- 1Department of Evolution, Ecology and Organismal Biology, The Ohio State University, Columbus, OH, United States
- 2Department of Entomology, National Museum of Natural History, Washington, DC, United States
- 3Department of Biology, University of Louisville, Louisville, KY, United States
- 4Smithsonian Tropical Research Institute, Balboa, Panama
- 5Red Imported Fire Ant Research Centre, South China Agricultural University, Guangzhou, China
Chemical communication is a fundamental, highly complex component of social insect societies. Ants in particular employ a remarkable diversity of chemical signals to maintain social cohesion among nestmates, gain essential resources through coordinated foraging, and warn of danger. Although the chemicals used can be functionally specific, they are vulnerable to exploitation by eavesdropping natural enemies (e.g., parasitoids, predators, parasites) and other associates (e.g., myrmecophiles). Ant nests are nutrient hotspots due to their collection of resources warranting keen defense systems; yet the heavily defended hideouts are frequently invaded. Many organisms exploit ant species, but how they locate hosts—including what host-derived cues are used—is still poorly understood. Here, we review current knowledge about how ant chemical communication systems can be exploited by unintended receivers. We take a case study approach and illustrate the diversity of ant associates and host traits that may predispose ants to exploitation. We identify knowledge gaps by reviewing host systems and listing: (1) the types of associates (e.g., fly, wasp, beetle) where eavesdropping is likely occurring, organized by the host communication system that is being exploited; (2) the ant parasites that exploit trail pheromones; and (3) the experimentally determined chemicals (i.e., alarm/defensive pheromones), used by eavesdroppers. At least 25 families of arthropods (10 orders) potentially eavesdrop on ant communication systems and nearly 20 host ant species are vulnerable to trail parasite ant species. We also propose future research that will improve our understanding of community assembly by examining host traits (e.g., latitude, nest characteristics, trail system) that influence their susceptibility to eavesdropping associates.
Exploitation of Chemical Communication
In nature, natural enemies impose strong selective pressures on animals. These well-documented interactions drive complex coevolutionary arms races wherein organisms avoid detection by natural enemies that in turn evolve to overcome their victim’s defenses. As individuals communicate with conspecifics, they also make information available to natural enemies and other associates that can be used to their advantage. Indeed, signals are often intercepted by unintended receivers who use them to exploit the signaler and ultimately benefit from accessing this communication system (Zuk and Kolluru, 1998; Stevens, 2013). This phenomenon, known as eavesdropping, can occur across sensory modalities in vertebrate and invertebrate communication networks (Otte, 1974; Stowe et al., 1995; Peake et al., 2001; Hamel and Cocroft, 2019). Chemical communication systems in particular, make signalers vulnerable to exploitation by a wide variety of enemies (e.g., parasitoids, predators, cleptoparasites, social parasites) and other associates (e.g., myrmecophiles). The diffusion of chemical signals, albeit less reliable than light and sound signals, can reveal the location of the signaler due to the odor gradients created. Chemical signals can also be made of molecules with low volatility that remain in the environment for days. Most studies investigating eavesdropping by natural enemies, however, have focused on the exploitation of acoustic and visual signals. Here, we evaluate the evidence for eavesdropping on chemical signals with the goal of providing hypotheses for future research that will fill the key gaps in our understanding of this phenomenon. We focused this review on ants (Hymenoptera: Formicidae) given that chemical communication is especially well developed in this insect family, providing an opportunity to cultivate general principles that relate to chemical communication and exploitation of chemical signals more broadly.
Ants are ecologically successful for a variety of reasons, including their social behavior, division of labor among distinct castes, and potentially large colony sizes. Ants communicate with their nestmates and with other organisms using a variety of mechanical (tactile, vibrations), visual, and chemical cues (Hölldobler and Wilson, 1990). However, it is clear that the evolutionary success of ants can be attributed in large part to their efficient chemical communication systems that enables large ant colonies to solve complex problems (Gordon and Mehdiabadi, 1999; Dornhaus et al., 2012). While the average ant worker has seven different glands, 75 different glands have so far been described in the Formicidae (Jackson and Morgan, 1993; Billen, 2009) and many of these secretions provide the basis for chemical communication systems. For example, exocrine secretions are used in defense and communication signals are commonly excreted from multiple glands used in synergy, therefore, the identification of the exact compound that elicits a behavioral response is challenging. Regardless, the literature on ant communication generally centers on detection of volatile emissions, thus we focus on chemical communication as the basis for this review. To our knowledge, all reports of eavesdropping in ants have involved chemical compounds, but tactile and vibrational cues may also be important under certain conditions. We mainly limit this review to sedentary nest-dwelling species because they form a tractable and ecologically relevant subset of the >16,000 known ant species (AntWeb1 [Accessed 9 September 2019.]). However, we also highlight traits shared among many ant species that are linked with trail pheromone use, defensive substances, and nestmate recognition. We show that, although pheromones are often directed toward nestmates (intended receivers), they can sometimes be detected and used as cues by a diverse array of heterospecifics or non-nestmate conspecifics (unintended receivers).
Trail Pheromones
Ants conspicuously depend on chemical trails when foraging, and these odor-guided recruitment messages are often complex by necessity (reviewed in Morgan, 2009; Czaczkes et al., 2015). A food-bearing ant returning to the nest typically follows a series of chemical signposts including the trail pheromone, home-range markings, nest-marking pheromone, and environmentally derived visual and olfactory landmarks (Hölldobler and Wilson, 1990; Steck, 2012). Multiple species may use the same chemicals as the basis for their trail pheromones (e.g., Z,E-α-farnesene, 2,5-Dimethyl-3-ethylpyrazine), but these are commonly augmented with colony-specific hydrocarbons (reviewed in Morgan, 2009; also see Blomquist and Bagnères, 2010). Moreover, blends of volatile chemicals within trail pheromones can influence different nestmate recruitment functions (e.g., attraction, repulsion, guidance) (Robinson et al., 2005). Some chemical trails are localized and persist for several days (Jackson et al., 2007), providing long-lasting cues that are detected by resource-seeking ant associates (Dejean and Beugnon, 1996). These eavesdroppers subsequently access ant nests or food sources (Table 1; e.g., cockroaches, caterpillars, ants) (Moser, 1964; Adams, 1990; Dejean and Beugnon, 1996; Menzel et al., 2010). Indeed, there are many examples of eavesdropping on trail pheromones (Table 2), but the specific chemicals underlying these associations remain unknown (see Table 3).
Defense Pheromones
Ants release a wide range of volatile chemicals to alert nestmates of impending threats (reviewed in Parry and Morgan, 1979; Attygalle and Morgan, 1984; Jackson and Morgan, 1993; Morgan, 2008). Behavioral responses to these volatiles include increased movement, gaped mandibles, sting extrusion, trail laying, and aggressive postures (Parry and Morgan, 1979). Alarm pheromones are most often produced by the mandibular, poison, or Dufour’s glands (Ali and Morgan, 1990), and are frequently used synergistically. Chemicals found in alarm pheromones include straight-chain and cyclic ketones, nitrogenated compounds, and formic acid (Maschwitz et al., 2008; Morgan, 2008; Vander Meer et al., 2010). The volatility of alarm pheromones makes them reliable long-range indicators of host presence, but also makes them spatiotemporally limited. Regardless, many parasitoids and predators of ants eavesdrop on alarm pheromones (Table 3) (Feener et al., 1996; Morrison and King, 2004; Witte et al., 2010).
Recognition Pheromones
Nestmate recognition in ants is primarily mediated via “signature mixtures” of branched and unsaturated cuticular hydrocarbons (CHCs) (Wyatt, 2010; Martin and Drijfhout, 2009; Leonhardt et al., 2016; Menzel et al., 2017). These pheromones are distinct from linear compounds with higher melting points, which function mainly to prevent insect desiccation (Howard and Blomquist, 2005; Martin and Drijfhout, 2009; Chung and Carroll, 2015). CHC signals are central to the social organization of an ant colony, thus they provide a weakness in colony defense due to the chemical mimicry or camouflage strategies used by nest associates. For example, many social parasites and myrmecophiles invade the ant nest as a “wolf in sheep’s clothing” using a CHC-based chemical disguise (Vander Meer and Wojcik, 1982; Lenoir et al., 2001; Akino, 2008; Blomquist and Bagnères, 2010). In such cases, CHC mimicry is not eavesdropping, but an infiltration strategy.
However, CHCs may be used by parasitoids to discriminate among the specific castes and life stages of their ant hosts (Table 1) when these chemical signatures function as signals. We define signals as sender-produced actions or structures that alter receiver behavior, and are the product of coevolutionary processes between actors (Maynard-Smith and Harper, 2003). Direct observation indicates ants can readily locate and manipulate their brood in different settings, implying that the same pheromones (and other cues) may be exploitable by brood-specific natural enemies. However, the unambiguous identification of ant brood-specific recognition signals has remained controversial (Morel and Vander Meer, 1988; Casacci et al., 2013). Evidence indicates at least some post-embryonic developmental ant stages emit some form of chemical (Walsh and Tschinkel, 1974; Brian, 1975) and even sound (Casacci et al., 2013). It is therefore possible that these associates eavesdrop upon nestmate recognition pheromones, but may also detect host-derived cues that range across sensory modalities. Determining eavesdropping on CHC signals is technically challenging because decoupling sensory modalities may be difficult, but still worthy of future studies. Host-derived short-range cues (e.g., tactile, chemical and auditory) as well as CHC-based signals are likely part of a complex of features enabling successful host exploitation.
How Enemies and Other Associates Find Hosts
The success of specialized ant associates is dependent on their ability to find hosts (or prey). The first challenge the exploiters face is locating their victim (Encounter Phase, Figure 1) (Combes, 2005). Although small ant colonies can quickly relocate in response to predation or disturbance (O’Shea-Wheller et al., 2015), species that invest substantial energy in building elaborate nest structures are often stationary targets (Hughes et al., 2008). In either case, associates locate hosts by using visual and olfactory cues that enable them to orient toward habitats occupied by their hosts (Morehead and Feener, 2000b; Lachaud and Pérez-Lachaud, 2012) (Figure 1A). Next, they detect host-derived chemical cues. These may be waste byproducts or long-range chemical signals such as trail, nest-marking and defense pheromones (Figure 1B). Once in close proximity, host acceptance prompts the Exploitation Phase. Species identification can be accomplished by detecting species-specific short-range chemical cues; at this stage, eavesdropping is possible if enemies or other associates are intercepting signals (e.g., sex and contact pheromones) (Figure 1C) (Bagnères and Wicker-Thomas, 2010). Predatory enemies would consume their prey at this stage. As the Exploitation Phase continues, social parasites (i.e., social species that exploit other social species), parasitoids, and myrmecophiles (i.e., associated organisms that live part or most of its life inside the host ant nest with ants, parasitoids excluded herein), appraise whether an individual or a colony has been previously attacked by conspecifics by detecting oviposition-marking pheromones, to avoid superparasitism (Figure 1D). Once the ant enemy or myrmecophile associate invades the organism or ant colony, it may regulate its host physiologically or behaviorally to maximize its own fitness returns (Figure 1E) (Vinson, 1976; Henne and Johnson, 2007; Mathis and Philpott, 2012; de Bekker et al., 2018). While we will focus on how olfaction influences host-finding behavior, it is important to keep in mind that multiple sensory modalities are likely at play and will affect ant associate behavior. Determining the role of different sensory modalities and their interaction (e.g., independent, additive, multiplicative) is necessary to test the chemical eavesdropping hypothesis. Furthermore, life experience and learning will also influence the success of host-finding (Vet and Dicke, 1992).
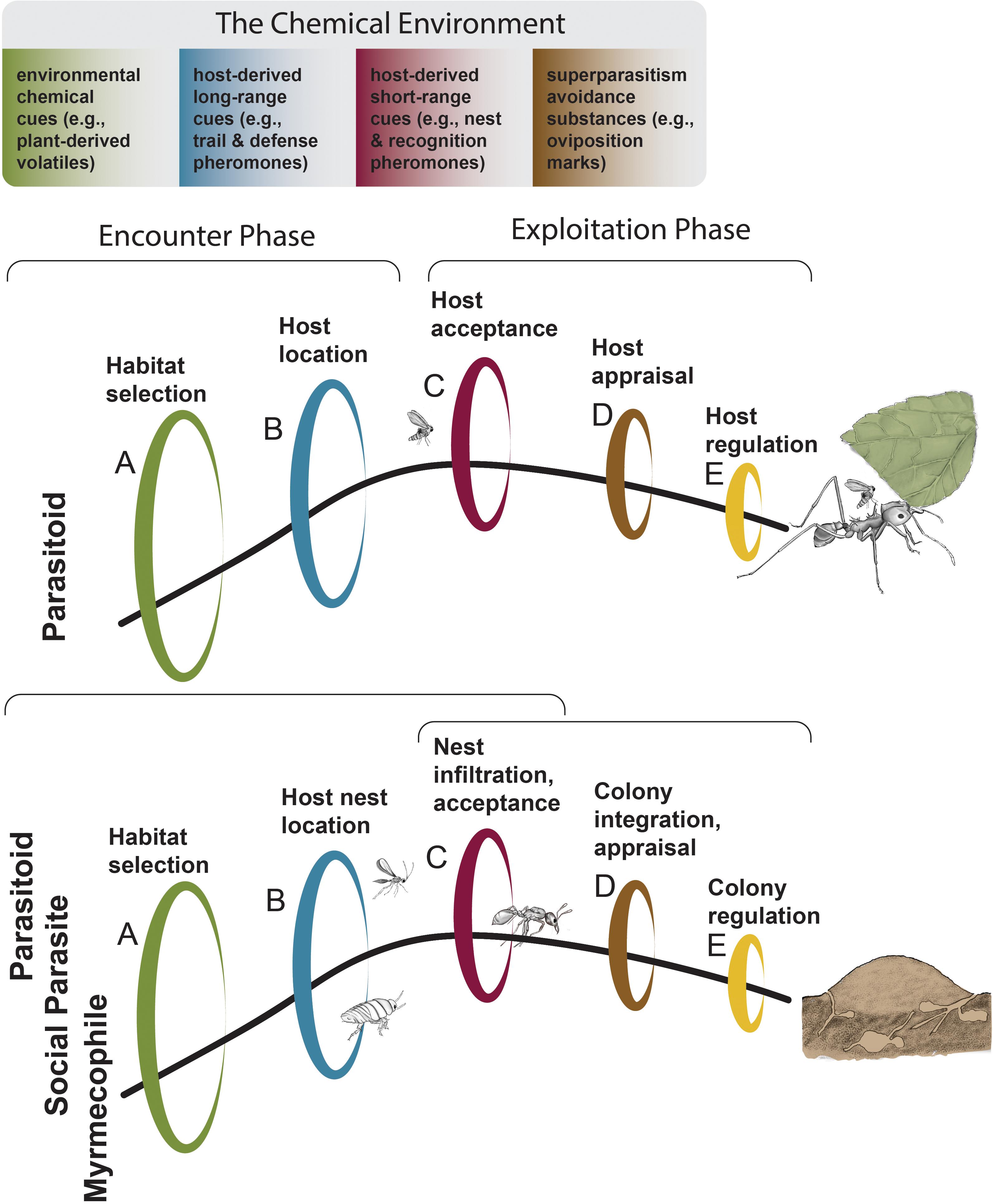
Figure 1. A graphic model of the sequential challenges associates face to successfully exploit an ant host. Different chemical cues can aid the associate in finding and appraising their host. When an enemy is intercepting a signal (e.g., pheromone), this is considered eavesdropping. (A) Habitat selection. Environmental chemical cues can aid the associate in narrowing search patterns. (B) Host location. Host-derived long-range chemical cues can guide the ant associate to a host individual (top panel) or nest (bottom panel) after arriving to the host habitat. (C) Host acceptance. Once the host individual (top panel) or nest (bottom panel) is located, the associate will determine if it is acceptable (e.g., correct species, life stage, caste, etc.). In some cases the associate will infiltrate the nest at this stage (bottom panel). Predators will consume their prey at this stage. (D) Host appraisal. In order to avoid superparasitism, some associate species are able to detect the chemical signature or oviposition-marking of hetero- or conspecifics, thus avoiding the fitness cost of sharing a host. In some cases this can occur at the same time as colony integration within the nest (bottom panel). (E) Host regulation. After infecting the host, the parasite can manipulate host behavior or physiology in order to maximize its fitness gains. Sequential challenges were inspired by Vinson (1976) and Mathis and Philpott (2012) whereas the host encounter phase and exploitation phases were inspired by Combes (2005). Illustrations by Rozlyn E. Haley adapted from photographs by Alex Wild.
