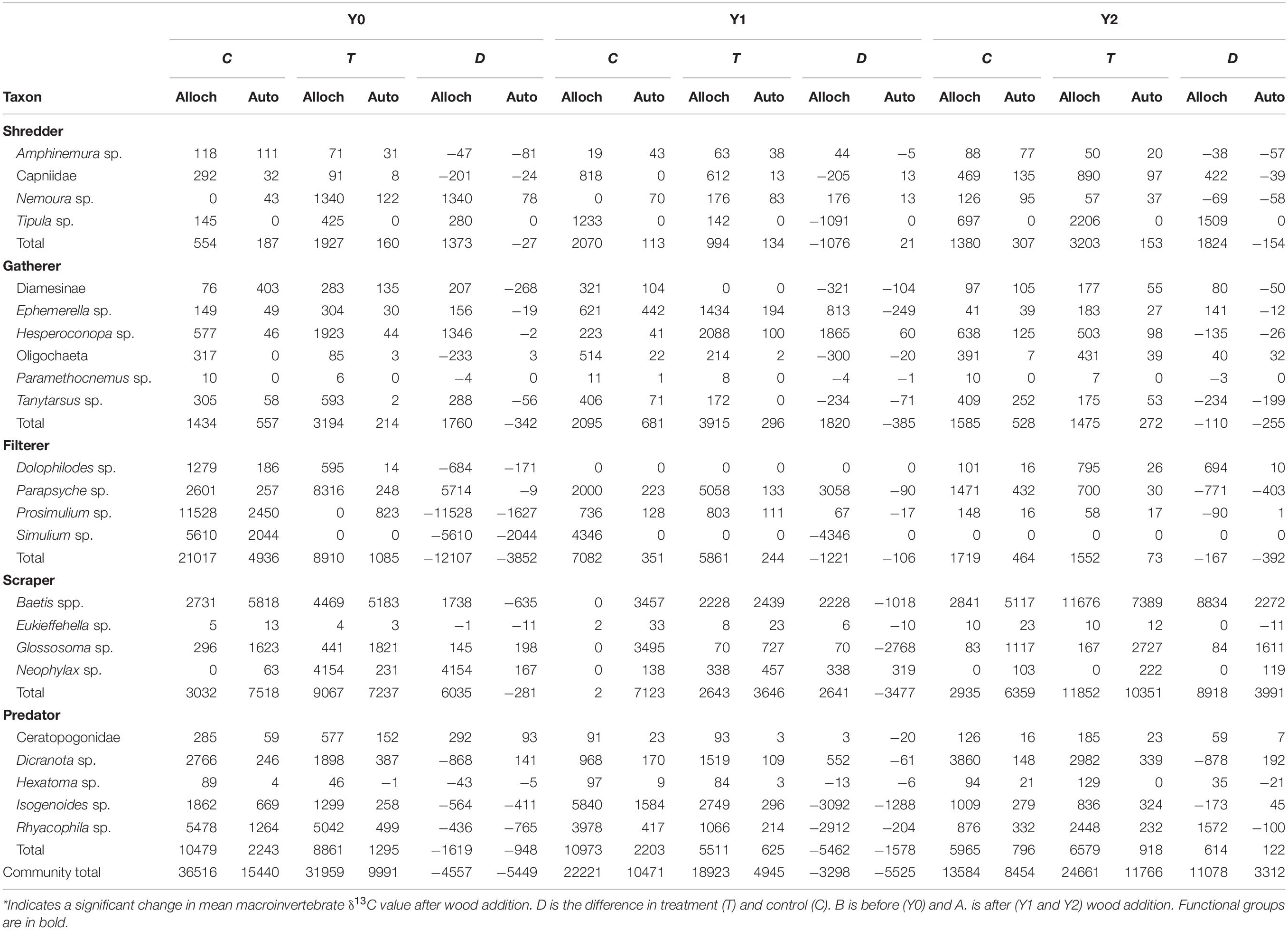
Table 3. Total amount of allocthonous (alloch) and autochthonous (auto) food sources consumed by each taxon (mg AFDM m–2 y–1).
