- 1Institute of Biology, University of Neuchâtel, Neuchâtel, Switzerland
- 2Institute of Biology, University of Latvia, Riga, Latvia
- 3Forest Dynamics, Swiss Federal Institute for Forest, Snow, and Landscape Research (WSL), Birmensdorf, Switzerland
Street greeneries and other green spaces within the urban matrix can potentially reduce air pollution and increase urban biodiversity. Yet, these services can be negatively affected by anthropogenic stress factors. In the boreo-nemoral zone, large amounts of salts are spread each year for deicing the pavement. To address the effect of deicing salt on street lines of lime trees and how this cascades up to influence the surrounding arthropod biodiversity, we compared heavily salt-polluted, and less polluted sites in the city of Riga, Latvia. We analyzed the impairment of foliar functions and development of aphid colonies using a common garden experiment. We found marked variation in the soil physico-chemical properties in polluted vs. unpolluted sites, and the overall composition of arthropod communities, considering their abundance together with their diversity, significantly responded to site contamination. In a common garden experiment, we also showed that the exposure to increased salt levels in the soil caused functional as well as structural injuries within foliage and slowed down the development of aphid colonies. Finally, the damage inflicted by the lime tree aphids, especially in unpolluted sites, was positively correlated to the production of mucilage in the leaves, suggesting herbivore-induced mucilage production in leaves. The effects of de-icing salts thus appeared to cascade up through the trophic chain and to negatively affect not only the trees but also the associated arthropod biota. These findings point at the necessity of a wider screening of plant species and cultivars that not only better tolerate anthropogenic stress but also promote the biodiversity in cities.
Introduction
By 2050, 80% of the world population is expected to live in cities (Cohen, 2003). Supplying future communities with an adequate level of natural ecosystem exposure thus represents a challenging issue (Chen and Jim, 2008; Klemm et al., 2015). In the framework of urban environmental policies, the green infrastructure, such as parks and street greeneries established in cities all around the world, are key elements that mitigate, for example, urban noise and air pollution, and improve urban biodiversity (Costanza and Folke, 1997; Bolund and Hunhammar, 1999; Thaiutsa et al., 2008; Seamans, 2013). However, urban trees are affected by considerable biotic and abiotic airborne and soil borne stress factors (Vollenweider and Günthardt-Goerg, 2005). A widespread source of stress for urban trees at mid and higher latitudes relates to snow clearing using anti-icing and de-icing salts spread on roads and sidewalks in winter. For this purpose, various deicing chemicals and abrasives, such as chloride salts, acetates, formates, urea, glycols, and even agro-based deicers, are being routinely used (Fay and Shi, 2012). Among these latter compounds, NaCl is certainly one of those salts most frequently spread in streets, because of its inexpensiveness and deicing efficiency (Berkheimer and Hanson, 2006; Fay and Shi, 2012). Therefore, compared to non-urbanized zones, as a consequence of snowmelt, runoff, infiltration, and plowing, disproportionally high amounts of NaCl end up in the urban soils close to roads, and sidewalks (Blomqvist and Johansson, 1999; Bryson and Barker, 2002; Czerniawska-Kusza et al., 2004; Legros, 2007). Moreover, some 20–63% of deicing road salt can spread tens of meters away from pavement via air transport (Blomqvist and Johansson, 1999). Therefore, de-icing salts spread in streets can have far-reaching consequences for the urban ecosystems.
Salt spreading on streets has been shown to affect the soil physical and chemical properties. In the soil, excess Na+ can bind to the negatively-charged aggregates of mineral and organic constituents of soils (the clay-humus complex), and in turn dislodge important plant nutrients such as Zn, K, Cu, Mn, or Ca ions, ultimately affecting soil aggregation (Legros, 2007). Compared to Na+, Cl− seems to interfere less with the soil chemistry (Dmuchowski et al., 2014). Hence, several studies demonstrate the link between Cl− mobility and contamination of groundwater (Ramakrishna and Viraraghavan, 2005) and document the toxicity of this anion for the vegetation (Paludan-Müller et al., 2002; Cekstere et al., 2008; Marosz and Nowak, 2008; Kwasowski and Czyz, 2010; Cekstere and Osvalde, 2013). In other words, salt accumulation in soils generally results in plants to uptake higher doses of Cl−, which has been directly correlated with increased leaf injury marks (Paludan-Müller et al., 2002) and a general decrease in tree vitality (Paludan-Müller et al., 2002; Dmuchowski et al., 2014).
Plant exposure to excessive salt contamination has short- as well as long-term effects. Physiological reactions to salt exposure include a reduction of shoot growth, as a possible consequence of root water shortage, and of leaf photosynthetic activity, in relation to decreased chlorophyll concentrations (Ashraf and Harris, 2004; Sudhir and Murthy, 2004). These effects can be mediated by a disturbed osmotic homeostasis, promoting “physiological drought,” as a consequence of salt accumulation (Bernstein and Hayward, 1957; Dobson, 1991; Cekstere et al., 2015). In other words, salt-stressed plants modify their hormonal signaling, such as by reducing root-to-shoot transport of kinetin, and increasing abscisic acid (ABA) production, which results in plant displaying symptoms similar to drought (Davies et al., 1986; Bano et al., 1993; Pospíšilová et al., 2000); such as enhanced production of smaller, darker, thicker leaves, reduced stomatal density (Bernstein, 1975; Abrams, 1994; Shannon, 1997), or higher root:shoot ratio (Künnemann and Gad, 1997; Munns, 2002). Salt injury is first observed at the leaf tip in the form of discoloration and necrosis. It then extends to the whole leaf margin, with leaf yellowing reaching the main veins, prior to leaf abscission (Bernstein, 1975). These symptoms are also indicative of an acceleration of cell senescence (Inada et al., 1998; Sudhir and Murthy, 2004; Gunthardt-Goerg and Vollenweider, 2007; Negrão et al., 2017). By chronic salt exposure, more severe injury, extending to the tree crown and including twig dieback, complete defoliation and even tree death in extreme cases, has been reported (Shannon, 1997; Cekstere and Osvalde, 2013).
The effects of injuries, anatomical adjustments, and eco-physiological responses caused by increased salt stress in plants can cascade up to affect the plants' associated arthropod community. Theory suggests that the diversity and abundance of arthropods' communities should reflect, from the bottom-up, the diversity and abundance of herbivores (Hunter and Price, 1992). In this regard, the plant stress hypothesis (White, 1974, 1984) predicts that palatability of plants to herbivores will increase with abiotic stress, whereas the plant vigor hypothesis (Price, 1991) predicts instead that more vigorous plants—those that are least stressed—will be the most palatable. Both theories agree, however, that variation in abiotic conditions is likely to modify plant traits so as to produce variation in plant palatability. Reviews on the topics have outlined high levels of specificity of plant-arthropod guild interactions with regard to the foliage-mediated effects of salt stress (Koricheva et al., 1998; Huberty and Denno, 2004). Specifically, Larsson (1989), based on experimental evidence, predicted that phloem and cambium feeders may respond more positively to plant stress than herbivore or gall-forming insects. Along these lines, some leaf miners were observed to successfully complete their development on salt-stressed plants, despite a reduction of leaf feeding (Martel, 1998). However, while several studies partially agree with these findings, other found non-significant effects (Baczewska et al., 2011; Muñoz et al., 2014), or even documented decreased aphid population growth in response to higher Cl− accumulation (e.g., Araya et al., 1991; Görür, 2006; Sienkiewicz-Paderewska et al., 2017). Hence, high salt content can injure leaves to a level that is actually toxic or inhibiting to the herbivores, and this should cascade up to affect the entire arthropod community living in the canopies.
The main objective in this study was to investigate the effects of soil salt pollution on foliage functional traits of lime trees, and relate them to the plant herbivore and arthropod diversity on lime tree canopies. Specifically, we had the following working hypotheses: (1) soil pollution with deicing salt causes physiological and structural injury to trees' foliage. (2) On salt-contaminated soils, salt accumulation in foliage of lime trees alters the abundance and diversity of arthropods established in the tree canopy, and (3) salt accumulation modifies the palatability to herbivores by changing physico-chemical properties of leaves.
Materials and Methods
Site Selection and Conditions
The study was conducted in the city of Riga, Latvia (56.9489°N, 24.1064°E), located within the boreo-nemoral climate zone (Kottek et al., 2006). During the 1997–2016 reference period, mean precipitation amounted to 865 mm annually, the temperatures averaged +18.7° C in summer (JJA) and −1.7° C in winter (DJF) with freezing temperatures during 90 days (source: Latvian Environment, Geology, and Meteorology Center, state limited liability). As a consequence, large quantities of NaCl, amounting to 4.06 kg m−2 yearly, have been applied every winter for deicing the street pavement (Cekstere et al., 2008). With 39% of Riga's streets lined by lime trees (or linden; Tilia x vulgaris H.), this ornamental tree is likely the most commonly planted species in street greeneries. Past surveys have highlighted that a majority of trees show salt stress symptoms, which a large fraction needs then to be replaced due to high mortality rates (Cekstere et al., 2008).
