- 1Department of Microbiology and Immunology, University of Western Ontario, London, ON, Canada
- 2Centre for Human Microbiome and Probiotic Research, Lawson Health Research Institute, London, ON, Canada
- 3Department of Biology, University of Western Ontario, London, ON, Canada
- 4Department of Surgery, University of Western Ontario, London, ON, Canada
Managed populations of the European honey bee (Apis mellifera) support the production of a global food supply. This important role in modern agriculture has rendered honey bees vulnerable to the noxious effects of anthropogenic stressors such as pesticides. Although the deleterious outcomes of lethal pesticide exposure on honey bee health and performance are apparent, the ominous role of sublethal pesticide exposure is an emerging concern as well. Here, we use a data harvesting approach to better understand the toxicological effects of pesticide exposure across the honey bee life cycle. Through compiling adult- and larval-specific median lethal dose (LD50) values from 93 published data sources, LD50 estimates for insecticides, herbicides, acaricides, and fungicides are highly variable across studies, especially for herbicides and fungicides, which are underrepresented in the meta-data set. Alongside major discrepancies in these reported values, further examination of the compiled data suggested that LD50 may not be an ideal metric for honey bee risk assessment. We also discuss how sublethal effects of pesticide exposure, which are not typically measured in LD50 studies, can diminish honey bee reproduction, immunity, cognition, and overall physiological functioning, leading to suboptimal honey bee performance and population reduction. In consideration of actionable solutions to mitigate the effects of sublethal pesticide exposure, we have identified the potential for probiotic supplementation as a promising strategy that can be easily incorporated alongside current agricultural infrastructure and apicultural management practices. Probiotic supplementation is regularly employed in apiculture but the potential for evidence-based targeted approaches has not yet been fully explored within a formal toxicological context. We discuss the benefits, practical considerations, and limitations for the use and delivery of probiotics to hives. Ultimately, by subverting the sublethal effects of pesticides we can help improve the long-term survival of these critical pollinators.
Introduction
Popular interest in the biology of the common European honey bee (Apis mellifera) has surged in recent years due to the stark population decline of this important pollinator (Goulson et al., 2015). Managed colonies of Apis mellifera, strictly speaking, are an invasive insect species to the Americas (Whitfield et al., 2006), but contribute to the production of roughly a third (∼35%) of the global food supply (Blacquière et al., 2012). In Canada, this single insect species is tied to a ∼$2.5 billion (CAD) industry of pollination services, whereby colonies are strategically situated in orchards and fields to promote farmer yields via the cross-fertilization of flowering crops (Mukezangango and Page, 2017). In the United States, the value of bee-mediated pollination is even larger (Calderone, 2012). Despite the value of honey bees to the agri-food industry, we have yet to fully understand how their populations cope with natural- and agriculture-induced stress, or to what extent this stress explains recent increases in reported mortalities (Goulson et al., 2015).
Though no single factor can provide a universal explanation for the apparent decline of honey bee populations, one overriding theme to emerge from the front lines of a global research effort is that more than one factor combines to overwhelm bee health. Among them, pesticide exposure (Sanchez-Bayo and Goka, 2014; Zhu et al., 2014), pathogens (Evans and Schwarz, 2011), and habitat loss (Clermont et al., 2015; Youngsteadt et al., 2015) are prime factors that disproportionately contribute to the decline. In particular, sublethal pesticide exposure has been a popular focus of political discussion, which has highlighted the potential conflict between parties that rely on the production and use of commercial pesticides and those who advocate for their regulation and alternative means of crop pest control. Moreover, the risk of pesticides to honey bees is especially alarming due to their long half-lives (Bonmatin et al., 2015) and presence in food (Lu et al., 2018) and honey (Mitchell et al., 2017).
Herbivorous pest insects are the intended target of systemic application of agriculture insecticides. Nonetheless, honey bees are insects just the same and thus cannot help but to be vulnerable through incidental exposure. These pesticides are applied to crops in two main ways: spraying and seed coating, both of which have effects on honey bee exposure. Spraying is typically accomplished through aerial application, but some use vehicle-based sprayers or manual units. These are effective as a means of pest control but can inadvertently affect honey bees through direct topical contact or through secondary exposure via bee consumption of contaminated pollen, nectar, or water (Fairbrother et al., 2014; Poquet et al., 2014; Park et al., 2015; Zhu et al., 2015). Furthermore, spray-based application allows pesticides to disseminate into the broader environment and contaminate surrounding habitats, including orchards and fields that are not intentionally sprayed (McArt et al., 2017; Simon-Delso et al., 2017). As an alternative to sprays, seed coatings can avoid some off-site targets by more carefully controlling pesticide delivery to its intended crop. However, seed coatings can also cause collateral damage because some pesticides remain active in plant tissue, including nectar and pollen (Krupke et al., 2012; Goulson, 2013; Alburaki et al., 2015; Samson-Robert et al., 2017).
In addition to inadvertent exposure from modern agricultural practices, honey bees can be deliberately exposed to miticides and fungicides by beekeepers through basic hive management practices that aim to combat pests and pathogens. Though best practice for beekeepers is intended to augment the bee’s own defences, their application may sometimes harm the pollinators.
In total, managed honey bee colonies can be exposed to a diverse set of pesticides, which can only be determined by detailed toxicological sampling (Tsvetkov et al., 2017). These chemicals affect bees through any combination of ingestion, contact exposure, or ambient intake through respiratory openings (spiracles). Contact exposure and ingestion as routes of contamination are well studied and reveal pesticide-specific effects on honey bee health (Villa et al., 2000; Stoner and Eitzer, 2013; Sanchez-Bayo and Goka, 2014; McArt et al., 2017). Honey bee respiration, which occurs in respiratory spiracles that are found along the thorax and abdomen of adults, is thought to only be a minor route of pesticide uptake (Geoghegan et al., 2013). Ultimately, these modes of exposure are responsible for the accumulation within individual bees, which can lead to bioaccumulation of pesticides throughout the hive (Figure 1).
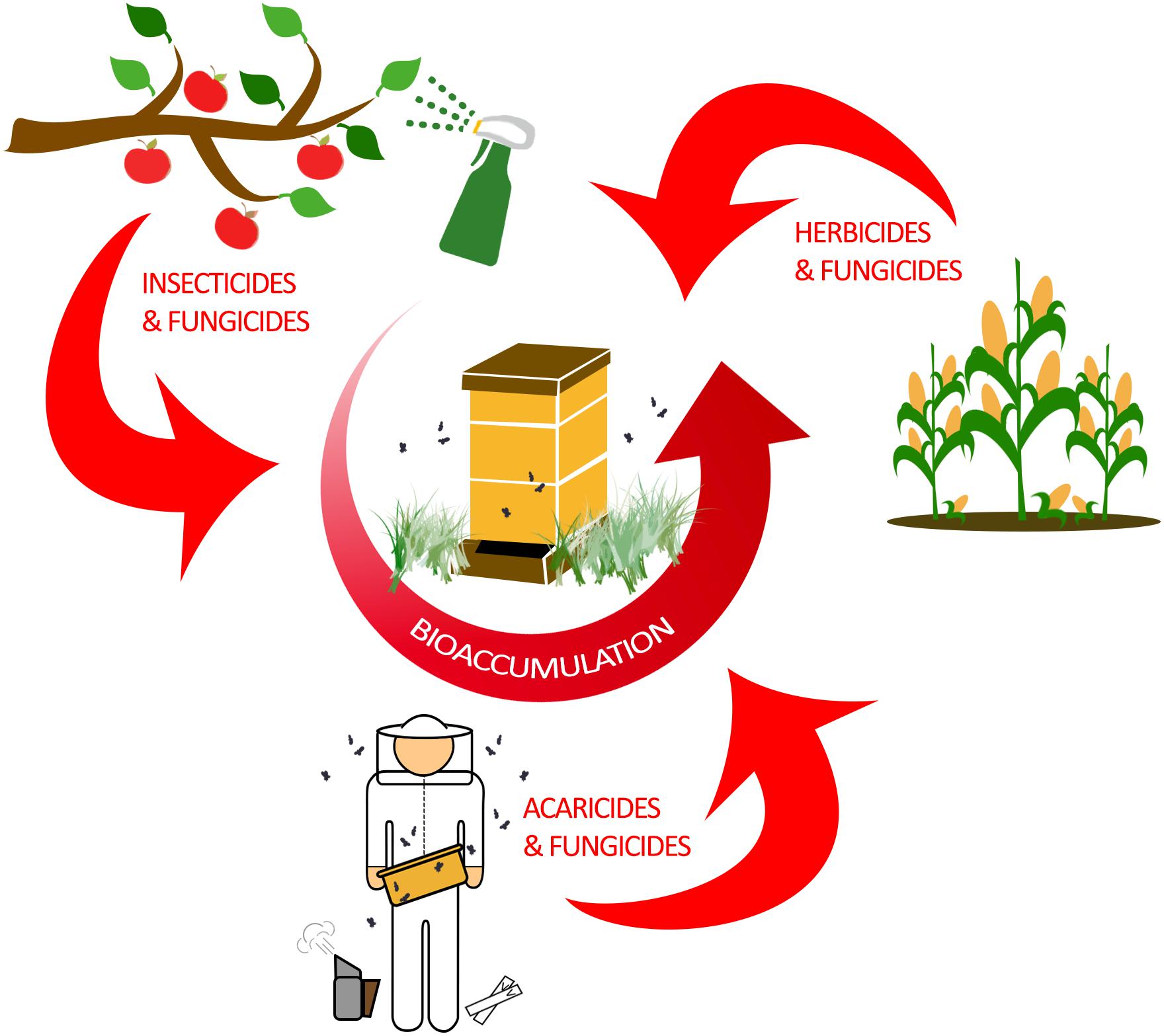
Figure 1. Bioaccumulation of pesticides in a honey bee colony. Honey bees are exposed to a wide variety of pesticides through agricultural practices and modern beekeeping. Typically, farming and other agricultural practices are responsible for exposing honey bees to insecticides, herbicides, and fungicides. As honey bees forage for nectar and pollen, they are incidentally exposed to pesticides which accumulate in the hive by physically transferring the contaminated food sources to unexposed bees. However, honey bees can also be intentionally exposed to acaricides and fungicides by beekeepers in efforts to control mite burden and fungal diseases in the hive. Ultimately, pesticide bioaccumulation in the hive has the potential to negatively impact all honey bee ranks.
The risk to honey bees as a result of pesticide exposure is evaluated by considering both the incidence of exposure and toxicity of pesticides used. Incidence of exposure is quantified by examining the actual usage rates of pesticides, mode of application, and environmentally-relevant concentrations. A widely-used metric for quantifying pesticide-specific toxicity is the lethal dose (LD) at which half the population dies, or the LD50. This latter metric uses acute exposure (24–96 h) of adult honey bees to predict a toxic dose. Despite its widely accepted use, reports of LD50 put less emphasis on larval toxicity and can also vary widely in methodology and sample size. Moreover, these LD50-based risk assessments do not consider the effect that sublethal pesticide exposure can have on honey bees.
In this paper, we evaluate the role of sublethal pesticide exposure (defined herein as exposure to insecticides, acaricides, fungicides, and herbicides) in the toxicity and management of honey bees. To initiate this effort, we first collected a meta-data set of pesticide-specific LD50 for honey bees by analyzing published literature in PubMed, Web of Science, and Google Scholar for studies that contained the following keywords [honey bee], [LD50], and specific pesticide names. We excluded articles if (1) they did not report LD50 values in weight per bee (however, LD50 values reported as weight per mass were converted to weight per single bee, assuming that one bee is 100 mg); (2) the article was inaccessible with current access. In addition to providing both adult and larval LD50 values, this database also allows for the assessment of variation in reported LD50 estimates. We then set out to understand how sublethal pesticide exposure can harm multiple aspects of honey bee health. Again, we conducted a bibliometric search for studies that contained combinations of the following keywords: [honey bees], [pesticide], [sublethal], [development], [immune], [metabolism], [cognition], and [reproduction]. After the initial screen, we used forward and reverse citation searches of individual articles to expand our data set. With the data from these studies, we are able to construct an impartial summary of the adverse effects that sublethal pesticide exposure has on honey bees.
We close by proposing three areas of focus for future studies: expanding the knowledge of sublethal pesticide exposure to other pesticides; understanding the role for the microbiota in aiding host survival toward pesticides; and testing the ability for probiotics to mitigate the sublethal effects of pesticides.
Pesticide Toxicology in Honey Bees
The dose at which 50% of the population dies (LD50) is a useful metric for quantifying pesticide-specific lethality and evaluating sublethal exposure in adult honey bees. Estimates of LD50 can vary by length of exposure and mode of delivery, so knowing the oral- and dermal-specific LD50 of individual pesticides can make a useful predictor of pesticide-associated risk. Further, by comparing LD50 obtained for pest and beneficial insect species, we can better assess the trade-off between intended target species and any collateral damage to pollinators. When combined with pesticide application rates, these values are useful for calculating the risk of pesticide use to pollinators. The Hazard Quotient (HQ = application rate/LD50) is a viable metric to calculate field use risk of pesticide application but can be erroneous alongside variable LD50 values (Stoner and Eitzer, 2013).
Despite the potential of comparative analysis, the variation that is associated with published estimates of LD50 is substantial for both contact (Table 1) and oral (Table 2) versions of this metric, which can reduce their value in risk assessment. The seemingly high variation in LD50 estimates, which can range up to 500-fold, may stem in part from differences in sample size, precision of measurement, and experimental protocol. Even for toxicological studies with a high degree of statistical power, the variance associated with LD50 can be large (Baines et al., 2017). This suggests that the genuine effect of pesticides on insect survivorship may vary strongly between populations, regardless of how it is measured. Biological sources of variation may stem from differences in age (young, nurse-age workers versus older, foraging-age workers), genotype (natural variation as well as apicultural strains), caste (workers, queens, drones), or life stage (larvae versus adults) (Rinkevich et al., 2015; Tosi and Nieh, 2019). A cursory comparison between adult- and larval-derived LD50 values suggests strong biological variance from life stages (Table 3). A detailed dataset of all LD50 values collected for this manuscript can be found in Supplementary Table S1.
Additional sources of variation can occur from the composition of the pesticide formulations that are used. While different amounts of solvents used for toxicology analysis can affect the readout (Wilkins et al., 2013), pesticide adjuvants (other ingredients found in pesticide formulations that are thought to be inert) can also influence pesticide toxicity (Chen et al., 2019). An emerging interest is the potential for synergistic toxicity between multiple pesticides that are applied in combination. These could increase overall honey bee mortality, albeit in unpredictable ways (Johnson et al., 2013; Zhu et al., 2014; Wade et al., 2019). Despite their relevance under normal field conditions, these aspects of pesticide toxicology are often overlooked in LD50 studies, which typically determine the toxicity of individual pesticides in standard laboratory solvents.
Pesticides Affect Detoxification and Metabolism in Honey Bees
Like most insects, honey bees use an array of enzymes to detoxify pollutants and other harmful chemicals that they may encounter, including pesticides (Gong and Diao, 2017). However, honey bees are genetically depauperate in a number of key detoxification genes, with the remainder of relevant genes expressed at low levels (Claudianos et al., 2006). Some key detoxifying genes that appear underrepresented in the honey bee genome include many of the cytochrome P450 monooxygenases (phase I detoxification—oxidation, reduction, and hydrolysis of xenobiotics), glutathione-S-transferases (phase II detoxification—increase water solubility of xenobiotics for excretion), and carboxyl/cholinesterases (insecticide resistance) compared to the well-studied insect model, Drosophila melanogaster (Claudianos et al., 2006). Although honey bees possess similar amounts of detoxification genes compared to other members of the Apidae family, they have far less compared to pest insects, thus making honey bees more susceptible to pesticide exposure (Sadd et al., 2015). The diminished repertoire of detoxifying genes in the honey bee might stem from compensatory mechanisms associated with their highly social behavior, including herd immunity (Evans et al., 2006; Cremer et al., 2007) and a ‘social detoxification system,’ which focuses on how hive behavioral dynamics can reduce the burden of toxin substances on the detoxification system of individual members (Berenbaum and Johnson, 2015). It is uncertain if the relatively small innate capacity of the honey bee is fully compensated by social effects or if the bees remain genetically more sensitive to the toxic effects of pesticides.
