- 1Department of Ecology, Universidade Federal Do Rio Grande Do Norte, Natal, Brazil
- 2Department of Biology, Miami University, Oxford, OH, United States
Animals can act as sources or sinks of nutrients in ecosystems, and their role in this context may depend on the fate of nutrients in decomposing carcasses, which may contain recalcitrant structures such as bones. Our goal was to assess whether a fish population with high biomass is a source or sink of nutrients to the pelagic zone of a eutrophic lake over time scales ranging from days to 20 years. We developed a population-level model based on a 20-year (1996–2015) dataset for gizzard shad (Dorosoma cepedianum) in Acton Lake, a eutrophic reservoir in southwest Ohio, U.S.A. In addition, we used data from experiments that quantified nutrient mineralization rates from carcasses as functions of fish size and temperature. Nitrogen (N) and phosphorus (P) remineralization rates from carcasses increased with temperature and decreased with fish size. Over the 20 years, almost all (~99%) of the nitrogen (N) and phosphorus (P) produced as gizzard shad carcasses was remineralized back to the water column. At the ecosystem scale, carcass nutrient dynamics followed a seasonal pattern, with a net accumulation of carcass nutrients in winter but a net depletion of the carcass nutrient pool in summer, due to mineralization. Dynamics of carcass production and remineralization were strongly influenced by young-of-year fish (YOY), for both N and P, because the number of fish born varied considerably across years, YOY have high mortality rates, and YOY carcasses decompose rapidly. On an annual basis, in a few years biomass production was higher than mineralization, suggesting that in these years fish biomass may act as a nutrient sink at the annual scale. However, nutrient excretion by the population greatly exceeded sequestration of nutrients in biomass (living and dead). Because most of the nutrients consumed (and excreted) by this population are derived from the benthos, at the lake-wide scale and considering all fluxes, the population is a significant net source of nutrients to the pelagic habitat. Our model demonstrates the relevance of considering spatial and temporal scale as well as long-term population dynamics when studying the role of animals as nutrient sources or sinks.
Introduction
Animal populations can be nutrient sources or sinks (Kitchell et al., 1975; Beasley et al., 2012; Barton et al., 2013; Atkinson et al., 2017; Subalusky and Post, 2018). In aquatic ecosystems, an animal population can act as a source of nutrients when releasing nutrients in available forms to other members of the ecosystem, and as a sink when removing nutrients from circulation in the ecosystem (Figure 1). Studies on nutrient cycling by fish (and other aquatic animals) have focused mostly on their role as a nutrient source through excretion (Atkinson et al., 2017; Subalusky and Post, 2018). However, because fish can represent a large proportion of animal biomass in many ecosystems (Barton et al., 2019), because they are long-lived compared to other organisms, and because their bodies contain recalcitrant tissues like bones and scales, it has been suggested that fish populations act as nutrient sinks in pelagic freshwaters (Kitchell et al., 1975; Sereda et al., 2008). The main ways in which a fish population can be a nutrient sink are (1) if biomass increases, i.e., when growth and reproduction exceed mortality, (2) if emigration from the ecosystem exceeds immigration to that ecosystem, and (3) if nutrients stored in carcasses are not mineralized back to the water column, but rather remain stored in sediments in a recalcitrant form for a long time (Vanni et al., 2013).
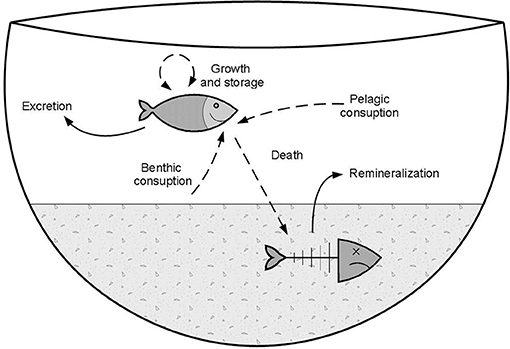
Figure 1. Conceptual model showing the fluxes of nutrients derived from fish (sources to the water column, solid arrows) and stored in fish biomass (sinks from the water column, dashed arrows). Fish can act as nutrient sinks when they remove nutrients from circulation and store them in their bodies for growth, or when fish die and nutrients are stored in recalcitrant tissues. On the other hand, they act as sources of nutrients to the water column when they are releasing nutrients through excretion and remineralization of nutrients stored in carcasses. Piscivory is omitted here for simplicity.
If animal biomass is relatively stable, the fate of carcasses may be especially important in determining whether animals are nutrient sources or sinks. In general, animal carcasses are nutrient-rich resources that generally decompose much faster than plant detritus (Barton et al., 2013, Benbow et al., 2019). However, vertebrate carcasses contain bone, a phosphorus (P)-rich component that decomposes much more slowly than other tissues (e.g., Parmenter and Lamarra, 1991; Subalusky et al., 2017). Thus, carcasses could represent a relatively long-term P sink, and if P is the limiting nutrient this could constrain primary production. In addition, carcasses are produced and deposited at various spatial and temporal scales, which can modulate their role as sources or sinks. For example, in some populations carcasses are produced in highly episodic mass mortality events, whereas in other populations mortality is more temporally constant or varies seasonally (Fey et al., 2015; Subalusky et al., 2017). Carcass deposition can also be spatially variable, producing nutrient hotspots in the landscape that influence soil, or sediment biogeochemistry and primary production (Bump et al., 2009; Keenan et al., 2018). More specifically, in aquatic ecosystems, carcasses of pelagic animals may sink to the sediments, out of the euphotic zone and away from pelagic primary producers; thus they can be a sink for the pelagic ecosystem, but whether they are depends on the rate at which carcass nutrients are remineralized and transported to pelagic primary producers (Beasley et al., 2012; Vanni et al., 2013).