Within a larger framework project on structural effects of deicing salt (Sciex-NMSch grant), 7 sites in Riga's downtown and one supplementary site in a National Botanical Garden (NBG) 20 km southeast of city center—and thus protected from salt contamination and urban air pollution—had been selected in September 2014 (Table S1). Altogether, these eight sites displayed a range of soil contamination representative of Riga's downtown (Cekstere et al., 2008; Cekstere and Osvalde, 2013). In the current study, these eight sites were also used to study foliar injury by aphids and relate it to NaCl contamination in foliage (see below). Out of the seven sites of Riga's downtown, we selected a subset of four, for characterizing changes in the physico-chemical properties of soil horizons and arthropod communities of tree canopy in response to salt contamination (Table S1, Figure S1). At each site, we selected a salt-polluted plot (P) and an uncontaminated plots (U), further away from salt pollution, but otherwise generally similar to the polluted plots close to the pavement. Thus, each of the U plots provided paired controls, being located in a tree alignment close (20–30 m) to the P plot but 5–50 m away from the street and thus preserved from contamination by salt sludge splashing and melting heaps of salt-contaminated snow.
Soil and Foliage Analyses From Polluted and Unpolluted Soils
The soil properties at each P and U plot were characterized by means of one 100 cm deep soil pit excavated in September 2015. The soil profile was described according to the “World reference base for soil resources” (IUSS Working Group WRB, 2015). For each horizon, the texture was assessed directly in the field and about 1 kg of soil was sampled for further physico-chemical analyses. In the laboratory, the soil samples were homogenized, dried at 40°C for 48 h, sieved at 2 mm, and ground using agate mortars for subsequent chemical analyses (see soil analyses, methods and results in Figure S2).
The levels of salt accumulation in the foliage of lime trees were characterized on the basis of 2014 data collection from the framework project, and completed in the case of P and U plots (n = 3 trees per plot) with supplementary assessments in 2015. At each plot, three trees with similar crown condition were randomly selected. One unshaded branch, about 50 cm long, in the lower part of crown canopy was pole-pruned at 3–5 m high within each tree on September 16, 2014 and July 20, 2015. The collected leaf samples were washed in distilled water, dried at 60°C and milled to powder using a laboratory mill (IKA, A11 basic, Germany). Leaf samples were then mineralized by dry-ashing, using concentrated HNO3 vapors and the mineral fraction dissolved using either 3% HCl (Na+) or distilled water (Cl−) and analyzed by flame photometry (Na+) and AgNO3 titration (Cl−) (Rinkis et al., 1987). Along with the leaf sampling of July 2015, three soil core samples (5 cm diameter, 20 cm deep) per site (24 samples in total) were also collected for soil Na+/Cl− concentration measurements. The concentration of Na+/Cl− in the soil samples was determined after 1M HCl/distilled water extraction, followed by flame photometry/AgNO3 titration (Cekstere and Osvalde, 2013). See results for NaCl concentrations in leaves and soil in Table S2, and Figure S1).
De-icing Salt Impact on Arthropods' Biodiversity in the Street Trees' Canopies
At both paired plots within each of the four sites from the downtown subset, the biodiversity of arthropods in the canopy of each tree (n = 3 trees per plot) was assessed by means of passive trapping in July 2015. The custom-made passive traps consisted of a disposable yellow-painted aluminum chafing dish, (18 × 13 and 6 cm deep) filled with 500 ml of a salty solution (10 g/l NaCl and a few drops of liquid soap). Traps were left hanging for 48 h at 3–4 m high in the lower canopy. All trapped arthropods were stored in 70% ethanol prior to counting and classification at the “morpho-species” level. Because all P and U sites were located in the same micro-climatic conditions of open spaces with same sun exposure, and with no high building or other manmade barriers as interference, this approach specifically aimed at comparing P and U plots across sites. However, we did not aim at producing a comprehensive characterization of the lime tree-associated arthropod biodiversity in Riga, which would have required long term monitoring aver the entire season of growth (Basset et al., 1996).
We first estimated the effect of salt contamination on total abundance of arthropods trapped, the abundance of the most frequently trapped arthropod, the lime aphids (Eucallipterus tiliae, Homoptera, Aphididae), and the arthropod Shannon diversity [calculated with the diversity function of R vegan package (Oksanen et al., 2013)] using mixed-effect models [pollution as fixed factor and site as random factor, lmer function in the package lme4 (Bates et al., 2015)]. Second, we estimated the effect of salt contamination (P vs. U) on the amount of arthropods and species composition by means of permutational multivariate ANOVA (PERMANOVA). We used the adonis function in the vegan package for calculating dissimilarity indices between samples based on Euclidean distances, although results were robust to other distance metrics such as Bray-Curtis. Sites were included as strata in the model. Contribution of the different arthropod species to the groups' (P vs. U) separation was analyzed using Regularized Discriminant Analysis (RDA).
Structural Injury in T. x vulgaris Foliage by Arthropods
Structural injury was assessed using foliar samples excised from the 2nd or 3rd leaf from twig apex, within the lower canopy branch harvested at the eight Riga and NBG sites on September 16, 2014 (framework project). Two 10 mm in diameter disks from one leaf per tree (3 trees per site, 48 samples in total) were excised from the median and still asymptomatic part of leaf blade and immediately fixed by immersion in either LM- or EM-grade glutaraldehyde buffered at pH 7.0 using 0.067 M Soerensen phosphate buffer. The disks were then stored at 4°C in renewed fixing solution, waiting for further processing. For descriptive and quantitative histological assessments using light microscopy (LM), one leaf disk per tree was dehydrated with 2-methoxyethanol (3 changes), ethanol, n-propanol, n-butanol (Feder and O'brien, 1968), and embedded in Technovit 7,100 (Kulzer HistoTechnik) resin. At least 10, 1.5 μm thick, semi-thin sections were then cut using a Reichert UltraCut S ultramicrotome and stained in 1% acid fuchsine and 0.05% toluidine blue in acetate buffer pH 4.4 (Feder and O'brien, 1968), prior to mounting in DPX. Observations were performed using the 5×-100× objectives of a Leica microscope Leitz DMRB, and micrographs taken using the INFINITY 2-1R camera and Lumenera Infinity Analyze (release 6.4) software (Lumenera Corp., Ottawa, Canada). For analyzing cellular and subcellular changes on transmitted electron microscopy (TEM), the other 24 leaf disks samples were post-fixed in buffered 2% OsO4, dehydrated by a series of graded ethanol, infiltrated by a series of graded propylene oxide/Epon 812 mixture (with DDSA, NMA, and DMP hardener) and embedded in Epon. Ultra-thin sections (70 nm) were cut using the aforementioned ultramicrotome, mounted on copper grids and stained using uranyl acetate 5% solution in water and lead citrate 2% solution in water (Reynolds procedure). Sections were observed using a Philips CM12 transmission electron microscope (TEM) and micrographs taken using the Gatan Microscopy Suite Software (Gatan Inc., Pleasanton, USA).
Biotic injury and the associated microscopic changes were diagnosed according to characteristic visible and microscopic symptoms (Fink, 1999; Vollenweider and Günthardt-Goerg, 2006; Gunthardt-Goerg and Vollenweider, 2007) and past experience (Cekstere et al., 2016; Vollenweider et al., 2016). Specifically, we quantified several structural markers related to aphid injury, including: percentage of the epidermis length filled with mucilage; frequency of the mucilage spills in the intercellular space; percentage length of necrosis in lower epidermis. After correcting biases to normality (using LOG for Muc_le and SQRT for the rest) in descriptors according to Legendre (1998), we plotted the variables using Principal Component Analysis (PCA, based on a correlation matrix). We next extracted the magnitude of the loadings for each variable and tested the correlation between the variables of the first three most important loadings (Cl−, Muc_uE, and Muc_lE) and the first axis of the PCA using multivariate mixed effect linear models by including sites as random factor [function lmer function in the package lme4 (Bates et al., 2015)]. By including site as random factor, we could account for potential non-independence between the three trees at each site.
Effect of Experimental Salt Exposure on Tilia x Vulgaris Foliage and Resistance
To measure the effect of salt contamination on the development of lime tree aphids under controlled conditions, we performed a common garden experiment using 6 year-old seedlings of Tilia x vulgaris. After planting (spring of 2014) in 10 L plastic pot filled with commercial neutralized peat substrate (KKS-M2, Laflora Ltd., Latvia; pH 5.0 ± 0.3; nutrients: N 80, P 75, K 200, Ca 3,000, Mg 360, S 40, Fe 80, Mn 5.0, Zn 2.20, Cu 2.00, Mo 0.25, B 0.55 mg/L; electric conductivity EC of 1.60 mS/cm), the tree seedlings were left to acclimate in an open greenhouse of the Institute of Biology (UL) in Salaspils, Latvia (56°51'45.5”N 24°21'37.3”E). The plants were kept watered to field capacity (60–70% substrate moisture) and fertilized, according to assessments of substrate and leaf concentration of nutrients, with the addition of macro- and micronutrients being repeated thrice over the vegetation season. On July 1, 2015, a controlled salt exposure experiment with 5 treatments [n = 8 tree replicates per treatment; treatment levels: 0, 10, 20, 30, and 40 mM NaCl, selected based on our previous studies in the street greenery of Riga (Cekstere and Osvalde, 2013)] was started. Over 4 days, NaCl solutions (10 mM NaCl/L) were added to each pot (except the control) to gradually reach the target concentrations of the treatment according to scheme: trees in the 10 mM treatment received 10 mM NaCl solution on day 1 and plain water thereafter; trees in the 20 mM treatment−10 mM NaCl solution on days 1 and 2 and plain water thereafter; trees in the 30 mM treatment−10 mM NaCl solution on days 1, 2, and 3 and plain water thereafter; trees in the 40 mM treatment−10 mM NaCl solution on days 1, 2, 3, and 4. The gradual increment was used to avoid osmotic shock on plants. The treatments were randomized according to a pseudo-latin square design.