Within colonies, caste and diet can modulate the expression of detoxification-related genes. Forager bees, for example, express more detoxification genes than do nurse bees (Vannette et al., 2015), potentially owing to the heightened risk of exposure toward environmentally-derived xenobiotics. Moreover, honey bee diets with ample pollen or nectar provide some resistance toward pesticides (Schmehl et al., 2014; Tosi et al., 2017b). This may be achieved by nutrient-mediated increases in detoxification enzyme gene expression or by improving physiological resilience through ensuring adequate nutrition levels (Mao et al., 2013).
A lesser explored mechanism for detoxification is the gut microbiota—a community of microorganisms residing within the honey bee gastrointestinal tract, consisting of a core set of evolutionarily adapted bacterial species (Ellegaard et al., 2019) that compensate for insufficiencies in honey bee metabolism (Kešnerová et al., 2017; Zheng et al., 2017); thus it may have a role in detoxification. The microbiota can aid xenobiotic detoxification by directly detoxifying harmful substances and indirectly through modulating the host’s detoxification response. The metagenome of the honey bee gut microbiota is enriched with carbohydrate and plant chemical metabolizing genes suggesting a direct role in detoxification (Engel et al., 2012; Kwong et al., 2014; Lee et al., 2015; Kešnerová et al., 2017). For example, Lactobacillus kunkeei is able to metabolize phenolic acids found in pollen (Filannino et al., 2016), while Gilliamella apicola has the potential to metabolize toxic mannose constituents that are found in nectar (Barker and Lehner, 1974; Zheng et al., 2016). By directly metabolizing toxic substances, the microbiota is able to increase honey bee resistance to xenobiotics. Microbes can also regulate honey bee detoxification systems leading to indirect detoxication capability. For instance, the presence of certain early colonizers during development, such as Snodgrassella alvi, can modulate phase I detoxification pathways by affecting the expression of cytochrome P450 (CYP) enzymes (Schwarz et al., 2016) that are critical for pesticide degradation (Claudianos et al., 2006).
We do not yet know how gut colonizers influence detoxification of pesticides in honey bees under natural conditions, though findings in other insects suggest a major role for symbiont-mediated regulation of detoxification pathways (Pietri and Liang, 2018). As the gut microbiota is dependant on caste (Jones et al., 2018), diet (Maes et al., 2016; Zheng et al., 2017), and environmental factors (Jones et al., 2017), including exposure to some pesticides (Dai et al., 2018), studies should test the ability of the microbiota to either directly degrade pesticides or promote indirect detoxification of them. Caution should be exercised when investigating monoculture-based experiments because they do not account for the complexities of the entire microbiota, which include host–microbe interactions, microbe–microbe interactions, and strain-specific differences in metabolic capacities.
Pesticides themselves can also play a role in modulating metabolism and expression of detoxification genes. Boncristiani et al. (2012) showed that honey bees exposed to thymol and coumaphos have altered cytochrome P450 subfamily and protein kinase superfamily-related gene expression. These two gene families can affect resistance to insecticides like DDT (Le Goff and Hilliou, 2017) and neonicotinoids (Gong and Diao, 2017). Moreover, honey bees exposed to myclobutanil (fungicide) have impaired cytochrome P450-mediated detoxification of quercetin (flavonol phytochemical found in nectar and pollen), which is metabolized by CYP9Q1 (Mao et al., 2017). These studies reveal that synergistic effects between multiple types of pesticides can amplify the effective toxicity. As for insecticides, imidacloprid exposure has been shown to broadly up-regulate cytochrome P450 expression (Derecka et al., 2013; De Smet et al., 2017; Zhu et al., 2017), likely in response to the pesticide itself, thereby facilitating its detoxification.
Honey bees exposed separately to myclobutanil, imidacloprid, or fipronil have disrupted ATP production (Nicodemo et al., 2014; Mao et al., 2017), suggesting that exposure to these pesticides alters cellular metabolism. In particular, Nicodemo et al. (2014) demonstrated that imidacloprid and fipronil reduce oxygen consumption and impair mitochondrial function. This reduction in aerobic respiration is accompanied by an increase in glycolysis and citric acid cycle-related gene expression in exposed honey bees (Roat et al., 2014; Renzi et al., 2016a). Thus, pesticide exposure may be favouring low-efficiency means of ATP production (glycolysis and citric acid cycle) over higher efficiency means (oxidative phosphorylation). Interestingly, using near-infrared light (670 nm) to restore mitochondrial function can mitigate ATP reduction, diminish physiological impairments, and improve survival in bumblebees (Bombus terrestris) (Powner et al., 2016).
Pesticides Negatively Affect Motor Function, Behavior, and Cognition
Honey bees are highly social insects. They rely on individual cognition to navigate their environment and respond to changing conditions and colony needs. Forager bee cognition is demonstrated by their ability to encode memories of resources, which are typically found within a 2 – 6 km radius of the hive (Hagler et al., 2011; Couvillon et al., 2015). These memories are then transmitted through waggle dances to other foragers to encourage the process of collecting hive resources, which promotes success of a colony (Henry et al., 2012). Exposure to pesticides appears to impair the foraging response in a dose-dependent relationship.
Acute neonicotinoid exposure induces a series of symptoms that are consistent with hyper-responsive neural impairments (Suchail et al., 2001). These are observed as excitation symptoms, which include increased time in the air, increased flight distances, and an inability to right themselves when placed on their backs (Yang et al., 2008; Williamson et al., 2014; Tosi et al., 2017a). By contrast, chronic exposure induces hypo-responsive neurological impairments (Suchail et al., 2001). These include decreased flight speed, decreased flight duration, and impaired navigation (Fischer et al., 2014; Tison et al., 2016; Tosi et al., 2017a). Thus, initial exposure to neonicotinoids can overstimulate honey bees and induce a hyper-responsiveness, which leads to exhaustion and hypo-responsiveness over continued exposure. One implication of this would be that neonicotinoid exposure drives foragers to go far distances, where they eventually become exhausted and lose their spatial awareness, thus hampering collection of resources by preventing them from returning to the hive. As a result, nurse bees may begin foraging at a younger age, thus creating a group of precocious foragers, which may reduce the number of nurse bees available for rearing brood (Robinson et al., 1989).
Honey bees likely cannot tell if food is contaminated with pesticides (Williamson et al., 2014; Kessler et al., 2015); so they are not averse to it. Fortunately, pesticide exposure reduces trophallactic transfer of food from donor to recipient (Bevk et al., 2012; Brodschneider et al., 2017). Although this may reduce the spread of pesticide-contaminated food within a colony, the change in social behavior may also compromise other forms of communication, including the waggle dance (which allows successful foragers to inform others in the colony on the direction and distance to food and water or new nesting sites) (Eiri and Nieh, 2016), or reduce larval feeding altogether (Gil and De Marco, 2005).
The most pronounced pesticide-induced cognitive impairments are on olfactory learning, visual learning, and memory. Olfactory learning occurs when honey bees learn to associate an odour with an award, which is often tested using the proboscis extension reflex (PER). Honey bees exposed to imidacloprid show reduced PER activity compared to unexposed bees (Decourtye et al., 2004; Han et al., 2010; Goñalons and Farina, 2018). Meanwhile, other pesticides show differential impairments to both short-term and long-term memory (Williamson and Wright, 2013; Wright et al., 2015). Pesticides have likewise been shown to affect visual and associative learning in honey bees (Hesselbach and Scheiner, 2018). For example, Han et al. (2010) found that using their T-tube maze, less than half of bees treated with imidacloprid were able to successfully make the correct decision in a visual learning task, suggesting that imidacloprid impaired visual learning. As this is used to remember food locations and predators, it may explain why Eastern honey bees (Apis cerana) exposed to sublethal imidacloprid do not show aversion to the predator hornet, Vespa velutina (Tan et al., 2014). Imidacloprid may reduce the visual association of the predator with the cognitive fear response. Pesticide exposure therefore appears to play a role in cognitive deficiencies, which may be mediated by direct effects of the chemicals on the brain.
On a cellular level, pesticides interfere with neuronal polarization in mushroom bodies, a segment of the honey bee brain that is associated with learning, memory, and sensory integration (Plath et al., 2017). Mushroom bodies are composed of Kenyon cells (neural cells). When these cells are exposed in vitro to coumaphos oxon (a metabolite of coumaphos) or imidacloprid, they show a modified synaptic profile, which is characterized by a slow depolarization, followed by increased excitability, then inhibition of the action potential (Palmer et al., 2013). These pesticides are partial agonists of nicotinic acetylcholine receptors; thus, they could be acting on these receptors and blocking a natural acetylcholine response, which will alter the neural cell action potential. The modified action potential elicited by this class of pesticides may explain some of the impairment to the aforementioned cognitive processes. In addition, there appear to be differences in the brain proteome and microRNA (miRNA) expression of bees exposed to pesticides (Roat et al., 2014; Shi et al., 2017a; Wu et al., 2017), which could lead to changes in brain development and structure that result in differential signalling. An alternate process to explain neural impairment following pesticide exposure is that pesticides may interfere with the perception of a stimulus rather than the cognition of one. Imidacloprid exposure has been shown to reduce calcium signalling in the antennal lobe in response to an odours stimulus (Andrione et al., 2016). This results in problems perceiving the stimulus as opposed to difficulty coding and recalling the stimulus (cognition). Ultimately, pesticide-induced cognitive related deficits may be a result of a combination of impairments to the honey bee brain.
Pesticides Can Obstruct Reproduction and Development
Exposure to pesticides can slow the reproductive cycle of queens (Figure 2), as illustrated upon exposure to sublethal doses of thiamethoxam during development, resulting in reduced body weight and a lower probability of queen success (Gajger et al., 2017). Likewise, laboratory experiments show that queens exposed to field-realistic concentrations of neonicotinoids carry fewer viable spermatozoa and lay fewer fertilized eggs that would normally develop into diploid (female) workers (Williams et al., 2015; Chaimanee et al., 2016; Wu-Smart and Spivak, 2016). Queens that underperform are eventually targeted by workers for replacement (Sandrock et al., 2014), but in the short-term reproductive succession is costly to the colony. Furthermore, queens exposed to sublethal doses of neonicotinoids have been shown to have reduced mating compared with unexposed queens (Forfert et al., 2017).
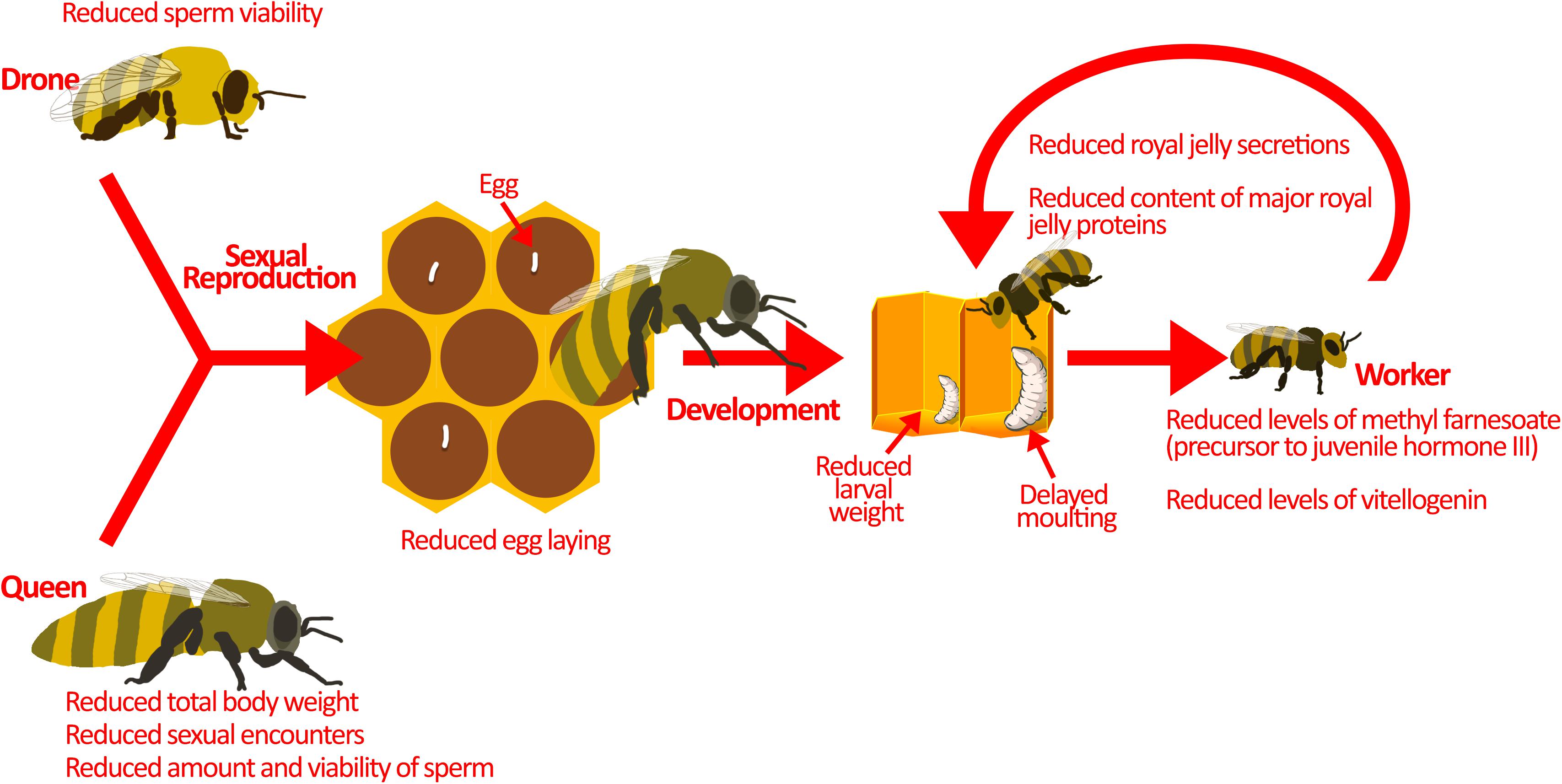
Figure 2. Pesticides interfere with colony reproduction. Sexual reproduction between drones and the queen is the source of genetic diversity in the hive. This is important for pathogen resistance and colony survival. Sublethal pesticide exposure reduces sexual reproduction by affecting the drones and the queen. Drones exposed to pesticides have lower sperm viability. On the other hand, queens exposed to pesticides display reduced sexual encounters, sperm amount, and sperm viability. Moreover, pesticide exposed queens have smaller body weights, which may explain the reduction in sperm amount and egg-laying. Developing larvae are also at-risk during pesticide exposure. They demonstrate atypical progression through developmental phases, reduced larval weight, and delayed moulting. These may be a result of direct pesticide exposure, but pesticides could also be indirectly affecting larvae. Nurse bees exposed to pesticides produce reduced amounts of jelly secretions, and that which is produced has less nutritional value, potentially explaining the indirect effects of pesticides on honey bee larvae. Image of larvae in the hive is adapted from Maori et al. (2019) under Creative Commons Attribution 4.0 International (https://www.sciencedirect.com/science/article/pii/S10972765 19301844).
Drones are also affected by pesticides. Sublethal concentrations of neonicotinoids and phenylpyrazoles can reduce sperm viability (Kairo et al., 2016, 2017a,b; Straub et al., 2016), which can hamper fertilization of queens and the production of diploid workers. Together, reduced sperm transfer and fertilization may limit the production of a genetically diverse workforce, which may compromise the division of labour (Jones et al., 2004) and response to disease (Sherman et al., 1988).