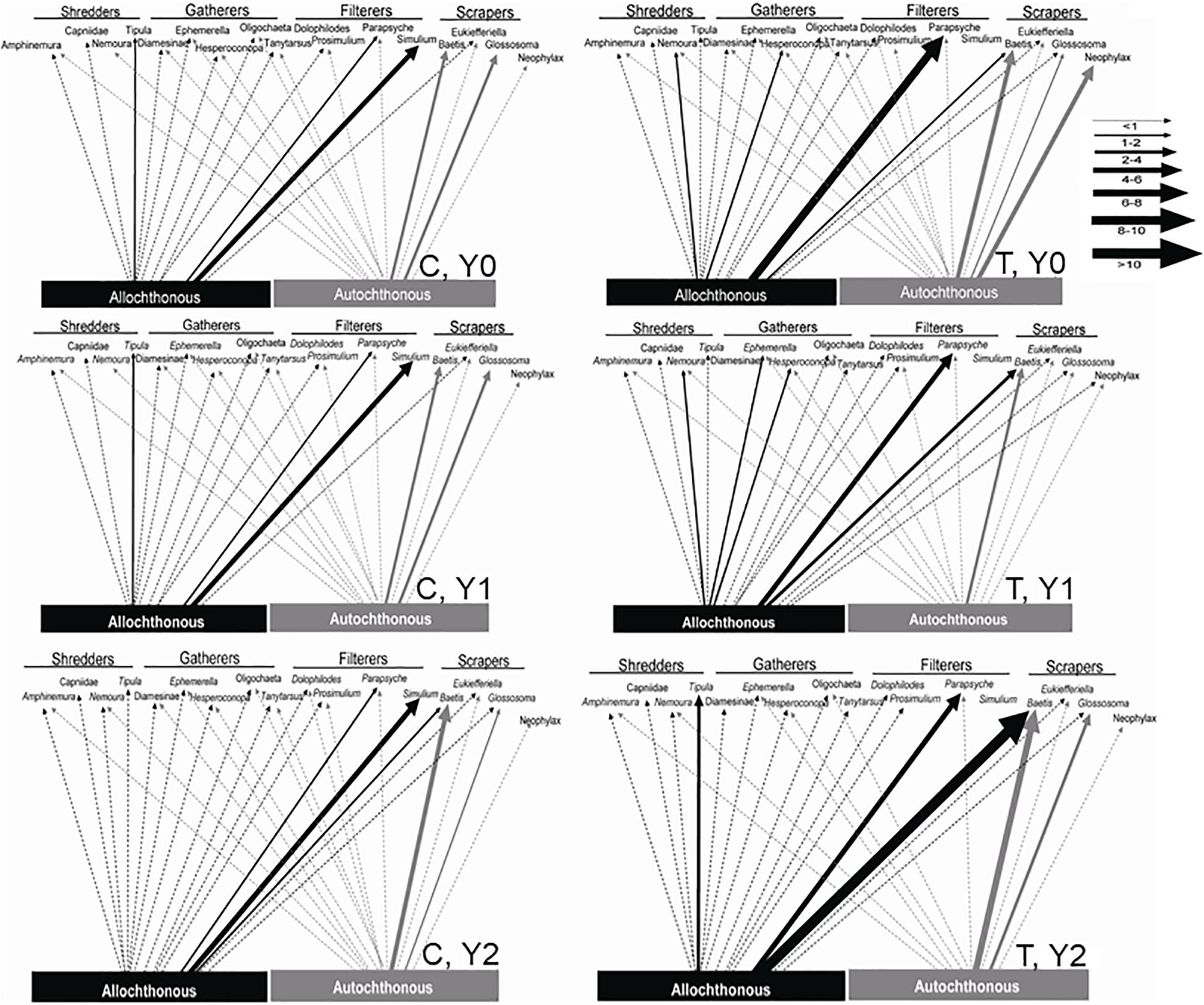
Figure 6. Allochthonous (dark lines) and autochthonous (light lines) C flow to macroinvertebrate taxa in an upstream control C and downstream wood-added reach (treatment, T) 1 year before (Y0) and 1 (Y1) and 2 years (Y2) after wood addition. Arrow widths represent the proportional importance of allochthonous and autochthonous C.