The effect of salt contamination on the chlorophyll fluorescence and concentration within seedling foliage was assessed in August 2015. For this, we used two tagged leaves from the first flush per plant. Measurements were performed ten times per leaf, and averaged to a single value per tree. The potential maximum quantum yield PSII (Fv/Fm) and performance index (Pindex) were determined using a HANSATECH Handy-PEA device (Hansatech Instruments Ltd., Pentney, United Kingdom). The chlorophyll content was measured using a chlorophyll meter SPAD-502 device (Konica Minolta, Chiyoda, Tokyo, Japan). The additional leaf functional traits (leaf dry weight, DW; leaf water content, WC; leaf area, LA; specific LA, SLA;) were measured using 8 leaves from first flush per seedling harvested on September 14–16, 2015, and averaged to a single value per tree. The LA estimates were obtained after scanning the leaves at 600 dpi (3,000 × 300 pixels images), using the transmitted light mode of a 2014 EPSON Perfection Scanner and the version 1.2 of Pixstat software (https://www.wsl.ch/en/services-and-products/software-websites-and-apps/pixstat.html). SLA was computed by dividing the LA by DW of each leaf. Finally, we measured the seedling trunk diameter at stem base on September 20, 2015, using a caliper. Because, we used similar size plants at the beginning of the experiment, differences in trunk diameter at the end of the experiment were meant to reflect NaCl treatment effect only. The effect of NaCl treatments (5 levels) on plants functional traits was analyzed using univariate ANOVAs, and differences among levels were assessed using Tukey's HSD post-hoc tests.
The effect of salt treatments from the common garden experiment on aphid development was tested by means of a no-choice cafeteria bioassay realized in July 2016. Prior to the bioassay, salt addition was repeated on May 6–9, using the same procedure as in 2015. One fully-expanded healthy leaf per plant with its petiole base wrapped in wet cotton cloth was placed in a Petri dish lined with humid filter paper and moved into a climate chamber (15–18°C, 55% Rh, and 16 h light). Two alate aphid females were immediately introduced in each Petri dish and the number of parthenogenetic offsprings on each leaf was counted after 5 days. Because we could not reach normality in the residual distribution, to test the effect of salt contamination on final aphid offsprings, we fitted an ANOVA using permutation tests [aovp function in the package lmPerm in R (Wheeler, 2010)]. Differences among salt levels were assessed using TukeyHSD post-hoc tests.
Results
Soil and Foliage Contamination by Deicing Salts in Riga
Deriving from Baltic Ice Lake sandy deposits, the analyzed soils in Riga's downtown showed a primarily sandy texture (>84% sand), with the mean content of organic matter in the topsoil not exceeding 6.5%. As a consequence, the soil structure was generally weak or absent. The soil horizons contained a high proportion of manmade technogenic artifacts (10–40%), typical for anthroposoils (IUSS Working Group WRB, 2015). In general, the soil pH of all the P and U plots was neutral or slightly alkaline with pHH2O ranging from 7.2 to 8.5 and pHKCl from 6.8 to 7.1 (Figure S2). All polluted (P) plots had higher Na+ and Cl− concentrations than unpolluted (U) plots, from the topsoil and down till 60 cm deep. Average Na/Cl concentrations in the topsoil ranged between 97–455/15–74 mg/kg for P plots, and 11–92/10–46 mg/kg for U plots (Table S2). In sum, more than 5.5 times and 2.5 times higher Na and Cl concentrations, respectively were thus measured in the polluted soils compared to unpolluted soils (Cekstere and Osvalde, 2013). This resulted in significant increase in the soil electrical conductivity in polluted soils (max value of 1.09 mS/cm).
Within foliage (2014 assessments), the Na+ and Cl− concentrations at the seven urban sites ranged between 960–9,400 and 2,375–9,000, respectively, compared to 68–151 and 600–2,050 ppm, respectively, for the (unpolluted) NGB site. Na+ and Cl− concentrations were thus 37.7 and 5.4 times higher, respectively, in the polluted compared to unpolluted environments. Compared to soil concentration and considering the four plots only (2015 assessments), the foliage concentration of Na+ and Cl− (3,970 ± 1,954 and 5,281 ± 1,396 ppm, respectively) were 13.3 and 91.8 times larger than those measured in the topsoil (Table S2).
De-icing Salt Impact on Arthropods' Biodiversity in the Street Tree Canopy
Using passive traps we collected about 2,450 specimens that we could divide into 212 morphospecies, consisting of 8 mites (Acaria), 5 spiders (Aranea), 11 beetles (Coleoptera), 73 flies (Diptera), 81 wasps and bees (Hymenoptera), 8 butterflies (Lepidptera), 2 neuropteran, 4 barklice (Psocoptera), 4 thrips (Thysanoptera), and 2 caddisflies (Trichoptera) species were observed in lime trees in the summer of 2015. Overall, we found 36% more arthropod individuals at the U vs. P plots (Figure 1A, pollution effect; Chisq = 3.65, p = 0.05). With 47% of all individuals on average, the lime aphid E. tiliae (Homoptera, Aphididae) formed the arthropod species most frequently observed in the traps. In a statistically non-significant trend, E. tiliae also tended to be 1.5 times more abundant in U plots than P plots (Figure 1B, pollution effect; Chisq = 3.15, p = 0.07). The arthropod diversity, as measured by Shannon index, remained similar across pollution plots (Figure 1C, pollution effect; Chisq = 0.03, p = 0.84). However, changes in the arthropod communities, considering their abundance together with their diversity, significantly responded to site contamination [Figure 1D, PERMANOVA, pollution effect; F(1, 19) = 1.55, p = 0.02]. The strongest explanatory variable in the RDA was the lime aphid with 11.4% of the total contribution to the first axis of the ordination (Figure 1D).
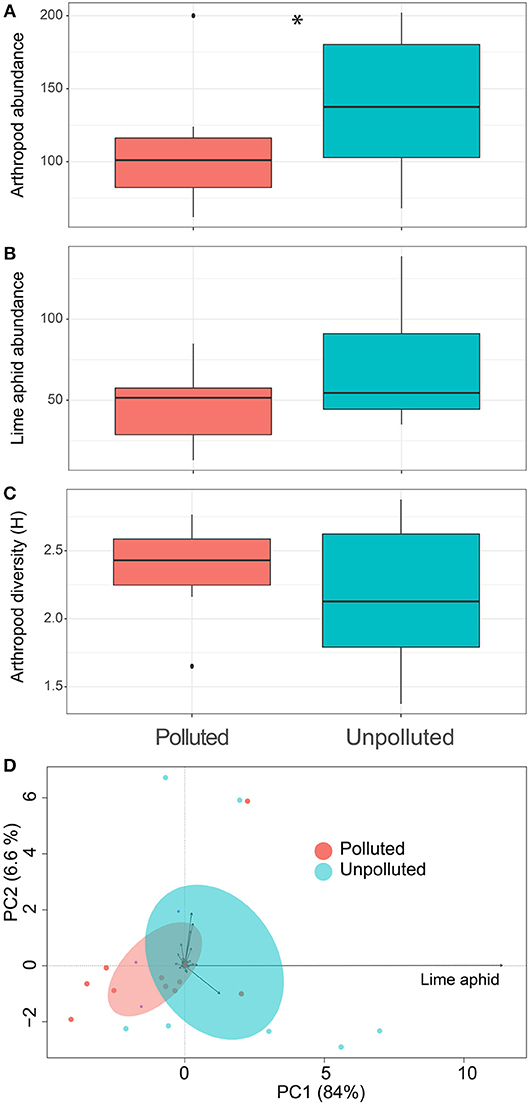
Figure 1. Effect of salt pollution on lime trees associated arthropod diversity. Boxplots show the effect of soil pollution on (A) total arthropod abundance, (B) lime aphid abundance, and (C) arthropod Shannon diversity. Asterisks show significant differences among pollution sites (Chi-squared test, p < 0.05). Panel (D) shows the principal component arthropod's ordination of the different sites. Blue is unpolluted soil, while red indicates highly polluted soils. Ellipses show 95% confidence intervals around the pollution groups.