While pesticides are known to interfere with reproduction, they have also been implicated in changes to larval development. Honey bee larvae reared in vitro with thiamethoxam (1/10 of LC50) show atypical progression through developmental stages, including skipping some stages, and reduced larval weight (Tavares et al., 2015). In addition, larvae exposed in vitro to the commonly used herbicide glyphosate show reduced weight and delayed moulting (uncapping of the brood cell) (Dai et al., 2018; Vázquez et al., 2018). These laboratory studies are corroborated by field data showing similar atypical developmental progression upon pesticide exposure (Wu et al., 2011). At the molecular level, honey bees exposed to imidacloprid show changes in miRNA transcription, which are responsible for development (Derecka et al., 2013). In particular, a reduction in the miRNA, mir-14, has been observed (Derecka et al., 2013). Although the exact function of mir-14 is unknown in honey bees, in D. melanogaster it has been shown to modulate metabolism, nutritional status, and larval survival (Varghese et al., 2010; Nelson et al., 2014). Thus, pesticide exposure impairs individual development, contributing to reduced colony strength.
Honey bee larval development is guided by hormone signalling and jelly supplementation. Honey bees treated with coumaphos and fluvalinate (acaricide) show reduced levels of methyl farnesoate, a precursor to juvenile hormone III (Schmehl et al., 2014), which is the main juvenile hormone in insects that functions to regulate honey bee caste differentiation (Nelson et al., 2007; Mutti et al., 2011) and division of labour (Fahrbach and Robinson, 1996). Exposure to neonicotinoids reduces expression of vitellogenin, another protein that is required for honey bee development (Abbo et al., 2017; Shi et al., 2017b). As brood develop, they primarily consume jelly, which is a nutritionally-rich food source produced and delivered by nurse bees. Sublethal neonicotinoids and fungicides reduce the size of the hypopharyngeal and mandibular glands where it is synthesized (Hatjina et al., 2013; Renzi et al., 2016b; Zaluski et al., 2017), which in turn decreases jelly secretions and may lead to reduced longevity and smaller honey bee populations (Yang et al., 2017). The jelly produced may further be deficient in major proteins (Wu et al., 2017) vital for honey bee development and physiology (Buttstedt et al., 2014). These changes in hormone signalling and reduced nutritional value of jelly can contribute to the atypical development of honey bee larvae exposed to pesticides. By limiting the amount of viable brood and the rate at which these few larvae develop, pesticide exposure effectively reduces the overall workforce and success of the colony.
Pesticides Disrupt Honey Bee Immunity
Honey bees exposed to pesticides have increased loads of bacterial, fungal, and viral pathogens (Pettis et al., 2012; Wu et al., 2012; DeGrandi-Hoffman et al., 2013; Di Prisco et al., 2013; Alburaki et al., 2015; Doublet et al., 2015; Chaimanee et al., 2016; Fine et al., 2017). This has raised concern for the potential of synergistic interactions between pesticides and pathogens that exacerbate mortality in honey bees (Alaux et al., 2010a; Pettis et al., 2013; Paris et al., 2017; Grassl et al., 2018; O’Neal et al., 2019; Straub et al., 2019; Tesovnik et al., 2019). Vidau et al. (2011) demonstrated that honey bees previously infected with Nosema ceranae were more sensitive to subsequent pesticide exposure. Parasites like Nosema might therefore increase pesticide-related mortality, possibly by altering the expression of detoxification enzymes. As the adult honey bee gut microbiota develops 4–6 days after eclosion and is composed of bacteria from older bees and the hive environment (Powell et al., 2014), one concern would be potential colonization with disease-causing microorganisms that could alter resistance to pesticides (Li et al., 2017; Koleoglu et al., 2018). Conversely, pesticides may cause immunosuppression in honey bees, rendering them more susceptible to pathogens. To better understand the possible synergism between pesticides and pathogens, it is essential to consider individual immunity and social immunity.
Individual honey bee immunity is divided into humoral and cellular immune responses, both of which are impaired by sublethal pesticide exposure (Figure 3). The humoral response is initiated by recognition of pathogen-associated molecular patterns (PAMPs), which trigger signalling through one of the four insect immune pathways: (1) the Toll pathway, (2) the Immune Deficiency (IMD) pathway, (3) the c-Jun N-terminal kinase (JNK) pathway, and (4) the Janus kinase/signal transducers and activators of transcription (JAK/STAT) pathway (Danihlík et al., 2015). Activation of these humoral immune pathways leads to the production of antimicrobial peptides (AMPs), which can be proteases, complement-like proteins, or broad-range microbiocidal proteins. In insects, these signalling pathways and proteins are conserved. However, honey bees harbour fewer paralogues, gene copies, and splice variants of immune genes compared to Drosophila and Anopheles (Evans et al., 2006).
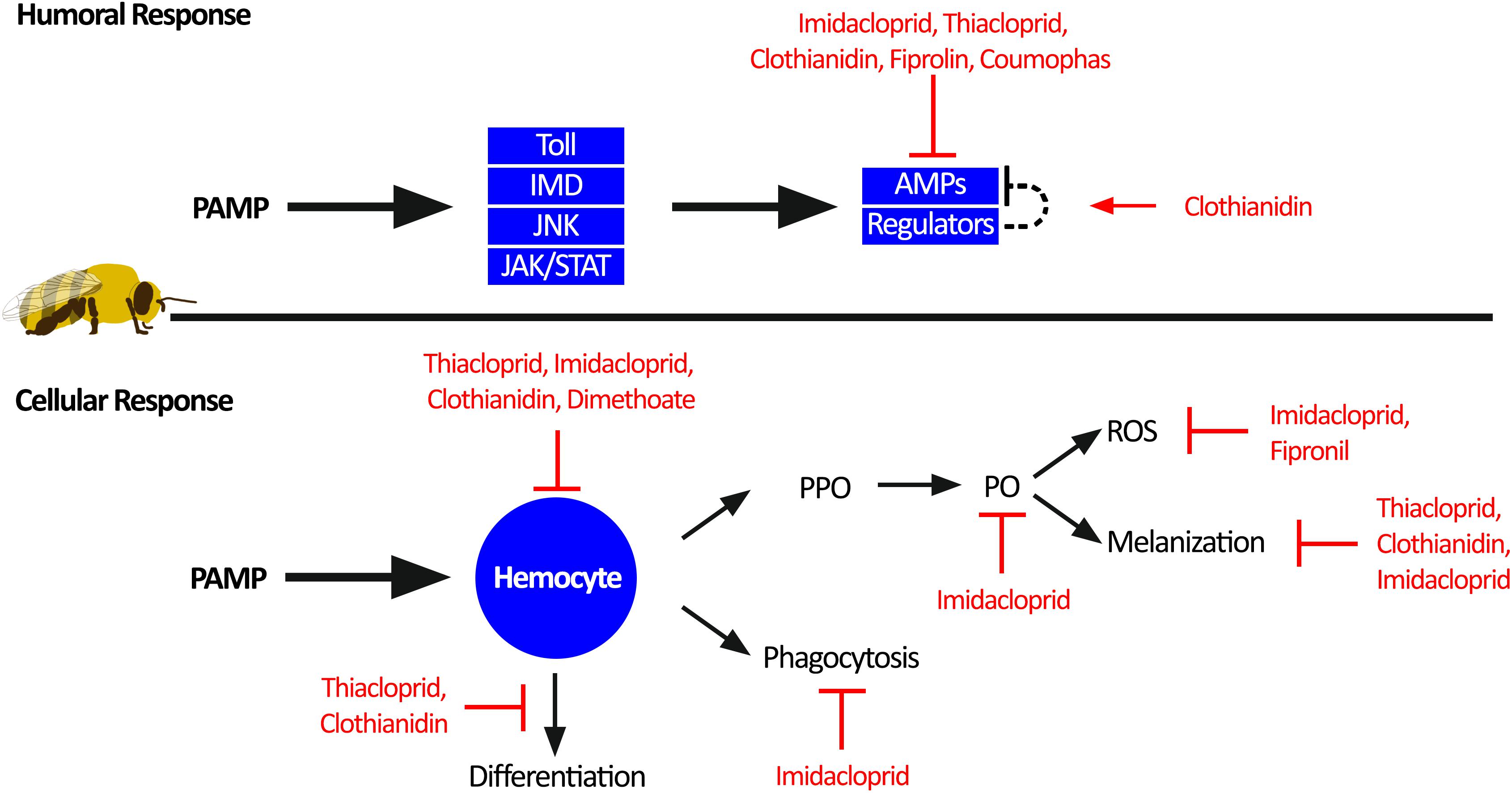
Figure 3. Individual honey bee immunity impairment by pesticides. Honey bee immune response toward pathogen-associated molecular patterns (PAMPs) can be divided into humoral response and cellular response. The humoral response generates antimicrobial peptides (AMPs) through activation of the four immune pathways: Toll, immune deficiency pathway (IMD), c-Jun N-terminal kinase (JNK), and Janus kinase/signal transducers and activators of transcription (JAK/STAT). Sublethal pesticide exposure impairs the humoral immune response by reducing the production of AMPs. The cellular immune response is orchestrated through hemocyte function. Hemocytes can facilitate melanization of pathogens and wounds through activation of prophenoloxidase (PPO) to phenoloxidase (PO) and reactive oxygen species (ROS) as a by-product. In addition, hemocytes can phagocytosis and clear invading pathogens, as well as differentiation into other immune cells. Multiple aspects of the cellular immune response are impaired by sublethal pesticide exposure.
Exposure to pesticides reduces global AMP generation, thus further compromising an already depauperate immune system (Garrido et al., 2013; Aufauvre et al., 2014; Tesovnik et al., 2017; Wu et al., 2017). Although the specific mechanisms by which AMP production is reduced are largely unknown, Di Prisco et al. (2013) demonstrated that honey bees exposed to clothianidin had increased expression of a leucine-rich repeat protein (Amel/LRR), which is similar to the D. melanogaster gene CG1399, a negative regulator of NF-κB signaling (Toll and IMD). Therefore, by increasing the expression of negative immune regulators, this pesticide acted to reduce AMP production, leading to higher infection titres of deformed wing virus (Di Prisco et al., 2013). Although this study only represents one specific mechanism for one class of pesticide, it is possible that combined exposure to multiple classes of pesticide may further dysregulate the immune response leading to drastic outcomes on pathogen load and mortality.
Activation of the cellular immune response also occurs through recognition of PAMPs, but instead triggers migration of hemocytes, which leads to encapsulation of the pathogen and activation of prophenoloxidase (PPO) to phenoloxidase (PO). Active PO catalyzes the production of a melanin polymer capsule around the pathogen (melanization response). Reactive oxygen species and nitric oxide intermediates are also created, which are important in pathogen defence (Paris et al., 2017; Walderdorff et al., 2018). Neonicotinoid exposure impairs this melanization response (Brandt et al., 2016, 2017), potentially due to reduction of PO activity (Zhu et al., 2017) or through the reduction of reactive oxygen species and nitric oxide (Paris et al., 2017; Walderdorff et al., 2018). Consequences of this would be reduced pathogen isolation and clearance, and slower wound healing, both of which could increase viral loads and systemic infections (Brandt et al., 2017).
Systemic infections in honey bees could be exacerbated by acaricide, neonicotinoid, or fungicide exposure, which reduces intestinal stem cell proliferation (Forkpah et al., 2014) and increases midgut apoptosis (Gregorc et al., 2018; Carneiro et al., 2019), potentially weakening the gut barrier. Hemocytes also function as phagocytic cells in the honey bee hemolymph; however exposure to neonicotinoids reduces hemocytes phagocytic activity (Walderdorff et al., 2018) and hemolymph antimicrobial activity (Brandt et al., 2016). These pesticide-exposed hemocytes also display altered differentiation profiles and reduced total cell counts (Brandt et al., 2016, 2017; López et al., 2017), another factor that would reduce the magnitude of the melanization response. Despite the documented consequences of neonicotinoid pesticides on hemocytes and cellular immune responses, the mechanisms remain elusive. Studies on D. melanogaster and Chilo suppressalis demonstrate that the nervous system can regulate hemocyte proliferation (Makhijani et al., 2017), and neurotransmitters have a role in modulating hemocyte phagocytosis (Wu et al., 2015; Qi et al., 2016). Thus, pesticides may act through the nervous system to dysregulate hemocytes. Future studies should explore the mechanisms of pesticide-induced impairment of hemocytes, with a focus on pesticide dysregulation of neuro-immune cell signaling.
Social immunity, where individuals contribute to group health, can arise in different ways—for example, through individual secretion of peptides that effectively sterilize the hive environment. Glucose oxidase (GOX) is secreted from the hypopharyngeal glands and catalyzes the production of hydrogen peroxide (H2O2) to sterilize the hive. Reeves et al. (2018) showed that GOX activity is increased in nurse bees and forager bees exposed to acaricides, tau-fluvalinate, and coumaphos. On the other hand, Alaux et al. (2010a) demonstrated that there is a synergistic interaction between imidacloprid exposure and Nosema infection, whereby GOX activity is reduced. Differences reported between these studies may stem from the use of dissimilar classes of pesticides or experimental methods used. For example, Alaux et al. (2010a) fed caged bees a sucrose solution, while Reeves et al. (2018) assayed bees directly collected from hives. Addressing these disparities, the latter set of bees were exposed to the natural environment, which would have allowed them access to pollen—a dietary component that increases GOX activity (Alaux et al., 2010b). Defensin 1 (Def 1) is a social immunity peptide that is secreted into the hive environment and is particularly effective against Gram-positive bacteria. Studies show that Def 1 expression may increase (thiamethoxam) (Tesovnik et al., 2017), decrease (fipronil) (Aufauvre et al., 2014) or remain unchanged (acaricides) (Garrido et al., 2013) in response to exposure of different types of pesticides.
Honey bees also practice various hygienic behaviors that reduce pathogen load within colonies, most notably self- or mutual-grooming and removal of dead bees. Wu-Smart and Spivak (2016) found that worker bees treated chronically with imidacloprid displayed significantly reduced hygienic removal of freeze-killed brood. Likewise, de Mattos et al. (2017) showed that synthetic acaricides (coumaphos, amitraz, and tau-fluvalinate), caused workers to groom less, which led to higher Varroa destructor loads. Meanwhile, Williamson et al. (2013) showed that acetylcholinesterase inhibitors (coumaphos, chlorpyrifos, aldicarb, and donepezil) actually increased worker bee grooming. The discrepancy between these two studies might be a result of the methodology used. While Williamson et al. (2013) recorded grooming without a stimulus, de Mattos et al. (2017) tested grooming in the presence of a Varroa mite. Nonetheless, it appears that the social immune response to pesticide exposure is variable and must be further explored.
A lesser understood aspect of honey bee immunity is the role of the microbiota in both direct and indirect pathogen inhibition. It is well documented that through direct inhibition, microbiota constituents are able to counter pathogens via competitive inhibition, production of organic acids, and secretion of antimicrobial molecules (Evans and Armstrong, 2005, 2006; Forsgren et al., 2010; Olofsson et al., 2016; Khaled et al., 2018). Additionally, the microbiota can indirectly improve pathogen resistance by stimulating multiple aspects of individual immunity. In particular, constituents of the gut microbiota can activate the humoral immune system to produce AMPs, that are critical to combating pathogens (Kwong et al., 2017; Li et al., 2017). Also, organisms such as Frischella perrara are able to stimulate the cellular immune response and induce dark-colored ‘scabs’ on the epithelium of the pylorus, which is a result of melanization (Emery et al., 2017).