In this paper, we assess whether a fish population with high biomass and growth rate is a source or sink of nitrogen and phosphorus to the pelagic zone of a eutrophic lake, at various time scales ranging from days to years. To accomplish this, we used a population-level model for gizzard shad (Dorosoma cepedianum) over a 20 year period in Acton Lake, a eutrophic reservoir in Southwest Ohio, U.S.A. Gizzard shad are abundant in lakes and reservoirs of the Midwest and southeast USA, and non-larval age classes feed mostly on organic detritus associated with sediments (Schaus et al., 1997; Higgins et al., 2006). Consumption of benthic-derived nutrients and subsequent excretion of these nutrients into the water column by gizzard shad represents an important nutrient source to phytoplankton (Shostell and Bukaveckas, 2004; Vanni et al., 2006; Schaus et al., 2010; Williamson et al., 2018). However, the role of nutrient storage in living fish and the fate of nutrients in carcasses is not well-known at the ecosystem scale. Our approach is comprehensive in that we explicitly quantified how this population can be a nutrient sink (storage in living biomass and carcasses) as well as a source (excretion, remineralization from carcasses) to pelagic primary producers, incorporating all life stages (including larvae) over a 20-year period.
Methods
Population Model
We used the gizzard shad population model of Williamson et al. (2018), which estimated fish population size and age structure for multiple cohorts over a 20-year period (1996–2015). The model combines data from electrofishing, hydroacoustics, and larval fish sampling, to track the number and size of fish in each age class, and ultimately the mass of nutrients sequestered in living biomass and produced as carcasses, on a daily basis. Details of the population model are described in Williamson et al. (2018). Briefly, the number of larval fish was estimated using weekly tows with an ichthyoplankton or neuston net during the period of larval production (generally, May-June), for all 20 years. This allowed us to estimate the number of fish born each year, following methods of Bremigan and Stein (2001). Non-larval fish were sampled each August (1999–2015) using hydroacoustics (Hale et al., 2008), which allowed us to annually estimate population size and age-structure. We do not have hydroacoustics estimates for 1996–1998; to obtain population estimates in August of each of these 3 years, we used a regression between electrofishing data (catch per unit effort, CPE) and hydroacoustics estimates developed for years in which we have both types of data (Williamson et al., 2018). Thus, for young-of-year (YOY) fish, we had estimates of cohort density (no. YOY fish per ha) during the hatching period (May–June) and in August, and for non-YOY fish we had estimates each August. The number of fish present in each cohort (ages 0, 1, 2, 3, and 4+ years) on each day in between population estimates was interpolated using daily instantaneous mortality rates, as described in Williamson et al. (2018). Fish size (wet mass) was obtained from larval fish tows, hydroacoustics and electrofishing, and interpolated on a daily basis (Williamson et al., 2018). Mortality, i.e., the number of fish in each cohort dying each day, was estimated by difference in cohort density on successive dates; this assumes no immigration or emigration in this population. We assumed that piscivory accounted for 15% of the mortality of gizzard shad smaller than 200 mm total length, when temperature was > 10°C; for fish >200 mm, and for all fish when temperature was <10°, we assumed no piscivory. These assumptions are based on piscivory estimates for largemouth bass and other piscivores in Acton Lake (Aman, 2007). Fish that died but were not consumed by piscivores were assumed to sink to the sediments as carcasses.
Nutrient Pools and Fluxes
Using data on the number and size of fish in each cohort, we estimated the mass of nutrients (N and P) in live fish biomass, and “lost” from the pelagic zone due to mortality on a daily basis. We also considered nutrients excreted by fish in the water column, using data from Williamson et al. (2018).
For each cohort, we modeled pools of carcass N and P as the balance of daily carcass production (mortality not due to piscivory) vs. mineralization. For each cohort, carcass production (no. carcasses produced ha−1 d−1) was converted to nutrient mass produced as carcasses (g N and P ha−1 d−1). To do so, fish wet mass was first converted to dry mass, and then to N and P mass using size-specific data derived from the Acton Lake gizzard shad population (Schaus et al., 1997; Pilati and Vanni, 2007; Torres and Vanni, 2007).
Remineralization of nutrients from gizzard shad carcasses was estimated using data from lab experiments that quantified nutrient mineralization rates from carcasses of different sizes, incubated at three temperatures (5, 15, and 25°C). Details on these experiments can be found in the Supplemental Material; briefly, we incubated YOY and adult carcasses at these three temperatures and measured their nutrient contents on several dates for up to 100 days (or shorter if carcasses fully decomposed). Based on these experiments, we calculated the decay rate (k) of carcass N and P by fitting the equation Mt = M0e-kt, where Mt and M0 are the masses of the element (N or P) remaining in carcasses at time t (days) and k is the instantaneous daily decay rate. Then we used two-way analysis of variance (ANOVA) to evaluate the individual and interactive effects of fish size and temperature on decay rates (k) for carcass N and P. When ANOVA detected significant effects of the experimental factors, we used Tukey's post-test for multiple comparisons to discriminate significant statistical differences between levels of experimental factors.