Structural Injury in T. x vulgaris Foliage by Arthropods
The leaves sampled in Riga and at NBG were generally sticky and shiny because of aphid honeydew and showed a rather leathery texture (field observations). Disk cross-sections showed many injuries at tissue and cell level (Figure 2). Typical of biotic injury, their distribution and severity were very variable, even within a single leaf section (about 5 mm long). In the leaf blade, they included an increased frequency of mucilage filling in the upper (Figures 2B,C,F,G vs. 2A) and lower (Figures 2B,D vs. 2A) epidermis. This compound identification was confirmed by (1) cell wall-like hues after toluidine blue staining (Figures 2B–D), (2) accumulation in periplasm (Figures 2F,G), or (3) granular ultrastructure and banding patterns. On average, 39.9 ± 1.8% (range: 27.3–59.1%) and 7.2 ± 1.1% (range: 1.3–23.7%) of upper and lower epidermis segments in leaf cross-sections were filled with mucilage. The frequency of intercellular mucilage in mesophyll amounted to 1.7 ± 0.3 spills mm−1. The PCA analysis showed that Cl (29.3), Muc_uE (27.4), and Muc_lE (23.3) were mostly contributing to the first axis of the ordination (Figure 3). Subsequent multivariate analysis identified a significant positive correlation between foliar salt contamination (Cl−) and first axis of the PCA [t(13) = 51.27, p < 0.001], and a negative correlation between Muc_lE and the first axis of the PCA [t(13) = 2.26, p = 0.04]. In other words, the frequency of mucilage-filled cells is higher in leaves growing in unpolluted sites, where herbivory is likely higher (e.g., National Botanical Garden, Figure 3).
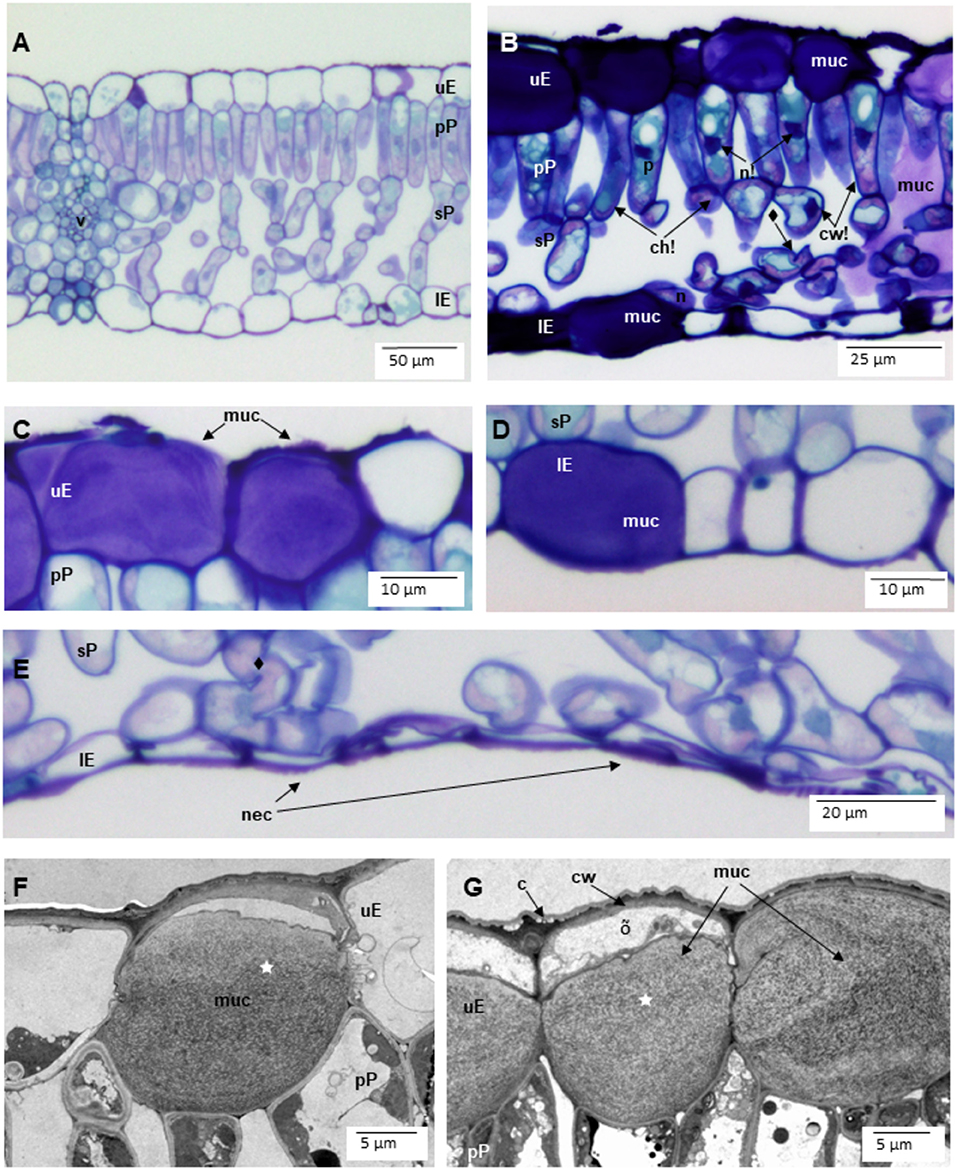
Figure 2. Symptoms of biotic injury, primarily by aphids, in leaves of Tilia x vulgaris trees from the street greenery of Riga and National Botanic Garden (NBG) in Latvia. (A) nearly asymptomatic leaf tissues. (B) in the leaf blade, the severely affected leaf parts showed a higher frequency of mucilages (muc) within upper (uE), and lower (lE) epidermis as well as mucilage spills in the intercellular space of palisade (pP) and spongy (sP) parenchyma. Mesophyll cells showed cell wall thickening (cw!), nucleus (n!), and chloroplast (ch!) condensation as well as increased amounts of vacuolar phenolics (p). Some cells were partially cytorrhised (♦). (C,D) mucilage structure in upper (C) and lower (D) epidermis (lE). (E) cytorrhisis indicative of necrosis (nec) in lower epidermis. (F,G) mucilage within epidermal cells showed a mostly granular structure, with banding patterns (⋆). Cell death was evidenced by disrupted protoplasm remnants (õ). Other abbreviations: c cuticula. Na, Cl concentration: 2,660, 5,000 ppm (A), 124, 5,000 ppm (B), 72, 530 ppm (C,D), 8,800, 6,896 ppm (E), 4,180, 11,500 (F), 5200, 10750 (G). Technical specifications: (A–E): 1.5 μm semi-thin cuttings stained with toluidine blue and acid fuchsine and observed in diascopic light microscopy; (F,G): 70 nm ultra-thin sections stained with uranyl acetate and lead citrate and observed in transmission electron microscopy.
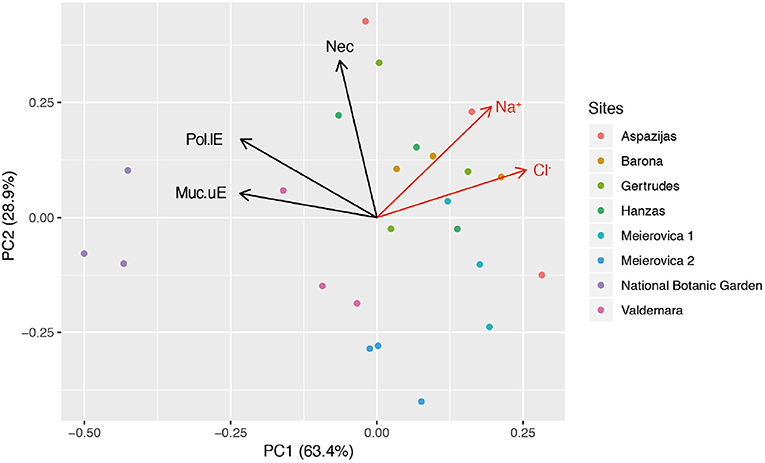
Figure 3. Unconstrained ordination (PCA) of structural parameters indicative of biotic injury (Muc_lE, Muc_uE, Nec_lE) in the plane formed by the first and second principal components. Na/Cl contamination within lime tree foliage at the research sites: (i) uncontaminated, below 98/940 ppm, NBG; (ii) moderate, contamination range 118–3,100/3,000–7,570 ppm, Valdemara, Meierovica 2; (iii) severe, contamination range 2,660–6,600/5,000–11,500 ppm, Barona, Meierovica 1; (iv) very high, contamination range 5,400–13,600/5,250–16,750 ppm, Gertrudes, Hanzas, Aspazijas. Abbreviations for the descriptor variables: Muc_lE/uE percentage length of lower/upper epidermis filled with mucilages, Nec_lE percentage length of lower epidermis showing necrosis, n = 3 tress per site.