While it has been shown that certain neonicotinoid pesticides like imidacloprid do not alter the microbiota (Raymann et al., 2018b), a wealth of evidence suggests that exposure to a variety of other types of pesticides can alter the abundance and composition of the gut microbiota (Kakumanu et al., 2016; Dai et al., 2018; Motta et al., 2018; Blot et al., 2019; Rouzé et al., 2019). These studies reveal that pesticide exposure mainly decreases Bifidobacterium spp. and Lactobacillus spp., with some of this research (Motta et al., 2018; Blot et al., 2019; Rouzé et al., 2019) suggesting that abundances of S. alvi and G. apicola may also be affected. These alterations are associated with exacerbated pathogen mortality (Mattila et al., 2012; Maes et al., 2016; Li et al., 2017; Rubanov et al., 2019). Therefore, by altering the microbiota of honey bees, pesticide exposure increases the insect’s susceptibility to pathogens.
Solutions For Mitigating the Deleterious Effects of Pesticides: the Potential of Probiotics
It is quite evident that pesticides have deleterious effects on honey bees. Thus, a solution is needed to limit these off-target effects. Naturally, by completely removing pesticides, honey bees would no longer be exposed to them, but this solution may reduce crop yield and burden the food supply (Hossard et al., 2014). Another idea is to circumvent pesticide use by mandating better farming practices that reduce damage from pests (Lechenet et al., 2014). However, these methods are subject to the legislative process and competing interests, and do not empower beekeepers themselves with a means to combat the pesticide issue. As pesticides may increase the likelihood of infection, beekeepers have a few methods to reduce pathogen-associated honey bee mortality. They routinely use fungicides and acaricides to prevent Nosema and Varroa infection. However, these chemicals adversely affect the bees. Antibiotics used to combat bacterial pathogens such as Paenibacillus larvae (American foulbrood) and Melissococcus plutonius (European foulbrood), also disrupt the microbiota of honey bees, increase antibiotic resistance in pathogens, and ultimately increase mortality (Alippi et al., 2014; Krongdang et al., 2017; Li et al., 2017; Raymann et al., 2017, 2018a). All things considered, conventional solutions are not effective at combating honey bee decline and the need for new approaches is evident.
One novel concept may be through supplementation with lactic acid bacteria (LAB; such as Lactobacillus and Bifidobacterium spp.) to mitigate the harmful effects of pesticides and pathogens. The basis for this is several-fold, but the most discernible benefit of LAB supplementation is that it can reduce pesticide absorption via degradation (Islam et al., 2010; Lénárt et al., 2013; Zhang et al., 2016; Li et al., 2018) or by sequestering ingested pesticides, thereby allowing them to pass through the digestive tract rather than be absorbed (Trinder et al., 2016). In other model organisms, LAB have been shown to reduce toxicity and have a protective effect to the host (Bouhafs et al., 2015; Bagherpour Shamloo et al., 2016), thus establishing a basis for future studies to investigate this potential in honey bees.
Collectively through direct and indirect mechanisms of pathogen resistance, supplementing honey bees with beneficial bacteria is able to reduce Nosema spore counts (Sabaté et al., 2012; Maggi et al., 2013; Corby-Harris et al., 2016; Arredondo et al., 2018) and P. larvae bacterial load (Forsgren et al., 2010; Arredondo et al., 2018; Daisley et al., 2019). In vivo evidence from a D. melanogaster model of pesticide exposure has shown that supplementation with LAB improves immunity of pesticide-exposed flies via immune stimulation (Daisley et al., 2017; Chmiel et al., 2019). Likewise, LAB are able to stimulate AMP production in honey bees and improve survival during Paenibacillus larvae infection (Evans and Lopez, 2004; Daisley et al., 2019). Together these studies demonstrate that beneficial bacteria can indirectly contribute to pathogen resistance by stimulating the immune system and assisting the host in overcoming infection.
Some LAB are able to directly inhibit pathogens, thus enhancing overall honey bee resistance to pathogens. For example, isolates of L. kunkeei have been shown to inhibit N. ceranae, P. larvae, and Serratia marcesscens (Forsgren et al., 2010; Olofsson et al., 2016; Al-Ghamdi et al., 2017; Arredondo et al., 2018). Lactobacillus kunkeei also produces biofilms in honey bees, which facilitates its vertical transmission from one generation to the next (Vásquez et al., 2012). Another LAB, Lactobacillus apis R4BT, can inhibit P. larvae and M. pluntonius, in vitro (Killer et al., 2014). Some Bifidobacterium species inhibit P. larvae and S. marcesscens, and when found adequately in the microbiota they are associated with reduced pathogen load (Forsgren et al., 2010; Mattila et al., 2012; Olofsson et al., 2016). Honey bee-derived Lactobacillus johnsonii CRL1647 is a well-documented LAB shown to reduce the abundance of Nosema and Varroa in the hive (Audisio et al., 2015). Although the mechanism for direct pathogen inhibition is not completely clear, it is likely a combination of the production of organic acids (Maggi et al., 2013), bacteriocins (Audisio et al., 2018), and other antimicrobial proteins (Butler et al., 2013). The net effect is that this is a viable method to mitigate the immune impairment caused by sublethal pesticide exposure, and beneficial bacterial supplementation could prove as an alternative to antibiotic use by reducing pathogen burden.
In addition, beneficial bacteria can bolster colony development, which is notably decreased by pesticide exposure. Honey bees supplemented with LAB typically produce more honey, have more pollen stores, and have increased brood counts (Audisio and Benítez-Ahrendts, 2011; Audisio et al., 2015; Alberoni et al., 2018; Fanciotti et al., 2018). For example, L. johnsonii CRL1647 stimulates egg-laying, which can increase the hive population (Audisio and Benítez-Ahrendts, 2011). These positive effects have been partially attributed to organic acid production (Maggi et al., 2013), but could also be attributed to microbiota restoration as ‘non-thriving’ hives typically have lower levels of Lactobacillus and Bifidobacterium compared to ‘thriving’ hives (Ribière et al., 2019).
The long-standing challenge to supplementing honey bees with beneficial bacteria is in the delivery method (Figure 4). A number of commercial bee supplements containing dried LAB suggest ‘dusting’ frames with the bacteria, which may also promote grooming. Although this application method is minimally invasive, the efficacy is not well known. Moreover, dusting is prone to uneven distribution and is negatively impacted by moisture and humidity.
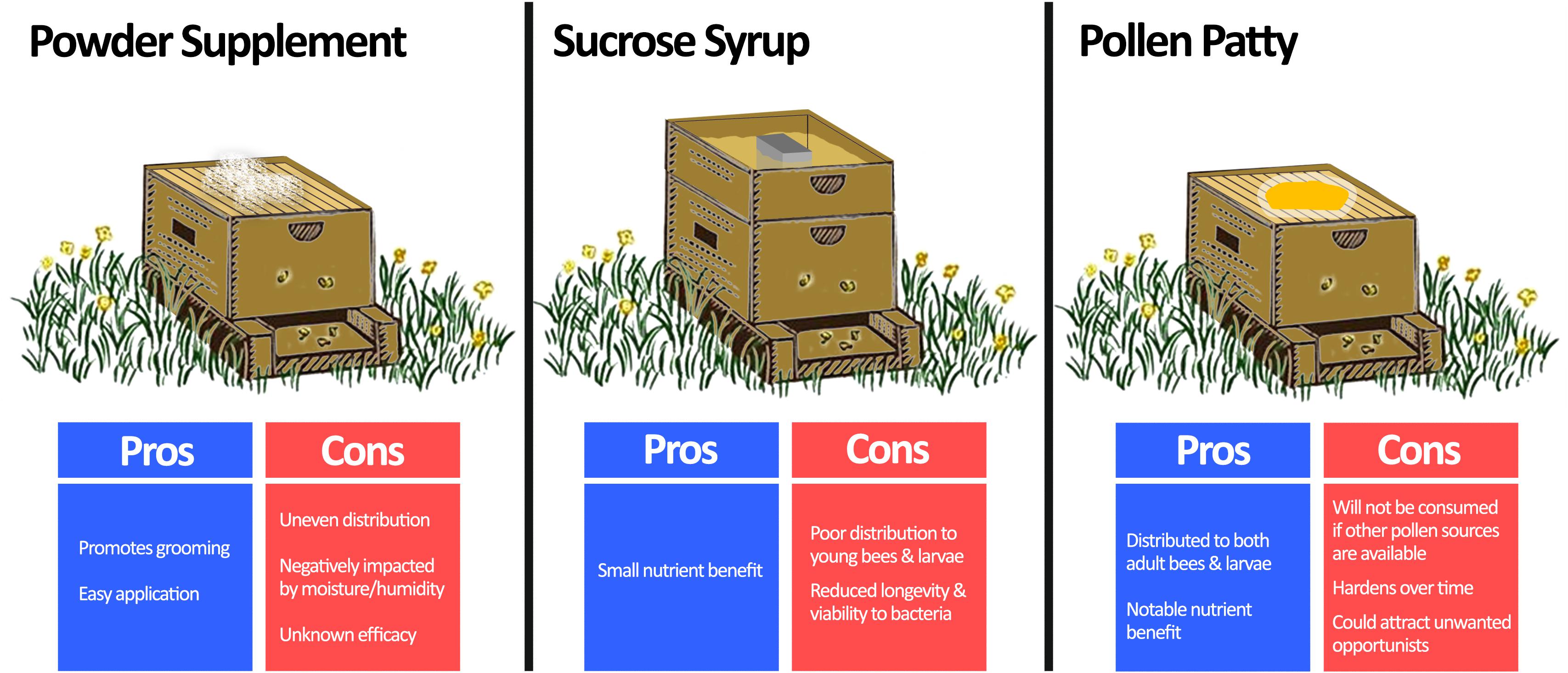
Figure 4. Comparison of methods for beneficial bacteria supplementation. Beneficial bacteria are usually combined with a vehicle to supplement honey bees in one of three ways: powder supplementation, sucrose syrup, or pollen patty. Powder supplementation can be easily performed by spreading a probiotic infused dust on the beehive, which also promotes bees to groom. However, it is prone to uneven distribution, negative impacts of moisture, and unknown efficacy as an application method. Sucrose syrup supplementation can be achieved by adding probiotics directly to conventional sucrose feeders for the hive. Although this method benefits from a small nutrient enhancement, the sucrose solution is not usually distributed well to all members of the hive, and it is an unfavourable environment for the bacteria. Pollen patty supplementation involves adding beneficial bacteria directly to a traditional pollen supplement. In addition to the added nutrient benefit, pollen patty supplementation will be distributed throughout the hive to both adult bees and larvae. However, if sufficient nutrient sources already exist, then the pollen patty may be disregarded by the hive. Moreover, it is prone to hardening over time and could attract unwanted pests. Langstroth beehive image modified from Net Art under the Creative Commons Attribution 2.0 Generic License (https://netart.us/box-shaped-beehive-coloring-page/).
More commonly, beneficial bacteria are added to sucrose-based syrup solutions. Numerous studies have demonstrated that various bacteria supplemented in this manner can reduce Nosema ceranae loads (Baffoni et al., 2016), improve overwintering death rates (Kuzyšinová et al., 2016), and increase brood populations and harvestable honey by ∼46% and ∼60%, respectively (Alberoni et al., 2018). However, a critical factor limiting the practicality of this method in the field is the lacklustre viability and activity of bacteria in sucrose-based solutions (>90% drop in original CFU after 96 h at 30°C) due to osmotic stress (Ptaszyńska et al., 2016b). Additionally, this method of supplementation may not transfer bacteria to younger bees and larvae (Brodschneider and Crailsheim, 2010).
Another option is to infuse beneficial bacteria into pollen-substitute patties, which have the advantage of improving honey bee nutrition. Pollen-substitute patties per se have been shown to benefit honey bee health through reducing titers of deformed wing virus (DeGrandi-Hoffman et al., 2010) and increasing hemolymph protein content (Jong et al., 2009). Evaluating pollen substitutes as a delivery method, Kaznowski et al. (2005) demonstrated that hives supplemented with probiotic-infused pollen substitutes had better overall survival, higher dry mass, and increased crude fat levels of bees when compared to groups receiving only the pollen-substitute. Another study showed that honey bees receiving probiotic bacteria delivered via pollen-substitutes have better developed peritrophic membranes (responsible for nutrient utilization and pathogen protection) compared to vehicle controls (Szymaś et al., 2012). Some points to consider are that pollen-substitute patties may attract unwanted opportunistic insects (for example the small hive beetle, Aethina tumida) and it may not be consumed if other pollen sources exist. Nonetheless, pollen substitutes are already used by beekeepers to ensure nutritional adequacy and can be easily supplemented with beneficial bacteria.
Along with the introduction of any live microorganism to the hive comes the risk of inducing hive microbial dysbiosis (Alberoni et al., 2016). A few documented cases exist in which negative effects were observed from supplying honey bees with supplemental bacteria. Ptaszyńska et al. (2016b) reported that supplementation with L. rhamnosus (no strain type provided) increased honey bee susceptibility toward nosemosis C. In the same year, Ptaszyńska et al. (2016a) also demonstrated that co-administration with three LAB (Lactobacillus acidophilus, Lactobacillus delbrueckii, and Bifidobacterium bifidum—no strain designations provided) led to a decrease in total yeast concentrations in adult honey bee guts, but an increase in N. ceranae spores following infection. It is difficult to ascertain the biological relevance of these findings as crucial details are missing, including (1) strain-type information of lactobacilli used, (2) confirmation that live bacteria actually reached their target destination in the adult honey bee gut, and (3) whether or not the apparent increase in Nosema spp. led to any measurable changes in individual or hive-level health outcomes. Johnson et al. (2014) found no net positive or negative effect on hive health or performance following supplementation of lactobacilli in a high-fructose corn syrup vehicle. Altogether, these findings remind us to take precautions with biological agents, and stress the importance of properly considering strain type when selecting candidate lactobacilli for in-hive supplementation.
Concluding Remarks
Pesticide exposure at high doses is a notable causal factor involved in honey bee population decline; however, sublethal pesticide exposure presents inconspicuous threats to honey bees. In particular, it negatively affects reproduction, immunity, physiology, and cognition. Beginning at the reproductive cycle, sublethal exposure to pesticides impairs sexual reproduction, reduces egg-laying, and hinders larval development.
Sublethal pesticide exposure is broadly immunosuppressive and leads to increased levels of pathogens, as well as eliciting a biphasic response on movement and flight, which is mediated by severity of exposure. Acute exposure causes hyper-responsiveness and increased movement, while chronic exposure leads to hypo-responsiveness and reduced movement. Sublethal pesticides exposure causes numerous impairments to brain function, affecting harmony and productivity in the hive, and the ability to find new resources.
Despite the extensive knowledge of the sublethal effects of neonicotinoids, research on other pesticide classes is underrepresented, with a notable absence in work on fungicides and herbicides. Future studies should aim to methodically determine and report LD50 values for any pesticide tested, thereby ensuring that LD50 values are accurate and comparable throughout the world.
Bacteria are able to directly detoxify toxins and/or stimulate host detoxification, which could be advantageous in reducing pesticide uptake in honey bees. As the microbiota is affected by diet, this may potentially lead to the development of products that reduce pathogen burden by complementing the immune system through modification of the gut microbiota. In that regard, probiotic supplementation could mitigate the sublethal effects of pesticides by reducing pesticide uptake, improving pathogen resistance, and mitigating sublethal effects on colony development. Until chemical agents are no longer used in agriculture, the ability to supplement honey bees with probiotics could help the insects fight the unintended pernicious effects.
Author Contributions
JC, BD, AP, GT, and GR conceived the concept for the manuscript, contributed to the manuscript revisions, read, and approved the submitted version. JC drafted the manuscript and collected the toxicology data. BD wrote sections of the manuscript. AP generated the figures for the manuscript.
Funding
This work was funded by the Government of Canada Natural Sciences and Engineering Research Council of Canada (NSERC) and the Ontario Ministry of Agriculture, Food and Rural Affairs (OMAFRA).
Conflict of Interest
The authors declare that the research was conducted in the absence of any commercial or financial relationships that could be construed as a potential conflict of interest.