Discussion
Implications for Management and Restoration
Combining δ13C signatures to get allochthonous and autochthonous C assimilation with secondary production provided a unique investigation into the macroinvertebrate trophic response to wood addition (Figure 6). The combined approach of C assimilation and macroinvertebrate secondary production indicated changes in the consumption and flow of allochthonous and autochthonous C through the macroinvertebrate food web. Here we demonstrated that the abundance of organic matter and locally exposed cobble and gravel, which supports periphyton, could drive increased allochthonous and autochthonous C flow to several macroinvertebrate functional feeding groups most notably greater autochthonous C contribution to scrapers and greater allochthonous C contribution to filterers shredders (Figure 3). Taxon-specific seasonal and annual shifts in C assimilation were documented (Supplementary Appendix 1). Still, we observed changes in total resource consumption from the coupled increase in organic matter standing crop and substrate sorting at a local scale (i.e., around the added logs) following wood addition (Entrekin et al., 2008). Unfortunately, we did not replicate this study, which is a commonly encountered challenge for reach-scale quantitative food web approaches that measure energy flow (e.g., Cross et al., 2007). Despite the limits for generalizations, our results support methods for future evaluations of restoration (see also Vander Zanden et al., 2006) by stressing the need for trophic-based analysis to assess restoration success or failure in terms of resource availability and energy flow. The combined structural and functional approach here points to physical changes from the restoration that increased allochthonous and autochthonous C flow (Figure 5) and increased food web connections predicted from large wood additions in temperate streams (Figure 6).
Modest changes in C flow following wood additions were from a combination of greater assimilation of allochthonous and autochthonous C by a few macroinvertebrate taxa. Baetis mayflies, a multivoltine taxa, responded with greater secondary production that was fueled by increasing amounts of allochthonous C. Glossosoma caddisflies also showed an increase in production fueled by autochthonous C (Figure 6). Allochthonous and autochthonous resources were nearly equally important in this forested temperate stream. Typically, allochthonous resources are most important and organic matter standing crop predicts macroinvertebrate secondary production (Wallace et al., 1987, 2015); however, in the main channel of our stream, autochthony was as well documented. For example, 2 years after the wood additions, ∼40–50% of the macroinvertebrate community was supported by autochthonous C (Figure 3). In contrast to a manipulation of allochthonous C in an Appalachian stream (Wallace et al., 1997b), autochthonous C was a more important and substantial resource to the food web (Figures 4, 5). The difference in our study compared to studies in the Appalachian stream is the absence of a dense understory of rhododendron and a mature forest (Greenwood and Rosemond, 2005; Wallace et al., 2015). In the Appalachian streams, rhododendron and a more mature forest canopy limited light for primary production (Greenwood and Rosemond, 2005). Light was not limiting during seasons of open canopy in our study stream and this was likely the primary reason for greater autochthonous resource availability in this study stream compared to others conducted in headwater streams draining in-tact forests (Hoellein et al., 2007). Still, allochthonous C was the primary C source contributing to macroinvertebrate secondary production, particularly for shredders (∼50–80% contributed to secondary production), gatherers (∼60–90%), filterers (∼55–90%), and predators (∼60–80%) (Figure 3). The overall contribution of allochthonous C to secondary production ranged from 50 to 60% because scraper production was relatively high compared to the other functional groups (Figure 4).
Seasonal Effects of Added Wood on Macroinvertebrate δ13C
While annual average macroinvertebrate isotopic signatures changed only a little through space and time, taxon-specific signatures did change seasonally (Figure 1). Taxa with seasonally variable δ13C signatures may act as ‘indicators’, useful in evaluating ecosystem restorations aimed at changing or increasing basal resources [sensu 44]. In this study, Glossosoma, considered obligate consumers of algae, showed little variability in δ13C across months, years, and stream reaches; therefore, their production rather than their δ13C signatures may serve as a metric of restoration success (Table 1 and Supplementary Appendix 1). In contrast, facultative feeders such as the shredding stonefly Nemoura sp., and Baetis spp., showed spatially and temporally variable δ13C values, and so their average δ13C values at a population level may be more indicative of seasonal changes in basal food resource availability. Using the natural abundance of C stable isotopes for assessing resource acquisition complements assessments based on community-level diversity and tolerance values (e.g., Gratton and Denno, 2006).
Influence of Large Dead Wood on Macroinvertebrate C Flow
Given the forested nature of our study stream and the well-documented role of leaves and wood in governing macroinvertebrate secondary production, we hypothesized that most C in stream macroinvertebrates would bear an allochthonous signature (Wallace et al., 2015). Indeed, we predicted primary production would be limited by the deciduous canopy that was 75% closed in the winter and spring and 90% closed in the summer and autumn (Entrekin et al., 2008). Furthermore, a comparison of organic matter standing crop across studies and years show a strong positive relationship with macroinvertebrate secondary production suggesting reliance on allochthonous C as a primary food resource (e.g., Newbold et al., 1997; Webster et al., 1997; Hall et al., 2001; Chadwick and Huryn, 2007; Cross et al., 2007; Entrekin et al., 2007). However, these studies did not measure assimilation and algae can contribute more to production than often assumed from feeding mode analysis and correlations to resource availability (Finlay, 2001; Hall et al., 2001; Marcarelli et al., 2011). Therefore, we were surprised to find that the autochthonous C supported 47–59% of secondary macroinvertebrate production in both reaches (Figure 3).