As we have explained above, many ant associates—specifically social parasites, myrmecophiles, and some parasitoids—infiltrate and integrate into the nest by exploiting nestmate recognition signaling systems (Lenoir et al., 2001; Akino, 2008). While eavesdropping involves enemies and other associates detecting host-derived signals during host location (Figure 1B) and host acceptance (Figure 1C), successful colony infiltration occurs during the host acceptance stage (Figure 1C, bottom panel). Detection of host pheromones (i.e., eavesdropping) is distinct from traits that have evolved in response to host resistance (e.g., alteration of CHCs resulting in chemical mimicry). Colony integration, often achieved by the same mechanisms as “infiltration,” refers to associates that live for longer periods of time with their host (Figure 1D, bottom panel). In this case, they can maintain a chemical disguise and use weaponry to regulate host behavior (Figure 1E) while avoiding expulsion from the nest. Although the cuticular hydrocarbon profiles of many ant hosts and their associates have been evaluated, we still do not know how most associates locate their host (Figures 1A–C). The examples we present will address chemical eavesdropping during host location and acceptance (Figures 1B,C), when essential host-derived pheromones (e.g., defense, trail, nestmate recognition) are detected by the eavesdropping associates.
Enemies and Associates of the Ants
Numerous specialized ant associate species exploit individual ants (e.g., parasitoids and predators) or take advantage of shared colony resources (e.g., cleptoparasites, social parasites and myrmecophiles) (Table 1). The best-studied enemies are the parasitoid scuttle flies (Brown and Feener, 1991; Brown, 2001; Disney et al., 2006, 2008; Patrock et al., 2009; Morrison, 2012; Pérez-Lachaud et al., 2017) and wasps (Lachaud and Pérez-Lachaud, 2012; Murray et al., 2013) that attack exposed foragers. Other associates consume ant larvae (Masner, 1959; Wing, 1983; Loiácono et al., 2000), attack the queen (Johnson et al., 2002; Barbero et al., 2009; Briano et al., 2012), or are benign myrmecophiles scavenging on waste or taking advantage of the protected shelter (Hölldobler and Wilson, 1990). Below we review a diversity of cases where natural history evidence suggests eavesdropping on host-derived communication systems (i.e., trail, alarm and recognition) to locate the host ant, the host nest, or the host brood. We limit the scope to organisms or lineages that are good candidates for hypothesis-driven research testing for chemical eavesdropping on ants.
Parasitoids of Workers
Scuttle Flies (Diptera: Phoridae)
Host-derived ant pheromones are attractants to the so called “ant-decapitating flies” or scuttle flies (Diptera: Phoridae) (Table 1 and Boxes 1A, 2A). These parasitoids develop in specific body regions of their host ants, with one or more flies emerging per ant. The phorid flies that attack fire ants and leaf-cutter ants (Box 1A) have been studied more intensively because their hosts are considered pests (Hölldobler and Wilson, 1990), but many other ant lineages are vulnerable to these parasitoids (Hsieh and Perfecto, 2012). Species in >20 genera of phorids (especially Pseudacteon, Apocephalus, Eibesfeldtphora, Myrmosicarius, Neodohrniphora) (reviewed in Folgarait, 2013), collectively attack more than 22 genera of ants in five subfamilies (Mathis and Philpott, 2012). The flies potentially affect colony-level fitness via worker mortality and behavioral changes (e.g., reduced foraging) (Feener and Brown, 1992; Milton and Athayde, 2000; Elizalde and Folgarait, 2011; Hsieh and Perfecto, 2012).
BOX 1.
Leaf-cutting ants and generalized natural history of the associates. Two leaf-cutting genera, Atta and Acromyrmex use sophisticated chemical communication systems to maintain efficiency, order, and protection for their conspicuous nests and trail systems (ca. 90 m long in Atta; Hölldobler and Wilson, 2011). Nest structures with millions of workers are labeled with colony-specific territorial pheromones [e.g., n-heptadecane, (Z)-9- nonadecene, 8,11-nonadecadiene] and trail pheromones (e.g., methyl 4-methylpyrrole-2-carboxylate, 3-ethyl-2,5-dimethylpyrazine) with Dufour’s gland secretions (Tumlinson et al., 1972; Evershed and Morgan, 1981; Salzemann et al., 1992). Colonies are protected by specialized workers that emit volatilized alarm pheromones (e.g., 4-Methyl-3-heptanol, 3-octanone, 2-heptanone, 4-Methyl-3-heptanone, citral) (Blum et al., 1968; Hölldobler and Wilson, 1990; Norman et al., 2017). (A) A phorid fly hovers above Atta workers, attracted to trail pheromones. The parasitoid larva will develop and decapitate the host ant just before pupation. (B) Diapriid wasp searching for the host nest (e.g., Acromyrmex). (C) The gravid female searches for brood chamber then lays an egg(s) into a host larva. The parasitoid consumes the host and emerges inside the ant nest. (D) Attaphila roach following host ant trail (dotted lines). Illustrations by Rozlyn E. Haley adapted from photographs by Alex Wild.
BOX 2.
Pendulous carton forming Azteca ants and generalized natural history of the associates. While territorial markings have yet to be discovered, these aggressive arboreal ants maintain foraging territories with trail pheromones from their Pavan’s gland and alarm pheromones from the pygidal glands (Adams, 1994). Straight-chain and cyclic ketones (e.g., 2-heptanone and 2-methylcyclopentanone, respectively) act as alarm pheromones attracting nearby nestmates (Wheeler et al., 1975; McCann et al., 2013) while volatile aldehydes and iridoids (e.g., nepetalactol, iridoidal isomers) may simultaneously signal nest location (Adams, 1994; Nascimento et al., 1998). Less volatile chemicals such as cuticular hydrocarbons provide short-range information that allows parasitoids to discriminate between species (Mathis and Tsutsui, 2016). (A) A phorid fly hovers outside Azteca nest, attracted by alarm pheromones. After locating a suitable host ant, the phorid lays an egg inside her. The parasitoid larva will develop and decapitate the host just before pupation. (B) Cephalotes cleptoparasite workers follow the trail pheromones (dotted line) of Azteca scouts to locate new food sources. Illustrations by Rozlyn E. Haley adapted from photographs by Alex Wild.
Phorids can often be observed hovering above disturbed ant nests (Witte et al., 2010), injured workers (Brown and Feener, 1991) and foraging trails (Tonhasca, 1996). Trail and alarm pheromones are reliable cues that likely “advertise” host presence, whereas specific ant targets are selected based on short-range chemical and visual cues (Farder-Gomes et al., 2017). In some cases, CHC profiles confirm species identification for final host acceptance by the attacking flies (Mathis and Tsutsui, 2016) (Figure 1C, top panel). When searching for an appropriate oviposition point, the flies may detect oviposition -marking pheromones (i.e., used to inform conspecifics of a previously utilized host) or other signs from previous parasitoids (Figure 1D, top panel). Finally, the developing phorid larva may manipulate the behavior of the host ant until development of the parasitoid is complete (often culminating in decapitation of the host; Figure 1E, top panel) (reviewed in Henne and Johnson, 2007; de Bekker et al., 2018). Stages leading to successful exploitation have been studied in various systems where colony size is large and ant nests are permanent (see case studies of Atta Box 1 and Azteca Box 2) but also in species where workers forage individually (e.g., Paraponera) or in large groups (e.g., army ants). Due to their high diversity, the phorid flies are an informative research model for understanding host specificity, and the ecology and evolution of eavesdropping of ant chemical signals.
More than 80 phorid fly species parasitize leaf-cutter ants (i.e., Atta and Acromyrmex, collectively 51 species) (Box 1A) (reviewed in Folgarait, 2013). Phorids frequently attack Atta on foraging trails or at refuse piles (Milton and Athayde, 2000; Elizalde and Folgarait, 2012; Folgarait, 2013), and the threat of phorids presumably causes some leaf-cutter species to forage crepuscularly or at night (Orr, 1992). Hovering phorid flies cause reduced foraging activity, and defensive postures in workers that include hitchhiking minima workers riding on leaf fragments (Milton and Athayde, 2000; Elizalde and Folgarait, 2012; Folgarait, 2013). Given that phorid flies target Atta on foraging trails (Box 1A), it is likely that these parasitoids eavesdrop on trail pheromones (Milton and Athayde, 2000; Elizalde and Folgarait, 2012; Folgarait, 2013). The compound 2,5-dimethyl-3-ethylpyrazine is found in several leaf-cutter trail pheromones (Cross et al., 1982; Morgan et al., 2006), and it is also an alarm pheromone of Solenopsis fire ants (Vander Meer et al., 2010) and an attractant for phorid flies (Sharma et al., 2011; Ngumbi and Fadamiro, 2015). Experiments are needed to determine if this compound attracts phorids to Atta trails.
To date, only the phorid genus Pseudacteon is known to be associated with Azteca spp. (Feener and Brown, 1997; Mathis et al., 2011). Azteca workers noticeably attempt to dodge phorid fly parasitoids when the nest is damaged or when the ants are otherwise alarmed. The phorids are attracted to alarm pheromones emitted from the nest surface (Box 2A) as in other parasitized species (Feener and Brown, 1997; Morehead and Feener, 2000a). Phorids tend to cluster near disturbed workers, and cause reduced survivorship in areas where Azteca nests are very dense (Vandermeer et al., 2008; Philpott et al., 2009). As the flies hover to find a suitable host, there is a conspicuous change in ant behavior. Sometimes the ants simply flee, other times they appear to act aggressively toward the flies, as described in other ant-phorid systems (Feener, 1988). The threat of phorid attack also reduces Azteca activity at baits, interferes with their ability to protect trophobionts from predators and parasites, and limits their ability to defend plants against herbivores (Philpott et al., 2004; Hsieh and Perfecto, 2012; Hsieh et al., 2012). Knowledge of the chemical ecology of Azteca is limited, but three species (i.e., Azteca instabilis, A. nigriventris, and A. velox) release the same cyclopentyl ketones from their pygidial gland as defensive substances (Wheeler et al., 1975; Mathis et al., 2011). Although these species do not build carton nests, similar and overlapping compounds are widespread among Azteca spp. (Box 2) (Billen, 1986; Nascimento et al., 1998; Longino, 2007; McCann et al., 2013). One Pseudacteon species eavesdrops specifically on cis-1-acetyl-2-methyl-cyclopentane to locate and attack A. instabilis (Mathis et al., 2011). However, there is convincing evidence that visual cues (e.g., motion and ant shape) are also important for host-finding phorids, suggesting that the alarm-defense pheromones are part of multisensory decision making, leading to parasitoid success (Morehead and Feener, 2000b; Mathis et al., 2011).
At least 36 Pseudacteon phorid species parasitize Solenopsis invicta and Solenopsis saevissima; 17 phorid species attack Solenopsis invicta alone (reviewed in Chen and Fadamiro, 2018). The small Pseudacteon flies are attracted to disturbed fire ant mounds and hover above distressed and alarmed ants. Once the host colony is located, the flies move closer to size-select their victims for optimal offspring development (Morrison and Gilbert, 1999). Despite numerous studies on these flies, exactly how they locate fire ant nests from long distances remains unclear (Mathis and Philpott, 2012). However, piperidine venom alkaloids and 2,5-dimethyl-3-ethylpyrazine are both defensive substances (e.g., venom, alarm pheromones) that attract phorid flies in close-range interactions (Sharma and Fadamiro, 2013; Ngumbi and Fadamiro, 2015). Natural history accounts of the attraction of phorid flies to disturbed nests thus supports the experimental studies concluding that alarm pheromones are involved in host location (Morrison and King, 2004; Sharma and Fadamiro, 2013; Ngumbi and Fadamiro, 2015). The chemical ecology of fire ants is relatively well studied (Tschinkel, 2006), thus they are excellent research models for identifying the exact compounds that lure parasitoids and determining if they are indeed used by eavesdropping enemies and associates.
There are other notable advances in our understanding of chemical eavesdropping by phorid flies in a number of other systems. Paraponera clavata (Formicidae: Paraponerinae) are attacked by the phorid Apocephalus paraponerae. These phorids are attracted to the two major products of the mandibular glands of P. clavata, 4-methyl-3-heptanol and 4-methyl-3-heptanone (Feener et al., 1996). Interestingly, these compounds are found in alarm pheromones of many ant species belonging to other subfamilies (Morgan, 2008), including Atta and Acromyrmex (Norman et al., 2017). Ant species in the subfamily Formicinae produce formic acid in their venom and this compound is the primary host location cue attracting Pseudacteon formicarum to Lasius niger and Lasius emarginatus (Formicidae: Formicinae) (Maschwitz et al., 2008). Similarly, Myrmica rubra produces 3-octanone, non-anone and 3-octanol in their mandibular gland (Cammaerts et al., 1981) and these defense substances serve as attractants for Pseudacteon brevicauda. Phorid flies are most often specialists, attacking a single host ant species (Porter, 1998; Weissflog et al., 2008; Witte et al., 2010; Folgarait, 2013) but the chemical attractants of phorids may be more indiscriminate for long-range host localization. Once the fly has entered the habitat and found the nest or trail, they appear to use species-specific hydrocarbons to ensure species specificity (Mathis and Tsutsui, 2016). Phorids are recorded as the most-commonly observed associates among army ant bivouacs, raid trails, and refuse piles (Rettenmeyer and Akre, 1968). Hundreds of species interact with army ants and the genus Megaselia is by far the most common. Although observations suggest flies follow trails, eavesdropping on signals has not been formally demonstrated and remains a hypothesis. The great majority of phorid flies associated with army ants are found only in refuse piles, suggesting most species are opportunistic rather than ant-specific associates (Rettenmeyer and Akre, 1968).
Parasitoids of Brood
Wasps
Approximately 140 identified endo- or ectoparasitic wasp species (superfamilies: Chalcidoidea, Ichneumonoidea, Diaprioidea) attack ants at different life stages, including brood (Table 1) (reviewed in Lachaud and Pérez-Lachaud, 2012). Host-finding strategies vary where some wasps pursue host workers and others seek the host nest, both using host-derived chemicals. Below we present cases where the natural history of the parasitoids suggests that chemical eavesdropping plays an important role in host localization.
Orasema wasps (Hymenoptera: Chalcidoidea: Eucharitidae)
The pantropical wasp genus Orasema includes many species that are specialist parasitoids of Myrmicine ants (Heraty, 1994; Murray et al., 2013; Torréns, 2013). They are important natural enemies of ant colonies and specifically attack brood. The cues that guide female Orasema wasps to certain plants within their host ant territories currently are unknown (Torréns, 2013), but plant-derived volatiles likely attract the gravid female wasps, as is the case in other parasitoid systems (e.g., Wei et al., 2007). Once the wasps locate their host habitat (Figure 1A, top panel) they lay their eggs on specific plant structures (e.g., extrafloral nectaries or leaves) (Box 3A) (Heraty, 1994; Carey et al., 2012; Herreid and Heraty, 2017). The active wasp larva is then picked up by, or attaches itself to a host ant forager, suggesting that the wasp larva eavesdrops on CHCs or other host recognition pheromones (Figures 1B,C, top panel). While the ectoparasitic larva feeds (Box 3B) (Das, 1963), nurse ants tend the parasitoid brood, which are chemically similar to host brood and remain undetected (Vander Meer et al., 1989).
