- 1Department of Entomology, Texas A&M AgriLife Research, Amarillo, TX, United States
- 2Division of Plant Sciences, University of Missouri, Columbia, MO, United States
Plants regularly encounter stress, and their responses to abiotic and biotic stressors have been a focus of research for decades. Stress caused by drought is one of the most often studied abiotic stresses owing to the increase in the incidence of drought driven by climate change. Severe drought has been shown to elicit a whole-plant response guided by key phytohormones, which not only respond to water stress but also play a critical role in the response of plants to biotic stress imposed by herbivores and pathogens. This is especially relevant for insect-transmitted pathogen systems, where plants, herbivores, and pathogens are linked in a web of direct and indirect interactions. Few studies have thus far explored the complex nature of drought-mediated tripartite interactions, however, and our ability to generalize and predict how plants respond to herbivore-transmitted pathogens while simultaneously countering the consequences of drought remains limited. The goal of this mini-review is to assess the current state of the field regarding the molecular mechanisms underlying plant responses to the combined effects of drought and simultaneous herbivory and pathogen transmission and their ecological consequences. We discuss plant responses to drought, herbivory, and pathogens as distinct and concurrent stresses, and highlight the implications of the tripartite interactions on insect vector and pathogen suppression in agroecosystems. This review provides a framework for future research linking generalized molecular responses in drought-stressed plants to tripartite species interactions and the ecology of insect-transmitted pathogens in the context of modern agriculture and water deficit driven by climate change.
Introduction
Climate change is driving an increase in incidence of drought at a global scale and extreme water deficiency is a significant impediment to plant fitness (Boyer, 1982, 2010; Bray et al., 2000). The impact of drought stress on plants extends beyond primary plant productivity, however, and results in profound changes in phytohormones and secondary metabolites involved in plant defenses (Asselbergh et al., 2008), the consequences of which for plant resistance to insects and pathogens are still poorly understood.
Plants commonly contend with multiple abiotic and biotic stresses simultaneously and must be capable of responding in a coordinated and dynamic fashion. This is particularly true for vector-borne plant pathogen systems where reciprocal direct and indirect feedback among plants, vectors, and pathogens results in changes in performance and behavior of the vectors and pathogens (Figure 1). Yet studies rarely measure the responses of plants to multiple stresses and even fewer studies have quantified the molecular mechanisms and ecological outcomes of tripartite plant-vector-pathogen interactions in the context of drought. However, the potential for non-additive effects of multiple stresses has contributed to a growing consensus that simplified investigations of pairwise interactions may not be sufficient (Mittler and Blumwald, 2010; Atkinson and Urwin, 2012; Atkinson et al., 2013; Prasch and Sonnewald, 2013; Bergès et al., 2018). Thus, the goal of this review is to outline the current state of the field and synthesize research on responses of drought-stressed plants to pathogen and herbivore attack. While reviews of the molecular mechanisms and consequences of single and multiple stresses have been assembled previously (e.g., Atkinson et al., 2015), this is the first synthesis of research focused specifically on the drought-mediated tripartite interactions among plants, insect vectors, and vector-borne pathogens. We highlight the general responses of plants to drought and biotic stress in order to contextualize the concepts of multi-stress responses. We examine the specific consequences of water deficit for plant interactions with pathogens and herbivores individually, and synthesize research on drought-mediated tripartite interactions. We also highlight the consequences of these responses for species interactions and for insect vector and pathogen suppression in agroecosystems. This mini-review aims to create a framework for linking key molecular mechanisms in plants under simultaneous drought and pathogen/vector attack to specific and predictable interactions among species.