Responses in Foliage of T. x vulgaris to Experimental Salt Exposure
The salt treatments affected the leaf physiology as well as morphology of salt-exposed seedlings (Figure 4). As indicated by the monotonic reduction of chlorophyll concentration [Figure 4A; F(4, 32) = 4.02, p = 0.01] and fluorescence [Figure 4B; F(4, 32) = 4.90, p = 0.003], both the chloroplast structure and functioning were impaired with increasing salt concentration. Significant correlation between the two latter variables (Figure S3) suggested a possible causal link. We found no effect of salt treatment on plant biomass [Figure 4C; F(4, 32) = 2.36, p = 0.07], but found that plants under high salt stress had almost half the amounts of water in their tissues than control plants [Figure 4D; F(4, 32) = 3.12, p = 0.03]. We also found no effect of salt stress on the diameter of the trunk, nor the leaf area (LA) (Figures 4E,F, p > 0.05, respectively). The most striking morphological change in response to salt exposure thus consisted in the monotonic increase of SLA [F(4, 32) = 14.78, p < 0.001; Figure 4G]. As a likely consequence of increasingly larger necrotic leaf rims [F(4, 32) = 4.64, p = 0.004; Figure 4H], the WC was decreased up to two third [F(4, 32) = 3.12, p = 0.03; Figure 4D], nevertheless not monotonically.
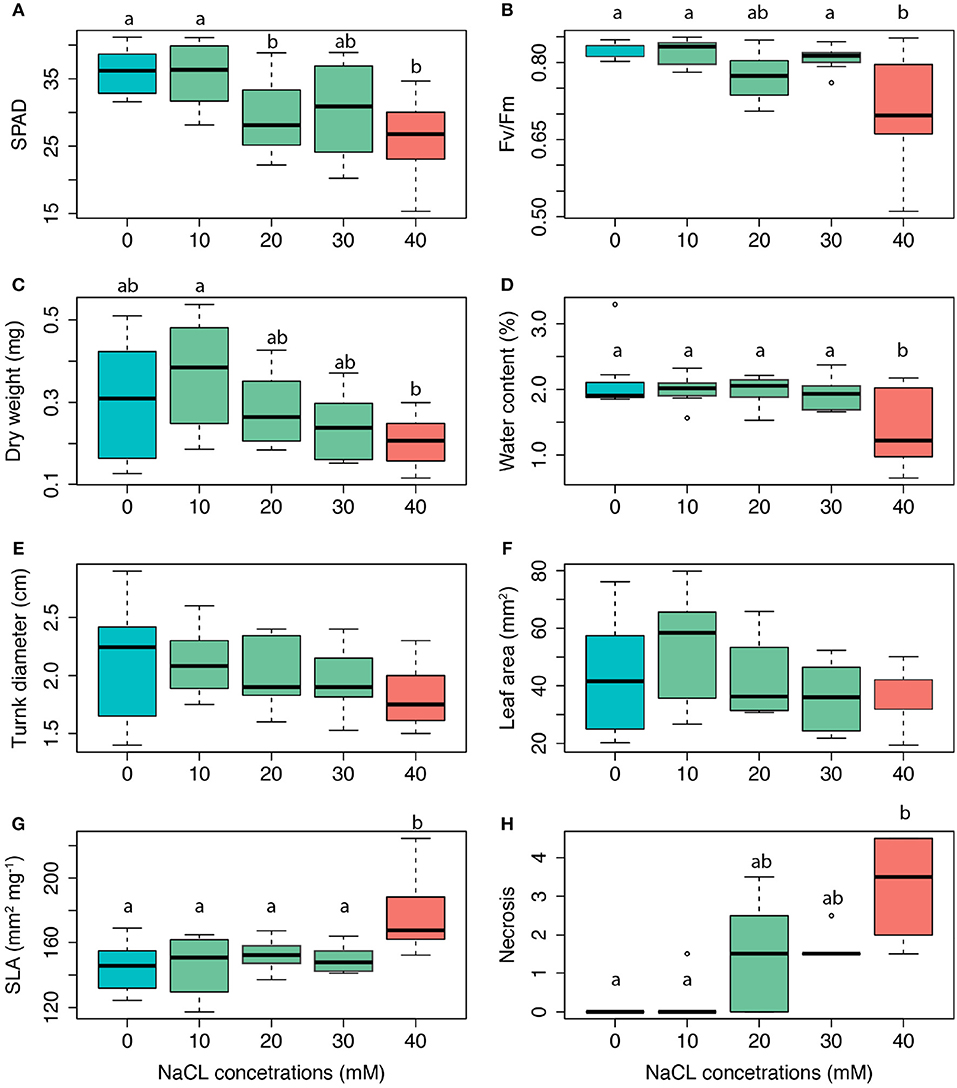
Figure 4. Changes in functional traits of Tilia x vulgaris seedlings in response to four salt addition treatments (0–40 mM NaCl). (A) chlorophyll content (SPAD units), (B) Fv/Fm potential maximum quantum yield of PSII, (C) DW leaf dry weight (mg), (D) WC water content (%), (E) trunk diameter at the base (cm); (F) LA leaf area (mm2), (G) SLA specific leaf area (mm2 mg−1), (H) leaf injury (necrosis %). Different letters indicate significant difference among the salt treatments (Tukey's HSD post-hoc test, p < 0.05).
Development of Aphid Colonies as a Function of Salt Exposure
The exposure of lime tree seedlings to salt significantly reduced the development of aphid colonies [F(4, 7) = 7.3, nb of iterations = 5,000, p = 0.01; Figure 5]. After 5 days of development, the aphid offspring on salt-exposed vs. control leaves was 2.6 times lower. However, this offspring reduction was not proportional, in which all salt-addition treatments resulted in no variation in aphid development.
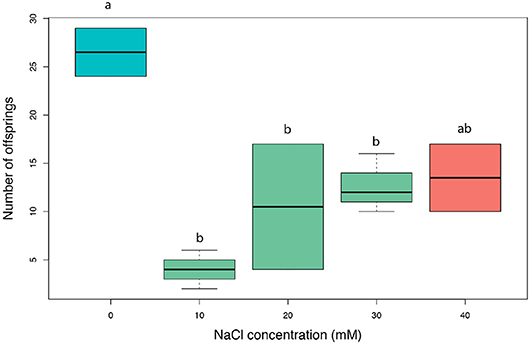
Figure 5. Amount of lime aphid (Eucallipterus tiliae) offsprings on single detached leaves of Tilia x vulgaris leaves from seedlings exposed to 0–40 mM NaCl, after 5 days of colony development.
Discussion
With this study we highlighted a cascade of events that starts with the application of de-icing salt to the streets of Riga in winter, followed by salt pollution in urban soils, and in the subsequent accumulation foliage of trees on the roadsides. Salt accumulation in trees modifies leaf-ecopyhsiological parameters to largely mimic drought and general stress injuries. Ultimately, this results in trees from salt-polluted habitats bearing lower arthropod numbers, including specialized herbivores (Figure 6). Below we discuss each step along the way.
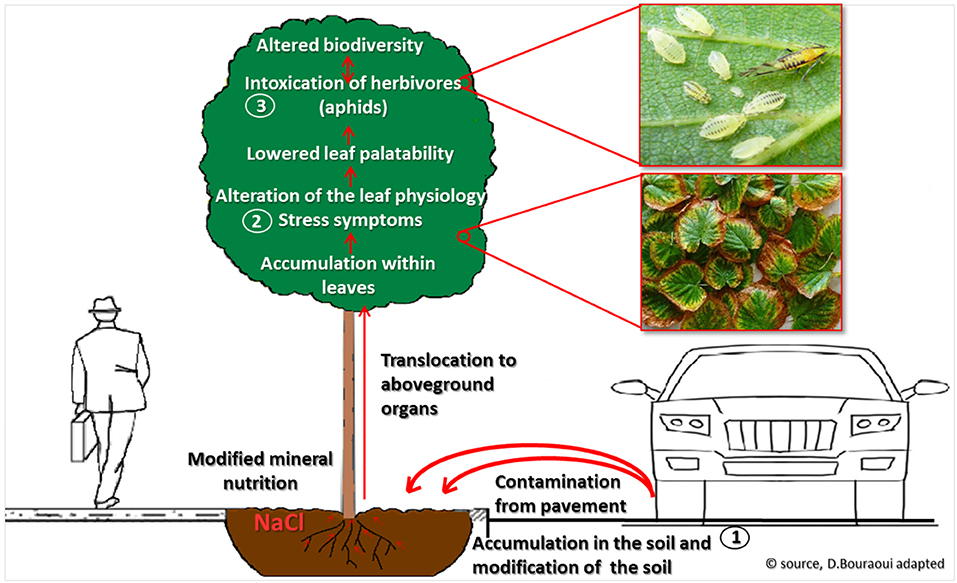
Figure 6. Cascading effects de-icing salts on street greenery and biodiversity, from soil infiltration and contamination (1), to foliage injury (2), and to altered diversity of arthropods communities in the canopy (3).