BOX 3.
Solenopsis fire ants and generalized natural history of the associates. Often foraging underground, fire ants use Z,E-α-Farnesene trail pheromones produced in their Dufour’s gland for recruitment and orientation (Suckling et al., 2010). Solenopsis invicta dominate habitats by recruiting nestmates with mandibular gland alarm pheromones (e.g., 2-ethyl-3,6-dimethylpyrazine) (Vander Meer et al., 2010) while also using toxic piperidene alkaloid venom against prey and competitors (Greenberg et al., 2008; Lai et al., 2010; Fox et al., 2019). In contrast, to these volatile defense pheromones, cuticular hydrocarbon-based nestmate and species recognition pheromones, primarily function in short-range communication (Leonhardt et al., 2016). (A) Eucharitid wasps, specialized parasitoids of ants, lay eggs in or on plant tissue. A 1st instar larvae attaches itself to a foraging ant then is carried back to the nest. (B) Once inside, the Eucharitid wasp larva locates, and then feeds on host brood (pupa pictured). (C) Inquiline social parasite queen Solenopsis daguerrei discovers a host nest, enters, and then attaches itself to the host queen. She will only produce sexual offspring, eventually killing the host colony. Illustrations by Rozlyn E. Haley adapted from photographs by Alex Wild.
Orasema wasps can be locally common in some regions of South America, with as many as half the fire ant colonies being affected (Varone et al., 2010). Field observations indicate that wasps parasitizing fire ants are often attacked and killed by workers upon emergence (Varone and Briano, 2009). In contrast, other wasp species that attack the brood of Ectatomma ants are carried outside the nest by the Ectatomma workers and dropped, apparently unharmed (Pérez-Lachaud et al., 2015). Orasema and other members of the species-rich wasp family Eucharitidae parasitize multiple ant subfamilies where the phylogenetic relationships within the genera are well understood (e.g., Ectatomma, Camponotus, Solenopsis) (Shoemaker et al., 2006; Clouse et al., 2015; Nettel-Hernanz et al., 2015). These lineages would be ideal for examining parasitoid-host coevolution or associate richness in the context of eavesdropping of chemical signals (see section Future Perspectives).
Diapriid wasps (Hymenoptera: Diaprioidea: Diapriidae)
The family Diapriidae has an estimated 4,000 species in three subfamilies (Ambositrinae, Belytinae, and Diapriinae) (reviewed in Lachaud and Pérez-Lachaud, 2012). The latter two lineages contain parasitoids of ant species; however, much of their natural history is unknown. Still, morphological adaptations such as winglessness and body sculpturing suggest host specificity and mimicry (Masner and García, 2002). Many species appear to be nocturnal, but those that attack army ants and fungus-growing ants attempt to enter host nests during the day (Masner and García, 2002; Fernández-Marín et al., 2006). Gravid females are challenged with the task of finding their host habitat (Figure 1A, bottom panel), then locating and invading the host colony which they presumably accomplish via species-specific nest marking cues (Figures 1B,C, bottom panel). They also must distinguish larvae from pupae during oviposition within the dark nest (Figure 1C, bottom panel). The young wasps develop amidst ant brood and emerge from the nest as adults seemingly unharmed by the resident ants.
Observations of host and parasitoid diapriid wasps (Hymenoptera: Diaprioidea: Diapriidae) are mainly descriptive accounts of parasitism (Loiácono et al., 2000; Fernández-Marín et al., 2006; Ramos-Lacau et al., 2007; Pérez-Ortega et al., 2010). Still, there are many reports of diapriid wasps associated with the nomadic columns of army ants (Loiácono et al., 2013a), most of which are in the tribe Diapriini; however, they are rarely collected because of their diminutive size. Several diapriids that associate with ants may actually exploit myrmecophiles and not the ants (e.g., dipterans Masner, 1977), thus the mere presence of these wasps in and around a nest is not definitive evidence for ant parasitism.
Diapriid wasp species from multiple genera (e.g., Acanthopria, Szelenyiopria, Mimopriella, Oxypria) associate with fungus-growing ant species (Hymenoptera: Formicidae: Myrmicinae: Attini: Attina) (Loiácono et al., 2000, 2013a,b; Fernández-Marín et al., 2006; Pérez-Ortega et al., 2010). In one study, 14 Cyphomyrmex rimosus colonies were artificially disturbed and forced to relocate their nests (Fernández-Marín et al., 2006). Males and females of Acanthopria wasps were reported to remain close to disturbed ants and brood but no mechanism to explain this close proximity was suggested. If nest disturbance attracts the wasps or induces the emergence of adults, then these individuals could be collected and used in trials with host ant pheromones to test for eavesdropping. The alarm pheromone, 1-octen-3-ol, produced by Cyphomyrmex rimosus and Trachymyrmex cornetzi (Norman et al., 2017; Hamilton et al., 2018) may be a good compound to explore first. Additionally, the chemical ecology of fungus-growing attine ants has recently expanded (Hogan et al., 2017; Norman et al., 2017; Hamilton et al., 2018) and the phylogenetic relationships within the Attina subtribe are well understood (Branstetter et al., 2017), therefore this lineage of host ants offers great experimental potential for the identification of parasitoid attractants.
Syrphid flies (Diptera: Syrphidae: Microdontinae)
Approximately 20% of all flies are parasitoids (Feener and Brown, 1997). The family Syrphidae (i.e., hoverflies, flower flies) contains ca. 6,000 species globally (Pérez-Lachaud et al., 2014), and includes many important pollinators, parasitoids, and predators (Table 1). The syrphid Hypselosyrphus trigonus is a neotropical parasitoid that resembles a stingless bee and attacks ponerine ants (e.g., Neoponera villosa) (Pérez-Lachaud et al., 2014; Pérez-Lachaud and Lachaud, 2017). Apparently, this is the first parasitoid fly known to attack the brood of ants (Pérez-Lachaud et al., 2014). The gravid female—much like diapriid wasps—seeks host habitat (Figure 1A, bottom panel), and then likely uses species-specific host cues to locate and enter the host nest (Figures 1B,C, bottom panel). Finally, they are challenged to determine the location of the brood in the nest and oviposit on prepupae (Figure 1C, bottom panel) (Pérez-Lachaud et al., 2014). While other microdontine syrphids are known ant brood predators (see below) (Elmes et al., 1999), this case of parasitism is remarkable given that Hypselosyrphus females must safely enter the ant colony, presumably undetected. Eavesdropping by these flies is so far untested.
Predators of Both Workers and Brood
Many predacious invertebrates feed on ant workers and brood, including spiders, caterpillars, beetles, and flies (Table 1). Just like the parasitoids, these predatory interactions occur both inside and outside of the nest, and have the potential to be facilitated by chemical eavesdropping. They are challenged with encountering their prey where first they locate habitat (Figure 1A) then a worker (Figure 1B, top panel) or brood within the nest (Figure 1B, bottom panel), depending on which life stage they prey on. Finally, they consume their prey (Figure 1C).
Paussus Ant-Nest Beetles (Coleoptera: Carabidae: Paussinae)
The paussines or ant-nest beetles are a large subfamily of nearly 800 species that are facultative and obligate associates that prey on the brood and workers of mainly myrmicine and formicine species (Table 1) (reviewed in Geiselhardt et al., 2007). They have characteristic adaptations (e.g., morphology, chemical weaponry, sound production) that indicate a long coevolutionary history with ants (Maurizi et al., 2012) but have also undergone rapid adaptive radiation (Moore and Robertson, 2014). While most ant-beetle interactions are described anecdotally in taxonomic papers, some natural history and experimental evidence indicates that paussines eavesdrop on pyrazine trail pheromones (Ali et al., 1988; Cammaerts et al., 1990). Laboratory experiments using glandular extractions of Pheidole pallidula trail pheromones showed that adult Paussus (Edaphopaussus) favieri follow host trails (Cammaerts et al., 1990). While inside the nest, the larvae and adult beetles prey on the host ants (eggs, larvae, adults). It is possible that they detect chemicals that mark different areas of the nest (e.g., brood chamber vs. non-brood chamber) (Heyman et al., 2017) or brood-specific contact pheromones (Walsh and Tschinkel, 1974); however, both hypotheses require experimental verification.
Myrmecophagous Spiders
Many spiders use chemical cues to locate mates and prey (Foelix, 2010; Johnson et al., 2011), and chemical eavesdropping on ants occurs, or is suspected to occur in a variety of cases (Table 1). The clearest example is the spider Habronestes bradleyi, which locates workers of its preferred prey, Iridomyrmex purpureus ants, by eavesdropping on their alarm pheromones (Table 3) (Allan et al., 1996). Specifically, Allan et al. (1996) experimentally demonstrated that 6-methyl-5-hepten-2-one released by injured or agitated ants is used as a prey location cue by the spider. Although less definitive, several other cases provide circumstantial evidence for chemical eavesdropping on ants by spiders. For example, orb-weaving spiders aggregate near lycaenid butterflies that feed from Acacia trees, apparently by detecting pheromones produced by Acacia-inhabiting ants (Elgar et al., 2016). In another example, the ant-eating spider Zodarion rubium recognizes specific chemical cues produced by glands from formicine ants (Cárdenas et al., 2012). Similarly, prey-seeking behaviors of the ant-eating jumping spider Habrocestum pulex are enhanced by exposure to ant chemical cues in soil (Clark et al., 2000). However, in cases where the spiders themselves are vulnerable to predation by ants, ant-derived compounds were shown to elicit habitat avoidance or increased dispersal behavior (Mestre et al., 2014; Penfold et al., 2017).
Syrphid Flies (Diptera: Syrphidae: Microdontinae)
The nearly 350 species of Microdon syrphid flies are known only from social insect nests (Table 1) (Wheeler, 1908). The gravid females of the myrmecophilous species locate the habitat and seek their host’s nest to deposit eggs in or on the nest structure, presumably eavesdropping on species-specific chemicals such as nest-marking pheromones (Figures 1A–C, bottom panel) (Akre et al., 1988). The oddly flattened larvae complete the host acceptance phase when they migrate into the nest (infiltration and integration), then to the brood chambers to consume larvae and pupae (Garnett et al., 1985). Microdon piperi larvae live inside the nest of Camponotus modor, where they feed on host brood. Adults of this species are attracted to excited host workers spraying formic acid alarm pheromones (Akre et al., 1988), suggesting that males and females may use host pheromones to find nests and potential mates, but this was not experimentally determined. Microdon mutabilis specifically attacks the larvae of the alpine ant Formica lemani (Elmes et al., 1999). Females of M. mutabilis extend their ovipositor when exposed to volatile odors from F. lemani colonies, suggesting that they use host-derived pheromones to locate the host nest before laying their eggs. The active compound was experimentally demonstrated to be methyl-6-methylsalicylate from the ants’ head extract (Table 3) (Schönrogge et al., 2008). This compound occurs in pheromones of various ants [e.g. Camponotus (Torres et al., 2001); Tetramorium (Morgan et al., 1990)], and therefore should be used in additional studies to determine eavesdropping in other systems.
Lycaenid Caterpillars (Lepidoptera: Lycaenidae)
Of the estimated 6,000 species of lycaenid butterflies, over 600 species (10 genera) associate symbiotically with ants, but the total number of myrmecophilous species is presumed to be much larger (reviewed in Pierce et al., 2002). These include a number of obligate parasitic or mutualist ant associates, and at least 10 species feed on brood inside ant nests (Table 1) (Pierce et al., 2002). Even where not strictly myrmecophilous, lycaenid associates display adaptations (both as caterpillars and adults) that protect them from ant aggression (Pierce et al., 2002), suggesting a long coevolutionary history between Lycaenidae and the Formicidae. Some myrmecophilous lycaenids eavesdrop on host trail pheromones (Dejean and Beugnon, 1996). The lycaenid caterpillars Euliphyra mirifica and E. leucyana for instance live inside the ant nest of Oecophylla longinoda and follow their trail pheromones (Dejean and Beugnon, 1996). In contrast to other lycaenid species, these are commensals and do not impose a significant fitness cost to the ants. Similar chemical eavesdropping by other lycaenid species is likely but in the context of predation (e.g., Liphyra brassolis) (Dodd, 1912) and warrants more research.
Resource Exploitation by Cleptoparasites, Social Parasites, and Myrmecophiles
Many ant species use chemical trails to recruit nestmates to food resources (Hölldobler and Wilson, 1990). While host ant colonies must rely on these trail systems, other organisms use them as highways to ambush foragers or to find and invade host nests (Table 1). The marked diversity of trail following eavesdroppers (e.g., roaches, caterpillars, and ant species) illustrates the risk associated with ant chemical communication. Direct observations of trail following myrmecophiles and cleptoparasites are plentiful (Table 2) but there is a noticeable gap in evidence demonstrating trail following in other types of parasites. Furthermore, the chemical composition of trail pheromones has been determined for many species, but the specific components are often difficult to synthesize (reviewed in Morgan, 2008, 2009). Thus experimental studies commonly rely on glandular extractions to demonstrate eavesdropping (note, despite our literature review, we could not find studies that directly test specific trail pheromone compounds and therefore none could be included in Table 3).
Trail Following Cleptoparasites
Long-lasting trail systems present an apparent vulnerability in host ant colony defense (Tables 1, 2). A comprehensive list of the cleptoparasites of ants (i.e., parasites that steal food from their hosts inside or outside the nest) is beyond the scope of this review; however, we present a few examples backed with experimental evidence for trail-following as a host-association mechanism. The natural history of cleptoparasites is partly determined by the location of host food sources, including (1) colony nest stores; (2) from workers via trophallaxis (i.e., mutual exchange of regurgitated liquids); and (3) at food sources or trails outside the nest. Cleptoparasites stealing food from inside the host ant nest are faced with the challenge of habitat and nest location (Figures 1A,B, bottom panel). By contrast, those stealing food from outside the nest must locate the habitat and intercept the host ants on trails to gather resources (Figures 1A,B, top panel). Regardless, eavesdropping cleptoparasites illustrate how the structure of social insect colonies (i.e., fixed nest location and central-point foraging) provide variable access to resources that potentially influences parasite community structure.
Cockroaches (Blattaria: Polyphagidae)
An emblematic example of a trail-following nest associate is provided by cockroaches living with leaf-cutting ants (Table 1). At least six species of Attaphila (Blattaria: Polyphagidae) cockroaches live in Atta and Acromyrmex nests (Rodríguez et al., 2013). These cockroaches are small (ca. 3.5 mm) and wingless with reduced sensory and glandular systems (Box 1D) (Brossut, 1976; Wheeler, 1900). They live in the ants’ fungus garden, where they graze (Wheeler, 1900; Phillips et al., 2017) and acquire nest-specific cuticular hydrocarbon signatures (Nehring et al., 2016) while behaving as cleptoparasites (Table 1). Attaphila climb onto virgin Atta queens just before they leave the nest, and remain attached until they are brought into the new host nest. This suggests that the roaches can differentiate between castes, and may use queen-specific pheromones (Dietemann et al., 2003). Phillips et al. (2017) suggested that the young cockroaches use host queens for dispersal and habitat location (Figure 1A, bottom panel) but can also move to more established nests by following Atta trails (Figure 1B, bottom panel) (Moser, 1964). Atta texana use methyl 4-methylpyrrole-2-carboxylate as their volatile trail pheromone (Tumlinson et al., 1972), but the concentration and exact trail components used by these cleptoparasites and other Atta myrmecophiles (Box 1) (Waller and Moser, 1990) deserve further exploration.