To estimate daily mineralization rates as a function of lake temperature, for each fish size class, we used Q10, defined as the factor by which a decay rate increased when temperature increased by 10°C. This factor is commonly used in studies testing the temperature effects on biological rates (Lloyd and Taylor, 1994; Downs et al., 2008). For example, if a rate doubles when temperature is increased from 5 to 15°, the Q10 = 2. The values of Q10 for N and P decay rates for YOY and adult carcasses were estimated by using the k values computed previously; we calculated separate Q10 values for 5–15° and 15–25°. Q10 values for adult carcasses were used for all non YOY age classes. Cohort carcass mineralization rates were summed to obtain carcass mineralization rates at the lake-wide scale. We assumed that all nutrients mineralized from carcasses were returned to the water column in dissolved inorganic form and therefore were a nutrient source to phytoplankton; i.e., we assumed no benthic uptake of nutrients remineralized from carcasses. We feel that this is a valid assumption because Acton Lake is turbid and very little light reaches sediments (Secchi depth usually ~0.5–0.7 m); therefore, uptake by benthic algae is minimal, although we acknowledge that some remineralized nutrients could be taken up, at least temporarily, by sediment microbes.
To estimate the flux of nutrients through piscivores, we assumed that piscivore biomass was constant at the lake-wide scale. We assumed that piscivores assimilated 80% of the N and P they ingested from gizzard shad bodies, and therefore egested 20% of ingested N and P as feces (Schindler and Eby, 1997). We assumed that piscivore gross growth efficiency (N or P growth/N or P ingestion) was 30% for both N and P (based on Schindler and Eby, 1997). Therefore, piscivores excreted 50% of the N and P they consumed; this excretion was considered a source to the water column. Because we assumed that piscivore population biomass was constant, the production of piscivore carcasses was considered to be 30% of ingested N and P, i.e., equal to their growth at the population level. Thus, nutrients consumed by piscivores were allocated as follows: 20% egested as feces; 30% to mortality (piscivore carcasses), and 50% excreted as dissolved nutrients. N and P in piscivore carcasses was assumed to be mineralized at rates equal to those for adult gizzard shad carcasses. N and P egested by piscivores as feces was assumed to remineralize at the same rate as YOY gizzard shad carcasses, i.e., faster than adult shad or piscivore carcasses.
The modeling approach we employed for the fate of nutrients through piscivores is obviously a simplification, although assumptions about piscivory are based on data from Acton Lake. Nevertheless, we explored additional piscivory scenarios in which we relaxed these assumptions and compared these simulations to our “baseline” model. Specifically, we explored additional scenarios regarding our assumption that piscivore biomass was constant, and that piscivory accounted for 15% of mortality of gizzard shad ≤ 200 mm when temperature was ≥ 10°. Data from Acton Lake show that piscivore catch-per-unit-effort declined in Acton Lake from 2003/2004 to about 2008, but no data are available before 2003 (Supplemental Material). We considered a scenario in which piscivore population biomass doubled from 1998 to 2003, from 20 to 40 kg wet mass/ha, to mirror the decline over the following 5 years. In addition, we explored scenarios in which we varied the percentage of shad mortality caused by piscivory, from 10 to 30% (see Supplemental Material for details), deviating from the baseline model in which it was 15%. In all scenarios, the flux of nutrients through piscivores was relatively small compared to that via non-piscivory gizzard shad mortality (see Results). Because the contribution of piscivores to the flux of nutrients was small, and for model simplification purposes, here we present the results that include the flux of nutrients through piscivory, i.e., piscivores excretion and mineralization from piscivores feces and carcasses, combined with non-piscivory gizzard shad mortality, i.e., carcass mineralization.
Results
Remineralization rates of carcass nutrients (k) increased with temperature and were higher for YOY than adults (Figures 2, 3, Supplemental Table 2). k was higher for N than P, reflecting the greater recalcitrance of P because of bones and scales. Q10 values for N and P ranged from ~1 to 3 depending on fish size and temperature increment (5–15 or 15–25°) (Table 1).
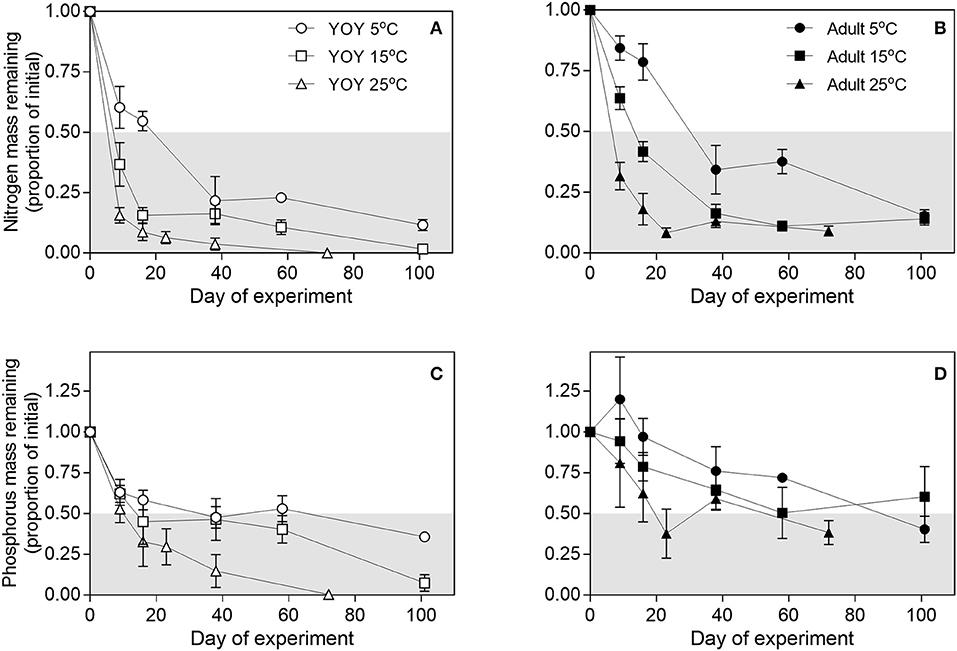
Figure 2. Individual and interactive effects of fish size and temperature on patterns of mass remaining for (A,B) nitrogen (C,D) phosphorus mass in decomposing fish carcasses throughout time. Values are expressed as the proportion of initial mass in carcasses. Data for young-the-year (YOY) and adult fish are shown in separate panels for clarity. The transition between the white and gray areas in the panels indicate the half-lives of mass decay. Data points are mean (n = 3) and error bars are ± 1SEM.