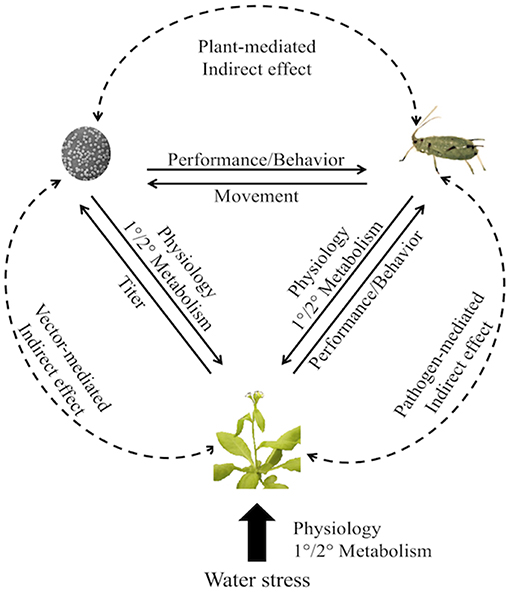
Figure 1. Direct and indirect interactions among insect vectors, pathogens, and drought-stressed host plants, with examples of responding traits. Solid lines are direct effects, dashed lines are indirect effects. Arrows indicate the effect of one factor on another. Water deficit affects plant primary (differentiation) and secondary (defense) metabolism. These changes can alter reciprocal direct and indirect feedback among the plants, vectors, and pathogens and result in changes in performance and behavior of the vectors and pathogens.
Overview of General Molecular Responses of Plants to Biotic Stress and Drought
Plants utilize a diverse array of responses to attack by herbivores and pathogens. Metabolic responses are regulated by interconnected signaling pathways in which salicylic acid (SA) and jasmonic acid (JA) play a key role (Stout et al., 2006). The SA-mediated response pathway was originally implicated in plant resistance to pathogens (Kunkel and Brooks, 2002; Shah, 2003) and the JA-mediated pathway was thought to confer resistance to insects (Howe et al., 1996). We now know, however, that these pathways are intricate, interconnected (Walling, 2000; Koornneef and Pieterse, 2008; Thaler et al., 2010), and complicated by reciprocal down-regulation (i.e., “crosstalk”) (Thaler et al., 2002) that requires plants to integrate and prioritize their defenses (Bostock, 2005). Consequently, plant-mediated indirect interactions between pathogens and herbivores can lead to either induced resistance or induced susceptibility as a result of a multiple-enemy attack (Bostock, 2005).
The JA- and SA-mediated pathways overlap with the pathways that regulate plant responses to drought regulated by the hormone abscisic acid (ABA) (Ramanjulu and Bartels, 2002; Bartels and Sunkar, 2005; Urano et al., 2009; Harb et al., 2010). ABA modulates plants responses to drought stress, and its accumulation results in increases of reactive oxygen species, changes in cell turgor, and stomatal closure (Lee and Luan, 2012). ABA also prioritizes plant responses to biotic stress (Asselbergh et al., 2008), and interactions among ABA, JA, and SA have been extensively studied (Lee and Luan, 2012; Kazan, 2015; Nguyen et al., 2016).
Abscisic acid can dramatically re-shape interactions between JA and SA (Yasuda et al., 2008; Fan et al., 2009; Kazan, 2015; Muñoz-Espinoza et al., 2015; Guo et al., 2016; Wei et al., 2018), which illustrates that plant responses to biotic stress are frequently altered under drought. In general, accumulations of ABA are accompanied by a decrease in SA (Yasuda et al., 2008; Fan et al., 2009; Muñoz-Espinoza et al., 2015; Guo et al., 2016; Liu et al., 2016; Nachappa et al., 2016). However, an increase in ABA can also result in concomitant rise in SA (Audenaert et al., 2002; Anderson et al., 2004; Mauch-Mani and Mauch, 2005). Interactions between ABA and JA are equally variable, and ABA can have synergistic and antagonistic interactions with JA (Asselbergh et al., 2008). For example, changes in proteins involved in JA synthesis and accumulation were noted in a diversity of plants exposed to water deficit (Fan et al., 2009; Harb et al., 2010; Bonhomme et al., 2012; Ahmad et al., 2016; Haider et al., 2017), while other studies have indicated lack of JA induction in drought-stressed plants (Muñoz-Espinoza et al., 2015; Nachappa et al., 2016). The exact nature of interactions among the defensive responses of plants to biotic and abiotic stresses remains understudied, however, despite their importance to plant-pathogen, plant-insect, and plant-pathogen-vector interactions (Asselbergh et al., 2008; Erb et al., 2011; Davis et al., 2015b; Nachappa et al., 2016).