Effect of Salt Pollution on Urban Soils
We found no effect of soil pollution on the soil physical structure. This result is likely driven by a general lack of soil structure in such sandy soils. The missing salt disruptive effect on soil structure therefore means that soil aggregation, porosity, and the soil moisture availability were also not affected by salt pollution; something that was shown in more structured urban soils of other cities (Tedeschi and Dell'Aquila, 2005; Legros, 2007; Rengasamy, 2010). Clearly, the most important consequence of salt pollution was the increase up to 10-folds in Na+ and Cl− soil concentrations, a recurrent observation for similar sampling along urban street greeneries (Czerniawska-Kusza et al., 2004; Cekstere et al., 2008; Fay and Shi, 2012; Dmuchowski et al., 2014). Given soil sampling in the fall (at the beginning of September) however, the concentration of salt contaminants during foliage development in spring was certainly underestimated, as a consequence of tree uptake and leaching (Cekstere et al., 2008). The highest concentration for Na+ cations within the topsoil (A horizon) vs. deeper subsoil (C and Z horizon) for Cl− anions was indicative of the fact that Na+ ions are retained by the negatively charged argilo-humic soil complexes, whereas the free Cl− ions remain in the soil solution (Cekstere et al., 2008; Fay and Shi, 2012). Since Na+ and Cl− concentrations were correlated to pH and EC, respectively, these parameters could show the same pattern of variation across the soil profile. In sum, salt contaminants spread on roadsides effectively infiltrate the surrounding urban soils, in turn modifying the soil physicochemical properties. The roadside plants collect these contaminants with their root systems and accumulate them in foliage during the subsequent growth season.
Effect of Salt Pollution on Plant Eco-Physiological Traits
On the experimentally salt-exposed seedlings, increasing leaf injury with increasing salt addition led to symptoms of leaves being morphologically similar to symptoms of leaves observed in the field, which confirmed the causal role and negative impact of salt accumulation on street-lining lime trees, as classically described by Bernstein (1975). In this instance, the T. x vulgaris species studied appears to be more salt-sensitive than other tree species commonly used in street greeneries (Paludan-Müller et al., 2002; Marosz and Nowak, 2008; Kwasowski and Czyz, 2010; Dmuchowski et al., 2013). For example, Populus talassica and P. euphratica showed salt symptoms after 8 days of exposure to 206 mM and 354 mM NaCl, respectively (Chen et al., 2001), vs. 7 days to 40 mM NaCl in the case of our T. x vulgaris common garden seedlings. Initial injury was observed for leaf dry matter content of Cl− exceeding 0.4% (10 mM treatment), just above the normal level of Cl− in Tilia leaves −0.3%—reported by Dmuchowski et al. (2014). Interestingly, such Cl− leaf dry matter contents was sizably lower than that one previously observed causing leaf injury in foliage of Tilia x vulgaris from Riga's street greeneries (0.66% Cekstere et al., 2008). The main difference between these two studies is that our seedlings were exposed to NaCl after full foliage development. Lower sensitivity of street foliage may then relate to morphological and physiological adjustments during, for instance, leaf differentiation, thus conferring better stress tolerance (Bernstein, 1975; Abrams, 1994; Künnemann and Gad, 1997; Shannon, 1997; Munns, 2002). The negative impact of salt accumulation on leaf functional traits, primarily the photosynthetic apparatus, was in agreement with findings in other studies (Brugnoli and Lauteri, 1991; Maxwell and Johnson, 2000; Parida and Das, 2005; Volpe et al., 2011; Negrão et al., 2017). With chronic salt exposure and besides phenological adjustments, stress-related changes in morpho-anatomical properties, mostly missing in the case of late exposure of seedlings in the common garden experiment, may thus be expected in street greeneries.
Effect of Salt Pollution on Canopy Arthropod Community
We found that trees growing on salt-polluted plots sustained lower overall arthropod abundance, but not lower diversity (Shannon diversity index). In comparing the otherwise highly similar unpolluted and polluted plots, leaves from salt-stressed plant contained lower amounts of chlorophyll, and therefore, likely, lower amount of N (Wright et al., 2004). Moreover, the reduction in water content of leaves was also shown to interfere with the herbivores ‘capacity to access drought-enhanced foliar nitrogen (Koricheva et al., 1998). Therefore, reduced arthropod abundance in salt-stressed plants could be partially explained by a reduction in N and water availability in the leaves, ultimately resulting in lower arthropod abundance overall. This assumption thus aligns with the plant vigor hypothesis (Price, 1991), and not with mere changes in microhabitat between U and P plots. The fact that the tree physiology, and not a potentially slightly warmer microclimate near the streets of the polluted trees drove our results, is supported by the fact that arthropods tend to gather in warmer microhabitats (Hodkinson, 2005). The warming effect would have resulted in more arthropods near the streets, and not the opposite as we observed. Finally, the incongruent relationship between insect abundance and diversity results were in turn somewhat surprising, since species diversity is generally positively linked to abundance (Bock et al., 2007), but not unusual for urban ecosystems (Marzluff, 2001; Shochat et al., 2006). In our case, diversity is the same across sites, or even tend to be higher in polluted sites. Since the specialist lime aphid abundance followed the expected trends, we could hypothesize that salt-polluted trees are less palatable for a more generalist arthropod community.
Structural Injury in T. x vulgaris Foliage by Arthropods
The microscopic analyses showed clear evidence of a reduction in aphid injury symptoms, paralleling with the decrease in aphid frequency, and with the increase of salt contamination in soils. Our findings are in good agreement with the research results conducted on Tilia “Euchlora” in street greenery (Sienkiewicz-Paderewska et al., 2017), where aphid's frequency was most strongly negatively affected by Cl− content in the leaves. Interestingly, among the three quantified markers of biotic injury, the mucilage frequency in lower epidermis was the parameter best correlating with Cl−, considered as more toxic than Na+ (Paludan-Müller et al., 2002; Dmuchowski et al., 2014). This finding was also consistent with the development of aphid colonies on the lower leaf side, as generally observed in the field. While there is some evidence of epidermal mucilages production coincident with leaf water loss (Lyshede, 1977; Mariani et al., 1988; Günthardt-Goerg et al., 1993), we here provide a first, yet still indirect, evidence of mucilage spilling in relation to aphid injury (Fink, 1999; Gunthardt-Goerg and Vollenweider, 2007). In other words, we showed that leaf from salt-polluted plants that are less damaged by aphids produced lower amounts of mucilage. This finding thus indicates that aphid injury stimulates mucilage spilling, and should be seen as an inducible defense mechanisms against aphid feeding in plants (Fink, 1999). Mucilage accumulation can confer the injured leaves a more leathery texture and contribute to increasing the leaf mass per area, a typical herbivory deterring reaction (Westoby et al., 2002). The principal role of leaf epidermis mucilage may thus primarily relate to leaf defense and not water homeostasis; similarly to pathologically induced mucilages in other plant organs (Fink, 1999). This determinant role of pathogens vs. water homeostasis is particularly corroborated in the context of our experiment, with the frequency of epidermis mucilage being decreased whereas the “physiological drought” triggered by salt was increased (Dobson, 1991).
Conclusion
We showed that salt accumulation in the street greeneries of Riga caused leaf injury, and affected the arthropods associated with this urban street ecosystem. Street lime trees showed high NaCl concentrations in their foliage, with physiological and structural reactions similar to those observed in street greeneries of other affected cities. The arthropod communities living in close contact with the street greeneries also showed changes. Therefore, the advocated replacement of sensitive lime trees by more tolerant tree species needs testing regarding consequences for all ecosystem services, also including urban biodiversity. Especially in historical centers, more sensitive lime trees are likely to be maintained for cultural reasons. Comparisons of performances between lime tree species and cultivars are thus also needed. Promoting more tolerant lime trees may thus also help to maintain specific lime tree extended arthropod communities, as an integral part of the environmental value of the urban ecosystem at mid-latitudes.
Data Availability
This manuscript contains previously unpublished data. The name of the repository and accession number are not available.
Author Contributions
DB performed the experiments, collected, and analyzed the data. GC designed the experiments and helped collecting and analyzing the data. AO supervised data collection and analysis. PV designed the experiments and supervised the data collection and analysis. SR designed the experiments, analyzed the data. DB and SR wrote the first draft of the manuscript. All authors contributed to the writing.
Funding
This work was supported by the Sciex-NMSch grant 14.038 to PV, Swiss National Science Foundation Grant 159869 to SR, COST action FP1204 (COST-STSM-ECOST-STSM-FP1204-010815-063102) to DB, and the Basic Research Funding (University of Latvia) for the project ZD2015/AZ81 to GC and AO.
Conflict of Interest Statement
The authors declare that the research was conducted in the absence of any commercial or financial relationships that could be construed as a potential conflict of interest.
Acknowledgments
We thankfully acknowledge technical support by Terry Menard (light and electron microscopy), Patrick Schleppi (leaf morphology), Andis Karlsons (arthropod sampling, field works of soil), Guntis Tabors (field works of soil), and the Center for Microscopy and Image Analysis of the University of Zurich (transmission electron microscopy).