Supplementary Material
The Supplementary Material for this article can be found online at: https://www.frontiersin.org/articles/10.3389/fevo.2020.00022/full#supplementary-material
TABLE S1 | Complete adult and larval LD50 values for multiple pesticides.
References
Abbo, P. M., Kawasaki, J. K., Hamilton, M., Cook, S. C., DeGrandi-Hoffman, G., Li, W. F., et al. (2017). Effects of imidacloprid and Varroa destructor on survival and health of European honey bees. Apis mellifera. Insect Sci. 24, 467–477. doi: 10.1111/1744-7917.12335
Alaux, C., Brunet, J.-L., Dussaubat, C., Mondet, F., Tchamitchan, S., Cousin, M., et al. (2010a). Interactions between Nosema microspores and a neonicotinoid weaken honeybees (Apis mellifera). Environ. Microbiol. 12, 774–782. doi: 10.1111/j.1462-2920.2009.02123.x
Alaux, C., Ducloz, F., Crauser, D., and Le Conte, Y. (2010b). Diet effects on honeybee immunocompetence. Biol. Lett. 6, 562–565. doi: 10.1098/rsbl.2009.0986
Alberoni, D., Baffoni, L., Gaggìa, F., Ryan, P. M., Murphy, K., Ross, P. R., et al. (2018). Impact of beneficial bacteria supplementation on the gut microbiota, colony development and productivity of Apis mellifera L. Benef. Microbes 9, 269–278. doi: 10.3920/BM2017.0061
Alberoni, D., Gaggìa, F., Baffoni, L., and Di Gioia, D. (2016). Beneficial microorganisms for honey bees: problems and progresses. Appl. Microbiol. Biotechnol. 100, 9469–9482. doi: 10.1007/s00253-016-7870-4
Alburaki, M., Boutin, S., Mercier, P.-L., Loublier, Y., Chagnon, M., and Derome, N. (2015). Neonicotinoid-coated Zea mays seeds indirectly affect honeybee performance and pathogen susceptibility in field trials. PLoS One 10:e0125790. doi: 10.1371/journal.pone.0125790
Al-Ghamdi, A., Ali Khan, K., Javed Ansari, M., Almasaudi, S. B., and Al-Kahtani, S. (2017). Effect of gut bacterial isolates from Apis mellifera jemenitica on Paenibacillus larvae infected bee larvae. Saudi J. Biol. Sci. 25, 383–387. doi: 10.1016/j.sjbs.2017.07.005
Alippi, A. M., León, I. E., and López, A. C. (2014). Tetracycline-resistance encoding plasmids from Paenibacillus larvae, the causal agent of American foulbrood disease, isolated from commercial honeys. Int. Microbiol. Off. J. Span. Soc. Microbiol. 17, 49–61. doi: 10.2436/20.1501.01.207
Andrione, M., Vallortigara, G., Antolini, R., and Haase, A. (2016). Neonicotinoid-induced impairment of odour coding in the honeybee. Sci. Rep. 6:38110. doi: 10.1038/srep38110
Arredondo, D., Castelli, L., Porrini, M. P., Garrido, P. M., Eguaras, M. J., Zunino, P., et al. (2018). Lactobacillus kunkeei strains decreased the infection by honey bee pathogens Paenibacillus larvae and Nosema ceranae. Benef. Microbes 9, 279–290. doi: 10.3920/BM2017.0075
Audisio, M. C., Albarracín, L., Torres, M. J., Saavedra, L., Hebert, E. M., and Villena, J. (2018). Draft genome sequences of Lactobacillus salivarius A3iob and Lactobacillus johnsonii CRL1647, novel potential probiotic strains for honeybees (Apis mellifera L.). Microbiol. Resour. Announc. 7:e00975-18. doi: 10.1128/MRA.00975-18
Audisio, M. C., and Benítez-Ahrendts, M. R. (2011). Lactobacillus johnsonii CRL1647, isolated from Apis mellifera L. bee-gut, exhibited a beneficial effect on honeybee colonies. Benef. Microbes 2, 29–34. doi: 10.3920/BM2010.0024
Audisio, M. C., Sabaté, D. C., and Benítez-Ahrendts, M. R. (2015). Effect of Lactobacillus johnsonii CRL1647 on different parameters of honeybee colonies and bacterial populations of the bee gut. Benef. Microbes 6, 687–695. doi: 10.3920/BM2014.0155
Aufauvre, J., Misme-Aucouturier, B., Viguès, B., Texier, C., Delbac, F., and Blot, N. (2014). Transcriptome analyses of the honeybee response to Nosema ceranae and insecticides. PLoS One 9:e91686. doi: 10.1371/journal.pone.0091686
Baffoni, L., Gaggìa, F., Alberoni, D., Cabbri, R., Nanetti, A., Biavati, B., et al. (2016). Effect of dietary supplementation of Bifidobacterium and Lactobacillus strains in Apis mellifera L. against Nosema ceranae. Benef. Microbes 7, 45–51. doi: 10.3920/BM2015.0085
Bagherpour Shamloo, H., Golkari, S., Faghfoori, Z., Movassaghpour, A., Lotfi, H., Barzegari, A., et al. (2016). Lactobacillus casei decreases organophosphorus pesticide diazinon cytotoxicity in human HUVEC cell line. Adv. Pharm. Bull. 6, 201–210. doi: 10.15171/apb.2016.028
Baines, D., Wilton, E., Pawluk, A., de Gorter, M., and Chomistek, N. (2017). Neonicotinoids act like endocrine disrupting chemicals in newly-emerged bees and winter bees. Sci. Rep. 7:10979. doi: 10.1038/s41598-017-10489-6
Barker, R. J., and Lehner, Y. (1974). Acceptance and sustenance value of naturally occurring sugars fed to newly emerged adult workers of honey bees (Apis mellifera L.). J. Exp. Zool. 187, 277–285. doi: 10.1002/jez.1401870211
Berenbaum, M. R., and Johnson, R. M. (2015). Xenobiotic detoxification pathways in honey bees. Curr. Opin. Insect Sci. 10, 51–58. doi: 10.1016/j.cois.2015.03.005
Bevk, D., Kralj, J., and Čokl, A. (2012). Coumaphos affects food transfer between workers of honeybee Apis mellifera. Apidologie 43, 465–470. doi: 10.1007/s13592-011-0113-x
Blacquière, T., Smagghe, G., van Gestel, C. A. M., and Mommaerts, V. (2012). Neonicotinoids in bees: a review on concentrations, side-effects and risk assessment. Ecotoxicol. Lond. Engl. 21, 973–992. doi: 10.1007/s10646-012-0863-x
Blot, N., Veillat, L., Rouzé, R., and Delatte, H. (2019). Glyphosate, but not its metabolite AMPA, alters the honeybee gut microbiota. PLoS One 14:e0215466. doi: 10.1371/journal.pone.0215466
Boncristiani, H., Underwood, R., Schwarz, R., Evans, J. D., Pettis, J., and vanEngelsdorp, D. (2012). Direct effect of acaricides on pathogen loads and gene expression levels in honey bees Apis mellifera. J. Insect Physiol. 58, 613–620. doi: 10.1016/j.jinsphys.2011.12.011
Bonmatin, J.-M., Giorio, C., Girolami, V., Goulson, D., Kreutzweiser, D. P., Krupke, C., et al. (2015). Environmental fate and exposure; neonicotinoids and fipronil. Environ. Sci. Pollut. Res. Int. 22, 35–67. doi: 10.1007/s11356-014-3332-7
Bouhafs, L., Moudilou, E. N., Exbrayat, J. M., Lahouel, M., and Idoui, T. (2015). Protective effects of probiotic Lactobacillus plantarum BJ0021 on liver and kidney oxidative stress and apoptosis induced by endosulfan in pregnant rats. Ren. Fail. 37, 1370–1378. doi: 10.3109/0886022X.2015.1073543
Brandt, A., Gorenflo, A., Siede, R., Meixner, M., and Büchler, R. (2016). The neonicotinoids thiacloprid, imidacloprid, and clothianidin affect the immunocompetence of honey bees (Apis mellifera L.). J. Insect Physiol. 86, 40–47. doi: 10.1016/j.jinsphys.2016.01.001
Brandt, A., Grikscheit, K., Siede, R., Grosse, R., Meixner, M. D., and Büchler, R. (2017). Immunosuppression in honeybee queens by the neonicotinoids thiacloprid and clothianidin. Sci. Rep. 7:4673. doi: 10.1038/s41598-017-04734-1
Brodschneider, R., and Crailsheim, K. (2010). Nutrition and health in honey bees. Apidologie 41, 278–294. doi: 10.1051/apido/2010012
Brodschneider, R., Libor, A., Kupelwieser, V., and Crailsheim, K. (2017). Food consumption and food exchange of caged honey bees using a radioactive labelled sugar solution. PLoS One 12:e0174684. doi: 10.1371/journal.pone.0174684
Butler, È, Alsterfjord, M., Olofsson, T. C., Karlsson, C., Malmström, J., and Vásquez, A. (2013). Proteins of novel lactic acid bacteria from Apis mellifera mellifera: an insight into the production of known extra-cellular proteins during microbial stress. BMC Microbiol 13:235. doi: 10.1186/1471-2180-13-235
Buttstedt, A., Moritz, R. F. A., and Erler, S. (2014). Origin and function of the major royal jelly proteins of the honeybee (Apis mellifera) as members of the yellow gene family. Biol. Rev. Camb. Philos. Soc. 89, 255–269. doi: 10.1111/brv.12052
Calderone, N. W. (2012). Insect pollinated crops, insect pollinators and US agriculture: trend analysis of aggregate data for the period 1992–2009. PLoS One 7:e37235. doi: 10.1371/journal.pone.0037235
Carneiro, L. S., Martínez, L. C., Gonçalves, W. G., Santana, L. M., and Serrão, J. E. (2019). The fungicide iprodione affects midgut cells of non-target honey bee Apis mellifera workers. Ecotoxicol. Environ. Saf. 186:109991. doi: 10.1016/j.ecoenv.2019.109991
Chaimanee, V., Evans, J. D., Chen, Y., Jackson, C., and Pettis, J. S. (2016). Sperm viability and gene expression in honey bee queens (Apis mellifera) following exposure to the neonicotinoid insecticide imidacloprid and the organophosphate acaricide coumaphos. J. Insect Physiol. 89, 1–8. doi: 10.1016/j.jinsphys.2016.03.004
Chen, L., Yan, Q., Zhang, J., Yuan, S., and Liu, X. (2019). Joint toxicity of acetamiprid and co-applied pesticide adjuvants on honeybees under semifield and laboratory conditions. Environ. Toxicol. Chem. 38, 1940–1946. doi: 10.1002/etc.4515
Chmiel, J. A., Daisley, B. A., Burton, J. P., and Reid, G. (2019). Deleterious effects of neonicotinoid pesticides on Drosophila melanogaster immune pathways. mBio 10:e01395-19. doi: 10.1128/mBio.01395-19
Claudianos, C., Ranson, H., Johnson, R. M., Biswas, S., Schuler, M. A., Berenbaum, M. R., et al. (2006). A deficit of detoxification enzymes: pesticide sensitivity and environmental response in the honeybee. Insect Mol. Biol. 15, 615–636. doi: 10.1111/j.1365-2583.2006.00672.x
Clermont, A., Eickermann, M., Kraus, F., Hoffmann, L., and Beyer, M. (2015). Correlations between land covers and honey bee colony losses in a country with industrialized and rural regions. Sci. Total Environ. 532, 1–13. doi: 10.1016/j.scitotenv.2015.05.128
Corby-Harris, V., Snyder, L., Meador, C. A. D., Naldo, R., Mott, B., and Anderson, K. E. (2016). Parasaccharibacter apium, gen. nov., sp. nov., improves honey bee (Hymenoptera: Apidae) resistance to Nosema. J. Econ. Entomol 109, 537–543. doi: 10.1093/jee/tow012
Couvillon, M. J., Riddell Pearce, F. C., Accleton, C., Fensome, K. A., Quah, S. K. L., Taylor, E. L., et al. (2015). Honey bee foraging distance depends on month and forage type. Apidologie 46, 61–70. doi: 10.1007/s13592-014-0302-5
Cremer, S., Armitage, S. A. O., and Schmid-Hempel, P. (2007). Social immunity. Curr. Biol. 17, R693–R702. doi: 10.1016/j.cub.2007.06.008
Dai, P., Yan, Z., Ma, S., Yang, Y., Wang, Q., Hou, C., et al. (2018). The herbicide glyphosate negatively affects midgut bacterial communities and survival of honey bee during larvae reared in vitro. J. Agric. Food Chem. 66, 7786–7793. doi: 10.1021/acs.jafc.8b02212
Daisley, B. A., Pitek, A. P., Chmiel, J. A., Al, K. F., Chernyshova, A. M., and Faragalla, K. M. (2019). Novel probiotic approach to counter Paenibacillus larvae infection in honey bees. ISME J. 14, 476–491. doi: 10.1038/s41396-019-0541-6
Daisley, B. A., Reid, G., Leong, H. S., Welle, H., Dube, J. S., Trinder, M., et al. (2017). Neonicotinoid-induced pathogen susceptibility is mitigated by Lactobacillus plantarum immune stimulation in a Drosophila melanogaster model. Sci. Rep. 7:2703. doi: 10.1038/s41598-017-02806-w
Danihlík, J., Aronstein, K., and Petřivalský, M. (2015). Antimicrobial peptides: a key component of honey bee innate immunity. J. Apic. Res. 54, 123–136. doi: 10.1080/00218839.2015.1109919
de Mattos, I. M., Soares, A. E. E., and Tarpy, D. R. (2017). Effects of synthetic acaricides on honey bee grooming behavior against the parasitic Varroa destructor mite. Apidologie 48, 483–494. doi: 10.1007/s13592-017-0491-9
De Smet, L., Hatjina, F., Ioannidis, P., Hamamtzoglou, A., Schoonvaere, K., Francis, F., et al. (2017). Stress indicator gene expression profiles, colony dynamics and tissue development of honey bees exposed to sub-lethal doses of imidacloprid in laboratory and field experiments. PLoS One 12:e0171529. doi: 10.1371/journal.pone.0171529
Decourtye, A., Devillers, J., Cluzeau, S., Charreton, M., and Pham-Delègue, M.-H. (2004). Effects of imidacloprid and deltamethrin on associative learning in honeybees under semi-field and laboratory conditions. Ecotoxicol. Environ. Saf. 57, 410–419. doi: 10.1016/j.ecoenv.2003.08.001
DeGrandi-Hoffman, G., Chen, Y., Huang, E., and Huang, M. H. (2010). The effect of diet on protein concentration, hypopharyngeal gland development and virus load in worker honey bees (Apis mellifera L.). J. Insect Physiol. 56, 1184–1191. doi: 10.1016/j.jinsphys.2010.03.017
DeGrandi-Hoffman, G., Chen, Y., and Simonds, R. (2013). The effects of pesticides on queen rearing and virus titers in honey bees (Apis mellifera L.). Insects 4, 71–89. doi: 10.3390/insects4010071
Derecka, K., Blythe, M. J., Malla, S., Genereux, D. P., Guffanti, A., Pavan, P., et al. (2013). Transient exposure to low levels of insecticide affects metabolic networks of honeybee larvae. PLoS One 8:e68191. doi: 10.1371/journal.pone.0068191
Di Prisco, G., Cavaliere, V., Annoscia, D., Varricchio, P., Caprio, E., Nazzi, F., et al. (2013). Neonicotinoid clothianidin adversely affects insect immunity and promotes replication of a viral pathogen in honey bees. Proc. Natl. Acad. Sci. U.S.A. 110, 18466–18471. doi: 10.1073/pnas.1314923110
Doublet, V., Labarussias, M., de Miranda, J. R., Moritz, R. F. A., and Paxton, R. J. (2015). Bees under stress: sublethal doses of a neonicotinoid pesticide and pathogens interact to elevate honey bee mortality across the life cycle. Environ. Microbiol. 17, 969–983. doi: 10.1111/1462-2920.12426