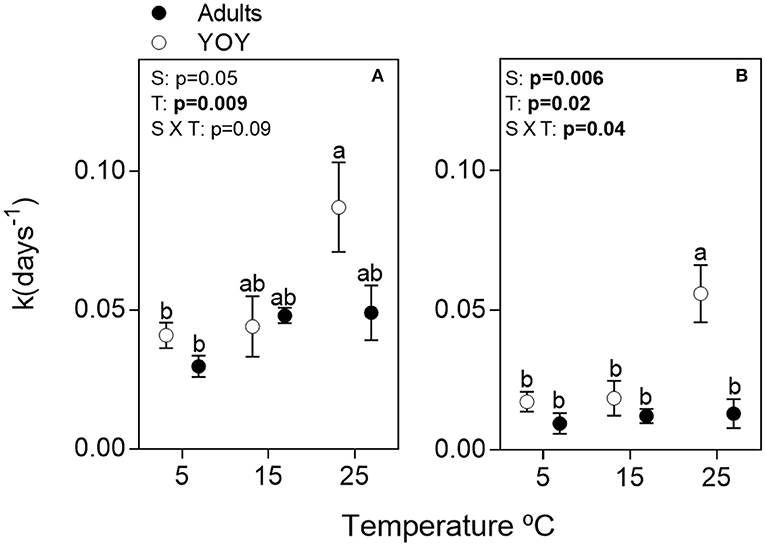
Figure 3. Individual and interactive effects of fish size (S) and temperature (T) on time-integrated fish carcass decay coefficients (k) for (A) nitrogen and (B) phosphorus. Data points are means (n = 3) and error bars are ± 1SE. Different letters above treatments indicate significant statistical differences among treatments. Bold p-values depict significant statistical effects (Tukey's pos-hoc test; p < 0.05).
Over the 20-year period, 99.4% of the N and 98.6% of the P produced as gizzard shad carcasses were remineralized back to the water column. The dynamics of carcass production and remineralization followed a seasonal pattern and were strongly influenced by young-of-year fish (YOY), for both N and P (Figure 4). Nutrients accumulated in the carcass pool over winter months, when mortality was high and mineralization rates were low; in contrast, a net depletion of the carcass nutrient pool occurred during warmer months when mineralization rates were high. During July and August, a small peak in the carcass nutrient pool was also detected, due to a short-term accumulation of carcasses of YOY fish, followed by a rapid decrease until October (Figure 4). These dynamics reflect the high mortality rates, and rapid carcass mineralization rates, of YOY fish.
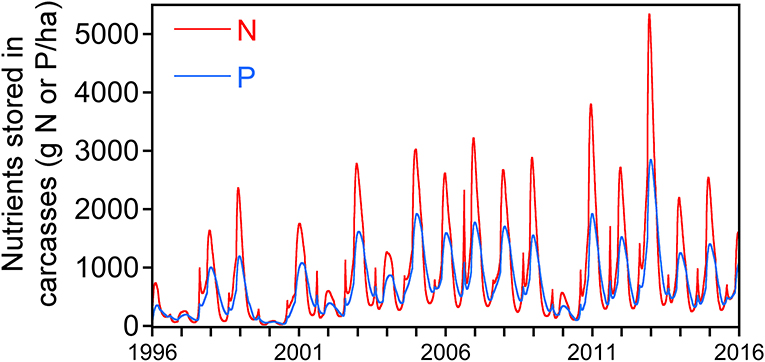
Figure 4. Twenty-year time series showing the dynamics of phosphorus (blue line) and nitrogen (red line) stored in carcasses over time. The large peak occurring during winter is due to high mortality (high carcass production) rate of all age classes during this time, whereas the smaller peaks occurring in late July-August are due to production of carcasses from high mortality of young-of-year fish.
On an annual basis, N and P sequestered by the gizzard shad population via biomass production was higher than carcass mineralization in a few years, suggesting that fish biomass (living and dead) may act as a nutrient sink on a yearly time scale (Figure 5). However, in most years mineralization exceeded biomass production. Furthermore, for both N and P, excretion rates were higher (often much higher) than biomass production in all years (Figure 5). Thus, considering all fluxes, this fish population is a net source of N and P to the water column on an annual basis.