Ants (Hymenoptera: Formicidae)
Some cleptoparasite ants eavesdrop on trail pheromones of other ant species to access food (Tables 1, 2) (Gobin et al., 1998; Powell et al., 2014). Such trail parasitism is best known among arboreal species, and is not limited to closely related or parabiotic (i.e., nest sharing) species (Table 2). For example, some Cephalotes and Camponotus species are conspicuous trail parasites of Azteca (Box 2B) (Wilson, 1965; Adams, 1990). Specifically, Cephalotes maculatus follows Azteca trail pheromones and coexists undetected at food resources with Azteca workers (Adams, 1990, 2016). Data from multiple surveys of >150 trees in Panama showed that Azteca trigona co-occurs more frequently with Cephalotes maculatus than expected by chance (Adams et al., 2017). A similar phenomenon was shown between the highly aggressive Crematogaster ampla host and the comparably demure Cephalotes specularis trail parasite (Powell et al., 2014). While the host actively defends foraging territories, the trail parasite eavesdrops on the host’s trail pheromones, locates, and then exploits the food resources. A remarkable 89% of the host territories contained the parasites, and host ants showed no aggression toward them. Trail pheromone eavesdropping behavior clearly is widespread in ants (Table 2), and hosts and parasites often belong to different ant subfamilies.
Trail Following Social Parasites (Hymenoptera: Formicidae)
Social parasitism has evolved independently numerous times among the Formicidae (Buschinger, 2009). While there are many types of social parasites (e.g., inquilines, slave-makers, thief ants, guest ants) they are faced with the same challenges. They must locate the habitat and nest of their host (Figures 1A,B, bottom panel), infiltrate the colony (Figure 1C, bottom panel), and extract colony resources (e.g., food and brood) (Figures 1C–E, bottom panel). Unfortunately, host nest searching behavior is infrequently observed in social parasites. Mycocepurus castrator inquiline parasites experimentally placed near a host nest quickly entered the nest signifying that they are able to sense their host (Rabeling and Bacci, 2010). Dulotic slave-making species and Megalomyrmex agro-predator raiders also find host colonies as young queens or later as scout workers (Boudinot et al., 2013; Ruano et al., 2013). This suggests they are using host-derived chemical cues to find the host nest and brood (Table 1). Trail pheromones and nest-marking pheromones with low volatility are found around host nesting areas (Hölldobler and Wilson, 1986; Cammaerts and Cammaerts, 1998; Steck, 2012) and likely play an important role in the host-finding of many ant associates (Table 1), not only cleptoparasites (Table 2). Eavesdropping on host-derived signals may be most effective when used in concert with nest searching behavior. For example, Gnamptogenys hartmanni, which are social parasites of Trachymyrmex and Sericomyrmex fungus-growing species, will search small holes near host nests (Dijkstra and Boomsma, 2003). Nest-searching behavior by scout ants coupled with the detection of host-derived trail and nest-marking pheromones likely work synergistically for host nest invasion. Finally, dulotic slave-maker parasites have evolved independently at least 10 times (reviewed in D’Ettorre and Heinze, 2001). A comparative study examining eavesdropping across lineages would offer great insight into which host traits leave host ants vulnerable to colony invasion.
Like slave-makers and agro-predators, socially parasitic inquiline queens also seek and invade host nests alone. Pogonomyrmex colei, an inquiline of Pogonomyrmex rugosus, finds its host colony by following host trunk trails with or without host workers, suggesting eavesdropping on host trail pheromones (Johnson et al., 1996). Another inquiline is the well-studied Solenopsis daguerrei, which lacks a worker caste. Young queens invade a fire ant colony and firmly attach to the functional host queen (Box 3C) (Calcaterra and Briano, 1999). The parasites lay eggs that are intermingled with eggs from the host queen and are reared by host workers, ultimately resulting in the production of parasitic males and females. This intimate and intricate association presumably involves eavesdropping at different levels. While host nest mounds (Box 3) and other environmental cues may aid in habitat location, trail or alarm pheromones may attract the host-seeking parasite queen. She likely locates the queen within the nest by eavesdropping on queen-specific pheromones as this level of recognition is essential for her success. Solenopsis daguerrei can parasitize a number of fire ant species that are chemically distinct (Fox, 2018) suggesting that ant chemical mechanisms for invasion are not species-specific. This also implies the “encounter phase” (Figures 1A-C, bottom panel) may involve host cues (e.g., nest-marking, trail, and alarm pheromones) that are shared among host species. As it stands, the chemical mechanisms for this intricate symbiosis warrants further studies but the known natural history points to eavesdropping as an essential component of social parasite success.
Trail Following Myrmecophiles
While myrmecophiles associate with countless ant lineages, the army ants likely host the most species that are currently known (Rettenmeyer et al., 2011). Indeed, the vast array of ant associates makes their functional classification exceptionally difficult (Gotwald, 1995). Here, we use “myrmecophile” in cases where the nature of the association is not well defined or is deemed commensal, but fundamentally involves any organism that lives at least part of its life associated with ants (see Table 1).
Trail-following army ant associates are easily observed in the field, thus contributing to the large list of taxa in this category (Table 1). However, identifying species remains a significant challenge (von Beeren et al., 2018). Many associates specifically follow ant trails, and some myrmecophiles are more sensitive to trail pheromones than are the ants (Akre and Rettenmeyer, 1968). All of the ca. 30 known species of Vatesus beetles (Staphylinidae: Tachyporinae) are army ant associates (von Beeren et al., 2016) whose adults and larvae follow ant trails (Akre and Rettenmeyer, 1968; Akre and Torgerson, 1969). While the nature of the association is often unknown, some beetles consume army ant booty (i.e., cleptoparasites) and dead army ant brood (i.e., commensals) (Akre and Torgerson, 1969).
Tiny myrmecophilous crickets are wingless ant symbionts comprising some 60 described species that span the temperate and tropical regions of the world (Kistner, 1982). These cricket species associate with ant species in multiple genera (e.g., Atta, Formica, Pogonomyrmex) (Wheeler, 1900; Waller and Moser, 1990). Some crickets are known to be egg predators or cleptoparasites, where they either disrupt food exchange between ants, or are directly fed (Henderson and Akre, 1986). In most cases, the natural history of the crickets is unknown, thus they are simply categorized as myrmecophiles. Host specificity differs where some species are ant generalists and others have traits that suggest a long coevolutionary history with their host (e.g., trail following, chemical integration strategy) (Wheeler, 1900; Akino et al., 1996; Wetterer and Hugel, 2008; Komatsu et al., 2013). Myrmecophila manni (Orthoptera: Gryllidae) live with Formica obscuripes, the western thatch ant, and follow their host trails after colony migration to a new nest, suggesting eavesdropping on trail pheromones (Henderson and Akre, 1986). However, the host-finding mechanisms used during the encounter phase (Figures 1A–D) of most ant-associated crickets is unknown, and the generality of trail following is in need of further study.
Conclusion
Successful antagonistic interactions are initiated by host-finding mechanisms that lead to efficient exploitation tactics. We have illustrated a parasitological framework to summarize the necessary encounter and exploitation phases used by ant enemies and other associates (Combes, 2005). We move beyond the initial stage of habitat location to focus on the stages where eavesdropping on host-derived pheromones is most likely to occur (Figures 1B,C). We distinguish between associates that attack individual ants outside the nest (e.g., phorid flies) and those that breach the protected fortress to reach ant colony resources (food, brood, workers, queen) (e.g., social parasites). Regardless of the initial target (individual or nest), associates locate their host using either long- or short-range chemical cues (Figure 1). We found that nine out of 10 different organism types (i.e., beetle, fly, spider, wasp, cockroach, butterfly, ant, silverfish, and cricket) may follow their host’s trail pheromones (Table 1). These include parasitoids, predators, and parasites (i.e., social parasites and cleptobionts), although more experimental work is needed to support this hypothesis. However, a number of cleptoparasite ant species have been shown to be “trail parasites” that tend to nest in the forest canopy (Table 2).
Another form of chemical communication dispersed by host ants is alarm pheromones. The volatile nature of alarm pheromones may contribute to the fact that most of the putative eavesdropping associates are flying insects (Table 1), likely detecting the compounds in air currents. Interestingly, alarm/defensive pheromones were the only experimentally determined compounds used by eavesdropping associates (Table 3). We also found that a single species may eavesdrop on multiple pheromones emitted by their host. For example, they may initially follow trail pheromones, then use recognition pheromones and contextual cues to locate the queen, workers, or brood (see Boxes 1B, 3C). We conclude that although there is extreme diversity in the organisms that exploit ants (25 families or arthropods in 10 orders; Table 1), the stages of successful exploitation (Figure 1) and host-finding mechanisms are shared among many.
The extensive natural history of host ant species and their associated parasitoids, predators, parasites, and myrmecophiles provides the needed groundwork for hypothesis-driven studies on chemical eavesdropping. The frequency and abundance of ant associates varies among host lineages, perhaps due to heritable traits that make some species more prone to eavesdroppers than others. More research is needed to identify these traits, and the specific compounds that natural enemies exploit. Determination of the key compounds, and their physiological and behavioral effects, will provide a foundation for comparative studies (e.g., Ngumbi and Fadamiro, 2015). Such studies will clarify the evolutionary trajectories of specific compounds (Norman et al., 2017; Brückner et al., 2018; Hamilton et al., 2018) and improve our understanding of their perception by ant associates. Finally, an evolutionary approach to ant chemistry and eavesdropping will provide a basis for understanding how eavesdroppers and other ant associates, can shape the evolution of pheromone profiles.
Future Perspectives
Are Eavesdropping Coinfection Rates (i.e., Associate Richness) Due to Predictable Host Traits?
Chemical communication structures communities in part by mediating species interactions. Ants are key components of most terrestrial ecosystems (Gao et al., 2016) and a tractable model for exploring the details of chemical communication systems. This review revealed that we know relatively little about the basics of eavesdropping on ant pheromones, and far less about its broader ecological consequences. We propose that ant-specific traits, including their communication systems, influence their susceptibility to eavesdropping associates. Inspired by parasitological theory (Combes, 2001, 2005; Poulin, 2007), below we outline some testable hypotheses that have the potential to advance this field. We encourage an integrative approach applied to multiple host-associate systems to account for confounding variables, and we identify lineages where comparative research is likely to be especially fruitful. We expect that DNA barcoding (Baker et al., 2016) and network-based approaches will facilitate more sophisticated studies, enhancing the understanding of the ecological impact of these associations (reviewed in Ivens et al., 2016).
We suggest that the traits of some ant species will determine their susceptibility to diverse associates including parasitoids, cleptoparasites, social parasites, myrmecophiles, and some predators (Table 1). We tailored our trait-linked hypotheses to address associate richness in the context of host-seeking strategies that involve eavesdropping on ant chemical communication.
Latitudinal gradient
Associate diversity varies with latitude. Given that ant species richness increases from the poles to the tropics (Willig et al., 2003; Economo et al., 2018), the potential for symbiotic interactions should also increase. Few studies have addressed this hypothesis in non-ant hosts (e.g., Salkeld et al., 2008; Torchin et al., 2015); to our knowledge, no one has investigated this pattern in ants despite prevalent lists of ant-nest associates (Hölldobler and Wilson, 1990; Waller and Moser, 1990; Schmid-Hempel, 1998; Navarrete-Heredia, 2001; Rettenmeyer et al., 2011; Briano et al., 2012). Studies involving host species that span broad latitudinal gradients would be ideal. Alternatively, comparative studies of host lineages that contain species with similar natural history traits may also prove useful. Key prediction: Per-host associate richness is relatively higher in tropical regions.
Diverse microhabitats
Associate diversity is affected by the structural complexity of the host nest. Ant nests are compartmentalized into different chambers containing the queen, brood, workers, food, and detritus. For example, leaf-cutter ants have four kinds of nest chambers (empty, garden filled, dirt/sand filled, and detritus filled chambers) (Moser, 2006; Forti et al., 2017) whereas other species may have 3-4 (entrance, queen, worker with brood, worker without brood) (Heyman et al., 2017), providing vastly different microhabitats. Ants often maintain unique chemical signatures in different chambers, providing spatiotemporal guidance for workers (Heyman et al., 2017). It seems likely that nest associates use these chemical cues to differentiate among regions of the complex nest structure. Refinement of associate niche axes based on subtle but consistent differences in chemistry within an ant nest presumably leads to increased associate richness. To test this hypothesis, ant associate abundance can be evaluated in comparative studies where similar species vary in nest complexity (single chamber e.g., Acromyrmex vs. complex nests e.g., Atta). Key prediction: As host nest structure increases, associate species richness increases.
Long-lived large colonies/gregariousness
Ant associates have more opportunities to detect alarm, nest, and trail pheromones from large, long-lived ant colonies. Eusocial insects, specifically queens, have increased longevity compared to solitary insects (Keller and Genoud, 1997) where some live up to 30 years (Boxes 1, 2) (Hölldobler and Wilson, 1990). These large old colonies can therefore accumulate numerous associates, as they provide resources and homeostatic conditions (Hughes et al., 2008). Territoriality behavior is often expressed in workers of large colony species, and disputes with competing hetero- and conspecifics are frequent (Adams, 2016). While conflicts may cause the ants to emit alarm pheromones, nest- and trail-marking pheromones allow species to partition rival nests in space; together these pheromones leave large, persistent colonies vulnerable to nest-seeking associates that can eavesdrop on these chemical cues (Orr et al., 2003). To test this hypothesis, ant associate abundance can be compared in young vs. old or large vs. small colonies. Key prediction: As host colony size and age increases, associate species richness increases.
Nest migration/extensive trail systems
Species with frequent nest migration are more likely to be discovered by ant associates. Army ants are constantly laying trail pheromones (Oldham et al., 1994), and excrete volatile alarm substances when in conflict (Brückner et al., 2018). They do not construct permanent nests, but instead have statary (i.e., stationary) and nomadic phases (Gotwald, 1995). Regardless, if workers are leaving the bivouac to conduct daily raids or the colony is migrating at night, trail and alarm pheromones may enhance their vulnerability to new associates. Eciton army ants and leaf-cutter Atta species are known for their large colony sizes and numerous and diverse nest associates; however, unlike army ants, Atta colonies are stationary and maintain the same trail systems for years (Box 1) (Kost et al., 2005). Future studies comparing Atta and Eciton could test if repeated nest migrations or widespread trail systems are responsible for their susceptibility to arthropod associates. Key prediction: Life histories that involve frequent nest migration with extensive trail systems have higher associate species richness.
Nesting location
Nesting habit influences the conspicuousness of ant colonies and their corresponding communication channels. Underground: Many ant species nest underground where communication systems are buffered by soil, thereby limiting associate encounter rate. Leaf litter: The physical structure of leaf litter changes almost daily and is three-dimensionally complex at small spatial scales (Yanoviak and Kaspari, 2000). Thus, ant pheromone trails are less reliable, short-lived and presumably harder to follow. However, the transitory nature of the substrate causes species to frequently move nest locations (also see Kaspari, 1996), which can expose them to opportunistic parasites [e.g., phorids attacking brood (Brown et al., 2017)]. Arboreal: Some arboreal species construct single or multiple visually conspicuous nests in trees (e.g., Wheeler, 1986; Adams and Longino, 2007). These ant species lay trails on vines, branches, and trunks that are exploited by cleptoparasite caterpillars (Dejean and Beugnon, 1996) and ants (Box 2 and Table 2) (Powell et al., 2014). It is probable that the location of trail systems determines the likelihood that they are exploited by eavesdroppers. For example, an underground trail system presumably is less likely to be detected by potential associates than an exposed arboreal trail system. Future studies contrasting closely related species with different trail strategies (e.g., arboreal versus leaflitter dwelling Cyphomyrmex species) (Weber, 1941; Adams and Longino, 2007) would be ideal to test this hypothesis. Key prediction: Associate species richness correlates with nest type and location.