The few studies that measured the contribution of primary production to macroinvertebrates in temperate headwater streams have based their conclusions on gut content analysis or tracer stable isotopes. For example, Mayer and Likens (1987) used gut contents to conclude that algae were more important than expected for caddisflies in forested headwater streams. More recently, allochthonous and autochthonous C resource contributions have been delineated for entire macroinvertebrate communities in a variety of biomes using stable isotopes with the consensus being algae were more important to the food web than expected (Guo et al., 2016; Brett et al., 2017; Neres-Lima et al., 2017). In fact, macroinvertebrates sampled from headwaters in the Salmon River, where the River Continuum Concept was developed, showed a surprising amount of diatoms in their guts (Rosi-Marshall et al., 2016). Still, macroinvertebrates in the Salmon River headwaters consumed more allochthonous C. In fact, macroinvertebrates also consumed about 80% allochthonous C in this study. These two perspectives are important to consider: consumption versus assimilation. Both processes are essential aspects of C cycling; however, each indicate different C fates. Carbon assimilation compared with available resources can be used to quantify trophic transfer efficiency, while assimilation subtracted from consumption quantifies egestion that is a measure of C recycling (i.e., carbon available in a different form to a range of organisms). Therefore, autochthonous C trophic transfer was relatively high compared to resource availability. Yet, allochthonous C consumption was relatively high and critical for C recycling. Lamberti and Steinman (1997) reviewed studies from 30 streams, mostly in the Northern Hemisphere, that indicated periphyton was an energetically important food resource in a range of stream sizes, biomes, and geographic areas. Furthermore, Finlay (2001) compiled macroinvertebrate δ13C values from 70 streams worldwide that indicated functional groups other than scrapers and shredders were assimilating significant amounts of epilithic algae, particularly in streams with catchments greater than 10 km2. Our results, confirming the importance of primary producers to macroinvertebrate production reflect that (1) algae are assimilated more efficiently than leaf litter because of higher nutritive value (e.g., lower C:N:P content) (Berg and Hellenthal, 1992), (2) algae have higher turnover rates that increase availability to macroinvertebrate consumers (Lamberti et al., 1989), and (3) algae are available year round even in streams with nearly closed deciduous canopy (Hamilton et al., 2004).
When we compared the amount of algal C available (calculated as gross primary production; GPP) to the total amount of algae consumed, we found that macroinvertebrates were consuming 4–36% of the total GPP [or about 8–72% net primary production (NPP)]. This autochthonous contribution of C to the macroinvertebrates is greater than we anticipated and greater than in tropical forested streams (Neres-Lima et al., 2017; Rosas et al., 2020). The contribution of autochthonous C was likely relatively high in this stream and perhaps others because: (1) filtering taxa were likely ingesting recycled autochthonous C in the form of epilithic scrapers and sloughed particles (e.g., Strayer, 1988; Benke and Wallace, 1997), (2) scrapers were likely increasing algal turnover rates through efficient grazing, while keeping biomass low (e.g., Lamberti et al., 1995), and (3), estimation errors associated with both whole-reach estimates of GPP and NPP (e.g., Hall and Tank, 2003) and using published estimates of C assimilation by macroinvertebrates using measurements of production could under estimate C consumption (e.g., Morin et al., 1987). Our results highlight assumptions that must be made and the difficulty in estimating the contribution of autochthonous and allochthonous resources in systems with consumers ingesting a complex mixture of both types simultaneously.
When we compare the amount of leaf litter consumed by macroinvertebrate community compared to the amount available, consumption values ranged from 13 to 40% of available. Organic matter evidently was not limiting macroinvertebrate population growth on an annual scale, although it might have been limited in some seasons, as has been shown in other studies (e.g., Richardson, 1991; Dobson and Hildrew, 1992; Marks, 2019). The addition of large wood increased the percentage of GPP and coarse benthic organic matter consumed by 25 and 10%, respectively, for the macroinvertebrate community in the treatment reach relative to the control reach. Other studies have found that changes in microhabitat, such as increased leaf litter accumulations, may change the invertebrate community structure and increase production (e.g., Wallace et al., 1997a; Kobayashi and Kagaya, 2004). In our study, increased patches of inorganic coarse substrate and deposited leaf litter and fine sediment associated with the added wood seemed to have increased production by the shorter-lived taxa, such as Baetis, resulting in increased community-level secondary production (Entrekin et al., 2009). Longer-term increases in organic matter retention are predicted to increase the relative importance of allochthonous organic matter to the food web and sustain greater production and trophic transfer in the future.