Eiri, D. M., and Nieh, J. C. (2016). A nicotinic acetylcholine receptor agonist affects honey bee sucrose responsiveness and decreases waggle dancing. J. Exp. Biol. 219, 2022–2029. doi: 10.1242/jeb.143727
Ellegaard, K. M., Brochet, S., Bonilla-Rosso, G., Emery, O., Glover, N., Hadadi, N., et al. (2019). Genomic changes underlying host specialization in the bee gut symbiont Lactobacillus Firm5. Mol. Ecol. 28, 2224–2237. doi: 10.1111/mec.15075
Emery, O., Schmidt, K., and Engel, P. (2017). Immune system stimulation by the gut symbiont Frischella perrara in the honey bee (Apis mellifera). Mol. Ecol. 26, 2576–2590. doi: 10.1111/mec.14058
Engel, P., Martinson, V. G., and Moran, N. A. (2012). Functional diversity within the simple gut microbiota of the honey bee. Proc. Natl. Acad. Sci. U.S.A. 109, 11002–11007. doi: 10.1073/pnas.1202970109
Evans, J. D., and Armstrong, T.-N. (2005). Inhibition of the American foulbrood bacterium, Paenibacillus larvae larvae, by bacteria isolated from honey bees. J. Apic. Res. 44, 168–171. doi: 10.1080/00218839.2005.11101173
Evans, J. D., and Armstrong, T.-N. (2006). Antagonistic interactions between honey bee bacterial symbionts and implications for disease. BMC Ecol. 6:4. doi: 10.1186/1472-6785-6-4
Evans, J. D., Aronstein, K., Chen, Y. P., Hetru, C., Imler, J.-L., Jiang, H., et al. (2006). Immune pathways and defense mechanisms in honey bees Apis mellifera. Insect Mol. Biol. 15, 645–656. doi: 10.1111/j.1365-2583.2006.00682.x
Evans, J. D., and Lopez, D. L. (2004). Bacterial probiotics induce an immune response in the honey bee (Hymenoptera: Apidae). J. Econ. Entomol. 97, 752–756. doi: 10.1093/jee/97.3.752
Evans, J. D., and Schwarz, R. S. (2011). Bees brought to their knees: microbes affecting honey bee health. Trends Microbiol. 19, 614–620. doi: 10.1016/j.tim.2011.09.003
Fahrbach, S. E., and Robinson, G. E. (1996). Juvenile hormone, behavioral maturation, and brain structure in the honey bee. Dev. Neurosci. 18, 102–114. doi: 10.1159/000111474
Fairbrother, A., Purdy, J., Anderson, T., and Fell, R. (2014). Risks of neonicotinoid insecticides to honeybees. Environ. Toxicol. Chem. Setac 33, 719–731. doi: 10.1002/etc.2527
Fanciotti, M. N., Tejerina, M., Benítez-Ahrendts, M. R., and Audisio, M. C. (2018). Honey yield of different commercial apiaries treated with Lactobacillus salivarius A3iob, a new bee-probiotic strain. Benef. Microbes 9, 291–298. doi: 10.3920/BM2017.0089
Filannino, P., Di Cagno, R., Addante, R., Pontonio, E., and Gobbetti, M. (2016). Metabolism of fructophilic lactic acid bacteria isolated from the Apis mellifera L. bee gut: phenolic acids as external electron acceptors. Appl. Environ. Microbiol. 82, 6899–6911. doi: 10.1128/AEM.02194-16
Fine, J. D., Cox-Foster, D. L., and Mullin, C. A. (2017). An inert pesticide adjuvant synergizes viral pathogenicity and mortality in honey bee larvae. Sci. Rep. 7:40499. doi: 10.1038/srep40499
Fischer, J., Müller, T., Spatz, A.-K., Greggers, U., Grünewald, B., and Menzel, R. (2014). Neonicotinoids interfere with specific components of navigation in honeybees. PLoS One 9:e91364. doi: 10.1371/journal.pone.0091364
Forfert, N., Troxler, A., Retschnig, G., Gauthier, L., Straub, L., Moritz, R. F. A., et al. (2017). Neonicotinoid pesticides can reduce honeybee colony genetic diversity. PLoS One 12:e0186109. doi: 10.1371/journal.pone.0186109
Forkpah, C., Dixon, L. R., Fahrbach, S. E., and Rueppell, O. (2014). Xenobiotic effects on intestinal stem cell proliferation in adult honey bee (Apis mellifera L) workers. PLoS One 9:e91180. doi: 10.1371/journal.pone.0091180
Forsgren, E., Olofsson, T. C., Váasquez, A., and Fries, I. (2010). Novel lactic acid bacteria inhibiting Paenibacillus larvae in honey bee larvae. Apidologie 41, 99–108. doi: 10.1051/apido/2009065
Gajger, I. T., Sakač, M., and Gregorc, A. (2017). Impact of thiamethoxam on honey bee queen (Apis mellifera carnica) reproductive morphology and physiology. Bull. Environ. Contam. Toxicol. 99, 297–302. doi: 10.1007/s00128-017-2144-0
Garrido, P. M., Antúnez, K., Martín, M., Porrini, M. P., Zunino, P., and Eguaras, M. J. (2013). Immune-related gene expression in nurse honey bees (Apis mellifera) exposed to synthetic acaricides. J. Insect Physiol. 59, 113–119. doi: 10.1016/j.jinsphys.2012.10.019
Geoghegan, T. S., Kimberly, J. H., and Scheringer, M. (2013). “Predicting honeybee exposure to pesticides from vapour drift using a combined pesticide emission and atmospheric transport model,” in Proceedings of the Conference on SETAC Australasia – Multidisciplinary Approaches to Managing Environmental Pollution (Melbourne: SETAC Australasia), 174.
Gil, M., and De Marco, R. J. (2005). Olfactory learning by means of trophallaxis in Apis mellifera. J. Exp. Biol. 208, 671–680. doi: 10.1242/jeb.01474
Goñalons, C. M., and Farina, W. M. (2018). Impaired associative learning after chronic exposure to pesticides in young adult honey bees. J. Exp. Biol. 221:176644. doi: 10.1242/jeb.176644
Gong, Y., and Diao, Q. (2017). Current knowledge of detoxification mechanisms of xenobiotic in honey bees. Ecotoxicol. Lond. Engl. 26, 1–12. doi: 10.1007/s10646-016-1742-7
Goulson, D. (2013). An overview of the environmental risks posed by neonicotinoid insecticides. J. Appl. Ecol. 50, 977–987. doi: 10.1111/1365-2664.12111
Goulson, D., Nicholls, E., Botías, C., and Rotheray, E. L. (2015). Bee declines driven by combined stress from parasites, pesticides, and lack of flowers. Science 347:1255957. doi: 10.1126/science.1255957
Grassl, J., Holt, S., Cremen, N., Peso, M., Hahne, D., and Baer, B. (2018). Synergistic effects of pathogen and pesticide exposure on honey bee (Apis mellifera) survival and immunity. J. Invertebr. Pathol. 159, 78–86. doi: 10.1016/j.jip.2018.10.005
Gregorc, A., Alburaki, M., Rinderer, N., Sampson, B., Knight, P. R., Karim, S., et al. (2018). Effects of coumaphos and imidacloprid on honey bee (Hymenoptera: Apidae) lifespan and antioxidant gene regulations in laboratory experiments. Sci. Rep. 8:15003. doi: 10.1038/s41598-018-33348-4
Hagler, J. R., Mueller, S., Teuber, L. R., Machtley, S. A., and Van Deynze, A. (2011). Foraging range of honey bees, Apis mellifera, in alfalfa seed production fields. J. Insect Sci. 11:144. doi: 10.1673/031.011.14401
Han, P., Niu, C.-Y., Lei, C.-L., Cui, J.-J., and Desneux, N. (2010). Use of an innovative T-tube maze assay and the proboscis extension response assay to assess sublethal effects of GM products and pesticides on learning capacity of the honey bee Apis mellifera L. Ecotoxicol. Lond. Engl. 19, 1612–1619. doi: 10.1007/s10646-010-0546-4
Hatjina, F., Papaefthimiou, C., Charistos, L., Dogaroglu, T., Bouga, M., Emmanouil, C., et al. (2013). Sublethal doses of imidacloprid decreased size of hypopharyngeal glands and respiratory rhythm of honeybees in vivo. Apidologie 44, 467–480. doi: 10.1007/s13592-013-0199-4
Henry, M., Béguin, M., Requier, F., Rollin, O., Odoux, J.-F., Aupinel, P., et al. (2012). A common pesticide decreases foraging success and survival in honey bees. Science 336, 348–350. doi: 10.1126/science.1215039
Hesselbach, H., and Scheiner, R. (2018). Effects of the novel pesticide flupyradifurone (Sivanto) on honeybee taste and cognition. Sci. Rep. 8:4954. doi: 10.1038/s41598-018-23200-0
Hossard, L., Philibert, A., Bertrand, M., Colnenne-David, C., Debaeke, P., Munier-Jolain, N., et al. (2014). Effects of halving pesticide use on wheat production. Sci. Rep. 4:4405. doi: 10.1038/srep04405
Islam, S. M. A., Math, R. K., Cho, K. M., Lim, W. J., Hong, S. Y., Kim, J. M., et al. (2010). Organophosphorus hydrolase (OpdB) of Lactobacillus brevis WCP902 from kimchi is able to degrade organophosphorus pesticides. J. Agric. Food Chem. 58, 5380–5386. doi: 10.1021/jf903878e
Johnson, B. R., Synk, W., Jasper, W. C., and Müssen, E. (2014). Effects of high fructose corn syrup and probiotics on growth rates of newly founded honey bee colonies. J. Apic. Res. 53, 165–170. doi: 10.3896/IBRA.1.53.1.18
Johnson, R. M., Dahlgren, L., Siegfried, B. D., and Ellis, M. D. (2013). Acaricide, fungicide and drug interactions in honey bees (Apis mellifera). PLoS One 8:e54092. doi: 10.1371/journal.pone.0054092
Jones, J. C., Fruciano, C., Hildebrand, F., Al Toufalilia, H., Balfour, N. J., Bork, P., et al. (2017). Gut microbiota composition is associated with environmental landscape in honey bees. Ecol. Evol. 8, 441–451. doi: 10.1002/ece3.3597
Jones, J. C., Fruciano, C., Marchant, J., Hildebrand, F., Forslund, S., Bork, P., et al. (2018). The gut microbiome is associated with behavioural task in honey bees. Insectes Sociaux 65, 419–429. doi: 10.1007/s00040-018-0624-9
Jones, J. C., Myerscough, M. R., Graham, S., and Oldroyd, B. P. (2004). Honey bee nest thermoregulation: diversity promotes stability. Science 305, 402–404. doi: 10.1126/science.1096340
Jong, D. D., Silva, E. J., da Kevan, P. G., and Atkinson, J. L. (2009). Pollen substitutes increase honey bee haemolymph protein levels as much as or more than does pollen. J. Apic. Res. 48, 34–37. doi: 10.3896/IBRA.1.48.1.08
Kairo, G., Biron, D. G., Ben Abdelkader, F., Bonnet, M., Tchamitchian, S., Cousin, M., et al. (2017a). Nosema ceranae, fipronil and their combination compromise honey bee reproduction via changes in male physiology. Sci. Rep. 7:8556. doi: 10.1038/s41598-017-08380-5
Kairo, G., Poquet, Y., Haji, H., Tchamitchian, S., Cousin, M., Bonnet, M., et al. (2017b). Assessment of the toxic effect of pesticides on honey bee drone fertility using laboratory and semifield approaches: a case study of fipronil. Environ. Toxicol. Chem. 36, 2345–2351. doi: 10.1002/etc.3773
Kairo, G., Provost, B., Tchamitchian, S., Ben Abdelkader, F., Bonnet, M., Cousin, M., et al. (2016). Drone exposure to the systemic insecticide fipronil indirectly impairs queen reproductive potential. Sci. Rep. 6:31904. doi: 10.1038/srep31904
Kakumanu, M. L., Reeves, A. M., Anderson, T. D., Rodrigues, R. R., and Williams, M. A. (2016). Honey bee gut microbiome is altered by in-hive pesticide exposures. Front. Microbiol. 7:1255. doi: 10.3389/fmicb.2016.01255
Kaznowski, A., Szymas, B., Jazdzinska, E., Kazimierczak, M., Paetz, H., and Mokracka, J. (2005). The effects of probiotic supplementation on the content of intestinal microflora and chemical composition of worker honey bees (Apis mellifera). J. Apic. Res. 44, 10–14. doi: 10.1080/00218839.2005.11101139
Kešnerová, L., Mars, R. A. T., Ellegaard, K. M., Troilo, M., Sauer, U., and Engel, P. (2017). Disentangling metabolic functions of bacteria in the honey bee gut. PLoS Biol. 15:e2003467. doi: 10.1371/journal.pbio.2003467
Kessler, S., Tiedeken, E. J., Simcock, K. L., Derveau, S., Mitchell, J., Softley, S., et al. (2015). Bees prefer foods containing neonicotinoid pesticides. Nature 521, 74–76. doi: 10.1038/nature14414
Khaled, J. M., Al-Mekhlafi, F. A., Mothana, R. A., Alharbi, N. S., Alzaharni, K. E., Sharafaddin, A. H., et al. (2018). Brevibacillus laterosporus isolated from the digestive tract of honeybees has high antimicrobial activity and promotes growth and productivity of honeybee’s colonies. Environ. Sci. Pollut. Res. Int. 25, 10447–10455. doi: 10.1007/s11356-017-0071-6
Killer, J., Dubná, S., Sedláček, I., and Švec, P. (2014). Lactobacillus apis sp. nov., from the stomach of honeybees (Apis mellifera), having an in vitro inhibitory effect on the causative agents of American and European foulbrood. Int. J. Syst. Evol. Microbiol 64, 152–157. doi: 10.1099/ijs.0.053033-0
Koleoglu, G., Goodwin, P. H., Reyes-Quintana, M., Hamiduzzaman, M. M., and Guzman-Novoa, E. (2018). Varroa destructor parasitism reduces hemocyte concentrations and prophenol oxidase gene expression in bees from two populations. Parasitol. Res. 117, 1175–1183. doi: 10.1007/s00436-018-5796-8
Krongdang, S., Evans, J. D., Pettis, J. S., and Chantawannakul, P. (2017). Multilocus sequence typing, biochemical and antibiotic resistance characterizations reveal diversity of North American strains of the honey bee pathogen Paenibacillus larvae. PLoS One 12:e0176831. doi: 10.1371/journal.pone.0176831
Krupke, C. H., Hunt, G. J., Eitzer, B. D., Andino, G., and Given, K. (2012). Multiple routes of pesticide exposure for honey bees living near agricultural fields. PLoS One 7:e29268. doi: 10.1371/journal.pone.0029268
Kuzyšinová, K., Mudroňová, D., Toporčák, J., Molnár, L., and Javorský, P. (2016). The use of probiotics, essential oils and fatty acids in the control of American foulbrood and other bee diseases. J. Apic. Res. 55, 386–395. doi: 10.1080/00218839.2016.1252067
Kwong, W. K., Engel, P., Koch, H., and Moran, N. A. (2014). Genomics and host specialization of honey bee and bumble bee gut symbionts. Proc. Natl. Acad. Sci. U.S.A. 111, 11509–11514. doi: 10.1073/pnas.1405838111
Kwong, W. K., Mancenido, A. L., and Moran, N. A. (2017). Immune system stimulation by the native gut microbiota of honey bees. R. Soc. Open Sci. 4:170003. doi: 10.1098/rsos.170003
Le Goff, G., and Hilliou, F. (2017). Resistance evolution in Drosophila: the case of CYP6G1. Pest Manag. Sci. 73, 493–499. doi: 10.1002/ps.4470
Lechenet, M., Bretagnolle, V., Bockstaller, C., Boissinot, F., Petit, M.-S., Petit, S., et al. (2014). Reconciling pesticide reduction with economic and environmental sustainability in arable farming. PLoS One 9:e97922. doi: 10.1371/journal.pone.0097922