Extant species-rich host lineage
The potential for host switching increases if the host lineage has many species with similar pheromones (e.g., alarm, trail pheromones, etc.) (Blum and Brand, 1972). While work centered on host ant preference by various associates is important foundational research [e.g., Eucharitidae wasps (Murray et al., 2013); Paussus ant-nest beetles (Moore and Robertson, 2014); phorid flies (Mathis and Philpott, 2012; Chen and Fadamiro, 2018); social parasites (Lenoir et al., 2001; Buschinger, 2009)], this hypothesis shifts the focus to the associate community where network-based analyses will prove useful (Ivens et al., 2016). In order to investigate this hypothesis, we must begin with host lineages having (1) a well-resolved phylogeny, (2) a well-studied communication system, and (3) numerous known associates. Although a daunting task, research programs focusing on ant lineages that contain pest species (e.g., Solenopsis, Atta) would be most promising. Key prediction: Associate richness is higher in host lineages with many closely related extant species.
Author Contributions
All authors listed have made a substantial, direct, and intellectual contribution to this work. RA originally drafted the Introduction, fungus-growing ant sections, Conclusion, and Future Perspectives. EF originally drafted the Solenopsis section. SY, RW, and CF originally drafted the Azteca section. EF, RW, and RA assembled the tables. RA designed the figures. All authors finalized the text in all sections.
Funding
Funding was provided in part by NSF IOS-1656625 to CF, NSF DEB-1252614 to SY, and NSF GRF-2018265609 to RW. RA is supported by The Ohio State University.
Conflict of Interest
The authors declare that the research was conducted in the absence of any commercial or financial relationships that could be construed as a potential conflict of interest.
Acknowledgments
We thank Rozlyn E. Haley for providing illustrations for Figure 1 and Boxes 1–3, adapted from Alex Wild’s photographs (www.alexanderwild.com). We thank Elaine Finch and Darria Streeter for assistance in gathering and organizing literature. We also thank Ximena Bernal and Rachel Page for helpful comments and the invitation to contribute to this special edition. Our manuscript was greatly improved by multiple rounds of thought provoking comments and useful suggestions. We sincerely thank our three reviewers.
Footnotes
References
Adams, B. J., Schnitzer, S. A., and Yanoviak, S. P. (2017). Trees as islands: canopy ant species richness increases with the size of liana-free trees in a neotropical forest. Ecography 40, 1067–1075. doi: 10.1111/ecog.02608
Adams, E. S. (1990). Interaction between the ants Zacryptocerus maculatus and Azteca trigona: interspecific parasitism of information. Biotropica 22, 200–206. doi: 10.2307/2388413
Adams, E. S. (1994). Territory defense by the ant Azteca trigona: maintenance of an arboreal ant mosaic. Oecologia 97, 202–208. doi: 10.1007/BF00323150
Adams, E. S. (2016). Territoriality in ants (Hymenoptera: Formicidae): a review. Myrmecol. News 23, 101–118.
Adams, R. M. M., and Longino, J. T. (2007). Nesting biology of the arboreal fungus-growing ant Cyphomyrmex cornutus and behavioral interactions with the social-parasitic ant Megalomyrmex mondabora. Insect. Soc. 54, 136–143. doi: 10.1007/s00040-007-0922-920
Akino, T. (2002). Chemical camouflage by myrmecophilous beetles. Chemoecology 12, 83–89. doi: 10.1007/s00049-002-8330-8334
Akino, T. (2008). Chemical strategies to deal with ants: a review of mimicry, camouflage, propaganda, and phytomimesis by ants (Hymenoptera: Formicidae) and other arthropods. Myrmecol. News 11, 173–181.
Akino, T., Mochizuki, R., Morimoto, M., and Yamaoka, R. (1996). Chemical camouflage of myrmecophilous cricket Myrmecophilus sp. to be integrated with several ant species. JPN. J. Appl. Entomol. Zool. 40, 39–46. doi: 10.1303/jjaez.40.39
Akre, R. D., Alpert, G., and Alpert, T. (1973). Life cycle and behavior of Microdon cothurnatus in Washington (Diptera: Syrphidae). J. Kansas Entomol. Soc. 46, 327–338.
Akre, R. D., Garnett, W. B., and Zack, S. (1988). Biology and behavior of Microdon piperi in the Pacific Northwest (Diptera: Syrphidae). J. Kansas Entomol. Soc. 61, 441–452.
Akre, R. D., and Rettenmeyer, C. W. (1968). Trail-following by guests of army ants (Hymenoptera: Formicidae: Ecitonini). J. Kansas Entomol. Soc. 41, 165–174.
Akre, R. D., and Torgerson, R. L. (1969). Behavior of Vatesus beetles associated with army ants. Pan Pac. Entomol. 48, 269–281. doi: 10.5860/choice.48-6651
Ali, M. F., and Morgan, E. D. (1990). Chemical communication in insect communities: a guide to insect pheromones with special emphasis on social insects. Biol. Rev. 65, 227–247. doi: 10.1111/j.1469-185X.1990.tb01425.x
Ali, M. F., Morgan, E. D., Detrain, C., and Attygalle, A. B. (1988). Identification of a component of the trail pheromone of the ant Pheidole pallidula (Hymenoptera: Formicidae). Physiol. Entomol. 13, 257–265. doi: 10.1111/j.1365-3032.1988.tb00477.x
Allan, R. A., Elgar, M. A., and Capon, R. J. (1996). Exploitation of an ant chemical alarm signal by the zodariid spider Habronestes bradleyi Walckenaer. Proc. R. Soc. B Biol. Sci. 263, 69–73. doi: 10.1098/rspb.1996.0012
Attygalle, A. B., and Morgan, E. D. (1984). Chemicals from the glands of ants. Chem. Soc. Rev. 13, 245–278. doi: 10.1039/cs9841300245
Bagnères, A., and Wicker-Thomas, C. (2010). “Chemical taxonomy with hydrocarbons,” in Insect Hydrocarbons Biology, Biochemistry, and Chemical Ecology, eds G. J. Blomquist and A.-G. Bagnères (New York: Cambridge University Press), 121. doi: 10.1017/cbo9780511711909.008
Baker, C. C. M., Bittleston, L. S., Sanders, J. G., and Pierce, N. E. (2016). Dissecting host-associated communities with DNA barcodes. Philos. Trans. R. Soc. B Biol. Sci. 371:20150328. doi: 10.1098/rstb.2015.0328
Barbero, F., Thomas, J. A., Bonelli, S., Balletto, E., and Schönrogge, K. (2009). Queen ants make distinctive sounds that are mimicked by a butterfly social parasite. Science 323, 782–785. doi: 10.1126/science.1163583
Baroni Urbani, C. (1969). “Trail sharing between Camponotus and Crematogaster: some comments and ideas,” in Proceedings of the 6th Congress of the International Union for the Study of Social Insects (IUSSI), Bern, 11–17.
Bhatkar, A. P. (1982). Orientation and defense of ladybeetles (Coleoptera, Coccinellidae) following ant trails in search of aphids. Folia Entomol. Mex. 53, 75–85.
Billen, J. (1986). Morphology and ultrastructure of the abdominal glands in Dolichoderine ants (Hymenoptera, Formicidae). Insect. Soc. 33, 278–295. doi: 10.1007/BF02224246
Billen, J. (2009). “Diversity and morphology of exocrine glands in ants,” in Proceedings of the XIX Simpósio de Mirmecologia (Ouro Preto), 17–21.
Binz, H., Foitzik, S., Staab, F., and Menzel, F. (2014). The chemistry of competition: exploitation of heterospecific cues depends on the dominance rank in the community. Anim. Behav. 94, 45–53. doi: 10.1016/j.anbehav.2014.05.024
Blomquist, G. J., and Bagnères, A. G. (2010). Insect Hydrocarbons Biology, Biochemistry, and Chemical Ecology. Cambridge: Cambridge University Press, doi: 10.1017/CBO9780511711909
Blum, M. S., and Brand, J. M. (1972). Social insect pheromones: their chemistry and function. Integr. Comp. Biol. 12, 553–576. doi: 10.1093/icb/12.3.553
Blum, M. S., Padovani, F., and Amante, E. (1968). Alkanones and terpenes in the mandibular glands of Atta species (Hymenoptera: Formicidae). Comp. Biochem. Physiol. 26, 291–299. doi: 10.1016/0010-406X(68)90333-90332
Boudinot, B. E., Sumnicht, T. P., and Adams, R. M. M. (2013). Central American ants of the genus Megalomyrmex Forel (Hymenoptera: Formicidae): six new species and keys to workers and males. Zootaxa 3732, 1–82. doi: 10.11646/zootaxa.3732.1.1
Branstetter, M. G., Ješovnik, A., Sosa-Calvo, J., Lloyd, M. W., Faircloth, B. C., Brady, S. G., et al. (2017). Dry habitats were crucibles of domestication in the evolution of agriculture in ants. Proc. R. Soc. B Biol. Sci. 284:20170095. doi: 10.1098/rspb.2017.0095
Brian, M. V. (1975). Larval recognition by workers of the ant Myrmica. Anim. Behav. 23, 745–756. doi: 10.1016/0003-3472(75)90102-90105
Briano, J., Calcaterra, L., and Varone, L. (2012). Fire ants (Solenopsis spp.) and their natural enemies in southern South America. Psyche 2012:198084. doi: 10.1155/2012/198084
Brossut, R. (1976). Étude morphologique de la Blatte myrmécophile Attaphila fungicola wheeler morphology of the myrmecophilous cockroach Attaphila fungicola wheeler. Insect. Soc. 23, 167–174. doi: 10.1007/bf02223849
Brown, B., Hash, J., Hartop, E., Porras, W., and Amorim, D. (2017). Baby killers: documentation and evolution of scuttle fly (Diptera: Phoridae) parasitism of ant (Hymenoptera: Formicidae) brood. Biodivers. Data J. 5:e11277. doi: 10.3897/BDJ.5.e11277
Brown, B. V. (2001). Taxonomic revision of Neodohrniphora, subgenus Eibesfeldtphora (Diptera: Phoridae). Insect. Syst. Evol. 32, 393–409. doi: 10.1163/187631201X00272
Brown, B. V., and Feener, D. H. (1991). Behavior and host location cues of Apocephalus paraponerae (Diptera: Phoridae), a parasitoid of the giant tropical ant, Paraponera clavata (Hymenoptera: Formicidae). Biotropica 23, 182–187.
Brückner, A., Hoenle, P. O., and von Beeren, C. (2018). Comparative chemical analysis of army ant mandibular gland volatiles (Formicidae: Dorylinae). PeerJ 2018:e5319. doi: 10.7717/peerj.5319
Buschinger, A. (2009). Social parasitism among ants: a review (Hymenoptera: Formicidae). Myrmecol. News 12, 219–235.
Calcaterra, L. A., and Briano, J. A. (1999). Field studies of the parasitic ant Solenopsis daguerrei (Hymenoptera: Formicidae) on fire ants in Argentina. Biol. Control 28, 88–95. doi: 10.1109/LED.2009.2038177
Cammaerts, M. C., and Cammaerts, R. (1998). Marking of nest entrance vicinity in the ant Pheidole pallidula (Formicidae, Myrmicinae). Behav. Process. 42, 19–31. doi: 10.1016/S0376-6357(97)00058-52
Cammaerts, M. C., Evershed, R. P., and Morgan, E. D. (1981). Comparative study of the mandibular gland secretion of four species of Myrmica ants. J. Insect Physiol. 27, 225–231. doi: 10.1016/0022-1910(81)90055-X
Cammaerts, R., Detrain, C., and Cammaerts, M. (1990). Host trail following by the myrmecophilous beetle Edaphopaussus favieri (Fairmaire) (Carabidae: Paussinae). Insect. Soc. 37, 200–211. doi: 10.1007/bf02224048
Cárdenas, M., Jiroš, P., and Pekár, S. (2012). Selective olfactory attention of a specialised predator to intraspecific chemical signals of its prey. Naturwissenschaften 99, 597–605. doi: 10.1007/s00114-012-0938-939
Carey, B., Visscher, K., and Heraty, J. (2012). Nectary use for gaining access to an ant host by the parasitoid Orasema simulatrix (Hymenoptera, Eucharitidae). J. Hymenopt. Res. 27, 47–65. doi: 10.3897/jhr.27.3067
Casacci, L. P., Thomas, J. A., Sala, M., Treanor, D., Bonelli, S., Balletto, E., et al. (2013). Ant pupae employ acoustics to communicate social status in their colony’s hierarchy. Curr. Biol. 23, 323–327. doi: 10.1016/j.cub.2013.01.010
Cazier, M. A., and Mortenson, M. A. (1965). Bionomical observations on myrmecophilous beetles of the genus Cremastocheilus. J. Kansas Entomol. Soc. 38, 19–44.
Chen, L., and Fadamiro, H. Y. (2018). Pseudacteon phorid flies: host specificity and impacts on Solenopsis fire ants. Annu. Rev. Entomol. 63, 47–67. doi: 10.1146/annurev-ento-020117-043049
Chung, H., and Carroll, S. B. (2015). Wax, sex and the origin of species: dual roles of insect cuticular hydrocarbons in adaptation and mating. Bioessays 37, 822–830. doi: 10.1002/bies.201500014
Clark, R. J., Jackson, R. R., and Cutler, B. (2000). Chemical cues from ants influence predatory behavior in Habrocestum pulex, an ant-eating jumping spider (Araneae, Salticidae). J. Arachnol. 28, 309–318. doi: 10.1636/0161-8202(2000)028
Clouse, R. M., Janda, M., Blanchard, B., Sharma, P., Hoffmann, B. D., Andersen, A. N., et al. (2015). Molecular phylogeny of Indo-Pacific carpenter ants (Hymenoptera: Formicidae, Camponotus) reveals waves of dispersal and colonization from diverse source areas. Cladistics 31, 424–437. doi: 10.1111/cla.12099
Combes, C. (2001). Parasitism: The Ecology and Evolution of Intimate Interactions. Chicago: The University of Chicago Press.
Cross, J. H., West, J. R., Silverstein, R. M., Jutsum, A. R., and Cherrett, J. M. (1982). Trail pheromone of the leaf-cutting ant, Acromyrmex octospinosus (Reich), (Formicidae: Myrmicinae). J. Chem. Ecol. 8, 1119–1124. doi: 10.1007/BF00986982
Czaczkes, T. J., Grüter, C., and Ratnieks, F. L. W. (2015). Trail pheromones: an integrative view of their role in social insect colony organization. Annu. Rev. Entomol. 60, 581–599. doi: 10.1146/annurev-ento-010814-020627
Das, G. M. (1963). Preliminary studies on the biology of Orasema assectator Kerrich (Hym., Eucharitidae), parasitic on Pheidole and causing damage to leaves of tea in Assam. Bull. Entomol. Res. 54, 373–378. doi: 10.1017/S0007485300048884
Davidson, D. W., Lessard, J. P., Bernau, C. R., and Cook, S. C. (2007). The tropical ant mosaic in a primary Bornean rain forest. Biotropica 39, 468–475. doi: 10.1111/j.1744-7429.2007.00304.x
de Bekker, C., Will, I., Das, B., and Adams, R. M. M. (2018). The ants (Hymenoptera: Formicidae) and their parasites: effects of parasitic manipulations and host responses on ant behavioral ecology. Myrmecol. News 28, 1–24. doi: 10.25849/myrmecol.news
Dejean, A. (1996). Trail sharing in African arboreal ants (Hymenoptera: Formicidae). Sociobiology 27, 1–10.