Data Availability Statement
All macroinvertebrate datasets generated for this study are included in the article/Supplementary Material.
Author Contributions
SE co-designed the experiments, analyzed the data, and was the lead manuscript writer. ER, JT, GL, and TH co-designed the experiments and provided critical input and edits to the manuscript.
Funding
The authors declare that this study received funding from provided by a grant from the USDA-NRI Managed Ecosystems Program (2003-35101-12871) to JT and GL. Additional funding to SE was provided by a Sigma Xi grant, a Bayer Fellowship, and a Warner-Lambert Fellowship from the University of Notre Dame. The funders were not involved in the study design, collection, analysis, interpretation of data, the writing of this article or the decision to submit it for publication.
Conflict of Interest
The funders were not involved in the study design, collection, analysis, interpretation of data, the writing of this article or the decision to submit it for publication.
The authors declare that the research was conducted in the absence of any commercial or financial relationships that could be construed as a potential conflict of interest.
Acknowledgments
We thank Aileen Kelly and Brian Holland for assistance with sample processing and Dennis Birdsell and Jon Loftus for analytical support at the Center for Environmental Science at University of Notre Dame. We also thank personnel of the Ottawa National Forest, United States Department of Agriculture Forest Service for support throughout the study. Comments by David Janetski, Scott Tiegs, Barbara Downes, Richard Johnson, CA, and two reviewers improved the manuscript substantially.
Supplementary Material
The Supplementary Material for this article can be found online at: https://www.frontiersin.org/articles/10.3389/fevo.2020.00114/full#supplementary-material
Footnotes
References
Benke, A., and Wallace, J. (2011). Secondary production, quantitative food webs, and trophic position. Nat. Educ. Knowledge 2:2. doi: 10.1002/ecy.2228
Benke, A. C., and Wallace, J. B. (1997). Trophic basis of production among riverine caddisflies: Implications for food web analysis. Ecology 78, 1132–1145.
Berg, M. B., and Hellenthal, R. A. (1992). Life histories and growth of lotic chironomids (Diptera: Chironomidae). Ecol. Populat. Biol. 85, 579–589.
Bicknell, A. W., Campbell, M., Knight, M. E., Bilton, D. T., Newton, J., and Votier, S. C. (2011). Effects of formalin preservation on stable carbon and nitrogen isotope signatures in calanoid copepods: implications for the sue of Continuous Plankton Recorder Survey samples in stable isotope analyses. Rapid Commun. Mass Spectr. 25, 1794–1800. doi: 10.1002/rcm.5049
Brett, M. T., Bunn, S. E., Chandra, S., Galloway, A., Guo, F., Kainz, M. J., et al. (2017). How important are terrestrial organic carbon inputs for secondary production in freshwater ecosystems? Freshw. Biol. 62, 833–853.
Chadwick, M. A., and Huryn, A. D. (2007). Role of habitat in determining macroinvertebrate production in an intermittent-stream system. Freshw. Biol. 52, 240–251.
Cole, J. J., Prairie, Y. T., Caraco, N. F., McDowell, W. H., Tranvik, L. J., Striegl, R. G., et al. (2007). Plumbing the global carbon cycle: integrating inland waters into the terrestrial carbon budget. Ecosystems 10, 172–185.
Cordova, J. M., Rosi-marshall, E. J., Yamamuro, A. M., and Lamberti, G. A. (2007). Quantity, controls and functions of large woody debris in Midwestern USA streams. River Res. Appl. 23, 21–33.
Cross, W. F., Wallace, J. B., and Rosemond, A. D. (2007). Nutrient enrichment reduces constraints on material flows in a detritus-based food web. Ecology 88, 2563–2575. doi: 10.1890/06-1348.1
Cummins, K. W., Wilzbach, M. A., Gates, D. M., Perry, J. B., and Taliaferro, W. B. (1989). Shredders and riparian vegetation - leaf litter that falls into streams influences communities of stream invertebrates. Bioscience 39, 24–30.
Dobson, M., and Hildrew, A. G. (1992). A test of resource limitation among shredding detritivores in low order streams in southern England. J. Anim. Ecol. 61, 69–77.
Entrekin, S. A., Rosi-marshall, E. J., Tank, J. L., Hoellein, T. J., and Lamberti, G. A. (2007). Macroinvertebrate secondary production in 3 forested streams of the upper Midwest, USA. J. North Am. Benthol. Soc. 26, 472–490.