Lee, F. J., Rusch, D. B., Stewart, F. J., Mattila, H. R., and Newton, I. L. G. (2015). Saccharide breakdown and fermentation by the honey bee gut microbiome. Environ. Microbiol. 17, 796–815. doi: 10.1111/1462-2920.12526
Lénárt, J., Bujna, E., Kovács, B., Békefi, E., Száraz, L., and Dernovics, M. (2013). Metabolomic approach assisted high resolution LC-ESI-MS based identification of a xenobiotic derivative of fenhexamid produced by Lactobacillus casei. J. Agric. Food Chem. 61, 8969–8975. doi: 10.1021/jf4022493
Li, C., Ma, Y., Mi, Z., Huo, R., Zhou, T., Hai, H., et al. (2018). Screening for Lactobacillus plantarum strains that possess organophosphorus pesticide-degrading activity and metabolomic analysis of phorate degradation. Front. Microbiol. 9:2048. doi: 10.3389/fmicb.2018.02048
Li, J. H., Evans, J. D., Li, W. F., Zhao, Y. Z., De Grandi-Hoffman, G., Huang, S. K., et al. (2017). New evidence showing that the destruction of gut bacteria by antibiotic treatment could increase the honey bee’s vulnerability to Nosema infection. PLoS One 12:e0187505. doi: 10.1371/journal.pone.0187505
López, J. H., Krainer, S., Engert, A., Schuehly, W., Riessberger-Gallé, U., and Crailsheim, K. (2017). Sublethal pesticide doses negatively affect survival and the cellular responses in American foulbrood-infected honeybee larvae. Sci. Rep. 7:40853. doi: 10.1038/srep40853
Lu, C., Chang, C.-H., Palmer, C., Zhao, M., and Zhang, Q. (2018). Neonicotinoid residues in fruits and vegetables: an integrated dietary exposure assessment approach. Environ. Sci. Technol. 52, 3175–3184. doi: 10.1021/acs.est.7b05596
Maes, P. W., Rodrigues, P. A. P., Oliver, R., Mott, B. M., and Anderson, K. E. (2016). Diet-related gut bacterial dysbiosis correlates with impaired development, increased mortality and Nosema disease in the honeybee (Apis mellifera). Mol. Ecol. 25, 5439–5450. doi: 10.1111/mec.13862
Maggi, M., Negri, P., Plischuk, S., Szawarski, N., De Piano, F., De Feudis, L., et al. (2013). Effects of the organic acids produced by a lactic acid bacterium in Apis mellifera colony development, Nosema ceranae control and fumagillin efficiency. Vet. Microbiol. 167, 474–483. doi: 10.1016/j.vetmic.2013.07.030
Makhijani, K., Alexander, B., Rao, D., Petraki, S., Herboso, L., Kukar, K., et al. (2017). Regulation of Drosophila hematopoietic sites by Activin-β from active sensory neurons. Nat. Commun. 8:15990. doi: 10.1038/ncomms15990
Mao, W., Schuler, M. A., and Berenbaum, M. R. (2013). Honey constituents up-regulate detoxification and immunity genes in the western honey bee Apis mellifera. Proc. Natl. Acad. Sci. U.S.A. 110, 8842–8846. doi: 10.1073/pnas.1303884110
Mao, W., Schuler, M. A., and Berenbaum, M. R. (2017). Disruption of quercetin metabolism by fungicide affects energy production in honey bees (Apis mellifera). Proc. Natl. Acad. Sci. U.S.A. 114, 2538–2543. doi: 10.1073/pnas.1614864114
Maori, E., Navarro, I. C., Boncristiani, H., Seilly, D. J., Rudolph, K. L. M., Sapetschnig, A., et al. (2019). A secreted RNA binding protein forms RNA-stabilizing granules in the honeybee royal jelly. Mol. Cell 74, 598–608.e6. doi: 10.1016/j.molcel.2019.03.010
Mattila, H. R., Rios, D., Walker-Sperling, V. E., Roeselers, G., and Newton, I. L. G. (2012). Characterization of the active microbiotas associated with honey bees reveals healthier and broader communities when colonies are genetically diverse. PLoS One 7:e32962. doi: 10.1371/journal.pone.0032962
McArt, S. H., Fersch, A. A., Milano, N. J., Truitt, L. L., and Böröczky, K. (2017). High pesticide risk to honey bees despite low focal crop pollen collection during pollination of a mass blooming crop. Sci. Rep. 7:46554. doi: 10.1038/srep46554
Mitchell, E. A. D., Mulhauser, B., Mulot, M., Mutabazi, A., Glauser, G., and Aebi, A. (2017). A worldwide survey of neonicotinoids in honey. Science 358, 109–111. doi: 10.1126/science.aan3684
Motta, E. V. S., Raymann, K., and Moran, N. A. (2018). Glyphosate perturbs the gut microbiota of honey bees. Proc. Natl. Acad. Sci. U.S.A. 115, 10305–10310. doi: 10.1073/pnas.1803880115
Mukezangango, J., and Page, S. (2017). Statistical Overview of the Canadian Honey and Bee Industry and the Economic Contribution of Honey Bee Pollination, 2016. Available at: https://www5.agr.gc.ca/eng/industry-markets-and-trade/canadian-agri-food-sector-intelligence/horticulture/horticulture-sector-reports/statistical-overview-of-the-canadian-honey-and-bee-industry- and-the-economic-contribution-of-honey-bee-pollination-2016/?id=15108649 70935 (accessed October 21, 2018).
Mutti, N. S., Dolezal, A. G., Wolschin, F., Mutti, J. S., Gill, K. S., and Amdam, G. V. (2011). IRS and TOR nutrient-signaling pathways act via juvenile hormone to influence honey bee caste fate. J. Exp. Biol. 214, 3977–3984. doi: 10.1242/jeb.061499
Nelson, C., Ambros, V., and Baehrecke, E. H. (2014). miR-14 regulates autophagy during developmental cell death by targeting ip3-kinase 2. Mol. Cell 56, 376–388. doi: 10.1016/j.molcel.2014.09.011
Nelson, C. M., Ihle, K. E., Fondrk, M. K. Jr., Page, R. E., and Amdam, G. V. (2007). The gene Vitellogenin has multiple coordinating effects on social organization. PLoS Biol. 5:e62. doi: 10.1371/journal.pbio.0050062
Nicodemo, D., Maioli, M. A., Medeiros, H. C. D., Guelfi, M., Balieira, K. V. B., De Jong, D., et al. (2014). Fipronil and imidacloprid reduce honeybee mitochondrial activity. Environ. Toxicol. Chem. 33, 2070–2075. doi: 10.1002/etc.2655
Olofsson, T. C., Butler, È, Markowicz, P., Lindholm, C., Larsson, L., and Vásquez, A. (2016). Lactic acid bacterial symbionts in honeybees – an unknown key to honey’s antimicrobial and therapeutic activities. Int. Wound J. 13, 668–679. doi: 10.1111/iwj.12345
O’Neal, S. T., Reeves, A. M., Fell, R. D., Brewster, C. C., and Anderson, T. D. (2019). Chlorothalonil exposure alters virus susceptibility and markers of immunity, nutrition, and development in honey bees. J. Insect Sci. 19:14. doi: 10.1093/jisesa/iez051
Palmer, M. J., Moffat, C., Saranzewa, N., Harvey, J., Wright, G. A., and Connolly, C. N. (2013). Cholinergic pesticides cause mushroom body neuronal inactivation in honeybees. Nat. Commun. 4:1634. doi: 10.1038/ncomms2648
Paris, L., Roussel, M., Pereira, B., Delbac, F., and Diogon, M. (2017). Disruption of oxidative balance in the gut of the western honeybee Apis mellifera exposed to the intracellular parasite Nosema ceranae and to the insecticide fipronil. Microb. Biotechnol. 10, 1702–1717. doi: 10.1111/1751-7915.12772
Park, M. G., Blitzer, E. J., Gibbs, J., Losey, J. E., and Danforth, B. N. (2015). Negative effects of pesticides on wild bee communities can be buffered by landscape context. Proc. R. Soc. B Biol. Sci. 282:20150299. doi: 10.1098/rspb.2015.0299
Pettis, J. S., Lichtenberg, E. M., Andree, M., Stitzinger, J., Rose, R., and vanEngelsdorp, D. (2013). Crop pollination exposes honey bees to pesticides which alters their susceptibility to the gut pathogen Nosema ceranae. PLoS One 8:e70182. doi: 10.1371/journal.pone.0070182
Pettis, J. S., vanEngelsdorp, D., Johnson, J., and Dively, G. (2012). Pesticide exposure in honey bees results in increased levels of the gut pathogen Nosema. Naturwissenschaften 99, 153–158. doi: 10.1007/s00114-011-0881-1
Pietri, J. E., and Liang, D. (2018). The links between insect symbionts and insecticide resistance: causal relationships and physiological tradeoffs. Ann. Entomol. Soc. Am. 111, 92–97. doi: 10.1093/aesa/say009
Plath, J. A., Entler, B. V., Kirkerud, N. H., Schlegel, U., Galizia, C. G., and Barron, A. B. (2017). Different roles for honey bee mushroom bodies and central complex in visual learning of colored lights in an aversive conditioning assay. Front. Behav. Neurosci. 11:98. doi: 10.3389/fnbeh.2017.00098
Poquet, Y., Bodin, L., Tchamitchian, M., Fusellier, M., Giroud, B., Lafay, F., et al. (2014). A pragmatic approach to assess the exposure of the honey bee (Apis mellifera) when subjected to pesticide spray. PLoS One 9:e113728. doi: 10.1371/journal.pone.0113728
Powell, J. E., Martinson, V. G., Urban-Mead, K., and Moran, N. A. (2014). Routes of acquisition of the gut microbiota of the honey bee Apis mellifera. Appl. Environ. Microbiol. 80, 7378–7387. doi: 10.1128/AEM.01861-14
Powner, M. B., Salt, T. E., Hogg, C., and Jeffery, G. (2016). Improving mitochondrial function protects bumblebees from neonicotinoid pesticides. PLoS One 11:e0166531. doi: 10.1371/journal.pone.0166531
Ptaszyńska, A. A., Borsuk, G., Mułenko, W., and Wilk, J. (2016a). Impact of vertebrate probiotics on honeybee yeast microbiota and on the course of nosemosis. Med. Weter 72, 430–434. doi: 10.21521/mw.5534
Ptaszyńska, A. A., Borsuk, G., Zdybicka-Barabas, A., Cytryńska, M., and Małek, W. (2016b). Are commercial probiotics and prebiotics effective in the treatment and prevention of honeybee nosemosis C? Parasitol. Res. 115, 397–406. doi: 10.1007/s00436-015-4761-z
Qi, Y., Huang, J., Li, M., Wu, Y., Xia, R., and Ye, G. (2016). Serotonin modulates insect hemocyte phagocytosis via two different serotonin receptors. eLife 5:e12241. doi: 10.7554/eLife.12241
Raymann, K., Bobay, L.-M., and Moran, N. A. (2018a). Antibiotics reduce genetic diversity of core species in the honeybee gut microbiome. Mol. Ecol. 27, 2057–2066. doi: 10.1111/mec.14434
Raymann, K., Motta, E. V. S., Girard, C., Riddington, I. M., Dinser, J. A., and Moran, N. A. (2018b). Imidacloprid decreases honey bee survival rates but does not affect the gut microbiome. Appl. Environ. Microbiol. 84:e00545-18. doi: 10.1128/AEM.00545-18
Raymann, K., Shaffer, Z., and Moran, N. A. (2017). Antibiotic exposure perturbs the gut microbiota and elevates mortality in honeybees. PLoS Biol. 15:e2001861. doi: 10.1371/journal.pbio.2001861
Reeves, A. M., O’Neal, S. T., Fell, R. D., Brewster, C. C., and Anderson, T. D. (2018). In-hive acaricides alter biochemical and morphological indicators of honey bee nutrition, immunity, and development. J. Insect Sci. Online 18, 1–6. doi: 10.1093/jisesa/iey086
Renzi, M. T., Amichot, M., Pauron, D., Tchamitchian, S., Brunet, J.-L., Kretzschmar, A., et al. (2016a). Chronic toxicity and physiological changes induced in the honey bee by the exposure to fipronil and Bacillus thuringiensis spores alone or combined. Ecotoxicol. Environ. Saf. 127, 205–213. doi: 10.1016/j.ecoenv.2016.01.028
Renzi, M. T., Rodríguez-Gasol, N., Medrzycki, P., Porrini, C., Martini, A., Burgio, G., et al. (2016b). Combined effect of pollen quality and thiamethoxam on hypopharyngeal gland development and protein content in Apis mellifera. Apidologie 47, 779–788. doi: 10.1007/s13592-016-0435-9
Ribière, C., Hegarty, C., Stephenson, H., Whelan, P., and O’Toole, P. W. (2019). Gut and whole-body microbiota of the honey bee separate thriving and non-thriving hives. Microb. Ecol. 78, 195–205. doi: 10.1007/s00248-018-1287-9
Rinkevich, F. D., Margotta, J. W., Pittman, J. M., Danka, R. G., Tarver, M. R., Ottea, J. A., et al. (2015). Genetics, synergists, and age affect insecticide sensitivity of the honey bee, Apis mellifera. PLoS One 10:e0139841. doi: 10.1371/journal.pone.0139841
Roat, T. C., dos Santos-Pinto, J. R. A., Dos Santos, L. D., Santos, K. S., Malaspina, O., and Palma, M. S. (2014). Modification of the brain proteome of Africanized honeybees (Apis mellifera) exposed to a sub-lethal doses of the insecticide fipronil. Ecotoxicol. Lond. Engl. 23, 1659–1670. doi: 10.1007/s10646-014-1305-8
Robinson, G. E., Page, R. E., Strambi, C., and Strambi, A. (1989). Hormonal and genetic control of behavioral integration in honey bee colonies. Science 246, 109–112. doi: 10.1126/science.246.4926.109
Rouzé, R., Moné, A., Delbac, F., Belzunces, L., and Blot, N. (2019). The honeybee gut microbiota is altered after chronic exposure to different families of insecticides and infection by Nosema ceranae. Microbes Environ. 34, 226–233. doi: 10.1264/jsme2.ME18169
Rubanov, A., Russell, K. A., Rothman, J. A., Nieh, J. C., and McFrederick, Q. S. (2019). Intensity of Nosema ceranae infection is associated with specific honey bee gut bacteria and weakly associated with gut microbiome structure. Sci. Rep. 9:3820. doi: 10.1038/s41598-019-40347-6
Sabaté, D. C., Cruz, M. S., Benítez-Ahrendts, M. R., and Audisio, M. C. (2012). Beneficial effects of Bacillus subtilis subsp. subtilis Mori2, a honey-associated strain, on honeybee colony performance. Probiotics Antimicrob. Proteins 4, 39–46. doi: 10.1007/s12602-011-9089-0
Sadd, B. M., Barribeau, S. M., Bloch, G., de Graaf, D. C., Dearden, P., Elsik, C. G., et al. (2015). The genomes of two key bumblebee species with primitive eusocial organization. Genome Biol. 16:76. doi: 10.1186/s13059-015-0623-3
Samson-Robert, O., Labrie, G., Chagnon, M., and Fournier, V. (2017). Planting of neonicotinoid-coated corn raises honey bee mortality and sets back colony development. PeerJ 5:e3670. doi: 10.7717/peerj.3670
Sanchez-Bayo, F., and Goka, K. (2014). Pesticide residues and bees - a risk assessment. PLoS One 9:e94482. doi: 10.1371/journal.pone.0094482