Dejean, A., and Beugnon, G. (1996). Host-ant trail following by myrmecophilous larvae of Liphyrinae (Lepidoptera. Lycaenidae). Oecologia 106, 57–62. doi: 10.1007/BF00334407
D’Ettorre, P., and Heinze, J. (2001). Sociobiology of slave-making ants. Acta Ethol. 3, 67–82. doi: 10.1007/s102110100038
Dietemann, V., Peeters, C., Liebig, J., Thivet, V., and Hölldobler, B. (2003). Cuticular hydrocarbons mediate discrimination of reproductives and nonreproductives in the ant Myrmecia gulosa. Proc. Natl. Acad. Sci. U.S.A. 100, 10341–10346. doi: 10.1073/pnas.1834281100
Dijkstra, M. B., and Boomsma, J. J. (2003). Gnamptogenys hartmani wheeler (Ponerinae: Ectatommini): an agro-predator of Trachymyrmex and Sericomyrmex fungus-growing ants. Naturwissenschaften 90, 568–571. doi: 10.1007/s00114-003-0478-474
Disney, R. H., Elizalde, L., and Folgarait, P. (2008). New species and records of scuttle flies (Diptera: Phoridae) associated with leaf-cutter ants and army ants (Hymenoptera: Formicidae) in Argentina. Sociobiology 51, 95–116.
Disney, R. H. L., Elizalde, L., and Folgarait, P. J. (2006). New species and revision of Myrmosicarius (Diptera: Phoridae) that parasitize leaf-cutter ants (Hymenoptera: Formicidae). Sociobiology 47, 771–809. doi: 10.3233/978-1-61499-304-9-120
Dodd, F. P. (1912). Some remarkable ant-friend lepidoptera of queensland. Trans. Ent. Soc. L. 10, 577–590. doi: 10.1111/j.1365-2311.1912.tb02198.x
Dornhaus, A., Powell, S., and Bengston, S. (2012). Group size and its effects on collective organization. Annu. Rev. Entomol. 57, 123–141. doi: 10.1146/annurev-ento-120710-100604
Durán, J. M. G., and van Achterberg, C. (2011). Oviposition behaviour of four ant parasitoids (Hymenoptera, Braconidae, Euphorinae, Neoneurini and Ichneumonidae, Hybrizontinae), with the description of three new european species. Zookeys 125, 59–106. doi: 10.3897/zookeys.125.1754
Economo, E. P., Narula, N., Friedman, N. R., Weiser, M. D., and Guénard, B. (2018). Macroecology and macroevolution of the latitudinal diversity gradient in ants. Nat. Commun. 9, 1–8. doi: 10.1038/s41467-018-04218-4214
Elgar, M. A., Nash, D. R., and Pierce, N. E. (2016). Eavesdropping on cooperative communication within an ant-butterfly mutualism. Sci. Nat. 103:84. doi: 10.1007/s00114-016-1409-1405
Elgert, B., and Rosengren, R. (1977). The guest ant Formicoxenus nitidulus follows the scent trail of its wood ant host (Hymenoptera: Formicidae). Memo. Soc. Pro Fauna Flora Fenn. 53, 35–38.
Elizalde, L., and Folgarait, P. J. (2011). Biological attributes of argentinian phorid parasitoids (Insecta: Diptera: Phoridae) of leaf-cutting ants, Acromyrmex and Atta. J. Nat. Hist. 45, 2701–2723. doi: 10.1080/00222933.2011.602478
Elizalde, L., and Folgarait, P. J. (2012). Behavioral strategies of phorid parasitoids and responses of their hosts, the leaf-cutting ants. J. Insect Sci. 12, 1–26. doi: 10.1673/031.012.13501
Elmes, G. W., Barr, B., Thomas, J. A., and Clarke, R. T. (1999). Extreme host specificity by Microdon mutabilis (Diptera: Syrphidae), a social parasite of ants. Proc. R. Soc. B Biol. Sci. 266, 447–453. doi: 10.1098/rspb.1999.0658
Erthal, M., and Tonhasca, A. (2001). Attacobius attarum spiders (Corinnidae): myrmecophilous predators of immature forms of the leaf-cutting ant Atta sexdens (Formicidae). Biotropica 33, 374–376. doi: 10.1021/ac60044a011
Evershed, R. P., and Morgan, E. D. (1981). Chemical investigations of the Dufour gland contents of attine ants. Insect. Biochem. 11, 343–351. doi: 10.1016/0020-1790(81)90013-90015
Farder-Gomes, C. F., Oliveira, M. A., Gonçalves, P. L., Gontijo, L. M., Zanuncio, J. C., Bragança, M. A. L., et al. (2017). Reproductive ecology of phorid parasitoids in relation to the head size of leaf-cutting ants Atta sexdens. Bull. Entomol. Res. 107, 487–492. doi: 10.1017/S0007485316001073
Feener, D. H. (1988). Effects of parasites on foraging and defense behavior of a termitophagous ant, Pheidole titanis wheeler (Hymenoptera: Formicidae). Behav. Ecol. Sociobiol. 22, 421–427. doi: 10.1007/BF00294980
Feener, D. H., and Brown, B. V. (1992). Reduced foraging of Solenopsis geminata (Hymenoptera: Formicidae) in the presence of parasitic Pseudacteon spp (Diptera: Phoridae). Ann. Entomol. Soc. Am. 85, 80–84. doi: 10.1093/aesa/85.1.80
Feener, D. H., and Brown, B. V. (1997). Diptera as parasitoids. Annu. Rev. Entomol. 42, 73–97. doi: 10.1146/annurev.ento.42.1.73
Feener, D. H., Jacobs, L. F., and Schmidt, J. O. (1996). Specialized parasitoid attracted to a pheromone of ants. Anim. Behav. 51, 61–66. doi: 10.1006/anbe.1996.0005
Fernández-Marín, H., Zimmerman, J. K., and Wcislo, W. T. (2006). Acanthopria and Mimopriella parasitoid wasps (Diapriidae) attack Cyphomyrmex fungus-growing ants (Formicidae, Attini). Naturwissenschaften 93, 17–21. doi: 10.1007/s00114-005-0048-z
Folgarait, P. J. (2013). Leaf-cutter ant parasitoids: current knowledge. Psyche 2013:539780. doi: 10.1155/2013/539780
Forti, L. C., de Andrade, A. P. P., Camargo, R., da, S., Caldato, N., and Moreira, A. A. (2017). Discovering the giant nest architecture of grass-cutting ants, Atta capiguara (Hymenoptera, Formicidae). Insects 8:E39. doi: 10.3390/insects8020039
Fox, E. G. P. (2018). Chemical blueprints to identifying fire ants: overview on venom alkaloids. bioRxiv [Preprint]. doi: 10.1101/407775
Fox, E. G. P., Wu, X., Wang, L., Chen, L., Lu, Y. Y., and Xu, Y. (2019). Queen venom isosolenopsin a delivers rapid incapacitation of fire ant competitors. Toxicon 158, 77–83. doi: 10.1016/j.toxicon.2018.11.428
Gallego-Ropero, M. C., and Feitosa, R. M. (2014). Evidences of batesian mimicry and parabiosis in ants of the Brazilian Savanna. Sociobiology 61, 281–285.
Gao, J., Barzel, B., and Barabási, A. L. (2016). Universal resilience patterns in complex networks. Nature 530, 307–312. doi: 10.1038/nature16948
Garnett, W. B., Akre, R. D., and Sehlke, G. (1985). Cocoon mimicry and predation by myrmecophilous diptera (Diptera: Syrphidae). Florida Entomol. 68:615. doi: 10.2307/3494863
Geiselhardt, S. F., Peschke, K., and Nagel, P. (2007). A review of myrmecophily in ant nest beetles (Coleoptera: Carabidae: Paussinae): linking early observations with recent findings. Naturwissenschaften 94, 871–894. doi: 10.1007/s00114-007-0271-x
Gobin, B., Peeters, C., Billen, J., and Morgan, E. D. (1998). Interspecific trail following and commensalism between the ponerine ant Gnamptogenys menadensis and the formicine ant Polyrhachis rufipes. J. Insect Behav. 11, 361–369.
Gordon, D. M., and Mehdiabadi, N. J. (1999). Encounter rate and task allocation in harvester ants. Behav. Ecol. Sociobiol. 45, 370–377. doi: 10.1007/s002650050573
Gotwald, W. H. J. (1995). Army Ants: The Biology of Social Predation. New York, NY: Cornell University Press.
Greenberg, L., Kabashima, J. N., Allison, C. J., Rust, M. K., Klotz, J. H., Hurvois, J., et al. (2008). Lethality of red imported fire ant venom to Argentine ants and other ant species. Ann. Entomol. Soc. Am. 101, 1162–1168. doi: 10.1603/0013-8746-101.6.1162
Hamel, J. A., and Cocroft, R. B. (2019). Maternal vibrational signals reduce the risk of attracting eavesdropping predators. Front. Ecol. Evol. 7:204. doi: 10.3389/fevo.2019.00204
Hamilton, N., Jones, T. H., Shik, J. Z., Wall, B., Schultz, T. R., Blair, H. A., et al. (2018). Context is everything: mapping Cyphomyrmex-derived compounds to the fungus-growing ant phylogeny. Chemoecology 28, 137–144. doi: 10.1007/s00049-018-0265-265
Henderson, G., and Akre, R. D. (1986). Biology of the myrmecophilous cricket, Myrmecophila manni (Orthoptera: Gryllidae). J. Kansas Entomol. Soc. 59, 454–467. doi: 10.11646/zootaxa.4533.1.1
Henderson, G., and Jeanne, R. L. (1990). A myrmecophilous histerid revisited (Coleoptera: Histeridae). Coleopt. Bull. 44, 442–444.
Henne, D. C., and Johnson, S. J. (2007). Zombie fire ant workers: behavior controlled by decapitating fly parasitoids. Insect. Soc. 54, 150–153. doi: 10.1007/s00040-007-0924-y
Henning, S. F. (1983). Chemical communication between lycaenid larvae (Lepidoptera: Lycaenidae) and ants (Hymenoptera: Formicidae). J. Entomol. Soc. South. Afr. 46, 341–366.
Heraty, J. M. (1994). “Biology and importance of two eucharitid parasites of Wasmannia and Solenopsis,” in Exotic Ants: Biology, Impact and Control of Introduced Species, ed. D. F. Williams (Boulder, Co: Williams D. F. Westview Press), 104–120.
Herreid, J. S., and Heraty, J. M. (2017). Hitchhikers at the dinner table: a revisionary study of a group of ant parasitoids (Hymenoptera: Eucharitidae) specializing in the use of extrafloral nectaries for host access. Syst. Entomol. 42, 204–229. doi: 10.1111/syen.12206
Hertel, F., and Colli, G. R. (1998). The use of leaf-cutter ants, Atta laevigata (Smith) (Hymenoptera: Formicidae), as a substrate for oviposition by the dung beetle Canthon virens Mannerheim (Coleoptera: Scarabaeidae) in Central Brazil. Coleopt. Bull. 52, 105–108.
Heyman, Y., Shental, N., Brandis, A., Hefetz, A., and Feinerman, O. (2017). Ants regulate colony spatial organization using multiple chemical road-signs. Nat. Commun. 8:15414. doi: 10.1038/ncomms15414
Hogan, C. T., Jones, T. H., Zhukova, M., Sosa-Calvo, J., and Adams, R. M. M. (2017). Novel mandibular gland volatiles from Apterostigma ants. Biochem. Syst. Ecol. 72, 56–62. doi: 10.1016/j.bse.2017.04.005
Hölldobler, B. (1967). Zur physiologie der gast-wirt-beziehungen (Myrmecophilie) bei ameisen. Z. Vgl. Physiol. 56, 1–21. doi: 10.1007/BF00333561
Hölldobler, B., and Kwapich, C. L. (2017). Amphotis marginata (Coleoptera: Nitidulidae) a highwayman of the ant Lasius fuliginosus. PLoS One 12:e0180847. doi: 10.1371/journal.pone.0180847
Hölldobler, B., and Wilson, E. O. (1986). Nest area exploration and recognition in leafcutter ants (Atta cephalotes). J. Insect Physiol. 32, 143–150. doi: 10.1016/0022-1910(86)90133-90132
Hölldobler, B., and Wilson, E. O. (1990). The Ants. Cambridge: Belknap Press of Harvard University Press.
Hölldobler, B., and Wilson, E. O. (2011). The Leafcutter Ants. Civilization by Instinct. New York, NY: W.W. Norton & Company, Inc.
Howard, R. W., and Blomquist, G. J. (2005). Ecological, behavioral, and biochemical aspects of insect hydrocarbons. Annu. Rev. Entomol. 50, 371–393. doi: 10.1146/annurev.ento.50.071803.130359
Hsieh, H.-Y., Liere, H., Soto, E. J., and Perfecto, I. (2012). Cascading trait-mediated interactions induced by ant pheromones. Ecol. Evol. 2, 2181–2191. doi: 10.1002/ece3.322
Hsieh, H. Y., and Perfecto, I. (2012). Trait-mediated indirect effects of phorid flies on ants. Psyche 2012, 8–11. doi: 10.1155/2012/380474
Hughes, D. P., Pierce, N. E., and Boomsma, J. J. (2008). Social insect symbionts: Evolution in homeostatic fortresses. Trends Ecol. Evol. 23, 672–677. doi: 10.1016/j.tree.2008.07.011
Ito, F., Hashim, R., Huei, Y. S., Kaufmann, E., Akino, T., and Billen, J. (2004). Spectacular Batesian mimicry in ants. Naturwissenschaften 91, 481–484. doi: 10.1007/s00114-004-0559-z
Ivens, A. B. F., von Beeren, C., Blüthgen, N., and Kronauer, D. J. C. (2016). Studying the complex communities of ants and their symbionts using ecological network analysis. Annu. Rev. Entomol. 61, 353–371. doi: 10.1146/annurev-ento-010715-023719
Jackson, B. D., and Morgan, E. D. (1993). Insect chemical communication: pheromones and exocrine glands of ants. Chemoecology 4, 125–144. doi: 10.1007/BF01256548
Jackson, D. E., Martin, S. J., Ratnieks, F. L. W., and Holcombe, M. (2007). Spatial and temporal variation in pheromone composition of ant foraging trails. Behav. Ecol. 18, 444–450. doi: 10.1093/beheco/arl104
Jackson, R. R., Salm, K., and Pollard, S. D. (2008). Snatching prey from the mandibles of ants, a feeding tactic adopted by East African jumping spiders. J. Arachnol. 36, 609–612.
Johnson, A., Revis, O., and Johnson, J. C. (2011). Chemical prey cues influence the urban microhabitat preferences of Western black widow spiders, Latrodectus hesperus. J. Arachnol. 39, 449–453. doi: 10.1636/hi11-19.1
Johnson, C. A., Topoff, H., Vander Meer, R. K., and Lavine, B. (2002). Host queen killing by a slave-maker ant queen: when is a host queen worth attacking? Anim. Behav. 64, 807–815. doi: 10.1006/anbe.2002.1971
Johnson, R. A., Parker, J. D., and Rissing, S. W. (1996). Rediscovery of the workerless inquiline ant Pogonomyrmex colei and additional notes on natural history (Hymenoptera: Formicidae). Insect. Soc. 43, 69–76. doi: 10.1007/BF01253957
Kaspari, M. (1996). Testing resource-based models of patchiness in four neotropical litter ant assemblages. Oikos 76:443. doi: 10.2307/3546338
Kaudewitz, F. (1955). Zum Gastverhältnis zwischen Crematogaster scutellaris mit Camponotus lateralis bicolor. Biol. Zent. Bl. 74, 69–87.