Entrekin, S. A., Tank, J. L., Rosi-marshall, E. J., Hoellein, T. J., and Lamberti, G. A. (2008). Responses in organic matter accumulation and processing to an experimental wood addition in three headwater streams. Freshw. Biol. 53, 1642–1657.
Entrekin, S. A., Tank, J. L., Rosi-marshall, E. J., Hoellein, T. J., and Lamberti, G. A. (2009). Response of secondary production by macroinvertebrates to large wood addition in three Michigan streams. Freshw. Biol. 54, 1741–1758.
Finlay, J. C. (2001). Stable-carbon-isotope ratios of river biota: Implications for energy flow in lotic food webs. Ecology 82, 1052–1064.
Frainer, A., Polvi, L. E., Jansson, R., and McKie, B. G. (2017). Enhanced ecosystem functioning following stream restoration: The roles of habitat heterogeneity and invertebrate species traits. J. Appl. Ecol. 55, 377–385.
Fry, B. (2002). Stable isotopic indicators of habitat use by Mississippi River fish. J. North Am. Benthol. Soc. 21, 676–685.
Gratton, C., and Denno, R. F. (2006). Arthropod food web restoration following removal of an invasive wetland plant. Ecol. Appl. 16, 622–631. doi: 10.1890/1051-0761(2006)016[0622:afwrfr]2.0.co;2
Greenwood, J. L., and Rosemond, A. D. (2005). Periphyton response to long-term nutrient enrichment in a shaded headwater stream. Can. J. Fish. Aqua. Sci. 2005, 2033–2045.
Guo, F., Kainz, M. J., Sheldon, F., and Bunn, S. E. (2016). The importance of high-quality algal food sources in stream food webs–current status and future perspectives. Freshw. Biol. 61, 815–831.
Hall, R. O. Jr., Likens, G. E., and Malcom, H. M. (2001). Trophic basis of invertebrate production in 2 streams at the Hubbard Brook Experimental Forest. J. North Am. Benthol. Soc. 20, 432–447.
Hall, R. O., and Meyer, J. L. (1998). The trophic significance of bacteria in a detritus-based stream food web. Ecology 79, 1995–2012.
Hall, R. O., and Tank, J. L. (2003). Ecosystem metabolism controls nitrogen uptake in streams in Grand Teton National Park. Wyoming. Limnol. Oceanogr. 48, 1120–1128.
Hamilton, S. K., Sippel, S. J., and Bunn, S. E. (2005). Separation of algae from detritus for stable isotope or ecological stoichiometry studies using density fractionation in colloidal silica. Limnol. Oceanogr. Methods 3, 149–157.
Hamilton, S. K., Tank, J., Raikow, D., Siler, E. R., Dorn, N. J., and Leonard, N. E. (2004). The role of instream vs allochthonous N in stream food webs: modeling the results of an isotope addition experiment. J. North Am. Benthol. Soc. 23, 429–448.
Hoellein, T. J., Tank, J. L., Rosi-marshall, E. J., and Entrekin, S. A. (2009). Temporal variation in substratum-specific rates of N uptake and metabolism and their contribution at the stream-reach scale. J. North Am. Benthol. Soc. 28, 305–318.
Hoellein, T. J., Tank, J. L., Rosi-Marshall, E. J., Entrekin, S. A., and Lamberti, G. A. (2007). Controls on spatial and temporal variation of nutrient uptake in three Michigan headwater streams. Limnol. Oceanogr. 52, 1964–1977.
Kail, J. (2002). Influence of large woody debris on the morphology of six central European streams. Geomorphology 1299, 1–17.
Kennedy, T. A., Finlay, J. C., and Hobbie, S. C. (2005). Eradication of invasive Tamarix ramosissima along a desert stream increases native fish density. Ecol. Appl. 15, 2072–2083.
Kobayashi, S., and Kagaya, T. (2004). Litter patch types determine macroinvertebrate assemblages in pools of a Japanese headwater stream. J. North Am. Benthol. Soc. 23, 78–89.
Lamberti, G. A., Gregory, S. V., Ashkenas, L. R., Li, J. L., Steinman, A. D., and David McIntire, C. (1995). Influence of grazer type and abundance on plant-herbivore interactions in streams. Hydrobiologia 306, 179–188.
Lamberti, G. A., and Steinman, A. D. (1997). A comparison of primary production in stream ecosystems. J. North Am. Benthol. Soc. 16, 95–104.