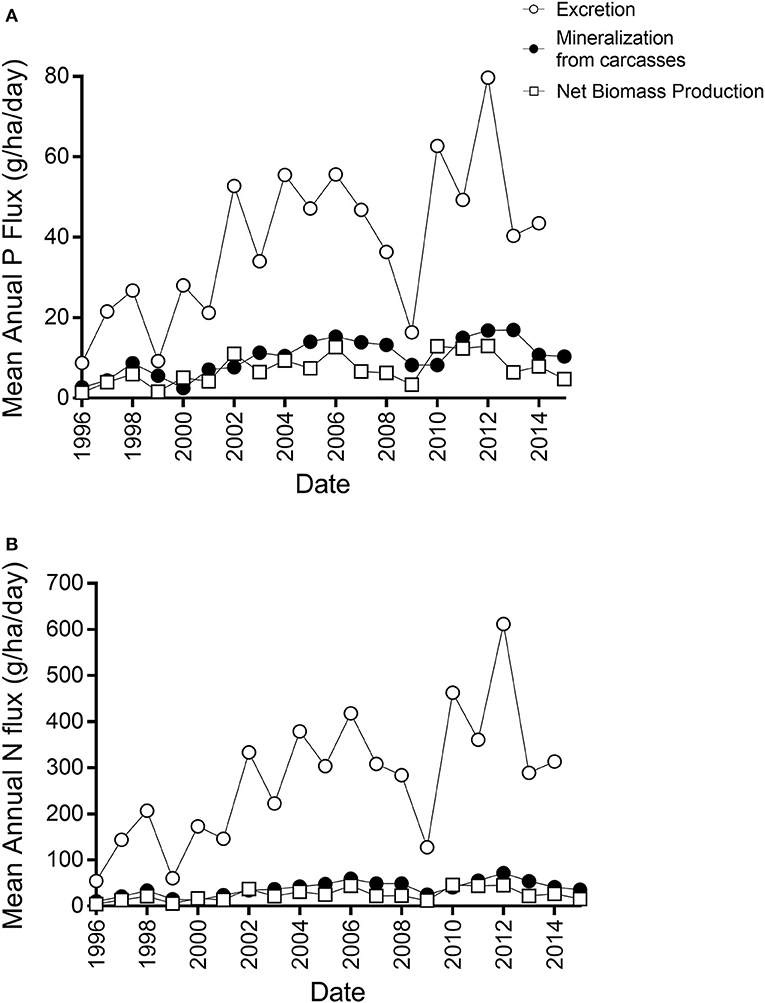
Figure 5. Annual fluxes of phosphorus (A) and nitrogen (B) for net biomass production (white square), carcass remineralization (black dots), and excretion (white dots).
On a daily basis, the gizzard shad population was usually a source of nutrients. Thus, over the study period, mineralization plus excretion exceeded carcass production on 92.6 and 86.8% of days for N and P, respectively. The days on which nutrient accumulation in carcasses was higher than mineralization plus excretion occurred only during winter.
Piscivory accounted for the consumption of 15,133 and 4,037 g P/ha, representing, respectively, only 5.5 and 5.1% of total gizzard shad N and P biomass “lost” to total mortality. Over the 20-year period, almost all nutrients (99.9% of N and 99.7% of P) consumed by piscivores were released back to the water column via excretion, egestion, and piscivore carcass decomposition.
Results of the piscivory scenarios showed that the ultimate fates of nutrients from gizzard shad mortality were relatively insensitive to the assumptions in the baseline model. Doubling piscivore biomass from 1998 to 2003 reduced the flux of dissolved nutrients through piscivores (via piscivore carcass and feces mineralization, plus piscivore excretion) by 13% (N) or 15% (P) over this 5-year period, as these nutrients were stored in living piscivore biomass rather than mineralized (Supplemental Material). However, in total all of these fluxes through piscivores represent <6% of the flux via mineralization of gizzard shad carcasses; therefore, doubling piscivore biomass reduced total fluxes of N and P to the water column by <1% compared to the baseline model. Varying the percentage of gizzard shad mortality attributed to piscivores also had a relatively small effect on net N and P fluxes to the water column. As this percentage increased from 10 to 30%, N and P mineralization from the gizzard shad carcass pool declined, but this was accompanied by compensatory increases in piscivore excretion plus the mineralization of piscivore carcasses and feces (Supplemental Figure 6). In all scenarios, the percentage of nutrients produced via shad mortality that remained as carcasses (shad plus piscivores) was <0.6% for N and <1.4% for P. Thus, in all scenarios >99% of N and >98% of P were returned to the water column.
Discussion
Our model suggests that, considering all fluxes of nutrients, this fish population is a net source of N and P to the water column, even though fish biomass is relatively high and fish are much more long-lived compared to phytoplankton, which are the dominant primary producers. In Acton Lake, phosphorus “trapped” in live gizzard shad biomass averaged about 47 μg P L−1 over our 20 year study, and lake “total P” (P in the water column, excluding fish) averaged about 105 μg P L−1 Therefore, the gizzard shad population contains a pool of P equal to roughly 45% of the traditionally-measured “total P.” However, almost all of this P becomes available relatively quickly after fish die and decompose. Thus, neither the high biomass of living fish nor the production of carcasses renders this population a long-term nutrient sink.