Supplementary Material
The Supplementary Material for this article can be found online at: https://www.frontiersin.org/articles/10.3389/fevo.2019.00282/full#supplementary-material
References
Abrams, M. D. (1994). Genotypic and phenotypic variation as stress adaptations in temperate tree species: a review of several case studies. Tree Physiol. 14, 833–842. doi: 10.1093/treephys/14.7-8-9.833
Araya, F., Abarca, O., Zuñiga, G., and Corcuera, L. (1991). Effects of NaCl on glycine-betaine and on aphids in cereal seedlings. Phytochemistry 30, 1793–95. doi: 10.1016/0031-9422(91)85014-Q
Ashraf, M., and Harris, P. J. C. (2004). Potential biochemical indicators of salinity tolerance in plants. Plant Sci. 166, 3–16. doi: 10.1016/j.plantsci.2003.10.024
Baczewska, A. H., Dmuchowski, W., Gozdowski, D., Styczek, M., and Bragoszewska, P. (2011). “Influence of saline stress on the abundance of lime aphid (Eucallipterus tiliae L.) on the leaves of street trees-crimean linden,” in Proceedings of Ecopole, 5, 3–19.
Bano, A., Dorffling, K., Bettin, D., and Hahn, H. (1993). Abscisic acid and cytokinins as possible root-to-shoot signals in xylem sap of rice plants in drying soil. Funct. Plant Biol. 20, 109–115. doi: 10.1071/PP9930109
Basset, Y., Springate, N. D., Aberlenc, H. P., and Delvare, G. (1996). “A review of methods for sampling arthropods in tree canopies,” in Canopy arthropods, eds N.E. Stork, J. Adis and R.K. Didham (London, UK: Chapman and Hall), 35.
Bates, D., Maechler, M., Bolker, B., Walker, S., Christensen, R. H. B., and Singmann, H. (2015). lme4: linear mixed-effects models using Eigen and S4. R Package Version 2014, 1.1–9. doi: 10.18637/jss.v067.i01
Berkheimer, S. F., and Hanson, E. (2006). Deicing salts reduce cold hardiness and increase flower bud mortality of highbush blueberry. J. Am. Soc. Horticult. Sci. 131, 11–16. doi: 10.21273/JASHS.131.1.11
Bernstein, L. (1975). Effects of salinity and sodicity on plant growth. Annu. Rev. Phytopathol. 13, 295–312. doi: 10.1146/annurev.py.13.090175.001455
Bernstein, L., and Hayward, H. E. (1957). Physiology of salt tolerance. Annu. Rev. Plant Physiol. 9, 25–46. doi: 10.1146/annurev.pp.09.060158.000325
Blomqvist, G., and Johansson, E.-L. (1999). Airborne spreading and deposition of de-icing salt: a case study. Sci. Total Environ. 235, 161–168. doi: 10.1016/S0048-9697(99)00209-0
Bock, C. E., Jones, Z. F., and Bock, J. H. (2007). Relationships between species richness, evenness, and abundance in a southwestern savanna. Ecology 88, 1322–1327. doi: 10.1890/06-0654
Bolund, P., and Hunhammar, S. (1999). Ecosystem services in urban areas. Ecol. Econom. 29, 293–301. doi: 10.1016/S0921-8009(99)00013-0
Brugnoli, E., and Lauteri, M. (1991). Effects of salinity on stomatal conductance, photosynthetic capacity, and carbon isotope discrimination of salt-tolerant (Gossypium hirsutum L.) and salt-sensitive (Phaseolus vulgaris L.) C(3) non-halophytes. Plant Physiol. 95, 628–635. doi: 10.1104/pp.95.2.628
Bryson, G. M., and Barker, A. V. (2002). Sodium accumulation in soils and plants along Massachusetts roadsides. Commun. Soil Sci. Plant Anal. 33, 67–78. doi: 10.1081/CSS-120002378
Cekstere, G., Karlsons, A., and Grauda, D. (2015). Salinity-induced responses and resistance in Trifolium repens L. Urban For. Urban Green. 14, 225–236. doi: 10.1016/j.ufug.2015.02.010
Cekstere, G., Nikodemus, O., and Osvalde, A. (2008). Toxic impact of the de-icing material to street greenery in Riga, Latvia. Urban For. Urban Green. 7, 207–217. doi: 10.1016/j.ufug.2008.02.004
Cekstere, G., and Osvalde, A. (2013). A study of chemical characteristics of soil in relation to street trees status in Riga (Latvia). Urban For. Urban Green. 12, 69–78. doi: 10.1016/j.ufug.2012.09.004
Cekstere, G., Osvalde, A., and Vollenweider, P. (2016). De-icing salt impact on leaves of street trees (Tilia x vulgaris) in Riga, Latvia. Acta Biol. Universit. Daugavpil. 16, 31–38.
Chen, S., Li, J., Wang, S., Hüttermann, A., and Altman, A. (2001). Salt, nutrient uptake and transport, and ABA of Populus euphratica; a hybrid in response to increasing soil NaCl. Trees 15, 186–194. doi: 10.1007/s004680100091
Chen, W. Y., and Jim, C. (2008). Assessment and valuation of the ecosystem services provided by urban forests. Ecol. Plann. Manage. Urban For. 53–83. doi: 10.1007/978-0-387-71425-7_5
Cohen, J. E. (2003). Human population: the next half century. Science 302, 1172–1175. doi: 10.1126/science.1088665
Costanza, R., and Folke, C. (1997). Valuing ecosystem services with efficiency, fairness and sustainability as goals. Nat. Serv. 49–70.
Czerniawska-Kusza, I., Kusza, G., and Duzynski, M. (2004). Effect of deicing salts on urban soils and health status of roadside trees in the Opole region. Environ. Toxicol. 19, 296–301. doi: 10.1002/tox.20037
Davies, W. J., Metcalfe, J., Lodge, T. A., and da Costa, A. R. (1986). Plant growth substances and the regulation of growth under drought. Funct. Plant Biol. 13, 105–125. doi: 10.1071/PP9860105
Dmuchowski, W., Baczewska, A. H., Gozdowski, D., and Bragoszewska, P. (2013). Effect of salt stress on the chemical composition of leaves of different trees species in urban environment. Fresen. Environ. Bull. 22, 987–994.
Dmuchowski, W., Baczewska, A. H., Gozdowski, D., Rutkowska, B., Szulc, W., Suwara, I., et al. (2014). Effect of salt stress caused by deicing on the content of microelements in leaves of linden. J. Elementol. 19, 65–79. doi: 10.5601/jelem.2014.19.1.588
Fay, L., and Shi, X. (2012). Environmental impacts of chemicals for snow and ice control: state of the knowledge. Water Air Soil Poll. 223, 2751–2770. doi: 10.1007/s11270-011-1064-6
Feder, N., and O'brien, T. P. (1968). Plant microtechnique: some principles and new methods. Am. J. Bot. 55, 123–142.
Görür, G. (2006). Effects of heavy metal accumulation in host plants to cabbage aphid (Brevicoryne brassicae) morphology. Ekologia 25, 314–321.
Günthardt-Goerg, M. S., Matyssek, R., Scheidegger, C., and Keller, T. (1993). Differentiation and structural decline in the leaves and bark of birch (Betula pendula) under low ozone concentrations. Trees 7, 104–114. doi: 10.1007/BF00225477
Gunthardt-Goerg, M. S., and Vollenweider, P. (2007). Linking stress with macroscopic and microscopic leaf response in trees: new diagnostic perspectives. Environ. Pollut. 147, 467–488. doi: 10.1016/j.envpol.2006.08.033
Hodkinson, I. D. (2005). Terrestrial insects along elevation gradients: species and community responses to altitude. Biol. Rev. 80, 489–513. doi: 10.1017/S1464793105006767
Huberty, A. F., and Denno, R. F. (2004). Plant water stress and its consequences for herbivorous insects: a new synthesis. Ecology 85, 1383–1398. doi: 10.1890/03-0352
Hunter, M. D., and Price, P. W. (1992). Playing chutes and ladders—heterogeneity and the relative roles of bottom-up and top-down forces in natural communities. Ecology 73, 724–732.
Inada, N., Sakai, A., Kuroiwa, H., and Kuroiwa, T. (1998). Three-dimensional analysis of the senescence program in rice (Oryza sativa L.) coleoptiles. Planta 206, 585–597. doi: 10.1007/s004250050436
IUSS Working Group WRB (2015). World Reference Base For Soil Resources 2014, Update 2015: International Soil Classification System for Naming Soils and Creating Legends for Soil Maps. World Soil Resources Reports No. 106, 192. FAO, Rome.
Klemm, W., Heusinkveld, B. G., Lenzholzer, S., and van Hove, B. (2015). Street greenery and its physical and psychological impact on thermal comfort. Landsc. Urban Plan. 138, 87–98. doi: 10.1016/j.landurbplan.2015.02.009
Koricheva, J., Larsson, S., and Haukioja, E. (1998). Insect performance on experimentally stressed woody plants: a meta-analysis. Annu. Rev. Entomol. 43, 195–216. doi: 10.1146/annurev.ento.43.1.195
Kottek, M., Grieser, J., Beck, C., Rudolf, B., and Rubel, F. (2006). World map of the köppen-geiger climate classification updated. Meteorol. Zeitschr. 15, 259–263. doi: 10.1127/0941-2948/2006/0130
Künnemann, T.-D., and Gad, G. (1997). Überleben Zwischen Land und Meer: Salzwiesen. Oldenburg: Verlag Isensee.