Keller, L., and Genoud, M. (1997). Extraordinary lifespans in ants: a test of evolutionary theories of ageing. Nature 389, 958–960. doi: 10.1038/40130
Kistner, D. H. (1982). “The social insects’ bestiary,” in Social Insects, Vol. 3, ed. H. R. Hermann (London: Academic Press), doi: 10.1073/pnas.96.15.8657
Komatsu, T. (2016). Diet and predatory behavior of the Asian ant-eating spider, Asceua (formerly Doosia) japonica (Araneae: Zodariidae). Springerplus 5, 1–7. doi: 10.1186/s40064-016-2234-1
Komatsu, T., Maruyama, M., and Itino, T. (2013). Nonintegrated host association of Myrmecophilus tetramorii, a specialist myrmecophilous ant cricket (Orthoptera: Myrmecophilidae). Psyche 2013: 568536. doi: 10.1155/2013/568536
Kost, C., De Oliveira, E. G., Knoch, T. A., and Wirth, R. (2005). Spatio-temporal permanence and plasticity of foraging trails in young and mature leaf-cutting ant colonies (Atta spp.). J. Trop. Ecol. 21, 677–688. doi: 10.1017/S0266467405002592
Lachaud, J. P., and Pérez-Lachaud, G. (2012). Diversity of species and behavior of hymenopteran parasitoids of ants: a review. Psyche 2012:24. doi: 10.1155/2012/134746
Lai, L. C., Chang, Y. Y., Hua, K. H., Wu, W. J., and Huang, R. N. (2010). Comparative toxicity of three fire ant (Hymenoptera: Formicidae) venoms to Spodoptera litura larvae. Sociobiology 56, 653–663.
Lenoir, A., Chalon, Q., Carvajal, A., Ruel, C., Barroso, A., Lackner, T., et al. (2012). Chemical integration of myrmecophilous guests in Aphaenogaster ant nests. Psyche 2012:12. doi: 10.1155/2012/840860
Lenoir, A., D’Ettorre, P., Errard, C., and Hefetz, A. (2001). Chemical ecology and social parasitism in ants. Annu. Rev. Entomol. 46, 573–599.
Leonhardt, S. D., Menzel, F., Nehring, V., and Schmitt, T. (2016). Ecology and evolution of communication in social insects. Cell 164, 1277–1287. doi: 10.1016/j.cell.2016.01.035
Loiácono, M., Margaría, C., and Aquino, D. (2013a). Diapriinae wasps (Hymenoptera: Diaprioidea: Diapriidae) associated with ants (Hymenoptera: Formicidae) in Argentina. Psyche 2013:320590. doi: 10.1155/2013/320590
Loiácono, M., Margaría, C., Moreira, D. D. O., and Aquino, D. (2013b). A new species of Szelenyiopria Fabritius (Hymenoptera: Diapriidae), larval parasitoid of Acromyrmex subterraneus subterraneus (Forel) (Hymenoptera: Formicidae) from Brazil. Zootaxa 3646, 228–234. doi: 10.11646/zootaxa.3646.3.2
Loiácono, M. S., Margaria, C. B., Quiran, E. M., and Corro Molas, B. M. (2000). Diápridos (Hymenoptera) parasitoides de larvas de la hormiga cortadora Acromyrmex lobicornis (Hymenoptera: Formicidae) en la Argentina. Rev. Soc. Ent. Argentina 59, 7–15.
Longino, J. T. (2007). A taxonomic review of the genus azteca. Zootaxa 1491, 1–63. doi: 10.11646/zootaxa.3734.2.1
Martin, S., and Drijfhout, F. (2009). A review of ant cuticular hydrocarbons. J. Chem. Ecol. 35, 1151–1161. doi: 10.1007/s10886-009-9695-9694
Maschwitz, U., Nässig, W., Dumpert, K., and Fiedler, K. (1988). Larval carnivory and myrmecoxeny, and imaginal myrmecophily in Miletine Lycaenids (Lepidoptera, Lycaenidae) on the Malay Peninsula. Trans. Lepidopterol. Soc. Japan 39, 167–181. doi: 10.18984/lepid.39.3-167
Maschwitz, U., Weissflog, A., Seebauer, S., and Disney, R. H. L. (2008). Studies on European ant decapitating flies (Diptera: Phoridae): I. releasers and phenology of parasitism of Pseudacteon formicarum. Sociobiology 51, 127–140.
Masner, L. (1959). A revision of Ecitophilous diapriid-genus mimopria holmgren (Hym., Proctotrupoidea). Insects Soc. 6, 361–367. doi: 10.1007/bf02225780
Masner, L. (1977). A new genus of ecitophilous diapriid wasps from Arizona (Hymenoptera: Proctotrupoidea: Diapriidae). Can. Entomol. 109, 33–36. doi: 10.4039/Ent10933-1
Masner, L., and García, J. R. (2002). The genera of diapriinae (Hymenoptera: Diapriidae) in the New World. Bull. Am. Museum Nat. Hist. 268, 1–138. doi: 10.1206/0003-00902002268
Mathis, K. A., and Philpott, S. M. (2012). Current understanding and future prospects of host selection, acceptance, discrimination, and regulation of phorid fly parasitoids that attack ants. Psyche 2012:895424. doi: 10.1155/2012/895424
Mathis, K. A., Philpott, S. M., and Moreira, R. F. (2011). Parasite lost: chemical and visual cues used by Pseudacteon in search of Azteca instabilis. J. Insect Behav. 24, 186–199. doi: 10.1007/s10905-010-9247-9243
Mathis, K. A., and Tsutsui, N. D. (2016). Cuticular hydrocarbon cues are used for host acceptance by Pseudacteon spp. phorid flies that attack Azteca sericeasur ants. J. Chem. Ecol. 42, 286–293. doi: 10.1007/s10886-016-0694-y
Maurizi, E., Fattorini, S., Moore, W., and Giulio, A. (2012). Behavior of Paussus favieri (Coleoptera, Carabidae, Paussini): a myrmecophilous beetle associated with Pheidole pallidula (Hymenoptera, Formicidae). Psyche 2012:940315. doi: 10.1155/2012/940315
Maynard-Smith, J., and Harper, D. (2003). Animal Signals. Oxford, UK: Oxford University Press, doi: 10.1016/j.cub.2013.07.070
McCann, S., Moeri, O., Jones, T., Scott, C., Khaskin, G., Gries, R., et al. (2013). Strike fast, strike hard: the red-throated caracara exploits absconding behavior of social wasps during nest predation. PLoS One 8:84114. doi: 10.1371/journal.pone.0084114
Menzel, F. (2009). Mechanisms and Adaptive Significance of Interspecific Associations Between Tropical Ant Species. Würzburg: University Library Würzburg.
Menzel, F., Blaimer, B. B., and Schmitt, T. (2017). How do cuticular hydrocarbons evolve? Physiological constraints and climatic and biotic selection pressures act on a complex functional trait. Proc. R. Soc. B Biol. Sci. 284:20161727. doi: 10.1098/rspb.2016.1727
Menzel, F., Pokorny, T., Blüthgen, N., and Schmitt, T. (2010). Trail-sharing among tropical ants: interspecific use of trail pheromones? Ecol. Entomol. 35, 495–503. doi: 10.1111/j.1365-2311.2010.01206.x
Mestre, L., Bucher, R., and Entling, M. H. (2014). Trait-mediated effects between predators: ant chemical cues induce spider dispersal. J. Zool. 293, 119–125. doi: 10.1111/jzo.12127
Milton, E. J., and Athayde, T. J. (2000). Biology and oviposition behavior of the phorid Apocephalus attophilus and the response of its host, the leaf-cutting ant Atta laevigata. Entomol. Exp. Appl. 95, 71–75. doi: 10.1046/j.1570-7458.2000.00643.x
Moore, W., and Robertson, J. A. (2014). Explosive adaptive radiation and extreme phenotypic diversity within ant-nest beetles. Curr. Biol. 24, 2435–2439. doi: 10.1016/j.cub.2014.09.022
Morehead, S. A., and Feener, D. H. (2000a). An experimental test of potential host range in the ant parasitoid Apocephalus paraponerae. Ecol. Entomol. 25, 332–340. doi: 10.1046/j.1365-2311.2000.00261.x
Morehead, S. A., and Feener, D. H. (2000b). Visual and chemical cues used in host location and acceptance by a Dipteran parasitoid. J. Insect Behav. 13, 613–625. doi: 10.1023/A:1007875921705
Morel, L., and Vander Meer, R. K. (1988). Do ant brood pheromones exist? Ann. Entomol. Soc. Am. 81, 705–710. doi: 10.1093/aesa/81.5.705
Morgan, D. E. (2009). Trail pheromones of ants. Physiol. Entomol. 34, 1–17. doi: 10.1111/j.1365-3032.2008.00658.x
Morgan, E. D. (2008). Chemical sorcery for sociality: exocrine secretions of ants (Hymenoptera: Formicidae). Myrmecol. News 11, 79–90.
Morgan, E. D., Jackson, B. D., Ollett, D. G., and Sales, G. W. (1990). Trail pheromone of the ant Tetramorium impurum and model compounds: structure-activity comparisons. J. Chem. Ecol. 77, 3493–3510. doi: 10.1007/BF01131231
Morgan, E. D., Keegans, S. J., Tits, J., Wenseleers, T., and Billen, J. (2006). Preferences and differences in the trail pheromone of the leaf-cutting ant Atta sexdens sexdens (Hymenoptera: Formicidae). Eur. J. Entomol. 103, 553–558. doi: 10.14411/eje.2006.075
Morrison, L. W. (2012). Biological control of Solenopsis fire ants by Pseudacteon parasitoids: theory and practice. Psyche 2012: 424817. doi: 10.1155/2012/424817
Morrison, L. W., and Gilbert, L. E. (1999). Host specificity in two additional Pseudactron spp (Diptera: Phoridae), parasitioids of Solenopsis fire ants (Hymenoptera: Formicidae). Florida Entomol. 82, 1995–2000.
Morrison, L. W., and King, J. R. (2004). Host location behavior in a parasitoid of imported fire ants. J. Insect Behav. 17, 367–383. doi: 10.1023/B:JOIR.0000031537.41582.d1
Moser, J. C. (1964). Inquiline roach responds to trail-marking substance of leaf-cutting ants. Science 143, 1048–1049. doi: 10.1126/science.143.3610.1048
Moser, J. C. (1967). Mating activities of Atta texana. Insect. Soc. 14, 295–312. doi: 10.1007/bf02252831
Moser, J. C. (2006). Complete excavation and mapping of a Texas leafcutting ant nest. Ann. Entomol. Soc. Am. 99, 891–897.
Murray, E. A., Carmichael, A. E., and Heraty, J. M. (2013). Ancient host shifts followed by host conservatism in a group of ant parasitoids. Proc. R. Soc. B 280:20130495. doi: 10.1098/rspb.2013.0495
Nascimento, R., Billen, J., Sant’Ana, A., Morgan, E. D., and Harada, A. Y. (1998). Pygidial gland of Azteca NR bicolor and Azteca charifex: morphology and chemical identification of volatile components. J. Chem. Ecol. 24, 1629–1637. doi: 10.1023/A:1020864427854
Navarrete-Heredia, J. L. (2001). Beetles associated with Atta and Acromyrmex ants (Hymenoptera: Formicidae: Attini). Am. Entomol. Soc. 127, 381–429.
Nehring, V., Dani, F. R., Calamai, L., Turillazzi, S., Bohn, H., Klass, K. D., et al. (2016). Chemical disguise of myrmecophilous cockroaches and its implications for understanding nestmate recognition mechanisms in leaf-cutting ants. BMC Ecol. 16:35. doi: 10.1186/s12898-016-0089-85
Nettel-Hernanz, A., Lachaud, J. P., Fresneau, D., López-Muñoz, R. A., and Poteaux, C. (2015). Biogeography, cryptic diversity, and queen dimorphism evolution of the Neotropical ant genus Ectatomma Smith, 1958 (Formicidae, Ectatomminae). Org. Divers. Evol. 15, 543–553. doi: 10.1007/s13127-015-0215-219
Ngumbi, E., and Fadamiro, H. (2015). Comparative responses of four Pseudacteon phorid fly species to host fire ant alarm pheromone and analogs. Chemoecology 25, 85–92. doi: 10.1007/s00049-014-0178-x
Norman, V. C., Butterfield, T., Drijfhout, F., Tasman, K., and Hughes, W. O. H. (2017). Alarm pheromone composition and behavioral activity in fungus-growing ants. J. Chem. Ecol. 43, 225–235. doi: 10.1007/s10886-017-0821-824
Oldham, N. J., Morgan, E. D., Gobin, B., and Billen, J. (1994). First identification of a trail pheromone of an army ant (Aenictus species). Experientia 50, 763–765. doi: 10.1007/BF01919378
Orr, M. R. (1992). Parasitic flies (Diptera: Phoridae) influence foraging rhythms and caste division of labor in the leaf-cutter ant, Atta cephalotes. Behav. Ecol. Sociobiol. 30, 395–402.
Orr, M. R., De Camargo, R. X., and Benson, W. W. (2003). Interactions between ant species increase arrival rates of an ant parasitoid. Anim. Behav. 65, 1187–1193. doi: 10.1006/anbe.2003.2176
O’Shea-Wheller, T. A., Sendova-Franks, A. B., and Franks, N. R. (2015). Differentiated anti-predation responses in a superorganism. PLoS One 10:141012. doi: 10.1371/journal.pone.0141012
Otte, D. (1974). Effects and functions in the evolution of signaling systems. Annu. Rev. Ecol. Syst. 5, 385–417. doi: 10.1146/annurev.es.05.110174.002125
Parry, K., and Morgan, E. D. (1979). Pheromones of ants: a review. Physiol. Entomol. 4, 161–189. doi: 10.1111/j.1365-3032.1979.tb00193.x
Patrock, R. J. W., Porter, S. D., Gilbert, L. E., and Folgarait, P. J. (2009). Distributional patterns of Pseudacteon associated with the Solenopsis saevissima complex in South America. J. Insect Sci. 9, 1–17. doi: 10.1673/031.009.6001
Peake, T. M., Terry, A. M. R., McGregor, P. K., and Dabelsteen, T. (2001). Male great tits eavesdrop on simulated male-to-male vocal interactions. Proc. R. Soc. B Biol. Sci. 268, 1183–1187. doi: 10.1098/rspb.2001.1648
Penfold, S., Dayananda, B., and Webb, J. K. (2017). Chemical cues influence retreat-site selection by flat rock spiders. Behaviour 154, 149–161. doi: 10.1163/1568539X-00003415
Pérez-Lachaud, G., Bartolo-Reyes, J. C., Quiroa-Montalván, C. M., Cruz-López, L., Lenoir, A., and Lachaud, J. P. (2015). How to escape from the host nest: imperfect chemical mimicry in eucharitid parasitoids and exploitation of the ants’ hygienic behavior. J. Insect Physiol. 75, 63–72. doi: 10.1016/j.jinsphys.2015.03.003
Pérez-Lachaud, G., Jahyny, B. J. B., Ståhls, G., Rotheray, G., Delabie, J. H. C., and Lachaud, J. P. (2017). Rediscovery and reclassification of the dipteran taxon Nothomicrodon wheeler, an exclusive endoparasitoid of gyne ant larvae. Sci. Rep. 7:45530. doi: 10.1038/srep45530
Pérez-Lachaud, G., Jervis, M. A., Reemer, M., and Lachaud, J. (2014). An unusual, but not unexpected, evolutionary step taken by syrphid flies: the first record of true primary parasitoidism of ants by Microdontinae. Biol. J. Linn. Soc. 111, 462–472. doi: 10.1111/bij.12220
Pérez-Lachaud, G., and Lachaud, J. P. (2017). Hidden biodiversity in entomological collections: the overlooked co-occurrence of dipteran and hymenopteran ant parasitoids in stored biological material. PLoS One 12:184614. doi: 10.1371/journal.pone.0184614
Pérez-Ortega, B., Fernández-Marín, H., Loiácono, M., Galgani, P., and Wcislo, W. (2010). Biological notes on a fungus-growing ant, Trachymyrmex cf. zeteki (Hymenoptera, Formicidae, Attini) attacked by a diverse community of parasitoid wasps (Hymenoptera, Diapriidae). Insect. Soc. 57, 317–322. doi: 10.1007/s00040-010-0086-81
Phillips, Z. I., Zhang, M. M., and Mueller, U. G. (2017). Dispersal of Attaphila fungicola, a symbiotic cockroach of leaf-cutter ants. Insect. Soc. 64, 277–284. doi: 10.1007/s00040-016-0535-536
Philpott, S. M., Maldonado, J., Vandermeer, J., and Perfecto, I. (2004). Taking trophic cascades up a level: behaviorally-modified effects of phorid flies on ants and ant prey in coffee agroecosystems. Oikos 105, 141–147. doi: 10.1111/j.0030-1299.2004.12889.x
Philpott, S. M., Perfecto, I., Vandermeer, J., and Uno, S. (2009). Spatial scale and density dependence in a host parasitoid system: an arboreal ant, Azteca instabilis, and its Pseudacteon phorid parasitoid. Environ. Entomol. 38, 790–796. doi: 10.1603/022.038.0331
Pierce, N., Braby, M., Heath, A., Lohman, D., Mathew, J., Rand, D., et al. (2002). The ecology and evolution of ant association in the Lycaenidae (Lepidoptera). Annu. Rev. Entomol. 47, 733–771. doi: 10.1080/01947641003598252
Porter, S. D. (1998). Biology and behavior of Pseudacteon decapitating flies (Diptera: Phoridae) that parasitize Solenopsis fire ants (Hymenoptera: Formicidae). Florida Entomol. 81, 292–309. doi: 10.2307/3495920
Poulin, R. (2007). Evolutionary Ecology of Parasites, 2nd Edn, Princeton, NJ: Princeton University Press.