The rate at which nutrients are remineralized from fish carcasses is likely to vary greatly among ecosystems. Boros et al. (2015) argued that fish carcasses are not likely to act as a long-term P sink in warm-temperate shallow lakes. In their experimental work, carcasses of gizzard shad and bluegill (Lepomis macrochirus) decomposed completely over ~3 months in mesocosms that were warm (mean temperature 24.5°C) and well oxygenated, i.e., conditions similar to those in Acton Lake in summer. Thus, Acton Lake has conditions that are favorable for carcass decomposition. In deeper, colder lakes fish carcasses may be nutrient sinks (Kitchell et al., 1975; Parmenter and Lamarra, 1991), but much depends on carcass remineralization rates. Parmenter and Lamarra (1991) studied carcass decomposition of rainbow trout (Oncorhynchus mykiss) in colder environments (2–5°C) and found that most of the decomposition activity took place during the initial 60 days of carcass decomposition. By the end of the experiment fish carrion lost 95% of original carcass N, but only 60% of carcass P due to its presence in more recalcitrant tissues. Chidami and Amyot (2008) argued that water temperature can be used as a surrogate for decomposition rates in freshwaters. Studying boreal lakes, they found that decreasing temperatures are related to increased decomposition half-lives, probably due to lower metabolic activity of decomposing bacteria and scavengers.
Animal size also plays a role. We found that remineralization rates were higher for small gizzard shad carcasses than for large carcasses, although all sizes decomposed rapidly at warm temperatures. Very large animal carcasses will probably decompose even more slowly; for example, Subalusky et al. (2017) found that wildebeest bones take 7 years to fully decompose even in a warm, tropical river. In ecosystems where bones mineralize slowly, carcasses are more likely to be long-term sinks for P, than for N.
In our model, carcasses mineralized fairly rapidly, so at an annual scale they were not a sink for nutrients. However, because of variation in water temperature and fish size, we found temporal variation in the amount of nutrients being stored in vs. mineralized from carcasses, at annual and seasonal scales. Thus, while the population is a net source of N and P to the water column, there are specific periods when N and P accumulate in live biomass or in carcasses, and during these periods the population acts as a temporary sink. During winter, for example, both excretion and decomposition rates are low, promoting a short-term nutrient accumulation in fish carcasses. In terms of fish population biomass, short term sinks also occur in summer during the period of YOY growth followed by higher rates of YOY mortality. The strong influence of the YOY on the dynamics of carcass production and N and P remineralization (Supplemental Figure 4) is due to the variability in the number of fish born across years (Kraft, 1992; Williamson et al., 2018), the high mortality rates of this age class, and the fact that YOY carcasses decompose rapidly. However, during these periods excretion rates are high and exceed this potential sink. These dynamics illustrate the importance of explicitly considering both temporal scale and the multiplicity of potential fluxes of nutrients when analyzing the extent to which fish are sources or sinks of nutrients.
It is potentially informative to compare nutrient fluxes from our model with other fluxes from sediments to water. Nowlin et al. (2005) measured N and P fluxes from Acton Lake sediments using sediment core incubations; these “direct” fluxes are probably mediated mainly by microbes but also may include excretion by small invertebrates that happen to be in the cores. In summer (the only period when core incubations were done), direct fluxes of NH4 exceed carcass N mineralization by 4-5X at the lake-wide scale (Table 2). For P, the results are more complex. As would be expected, direct flux of P is much higher (>9X) from anoxic, hypolimnetic sediments than from oxic sediments; the mineralization rate of P from carcasses is similar in magnitude to direct flux of P from oxic sediments (Table 2). At a lake-wide scale, accounting for the areas of oxic and anoxic sediments, direct P flux exceeds carcass mineralization by 4-5X. However, Nowlin et al. (2005) showed that very little of the P released from hypolimnetic sediments is transported to the euphotic zone where phytoplankton can use the P, probably because P precipitates with calcium in the hypolimnion and sinks to sediments. Regardless of the relative magnitudes of direct fluxes vs. carcass mineralization rates, it is clear from our study that carcasses do not represent long-term sinks. Furthermore, during summer, excretion by gizzard shad exceeds direct fluxes from sediments for both N and P (Table 2).
Our study is unique in that we have extensive data on the fish population dynamics (including all age classes) at the ecosystem level and over a 20-year period. This large and comprehensive dataset allowed us to produce robust estimates for population dynamics as well as fluxes of nutrients mediated by the population. With the exception of research on Pacific salmon (e.g., Tiegs et al., 2011; Rüegg et al., 2014), the fate of carcasses in freshwater environments has not been explored as much as in marine and terrestrial systems (Beasley et al., 2012), so our study helps to fill this gap in the nutrient cycling literature. We encourage additional whole-ecosystem studies that assess whether animals are nutrient sources or sinks. The fates of nutrients associated with different mortality sources need to be explored more (Barton et al., 2019). Based on our results, nutrients consumed by fish piscivores are not likely to be long-term sinks. However, piscivorous birds may function as sinks by transporting nutrients away from an aquatic ecosystem. In addition, the role of scavengers in processing carcass nutrients needs to be evaluated. More broadly, to fully understand the role of animals as nutrient sources or sinks, we need studies in a range of environments that reflects the breath of variation in habitat features such as depth, temperature, the balance of benthic vs. pelagic production, and other potential drivers.
Ethics Statement
This study was carried out in accordance with the recommendations of the Miami University Institutional Animal Care and Use Committee.
Author Contributions
RN and MV conceived the population model. LC and SP conducted the lab experiments. MG and MV collected the data on the fish population. RN, MV, and LC wrote the final versions of the manuscript. All authors contributed to writing the paper.
Funding
Our research on gizzard shad was supported primarily by National Science Foundation awards 9318452, 9726877, 0235755, 0743192, and 1255159, and the Federal Aid in Sport Fish Restoration Program (F-69-P, Fish Management in Ohio) administered jointly by the U.S. Fish and Wildlife Service and the Ohio Department of Natural Resources-Division of Wildlife.
Conflict of Interest Statement
The authors declare that the research was conducted in the absence of any commercial or financial relationships that could be construed as a potential conflict of interest.