Lamberti, G. L., Gregory, S. V., Ashkenas, L. R., Steinman, A. D., and David McIntire, C. (1989). Productive capacity of periphyton as a determinant of plant-herbivore interactions in streams. Ecology 1989, 1840–1856.
Lepori, F., Gaul, D., Palm, D., and Malmqvist, B. (2006). Food-web responses to restoration of channel heterogeneity in boreal streams. Can. J. Fish. Aqua. Sci. 63, 2478–2486.
Lepori, F., Palm, D., and Malmqvist, B. (2005). Effects of stream restoration on ecosystem functioning: detritus retentiveness and decomposition. J. Appl. Ecol. 42, 228–238.
Marcarelli, A. M., Baxter, C. V., Mineau, M. M., and Hall, R. O. Jr. (2011). Quantity and quality: unifying food web and ecosystem perspectives on the role of resource subsidies in freshwaters. Ecology 92, 1215–1225. doi: 10.1890/10-2240.1
Marks, J. C. (2019). Revisiting the fates of dead leaves that fall into streams. Ann. Rev. Ecol. Evol. Syst. 50, 547–568.
Mayer, M., and Likens, G. E. (1987). The importance of algae in a shaded headwater stream as food for an abundant caddisfly (Trichoptera). J. North Am. Benthol. Soc. 6, 262–269.
McCutchan, J. H. Jr., Lewis, W. M. Jr., Kendall, C., and McGrath, C. C. (2003). Variation in trophic shift for stable isotope ratios of carbon, nitrogen, and sulfur. Oikos 102, 378–390. doi: 10.1002/rcm.8589
McNeely, C., Finlay, J. C., and Power, M. E. (2007). Grazer traits, competition, and carbon sources to a headwater-stream food web. Ecology 88, 391–401. doi: 10.1890/0012-9658(2007)88[391:gtcacs]2.0.co;2
Minshall, G. W., and Rugenski, A. (2006). “Riparian processes and interactions,” in Methods in Stream Ecology, eds F. R. Hauer and G. A. Lamberti (Cambridge, MA: Academic Press), 721–742.
Moore, J. C., Berlow, E. L., Coleman, D. C., de Ruiter, P. C., Dong, Q., Hastings, A., et al. (2004). Detritus, trophic dynamics and biodiversity. Ecol. Lett. 7, 584–600.
Morin, A., Mousseau, T. A., and Roff, D. A. (1987). Accuracy and precision of secondary production estimates. Limnol. Oceanogr. 32, 1342–1352.
Negishi, J. N., and Richardson, J. S. (2003). Responses of organic matter and macroinvertebrates to placements of boulder clusters in a small stream of southwestern British Columbia. Canada. Can. J. Fish. Aqua. Sci. 60, 247–258.
Neres-Lima, V., Machado-Silva, F., Baptista, D. F., Oliveira, R., Andrade, P. M., Oliveira, A. F., et al. (2017). Allochthonous and autochthonous carbon flows in food webs of tropical forest streams. Freshw. Biol. 62, 1012–1023.
Newbold, J. D., Bott, T. L., Kaplan, L. A., Sweeney, B. W., and Vannote, R. L. (1997). Organic matter dynamics in White Clay Creek, Pennsylvania, USA. J. North Am. Benthol. Soc. 16, 46–50.
Phillips, D. L., and Gregg, J. W. (2001). Uncertainty in source partitioning using stable isotopes. Oecologia 128, 304–304. doi: 10.1007/s004420100723
Polis, G. A., and Strong, D. R. (1996). Food web complexity and community dynamics. Am. Nat. 147, 813–846. doi: 10.1007/s00442-006-0505-1
Power, M. E., Stout, R. J., Cushing, C. E., Harper, P. P., Hauer, F. R., Matthews, W. J., et al. (1988). Biotic and abiotic controls in river and stream communities. J. North Am. Benthol. Soc. 7, 456–479. doi: 10.1002/ecy.1671
Richardson, J. S. (1991). Seasonal food limitation of detritivores in a montane stream: an experimental test. Ecology 72, 873–887.
Rosas, K. G., Colón-Gaud, C., and Ramírez, A. (2020). Trophic basis of production in tropical headwater streams, Puerto Rico: an assessment of the importance of allochthonous resources in fueling food webs. Hydrobiologia 847, 1–15.
Rosemond, A. D., Mulholland, P. J., and Elwood, J. W. (1993). Top-down and bottom-up control of stream periphyton - effects of nutrients and herbivores. Ecology 74, 1264–1280.