Powell, S., Del-Claro, K., Feitosa, R. M., and Brandão, C. R. F. (2014). Mimicry and eavesdropping enable a new form of social parasitism in ants. Am. Nat. 184, 500–509. doi: 10.1086/677927
Rabeling, C., and Bacci, M. Jr. (2010). A new workerless inquiline in the Lower Attini (Hymenoptera: Formicidae), with a discussion of social parasitism in fungus-growing ants. Syst. Entomol. 35, 379–392. doi: 10.1111/j.1365-3113.2010.00533.x
Ramos-Lacau, L. S., Delabie, J. H. C., Bueno, O. C., Villemant, C., Prates Luz, H., Andrade Miranda, M. M., et al. (2007). Estratégia comportamental de Acanthopria Ashmead (Hymenoptera: Diapriidae), parasitóide de Cyphomyrmex transversus Emery (Hymenoptera: Formicidae). Biology 69, 451–454.
Rettenmeyer, C. W., and Akre, R. D. (1968). Ectosynibiosis between phorid flies and army ants. Ann. Entomol. Soc. Am. 61, 1317–1326. doi: 10.1093/aesa/61.5.1317
Rettenmeyer, C. W., Rettenmeyer, M. E., Joseph, J., and Berghoff, S. M. (2011). The largest animal association centered on one species: the army ant Eciton burchellii and its more than 300 associates. Insect. Soc. 58, 281–292. doi: 10.1007/s00040-010-0128-128
Robinson, E. J. H., Jackson, D. E., Holcombe, M., and Ratnieks, F. L. W. (2005). Insect communication: “no entry” signal in ant foraging. Nature 438:442. doi: 10.1038/438442a
Rodríguez, J., Montoya-Lerma, J., and Calle, Z. D. (2013). Primer registro de Attaphila fungicola (Blattaria: Polyphagidae) en nidos de Atta cephalotes (Hymenoptera: Myrmicinae) en Colombia. Boletín Científico. Cent. Museos. Mus. Hist. Nat. 17, 219–225.
Ruano, F., Sanllorente, O., Lenoir, A., and Tinaut, A. (2013). Rossomyrmex, the slave-maker ants from the arid steppe environments. Psyche A J. Entomol. 2013:541804. doi: 10.1155/2013/541804
Salkeld, D. J., Trivedi, M., and Schwarzkopf, L. (2008). Parasite loads are higher in the tropics: temperate to tropical variation in a single host-parasite system. Ecography 31, 538–544. doi: 10.1111/j.0906-7590.2008.05414.x
Salzemann, A., Nagnan, P., Tellier, F., and Jaffe, K. (1992). Leaf-cutting ant Atta laevigata (Formicidae: Attini) marks its territory with colony-specific dufour gland secretion. J. Chem. Ecol. 18, 183–196. doi: 10.1007/BF00993752
Schönrogge, K., Napper, E. K. V., Birkett, M. A., Woodcock, C. M., Pickett, J. A., Wadhams, L. J., et al. (2008). Host recognition by the specialist hoverfly Microdon mutabilis, a social parasite of the ant Formica lemani. J. Chem. Ecol. 34, 168–178. doi: 10.1007/s10886-007-9417-9418
Sharma, K., Vander Meer, R. K., and Fadamiro, H. Y. (2011). Phorid fly, Pseudacteon tricuspis, response to alkylpyrazine analogs of a fire ant, Solenopsis invicta, alarm pheromone. J. Insect Physiol. 57, 939–944. doi: 10.1016/j.jinsphys.2011.04.007
Sharma, K. R., and Fadamiro, H. Y. (2013). Fire ant alarm pheromone and venom alkaloids act in concert to attract parasitic phorid flies, Pseudacteon spp. J. Insect Physiol. 59, 1119–1124. doi: 10.1016/j.jinsphys.2013.08.010
Shoemaker, D. D., Ahrens, M. E., and Ross, K. G. (2006). Molecular phylogeny of fire ants of the Solenopsis saevissima species-group based on mtDNA sequences. Mol. Phylogenet. Evol. 38, 200–215. doi: 10.1016/j.ympev.2005.07.014
Silveira-Guido, A., Carbonell, J., and Crisci, C. (1973). Animals associated with the Solenopsis (Fire ants) complex, with special reference to Labauchena daguerrei. Proc. Tall Timbers Conf. Ecol. Anim. Control Habitat 4, 41–52.
Staverløkk, A., and Ødegaard, F. (2016). New records of parasitic hymenoptera associated with ants, including four new species to Norway. J. Entomol. 63, 188–196.
Steck, K. (2012). Just follow your nose: homing by olfactory cues in ants. Curr. Opin. Neurobiol. 22, 231–235. doi: 10.1016/j.conb.2011.10.011
Stevens, M. (2013). Sensory Ecology, Behaviour, and Evolution. Oxford: Oxford University Press, doi: 10.1017/CBO9781107415324.004
Stowe, M. K., Turlings, T. C., Loughrin, J. H., Lewis, W. J., and Tumlinson, J. H. (1995). The chemistry of eavesdropping, alarm, and deceit. Proc. Natl. Acad. Sci. U.S.A. 92, 23–28. doi: 10.1073/pnas.92.1.23
Suckling, D. M., Stringer, L. D., Bunn, B., El-Sayed, A. M., and Vander Meer, R. K. (2010). Trail pheromone disruption of red imported fire ant. J. Chem. Ecol. 36, 744–750. doi: 10.1007/s10886-010-9810-9816
Swain, R. B. (1980). Trophic competition among parabiotic ants. Insect. Soc. 27, 377–390. doi: 10.1007/BF02223730
Tonhasca, A. (1996). Interactions between a parasitic fly, Neodohrniphora declinata (Diptera: Phoridae), and its host, the leaf-cutting ant Atta sexdens rubropilosa (Hymenoptera: Formicidae). Ecotropica 2, 157–164.
Torchin, M. E., Miura, O., and Hechinger, R. F. (2015). Parasite species richness and intensity of interspecific interactions increase with latitude in two wide-ranging hosts. Ecology 96, 3033–3042. doi: 10.1890/15-0518.1
Torréns, J. (2013). A review of the biology of Eucharitidae (Hymenoptera: Chalcidoidea) from Argentina. Psyche 2013:926572. doi: 10.1155/2013/926572
Torres, J. A., Snelling, R. R., Blum, M. S., Flournoy, R. C., Jones, T. H., and Duffield, R. M. (2001). Mandibular gland chemistry of four Caribbean species of Camponotus (Hymenoptera: Formicidae). Biochem. Syst. Ecol. 29, 673–680. doi: 10.1016/S0305-1978(00)00107-101
Tumlinson, J., Moser, J., Silverstein, R. M., Brownlee, R. G., and Ruth, J. M. (1972). A volatile trail pheromone of the leaf-cutting ant, Atta texana. J. Insect Physiol. 18, 809–814. doi: 10.1016/0022-1910(72)90018-2
Uribe, S., Brown, B. V., Correa, G., and Ortiz, A. (2016). Phorids associated with nests of Atta cephalotes (Hymenoptera: Formicidae) in a forest and a plantation. Rev. Colomb. Entomol. 42, 48–53.
Vander Meer, R. K., Jouvenaz, D. P., and Wojcik, D. P. (1989). Chemical mimicry in a parasitoid (Hymenoptera: Eucharitidae) of fire ants (Hymenoptera: Formicidae). J. Chem. Ecol. 15, 2247–2261. doi: 10.1007/BF01014113
Vander Meer, R. K., Preston, C. A., and Choi, M.-Y. (2010). Isolation of a pyrazine alarm pheromone component from the fire ant, Solenopsis invicta. J. Chem. Ecol. 36, 163–170. doi: 10.1007/s10886-010-9743-9740
Vander Meer, R. K., and Wojcik, D. P. (1982). Chemical mimicry in the myrmecophilous beetle Myrmecaphodius excavaticollis. Science 218, 806–808. doi: 10.1126/science.218.4574.806
Vandermeer, J., Perfecto, I., and Philpott, S. M. (2008). Clusters of ant colonies and robust criticality in a tropical agroecosystem. Nature 451, 457–459. doi: 10.1038/nature06477
Varone, L., and Briano, J. (2009). Bionomics of Orasema simplex (Hymenoptera: Eucharitidae), a parasitoid of Solenopsis fire ants (Hymenoptera: Formicidae) in Argentina. Biol. Control 48, 204–209. doi: 10.1016/j.biocontrol.2008.10.003
Varone, L., Heraty, J. M., and Calcaterra, L. A. (2010). Distribution, abundance and persistence of species of Orasema (Hymenoptera: Eucharitidae) parasitic on fire ants in South America. Biol. Control 55, 72–78. doi: 10.1016/j.biocontrol.2010.06.017
Vet, L. E. M., and Dicke, M. (1992). Ecology of infochemical use by natural enemies in a tritrophic context. Annu. Rev. Entomol. 37, 141–172. doi: 10.1146/annurev.ento.37.1.141
Vinson, S. B. (1976). Host selection by insect parasitoids. Annu. Rev. Entomol. 21, 109–133. doi: 10.1146/annurev.en.21.010176.000545
von Beeren, C., Brückner, A., Maruyama, M., Burke, G., Wieschollek, J., and Kronauer, D. J. C. (2018). Chemical and behavioral integration of army ant-associated rove beetles - a comparison between specialists and generalists. Front. Zool. 15:8. doi: 10.1186/s12983-018-0249-x
von Beeren, C., Maruyama, M., and Kronauer, D. J. C. (2016). Cryptic diversity, high host specificity and reproductive synchronization in army ant-associated Vatesus beetles. Mol. Ecol. 25, 990–1005. doi: 10.1111/mec.13500
Waller, D. A., and Moser, J. C. (1990). “Invertebrate enemies and nest associates of the leaf-cutting ant Atta texana (Buckley) (Formicudae, Attini),” in Applied Myrmecology A World Perspective, eds R. K. Vander Meer, K. Jaffe, and A. Cedeno (Oxford: Westview Press), 255–273.
Walsh, J. P., and Tschinkel, W. R. (1974). Brood recognition by contact pheromone in the red imported fire ant, Solenopsis invicta. Anim. Behav. 22, 695–704. doi: 10.1016/S0003-3472(74)80020-80025
Weber, N. A. (1941). The biology of the fungus-growing ants. Part Vll. the barro colorado Island, Canal Zone, species. Rev. Entomol. 12, 93–130.
Wei, J., Wang, L., Zhu, J., Zhang, S., Nandi, O. I., and Kang, L. (2007). Plants attract parasitic wasps to defend themselves against insect pests by releasing hexenol. PLoS One 2:e852. doi: 10.1371/journal.pone.0000852
Weissflog, A., Maschwitz, U., Seebauer, S., Disney, R. H. L., and Witte, V. (2008). Studies on European ant decapitating flies (Diptera: Phoridae): II. Observations that contradict the reported catholicity of host choice by Pseudacteon formicarum. Sociobiology 51, 87–94.
Wetterer, J. K., and Hugel, S. (2008). Worldwide spread of the ant cricket Myrmecophilus americanus, a symbiont of the longhorn crazy ant, Paratrechina longicornis. Sociobiology 52, 157–165.
Wheeler, D. E. (1986). Polymorphism and division of labor in Azteca chartifex laticeps (Hymenoptera: Formicidae). J. Kansas Entomol. Soc. 59, 542–548.
Wheeler, J. W., Evans, S. L., Blum, M. S., and Torgerson, R. L. (1975). Cyclopentyl ketones: identification and function in Azteca ants. Science 187, 254–255. doi: 10.1126/science.1111099
Wheeler, W. M. (1900). The habits of Myrmecophila nebrascensis bruner. Psyche 9, 111–115. doi: 10.1155/1900/75323
Wheeler, W. M. (1908). Studies on myrmecophiles. III. Microdon. J. New York Entomol. Soc. 16, 202–213.
Willig, M. R., Kaufman, D. M., and Stevens, R. D. (2003). Latitudinal gradients of biodiversity: pattern, process, scale, and synthesis. Annu. Rev. Ecol. Evol. Syst. 34, 273–309. doi: 10.1146/annurev.ecolsys.34.012103.144032
Wing, K. (1983). Tutelina similis (Araneae: Salticidae): an ant mimic that feeds on ants. J. Kansas Entomol. Soc. 56, 55–58.
Witte, V., Disney, R. H. L., Weissflog, A., and Maschwitz, U. (2010). Studies in European ant-decapitating flies (Diptera: Phoridae): ant alarm pheromone as host finding cue in Pseudacteon brevicauda, a parasite of Myrmica rubra (Formicidae: Myrmicinae). J. Nat. Hist. 44, 905–912. doi: 10.1080/00222930903437366
Wyatt, T. D. (2010). Pheromones and signature mixtures: defining species-wide signals and variable cues for identity in both invertebrates and vertebrates. J. Comp. Physiol. A Neuroethol. Sens. Neural Behav. Physiol. 196, 685–700. doi: 10.1007/s00359-010-0564-y
Yanoviak, S. P., and Kaspari, M. (2000). Community structure and the habitat templet: ants in the tropical forest canopy and litter. Oikos 89, 259–266. doi: 10.1034/j.1600-0706.2000.890206.x
Keywords: formicidae, symbionts, parasitoids, fungus-growing ants, fire ants, Azteca, semiochemical, unintended receivers
Citation: Adams RMM, Wells RL, Yanoviak SP, Frost CJ and Fox EGP (2020) Interspecific Eavesdropping on Ant Chemical Communication. Front. Ecol. Evol. 8:24. doi: 10.3389/fevo.2020.00024
Received: 18 January 2019; Accepted: 28 January 2020;
Published: 13 March 2020.
Edited by:
Ximena E. Bernal, Purdue University, United StatesReviewed by:
Mark A. Elgar, The University of Melbourne, AustraliaPatricia Julia Folgarait, National University of Quilmes, Argentina
Volker Nehring, University of Freiburg, Germany
Copyright © 2020 Adams, Wells, Yanoviak, Frost and Fox. This is an open-access article distributed under the terms of the Creative Commons Attribution License (CC BY). The use, distribution or reproduction in other forums is permitted, provided the original author(s) and the copyright owner(s) are credited and that the original publication in this journal is cited, in accordance with accepted academic practice. No use, distribution or reproduction is permitted which does not comply with these terms.
*Correspondence: Rachelle M. M. Adams, YWRhbXMuMTk3MEBvc3UuZWR1