Acknowledgments
LC appreciates the support of Brazilian National Council for Scientific and Technological Development (CNPq) for a post-graduate scholarship. RN was thankful to Coordination of Improvement of Higher Education Personnel (CAPES) for post-graduate scholarship and Fulbright Brazil for a doctoral dissertation research award. We were also thankful to Joseph D. Conroy from the Ohio Division of Wildlife for providing piscivore data, to the Society for Freshwater Science and the organization committee of the SFS annual meeting.
Supplementary Material
The Supplementary Material for this article can be found online at: https://www.frontiersin.org/articles/10.3389/fevo.2019.00340/full#supplementary-material
References
Aman, C. R. (2007). Factors Influencing Saugeye Stocking Success in Ohio Reservoirs. Masters thesis, The Ohio State University, Columbus, Ohio, USA.
Atkinson, C. L., Capps, K. A., Rugenski, A. T., and Vanni, M. J. (2017). Consumer-driven nutrient dynamics in freshwater ecosystems : from individuals to ecosystems. Biol. Rev. 92, 2003–2023. doi: 10.1111/brv.12318
Barton, P. S., Cunningham, S. A., Lindenmayer, D. B., and Manning, A. D. (2013). The role of carrion in maintaining biodiversity and ecological processes in terrestrial ecosystems. Oecologia 171, 761–772. doi: 10.1007/s00442-012-2460-3
Barton, P. S., Evans, M. J., Foster, C. N., Pechal, J. L., Bump, J. K., Quaggiotto, M. M., et al. (2019). Towards quantifying carrion biomass in ecosystems. Trends Ecol. Evol. doi: 10.1016/j.tree.2019.06.001. [Epub ahead of print].
Beasley, J. C., Olson, Z. H., and DeVault, T. L. (2012). Carrion cycling in food webs : comparisons among terrestrial and marine ecosystems. Oikos 121, 1021–1026. doi: 10.1111/j.1600-0706.2012.20353.x
Benbow, M. E., Barton, P. S., Ylyshen, M. D., Beasley, J. C., DeVault, T. L., Strickland, M. S., et al. (2019). Necrobiome framework for bridging decomposition ecology of autotrophically and heterotrophically derived organic matter. Ecol. Monogr. 89:e01331. doi: 10.1002/ecm.1331
Boros, G., Takács, P., and Vanni, M. J. (2015). The fate of phosphorus in decomposing fish carcasses: a mesocosm experiment. Freshw. Biol. 60, 479–489. doi: 10.1111/fwb.12483
Bremigan, M. T., and Stein, R. A. (2001). Variable gizzard shad recruitment with reservoir productivity: causes and implications for classifying systems. Ecol. Appl. 11, 1425–1437. doi: 10.1890/1051-0761(2001)0111425:VGSRWR2.0.CO;2
Bump, J. K., Peterson, R. O., and Vucetich, J. A. (2009). Wolves modulate soil nutrient heterogeneity and foliar nitrogen by configuring the distribution of ungulate carcasses. Ecology 90, 3159–3167. doi: 10.1890/09-0292.1
Chidami, S., and Amyot, M. (2008). Fish decomposition in boreal lakes and biogeochemical implications. Limnol. Oceanogr. 53, 1988–1996. doi: 10.4319/lo.2008.53.5.1988
Downs, C. J., Hayes, J. P., and Tracy, C. R. (2008). Scaling metabolic rate with body mass and inverse body temperature: a test of the Arrhenius fractal supply model. Funct. Ecol. 22, 239–244 doi: 10.1111/j.1365-2435.2007.01371.x
Fey, S. B., Siepielski, A. M., Nusslé, S., Cervantes-yoshida, K., and Hwan, J. L. (2015). Recent shifts in the occurrence, cause, and magnitude of animal mass mortality events. Proc. Natl. Acad. Sci. U.S.A. 112, 1083–1088. doi: 10.1073/pnas.1414894112
Hale, R. S., Degan, D. J., Renwick, W. H., Vanni, M. J., and Stein, R.a (2008). Assessing fish biomass and prey availability in ohio reservoirs. Am. Fish. Soc. Symp. 62, 517–541.