Rosi-Marshall, E. J., Vallis, K. L., Baxter, C. V., and Davis, J. M. (2016). Retesting a prediction of the River Continuum Concept: autochthonous versus allochthonous resources in the diets of invertebrates. Freshw. Sci 35, 534–543.
Rosi-Marshall, E. J., and Wallace, J. B. (2002). Invertebrate food webs along a stream resource gradient. Freshw. Biol. 47, 129–141.
Sarakinos, H. C., Johnson, M. L., and Vander Zanden, M. J. (2002). A synthesis of tissue-preservation effects on carbon and nitrogen stable isotope signatures. Can. J. Zool. 80, 381–387.
Schlacher, T. A., and Connolly, R. M. (2014). Effects of acid treatment on carbon and nitrogen stable isotope ratios in ecological samples: a review and synthesis. Methods Ecol. Evol. 5, 541–550.
Stewart-Oaten, A., Murdoch, W. W., and Parker, K. R. (1986). Environmental impact assessment: “pseudoreplication” in time? Ecology 67, 929–940.
Sudduth, E. B., Hassett, B. A., Cada, P., and Bernhardt, E. S. (2011). Testing the Field of Dreams Hypothesis: functional responses to urbanization and restoration in stream ecosystems. Ecol. Appl. 21, 1972–1988. doi: 10.1890/10-0653.1
Tiegs, S. D., Peter, F. D., Robinson, C. T., Uehlinger, U., and Gessner, M. O. (2008). Leaf decomposition and invertebrate colonization responses to manipulated litter quantity in streams. J. North Am. Benthol. Soc. 27, 321–331.
Underwood, A. J. (1992). Beyond BACI: the detection of environmental impacts on populations in the real, but variable, world. J. Exp. Mar. Biol. Ecol. 161, 145–178.
Vander Zanden, J., Olden, J. D., Gratton, C., and Tunney, T. D. (2006). “Food web approaches in restoration ecology,” in Foundations of Restoration Ecology, eds D. A. Falk, et al. (Washington, DC: Island Press), 165–189.
Wallace, J. B., Benke, A. C., Lingle, A. H., and Parsons, K. (1987). Trophic pathways of macroinvertebrate primary consumers in subtropical blackwater streams. Arch. Hdrobiol. 74, 423–451.
Wallace, J. B., Cuffney, T. F., Eggert, S. L., and Whiles, M. R. (1997a). Stream organic matter inputs, storage, and export for Satellite Branch at Coweeta Hydrologic Laboratory, North Carolina, USA. J. North Am. Benthol. Soc. 16, 67–74.
Wallace, J. B., Eggert, S. L., Meyer, J. L., and Webster, J. R. (1997b). Multiple trophic levels of a forest stream linked to terrestrial litter inputs. Science 277, 102–104.
Wallace, J. B., Eggert, S. L., Meyer, J. L., and Webster, J. R. (2015). Stream invertebrate productivity linked to forest subsidies: 37 stream-years of reference and experimental data. Ecology 96, 1213–1228. doi: 10.1890/14-1589.1
Webster, C. R., Huckins, C. J. F., and Shields, J. M. (2008). Spatial distribution of riparian zone coarse woody debris in a managed northern temperate watershed. Am. Midland Nat. 159, 225–237.
Keywords: headwaters, large woody debris, restoration, stable isotopes, organic matter
Citation: Entrekin SA, Rosi EJ, Tank JL, Hoellein TJ and Lamberti GA (2020) Quantitative Food Webs Indicate Modest Increases in the Transfer of Allochthonous and Autochthonous C to Macroinvertebrates Following a Large Wood Addition to a Temperate Headwater Stream. Front. Ecol. Evol. 8:114. doi: 10.3389/fevo.2020.00114
Received: 05 September 2019; Accepted: 08 April 2020;
Published: 06 May 2020.
Edited by:
Carla Atkinson, The University of Alabama, United StatesReviewed by:
Arthur Benke, The University of Alabama, United StatesCaleb Robbins, The University of Kansas, United States
Copyright © 2020 Entrekin, Rosi, Tank, Hoellein and Lamberti. This is an open-access article distributed under the terms of the Creative Commons Attribution License (CC BY). The use, distribution or reproduction in other forums is permitted, provided the original author(s) and the copyright owner(s) are credited and that the original publication in this journal is cited, in accordance with accepted academic practice. No use, distribution or reproduction is permitted which does not comply with these terms.
*Correspondence: Sally A. Entrekin, c2FsbHllQHZ0LmVkdQ==; c2VudHJla2lAZ21haWwuY29t