Kwasowski, W., and Czyz, M. (2010). Reaction of lime trees (Tilia sp.) growing along the Zwirki i Wigury Street in Warsaw on soil salinity caused by chemical technology of snow removal. Ecol. Quest. 14:81. doi: 10.12775/v10090-011-0023-6
Larsson, S. (1989). Stressful times for the plant stress: insect performance hypothesis. Oikos 56, 277–283. doi: 10.2307/3565348
Lyshede, O. B. (1977). Studies on the mucilaginous cells in the leaf of Spartocytisus filipes W.B. Planta 133, 255–260. doi: 10.1007/BF00380686
Mariani, P., Rascio, N., Baldan, B., Paiero, P., and Urso, T. (1988). Epidermal mucilage cells in leaves of Salix species. Flora 181, 137–145. doi: 10.1016/S0367-2530(17)30359-6
Marosz, A., and Nowak, J. S. (2008). Effect of salinity stress on growth and macroelements uptake of four tree species. Dendrobiology 59, 23–29.
Martel, J. (1998). Plant-mediated effects of soil salinity on a gall-inducing caterpillar Epiblema scudderiana (Lepidoptera: Tortrieidae) and the in?uence of feeding guild. Eur. J. Entomol. 95, 545–557.
Marzluff, J. M. (2001). “Worldwide urbanization and its effects on birds,” in Avian Ecology and Conservation in an Urbanizing World, eds J.M. Marzluff, R. Bowman and R. Donnelly (Boston, MA: Springer US), 19–47. doi: 10.1007/978-1-4615-1531-9_2
Maxwell, K., and Johnson, G. N. (2000). Chlorophyll fluorescence—a practical guide. J. Exp. Bot. 51, 659–668. doi: 10.1093/jexbot/51.345.659
Munns, R. (2002). Comparative physiology of salt and water stress. Plant Cell Environ. 25, 239–250. doi: 10.1046/j.0016-8025.2001.00808.x
Muñoz, P. T., Torres, F. P., and Megías, A. G. (2014). Effects of roads on insects: a review. Biodivers. Conserv. 24, 659–682. doi: 10.1007/s10531-014-0831-2
Negrão, S., Schmöckel, S. M., and Tester, M. (2017). Evaluating physiological responses of plants to salinity stress. Ann. Bot. 119, 1–11. doi: 10.1093/aob/mcw191
Oksanen, J., Blanchet, F. G., Kindt, R., Legendre, P., Minchin, P. R., O'Hara, R. B., et al. (2013). “vegan: Community Ecology Package”. 2.0-10 ed. Available online at: http://vegan.r-forge.r-project.org/ (accessed January 04, 2013).
Paludan-Müller, G., Saxe, H., Pedersen, L. B., and Randrup, T. B. (2002). Differences in salt sensitivity of four deciduous tree species to soil or airborne salt. Physiol. Plant. 114, 223–230. doi: 10.1034/j.1399-3054.2002.1140208.x
Parida, A. K., and Das, A. B. (2005). Salt tolerance and salinity effects on plants: a review. Ecotoxicol. Environ. Saf. 60, 324–349. doi: 10.1016/j.ecoenv.2004.06.010
Pospíšilová, J., Synková, H., and Rulcová, J. (2000). Cytokinins and water stress. Biol. Plant. 43, 321–328. doi: 10.1023/A:1026754404857
Price, P. W. (1991). The plant vigor hypothesis and herbivore attack. Oikos 62, 244–251. doi: 10.2307/3545270
Ramakrishna, D. M., and Viraraghavan, T. (2005). Environmental impact of chemical deicers–a review. Water Air Soil Pollut. 166, 49–63. doi: 10.1007/s11270-005-8265-9
Rengasamy, P. (2010). Soil processes affecting crop production in salt-affected soils. Funct. Plant Biol. 37, 613–620. doi: 10.1071/FP09249
Seamans, G. S. (2013). Mainstreaming the environmental benefits of street trees. Urban For. Urban Green. 12, 2–11. doi: 10.1016/j.ufug.2012.08.004
Shannon, M. C. (1997). Adaptation of plants to salinity. Adv. Agron. 60, 75–120. doi: 10.1016/S0065-2113(08)60601-X
Shochat, E., Warren, P. S., Faeth, S. H., McIntyre, N. E., and Hope, D. (2006). From patterns to emerging processes in mechanistic urban ecology. Trends Ecol. Evol. 21, 186–191. doi: 10.1016/j.tree.2005.11.019
Sienkiewicz-Paderewska, D., Dmuchowski, W., Baczewska, A. H., Bragoszewska, P., and Gozdowski, D. (2017). The effect of salt stress on lime aphid abundance on Crimean linden (Tilia ‘Euchlora') leaves. Urban For. Urban Green. 21, 74–79. doi: 10.1016/j.ufug.2016.11.010
Sudhir, P., and Murthy, S. D. S. (2004). Effects of salt stress on basic processes of photosynthesis. Photosynthetica 42, 481–486. doi: 10.1007/S11099-005-0001-6
Tedeschi, A., and Dell'Aquila, R. (2005). Effects of irrigation with saline waters, at different concentrations, on soil physical and chemical characteristics. Agricult. Water Manage. 77, 308–322. doi: 10.1016/j.agwat.2004.09.036
Thaiutsa, B., Puangchit, L., Kjelgren, R., and Arunpraparut, W. (2008). Urban green space, street tree and heritage large tree assessment in Bangkok, Thailand. Urban For. Urban Green. 7, 219–229. doi: 10.1016/j.ufug.2008.03.002
Vollenweider, P., and Günthardt-Goerg, M. S. (2005). Diagnosis of abiotic and biotic stress factors using the visible symptoms in foliage. Environ. Pollut. 137, 455–465. doi: 10.1016/j.envpol.2005.01.032
Vollenweider, P., and Günthardt-Goerg, M. S. (2006). Erratum to “Diagnosis of abiotic and biotic stress factors using the visible symptoms in foliage” [Environ. Pollut. 137 (2005) 455–465]. Environ. Pollut. 140, 562–571. doi: 10.1016/j.envpol.2006.01.002
Vollenweider, P., Menard, T., Arend, M., Kuster, T. M., and Günthardt-Goerg, M. S. (2016). Structural changes associated with drought stress symptoms in foliage of Central European oaks. Trees 30, 883–900. doi: 10.1007/s00468-015-1329-6
Volpe, V., Manzoni, S., Marani, M., and Katul, G. (2011). Leaf conductance and carbon gain under salt-stressed conditions. J. Geophys. Res. 116:G04035. doi: 10.1029/2011JG001848
Westoby, M., Falster, D. S., Moles, A. T., Vesk, P. A., and Wright, I. J. (2002). Plant ecological strategies: some leading dimensions of variation between species. Annu. Rev. Ecol. Syst. 33, 125–159. doi: 10.1146/annurev.ecolsys.33.010802.150452
Wheeler, R. E. (2010). “multResp() lmPerm”. The R project for statistical computing. Available online at: http://www.r-project.org/ (accessed August 02, 2016).
White, T. C. (1974). A hypothesis to explain outbreaks of looper caterpillars, with special reference to populations of Selidosema suavis in a plantation of Pinus radiata in New Zealand. Oecologia 16, 279–301. doi: 10.1007/BF00344738
White, T. C. R. (1984). The abundance of invertebrate herbivores in relation to the availability of nitrogen in stressed food plants. Oecologia 63, 90–105. doi: 10.1007/BF00379790
Keywords: arthropod biodiversity, deicing salt, Eucallipterus tiliae, multidisciplinary functional approach, plant tolerance, urban ecology, urban soils
Citation: Bouraoui D, Cekstere G, Osvalde A, Vollenweider P and Rasmann S (2019) Deicing Salt Pollution Affects the Foliar Traits and Arthropods' Biodiversity of Lime Trees in Riga's Street Greeneries. Front. Ecol. Evol. 7:282. doi: 10.3389/fevo.2019.00282
Received: 28 February 2019; Accepted: 11 July 2019;
Published: 31 July 2019.
Edited by:
Shannon Murphy, University of Denver, United StatesReviewed by:
Elsa Youngsteadt, North Carolina State University, United StatesSteve Frank, North Carolina State University, United States
Copyright © 2019 Bouraoui, Cekstere, Osvalde, Vollenweider and Rasmann. This is an open-access article distributed under the terms of the Creative Commons Attribution License (CC BY). The use, distribution or reproduction in other forums is permitted, provided the original author(s) and the copyright owner(s) are credited and that the original publication in this journal is cited, in accordance with accepted academic practice. No use, distribution or reproduction is permitted which does not comply with these terms.
*Correspondence: Sergio Rasmann, c2VyZ2lvLnJhc21hbm5AdW5pbmUuY2g=