Higgins, K. A., Vanni, M. J., and Gonza, M. J. (2006). Detritivory and the stoichiometry of nutrient cycling by a dominant fish species in lakes of varying productivity. Oikos. 114, 419–430. doi: 10.1111/j.2006.0030-1299.14745.x
Keenan, S. W., Schae, S. M., Jin, V. L., and Debruyn, J. M. (2018). Mortality hotspots : nitrogen cycling in forest soils during vertebrate decomposition. Soil Biol. Biochem. 121, 165–176. doi: 10.1016/j.soilbio.2018.03.005
Kitchell, J. F., Koonce, J. F., and Tennis, P. S. (1975). Phosphorus flux through fishes. SIL Proc. 19, 2478–2484. doi: 10.1080/03680770.1974.11896332
Kraft, C. E. (1992). Estimates of phosphorus and nitrogen cycling by fish using a bioenergetics approach. Can. J. Fish. Aquat. Sci. 49, 2596–2604 doi: 10.1139/f92-287
Lloyd, J., and Taylor, J. A. (1994). On the temperature-dependence of soil respiration. Funct. Ecol. 8, 315–323 doi: 10.2307/2389824
Luhring, T. M., Delong, J. P., Semlitsch, R. D., and Lomas, M. W. (2017). Stoichiometry and life-history interact to determine the magnitude of cross-ecosystem element and biomass fluxes. Front. Microbiol. 8:814. doi: 10.3389/fmicb.2017.00814
Nowlin, W. H., Evarts, J. L., and Vanni, M. J. (2005). Release rates and potential fates of nitrogen and phosphorus from sediments in a eutrophic reservoir. Freshw. Biol. 50, 301–322. doi: 10.1111/j.1365-2427.2004.01316.x
Parmenter, R. R., and Lamarra, V. A. (1991). Nutrien cycling in a freshwater marsh : The decomposition of fish and waterfowl carrion. Limnol. Oceanogr. 36, 976–987. doi: 10.4319/lo.1991.36.5.0976
Pilati, A., and Vanni, M. J. (2007). Ontogeny, diet shifts, and nutrient stoichiometry in fish. Oikos 116, 1663–1674. doi: 10.1111/j.0030-1299.2007.15970.x
Rüegg, J., Tiegs, S. D., and Lamberti, G. A. (2014). Habitat influences Pacific salmon (Oncorhynchus spp.) tissue decomposition in riparian and stream ecosystems. Aquat. Sci. 76, 623–632. doi: 10.1007/s00027-014-0359-2
Schaus, M. H., Godwin, W., Battoe, L., Coveney, M., Lowe, E., Roth, R., et al. (2010). Impact of the removal of gizzard shad (Dorosoma cepedianum) on nutrient cycles in Lake Apopka, Florida. Freshw. Biol. 55, 2401–2413. doi: 10.1111/j.1365-2427.2010.02440.x
Schaus, M. H., Vanni, M. J., Wissing, T. E., and Stein, R. A. (1997). Nitrogen and phosphorus excretion by detritivorous gizzard shad in a reservoir ecosystem. Limnol. Oceanogr. 42, 1386–1397. doi: 10.4319/lo.1997.42.6.1386
Schindler, D. E., and Eby, L. A. (1997). Stoichiometry of fishes and their prey : implications for nutrient recycling. Ecology 78, 1816–1831. doi: 10.1890/0012-9658(1997)078[1816:SOFATP]2.0.CO;2
Sereda, J. M., Hudson, J. J., Taylor, W. D., and Demers, E. (2008). Fish as sources and sinks of nutrients in lakes. Freshw. Biol. 53, 278–289.
Shostell, J., and Bukaveckas, P. A. (2004). Seasonal and interannual variation in nutrient fluxes from tributary inputs, consumer recycling and algal growth in a eutrophic river impoundment. Aquat. Ecol. 38, 359–373. doi: 10.1023/B:AECO.0000035167.67399.63
Subalusky, A. L., Dutton, C. L., Rosi, E. J., and Post, D. M. (2017). Annual mass drownings of the Serengeti wildebeest migration influence nutrient cycling and storage in the Mara River. Proc. Natl. Acad. Sci. U.S.A. 114, 7647–7652. doi: 10.1073/pnas.1614778114
Subalusky, A. L., and Post, D. M. (2018). Context dependency of animal resource subsidies. Biol. Rev. 94, 517–538. doi: 10.1111/brv.12465
Tiegs, S. D., Levi, P. S., Ru, J., Chaloner, D. T., Tank, J. L., and Lamberti, G. A. (2011). Ecological effects of live salmon exceed those of carcasses during an annual spawning migration. Ecosystems 14, 598–614. doi: 10.1007/s10021-011-9431-0
Torres, L. E., and Vanni, M. J. (2007). Stoichiometry of nutrient excretion by fish: interspecific variation in a hypereutrophic lake. Oikos 116, 259–270. doi: 10.1111/j.0030-1299.2007.15268.x
Vanni, M. J., Boros, G., and McIntyre, P. B. (2013). When are fish sources vs. sinks of nutrients in lake ecosystems? Ecology 94, 2195–2206. doi: 10.1890/12-1559.1
Vanni, M. J., Bowling, A. M., Dickman, E. M., Hale, R. S., Higgins, K.A, Horgan, M. J., et al. (2006). Nutrient cycling by fish supports relatively more primary production as lake productivity increases. Ecology 87, 1696–709. doi: 10.1890/0012-9658(2006)87[1696:NCBFSR]2.0.CO;2
Keywords: nutrient cycling, decomposition, nitrogen, phosphorus, mineralization
Citation: Nobre RLG, Carneiro LS, Panek SE, González MJ and Vanni MJ (2019) Fish, Including Their Carcasses, Are Net Nutrient Sources to the Water Column of a Eutrophic Lake. Front. Ecol. Evol. 7:340. doi: 10.3389/fevo.2019.00340
Received: 10 December 2018; Accepted: 26 August 2019;
Published: 10 September 2019.
Edited by:
M. Eric Benbow, Michigan State University, United StatesReviewed by:
Randolph M. Chambers, College of William and Mary, United StatesMollie Day McIntosh, Xavier University, United States
Copyright © 2019 Nobre, Carneiro, Panek, González and Vanni. This is an open-access article distributed under the terms of the Creative Commons Attribution License (CC BY). The use, distribution or reproduction in other forums is permitted, provided the original author(s) and the copyright owner(s) are credited and that the original publication in this journal is cited, in accordance with accepted academic practice. No use, distribution or reproduction is permitted which does not comply with these terms.
*Correspondence: Regina L. G. Nobre, cmVnaW5hbm9icmUuZWNvQGdtYWlsLmNvbQ==
†Present Address: Sarah E. Panek, Florida Department of Environmental Protection, Tallahassee, FL, United States