Sandrock, C., Tanadini, M., Tanadini, L. G., Fauser-Misslin, A., Potts, S. G., and Neumann, P. (2014). Impact of chronic neonicotinoid exposure on honeybee colony performance and queen supersedure. PLoS One 9:e103592. doi: 10.1371/journal.pone.0103592
Schmehl, D. R., Teal, P. E. A., Frazier, J. L., and Grozinger, C. M. (2014). Genomic analysis of the interaction between pesticide exposure and nutrition in honey bees (Apis mellifera). J. Insect Physiol. 71, 177–190. doi: 10.1016/j.jinsphys.2014.10.002
Schwarz, R. S., Moran, N. A., and Evans, J. D. (2016). Early gut colonizers shape parasite susceptibility and microbiota composition in honey bee workers. Proc. Natl. Acad. Sci. U.S.A. 113, 9345–9350. doi: 10.1073/pnas.1606631113
Sherman, P. W., Seeley, T. D., and Reeve, H. K. (1988). Parasites, pathogens, and polyandry in social hymenoptera. Am. Nat. 131, 602–610. doi: 10.1086/284809
Shi, T.-F., Wang, Y.-F., Liu, F., Qi, L., and Yu, L.-S. (2017a). Influence of the neonicotinoid insecticide thiamethoxam on miRNA expression in the honey bee (Hymenoptera: Apidae). J. Insect Sci. Online 17:96. doi: 10.1093/jisesa/iex074
Shi, T.-F., Wang, Y.-F., Liu, F., Qi, L., and Yu, L.-S. (2017b). Sublethal effects of the neonicotinoid insecticide thiamethoxam on the transcriptome of the honey bees (Hymenoptera: Apidae). J. Econ. Entomol. 110, 2283–2289. doi: 10.1093/jee/tox262
Simon-Delso, N., San Martin, G., Bruneau, E., Delcourt, C., and Hautier, L. (2017). The challenges of predicting pesticide exposure of honey bees at landscape level. Sci. Rep. 7:3801. doi: 10.1038/s41598-017-03467-5
Stoner, K. A., and Eitzer, B. D. (2013). Using a hazard quotient to evaluate pesticide residues detected in pollen trapped from honey bees (Apis mellifera) in Connecticut. PLoS One 8:e77550. doi: 10.1371/journal.pone.0077550
Straub, L., Villamar-Bouza, L., Bruckner, S., Chantawannakul, P., Gauthier, L., Khongphinitbunjong, K., et al. (2016). Neonicotinoid insecticides can serve as inadvertent insect contraceptives. Proc. R. Soc. B Biol. Sci. 283:20160506. doi: 10.1098/rspb.2016.0506
Straub, L., Williams, G. R., Vidondo, B., Khongphinitbunjong, K., Retschnig, G., Schneeberger, A., et al. (2019). Neonicotinoids and ectoparasitic mites synergistically impact honeybees. Sci. Rep. 9:8159. doi: 10.1038/s41598-019-44207-1
Suchail, S., Guez, D., and Belzunces, L. P. (2001). Discrepancy between acute and chronic toxicity induced by imidacloprid and its metabolites in Apis mellifera. Environ. Toxicol. Chem. 20, 2482–2486. doi: 10.1002/etc.5620201113
Szymaś, B., Łangowska, A., and Kazimierczak-Baryczko, M. (2012). Histological structure of the midgut of honey bees (Apis mellifera L.) fed pollen substitutes fortified with probiotics. J. Apic. Sci. 56, 5–12. doi: 10.2478/v10289-012-0001-2
Tan, K., Chen, W., Dong, S., Liu, X., Wang, Y., and Nieh, J. C. (2014). Imidacloprid alters foraging and decreases bee avoidance of predators. PLoS One 9:e102725. doi: 10.1371/journal.pone.0102725
Tavares, D. A., Roat, T. C., Carvalho, S. M., Silva-Zacarin, E. C. M., and Malaspina, O. (2015). In vitro effects of thiamethoxam on larvae of Africanized honey bee Apis mellifera (Hymenoptera: Apidae). Chemosphere 135, 370–378. doi: 10.1016/j.chemosphere.2015.04.090
Tesovnik, T., Cizelj, I., Zorc, M., Čitar, M., Božič, J., Glavan, G., et al. (2017). Immune related gene expression in worker honey bee (Apis mellifera carnica) pupae exposed to neonicotinoid thiamethoxam and Varroa mites (Varroa destructor). PLoS One 12:e0187079. doi: 10.1371/journal.pone.0187079
Tesovnik, T., Zorc, M., Ristanić, M., Glavinić, U., Stevanović, J., Narat, M., et al. (2019). Exposure of honey bee larvae to thiamethoxam and its interaction with Nosema ceranae infection in adult honey bees. Environ. Pollut. 256:113443. doi: 10.1016/j.envpol.2019.113443
Tison, L., Hahn, M.-L., Holtz, S., Rößner, A., Greggers, U., Bischoff, G., et al. (2016). Honey bees’ behavior is impaired by chronic exposure to the neonicotinoid thiacloprid in the field. Environ. Sci. Technol. 50, 7218–7227. doi: 10.1021/acs.est.6b02658
Tosi, S., Burgio, G., and Nieh, J. C. (2017a). A common neonicotinoid pesticide, thiamethoxam, impairs honey bee flight ability. Sci. Rep. 7:1201. doi: 10.1038/s41598-017-01361-8
Tosi, S., and Nieh, J. C. (2019). Lethal and sublethal synergistic effects of a new systemic pesticide, flupyradifurone (Sivanto®), on honeybees. Proc. R. Soc. B Biol. Sci. 286:20190433. doi: 10.1098/rspb.2019.0433
Tosi, S., Nieh, J. C., Sgolastra, F., Cabbri, R., and Medrzycki, P. (2017b). Neonicotinoid pesticides and nutritional stress synergistically reduce survival in honey bees. Proc. R. Soc. B Biol. Sci. 284:20171711. doi: 10.1098/rspb.2017.1711
Trinder, M., McDowell, T. W., Daisley, B. A., Ali, S. N., Leong, H. S., Sumarah, M. W., et al. (2016). Probiotic Lactobacillus rhamnosus reduces organophosphate pesticide absorption and toxicity to Drosophila melanogaster. Appl. Environ. Microbiol. 82, 6204–6213. doi: 10.1128/AEM.01510-16
Tsvetkov, N., Samson-Robert, O., Sood, K., Patel, H. S., Malena, D. A., Gajiwala, P. H., et al. (2017). Chronic exposure to neonicotinoids reduces honey bee health near corn crops. Science 356, 1395–1397. doi: 10.1126/science.aam7470
Vannette, R. L., Mohamed, A., and Johnson, B. R. (2015). Forager bees (Apis mellifera) highly express immune and detoxification genes in tissues associated with nectar processing. Sci. Rep. 5:16224. doi: 10.1038/srep16224
Varghese, J., Lim, S. F., and Cohen, S. M. (2010). Drosophila miR-14 regulates insulin production and metabolism through its target, sugarbabe. Genes Dev. 24, 2748–2753. doi: 10.1101/gad.1995910
Vásquez, A., Forsgren, E., Fries, I., Paxton, R. J., Flaberg, E., Szekely, L., et al. (2012). Symbionts as major modulators of insect health: lactic acid bacteria and honeybees. PLoS One 7:e33188. doi: 10.1371/journal.pone.0033188
Vázquez, D. E., Ilina, N., Pagano, E. A., Zavala, J. A., and Farina, W. M. (2018). Glyphosate affects the larval development of honey bees depending on the susceptibility of colonies. PLoS One 13:e0205074. doi: 10.1371/journal.pone.0205074
Vidau, C., Diogon, M., Aufauvre, J., Fontbonne, R., Viguès, B., Brunet, J.-L., et al. (2011). Exposure to sublethal doses of fipronil and thiacloprid highly increases mortality of honeybees previously infected by Nosema ceranae. PLoS One 6:e21550. doi: 10.1371/journal.pone.0021550
Villa, S., Vighi, M., Finizio, A., and Bolchi Serini, G. (2000). Risk assessment for honeybees from pesticide-exposed pollen. Ecotoxicology 9, 287–297. doi: 10.1023/A:1026522112328
Wade, A., Lin, C.-H., Kurkul, C., Regan, E. R., and Johnson, R. M. (2019). Combined toxicity of insecticides and fungicides applied to California almond orchards to honey bee larvae and adults. Insects 10:20. doi: 10.3390/insects10010020
Walderdorff, L., Laval-Gilly, P., Bonnefoy, A., and Falla-Angel, J. (2018). Imidacloprid intensifies its impact on honeybee and bumblebee cellular immune response when challenged with LPS (lippopolysacharide) of Escherichia coli. J. Insect Physiol. 108, 17–24. doi: 10.1016/j.jinsphys.2018.05.002
Whitfield, C. W., Behura, S. K., Berlocher, S. H., Clark, A. G., Johnston, J. S., Sheppard, W. S., et al. (2006). Thrice out of Africa: ancient and recent expansions of the honey bee, Apis mellifera. Science 314, 642–645. doi: 10.1126/science.1132772
Wilkins, S., Jarratt, N., Harkin, S., Thompson, H., and Coulson, M. (2013). Effects of solvent on the toxicity of dimethoate in a honey bee in vitro larval study. Pest Manag. Sci. 69, 462–463. doi: 10.1002/ps.3465
Williams, G. R., Troxler, A., Retschnig, G., Roth, K., Yañez, O., Shutler, D., et al. (2015). Neonicotinoid pesticides severely affect honey bee queens. Sci. Rep. 5:14621. doi: 10.1038/srep14621
Williamson, S. M., Moffat, C., Gomersall, M. A. E., Saranzewa, N., Connolly, C. N., and Wright, G. A. (2013). Exposure to acetylcholinesterase inhibitors alters the physiology and motor function of honeybees. Front. Physiol. 4:13. doi: 10.3389/fphys.2013.00013
Williamson, S. M., Willis, S. J., and Wright, G. A. (2014). Exposure to neonicotinoids influences the motor function of adult worker honeybees. Ecotoxicol. Lond. Engl. 23, 1409–1418. doi: 10.1007/s10646-014-1283-x
Williamson, S. M., and Wright, G. A. (2013). Exposure to multiple cholinergic pesticides impairs olfactory learning and memory in honeybees. J. Exp. Biol. 216, 1799–1807. doi: 10.1242/jeb.083931
Wright, G. A., Softley, S., and Earnshaw, H. (2015). Low doses of neonicotinoid pesticides in food rewards impair short-term olfactory memory in foraging-age honeybees. Sci. Rep. 5:15322. doi: 10.1038/srep15322
Wu, J. Y., Anelli, C. M., and Sheppard, W. S. (2011). Sub-lethal effects of pesticide residues in brood comb on worker honey bee (Apis mellifera) development and longevity. PLoS One 6:e14720. doi: 10.1371/journal.pone.0014720
Wu, J. Y., Smart, M. D., Anelli, C. M., and Sheppard, W. S. (2012). Honey bees (Apis mellifera) reared in brood combs containing high levels of pesticide residues exhibit increased susceptibility to Nosema (Microsporidia) infection. J. Invertebr. Pathol. 109, 326–329. doi: 10.1016/j.jip.2012.01.005
Wu, M.-C., Chang, Y.-W., Lu, K.-H., and Yang, E.-C. (2017). Gene expression changes in honey bees induced by sublethal imidacloprid exposure during the larval stage. Insect Biochem. Mol. Biol. 88, 12–20. doi: 10.1016/j.ibmb.2017.06.016
Wu, S.-F., Xu, G., Stanley, D., Huang, J., and Ye, G.-Y. (2015). Dopamine modulates hemocyte phagocytosis via a D1-like receptor in the rice stem borer, Chilo suppressalis. Sci. Rep. 5:12247. doi: 10.1038/srep12247
Wu-Smart, J., and Spivak, M. (2016). Sub-lethal effects of dietary neonicotinoid insecticide exposure on honey bee queen fecundity and colony development. Sci. Rep. 6:32108. doi: 10.1038/srep32108
Yang, E. C., Chuang, Y. C., Chen, Y. L., and Chang, L. H. (2008). Abnormal foraging behavior induced by sublethal dosage of imidacloprid in the honey bee (Hymenoptera: Apidae). J. Econ. Entomol. 101, 1743–1748. doi: 10.1603/0022-0493-101.6.1743
Yang, W., Tian, Y., Han, M., and Miao, X. (2017). Longevity extension of worker honey bees (Apis mellifera) by royal jelly: optimal dose and active ingredient. PeerJ 5:e3118. doi: 10.7717/peerj.3118
Youngsteadt, E., Appler, R. H., López-Uribe, M. M., Tarpy, D. R., and Frank, S. D. (2015). Urbanization increases pathogen pressure on feral and managed honey bees. PLoS One 10:e0142031. doi: 10.1371/journal.pone.0142031
Zaluski, R., Justulin, L. A., Orsi, R., and de, O. (2017). Field-relevant doses of the systemic insecticide fipronil and fungicide pyraclostrobin impair mandibular and hypopharyngeal glands in nurse honeybees (Apis mellifera). Sci. Rep. 7:15217. doi: 10.1038/s41598-017-15581-5
Zhang, Y.-H., Xu, D., Zhao, X.-H., Song, Y., Liu, Y.-L., and Li, H.-N. (2016). Biodegradation of two organophosphorus pesticides in whole corn silage as affected by the cultured Lactobacillus plantarum. 3 Biotech 6:73. doi: 10.1007/s13205-016-0364-3
Zheng, H., Nishida, A., Kwong, W. K., Koch, H., Engel, P., and Steele, I. (2016). Metabolism of toxic sugars by strains of the bee gut symbiont Gilliamella apicola. mBio 7:e01326-16. doi: 10.1128/mBio.01326-16
Zheng, H., Powell, J. E., Steele, M. I., Dietrich, C., and Moran, N. A. (2017). Honeybee gut microbiota promotes host weight gain via bacterial metabolism and hormonal signaling. Proc. Natl. Acad. Sci. U.S.A. 114, 4775–4780. doi: 10.1073/pnas.1701819114
Zhu, W., Schmehl, D. R., Mullin, C. A., and Frazier, J. L. (2014). Four common pesticides, their mixtures and a formulation solvent in the hive environment have high oral toxicity to honey bee larvae. PLoS One 9:e77547. doi: 10.1371/journal.pone.0077547
Zhu, Y. C., Adamczyk, J., Rinderer, T., Yao, J., Danka, R., Luttrell, R., et al. (2015). Spray toxicity and risk potential of 42 commonly used formulations of row crop pesticides to adult honey bees (Hymenoptera: Apidae). J. Econ. Entomol. 108, 2640–2647. doi: 10.1093/jee/tov269
Keywords: honey bee, pesticide, toxicology, immune, development, cognition, probiotic, LD50 (median lethal dose)
Citation: Chmiel JA, Daisley BA, Pitek AP, Thompson GJ and Reid G (2020) Understanding the Effects of Sublethal Pesticide Exposure on Honey Bees: A Role for Probiotics as Mediators of Environmental Stress. Front. Ecol. Evol. 8:22. doi: 10.3389/fevo.2020.00022
Received: 26 August 2019; Accepted: 27 January 2020;
Published: 19 February 2020.
Edited by:
William G. Meikle, Agricultural Research Service, United States Department of Agriculture, United StatesReviewed by:
Vincent Ricigliano, Honey Bee Breeding, Genetics, and Physiology Research (USDA-ARS), United StatesMark J. Carroll, Carl Hayden Bee Research Center, USDA-ARS, United States
Copyright © 2020 Chmiel, Daisley, Pitek, Thompson and Reid. This is an open-access article distributed under the terms of the Creative Commons Attribution License (CC BY). The use, distribution or reproduction in other forums is permitted, provided the original author(s) and the copyright owner(s) are credited and that the original publication in this journal is cited, in accordance with accepted academic practice. No use, distribution or reproduction is permitted which does not comply with these terms.
*Correspondence: Gregor Reid, Z3JlZ29yQHV3by5jYQ==