Pathogen-Plant Interactions in Water Deficit
In general, there is an inverse relationship between drought and resistance to pathogens driven by the negative cross talk between ABA and SA. For example, an ABA-mediated decrease in SA enhanced the susceptibility of Arabidopsis and tomato to Pseudomonas syringae (Mohr and Cahill, 2007) and tomato to Botrytis cinereal (Audenaert et al., 2002). Further, applications of exogenous ABA suppressed systemic acquired resistance (SAR) to pathogens, while induction of SAR decreased expression of ABA-biosynthesis genes, suggesting that the antagonistic interaction between these pathways is reciprocal (Yasuda et al., 2008). It is noteworthy that the crosstalk between ABA and SA can be accompanied by a synergism between ABA and JA in some (Fan et al., 2009), but not all cases (Anderson et al., 2004; Yasuda et al., 2008), emphasizing the complex and intricate links among these phytohormones.
There are instances, however, where ABA or drought stress in general promote enhanced plant resistance to pathogens. Stomatal closure induced by ABA to reduce transpiration can prevent pathogen entry (Melotto et al., 2006), and ABA signaling has been implicated in defense against necrotrophic pathogens, which induce JA-mediated defenses. This outcome suggests that instances of ABA-JA synergism can have positive outcomes for plant resistance and can vary across and within plant species (Asselbergh et al., 2008; Ramegowda and Senthil-Kumar, 2015).
Insect Vector-Plant Interactions in Water Deficit
Sap-feeding herbivores including insect vectors are predicted to respond positively to drought-stressed plants due to enhanced nutritional quality (Joern and Mole, 2005; Yan et al., 2015; Nachappa et al., 2016; Sconiers and Eubanks, 2017; Florencio-Ortiz et al., 2018). Specifically, physiological changes stemming from ABA-regulated osmotic adjustments that enable plants to maintain cell turgor by accumulating solutes (Ingram and Bartels, 1996; Harb et al., 2010) can benefit herbivores through increases in nitrogen and carbohydrate availability (Huberty and Denno, 2004). However, empirical support for this prediction is mixed.
Drought can have particularly complex and inconsistent effects on aphids, common vectors of phytopathogens. Aphids can respond positively (Tariq et al., 2012), negatively (Pons and Tatchell, 1995; Mcvean and Dixon, 2001; Hale et al., 2003; Simpson et al., 2012; Guo et al., 2016; Nachappa et al., 2016), or neutrally (Larsson and Björkman, 1993; Nachappa et al., 2016; Sconiers and Eubanks, 2017) to drought-stressed plants. The variable effects of drought on aphid fitness may be driven by the intensity of water deficit, whereby performance of a single aphid species increases under moderate stress but decreases under severe drought stress (Banfield-Zanin and Leather, 2015). Furthermore, aphids are highly susceptible to changes in osmotic pressure, which can constrain their ability to exploit elevated nutrients in drought-stressed plants, as was demonstrated in the lack of concomitant increase in performance of soybean aphids (Aphis glycines) exposed to soybean (Glycine max) under drought stress (Nachappa et al., 2016). Increased concentrations of JA-regulated glucosinolates have also been implicated in the lack of an enhanced aphid performance despite higher levels of nitrogen in stressed plants (Tariq et al., 2012).
Thrips and whiteflies are two other major insect vectors of phytopathogens, but few studies have explored the impact of drought on their fitness as vectors. Whiteflies, for example, were found to favor plants under low rates of irrigation (Paris et al., 1993; Skinner, 1996), while their oviposition was decreased by water deficit (Inbar et al., 2001). Similarly, thrips were more abundant on plants under water deficit and benefited from intermittent drought stress in cotton (Sconiers and Eubanks, 2017). Further, intense and severe drought was linked to outbreaks of Thrips tabaci in onion, which resulted in significant losses to crop productivity (Fournier et al., 1995).
The consequences of drought for vector fitness may also depend on its infection status. For instance, water deficit in tomato increased survival of Bactericera cockerelli (Hemiptera: Triozidae) infected with a bacterium Candidatus Liberibacter solanacearum, and resulted in a 60% increase in the number of adults (Huot and Tamborindeguy, 2017). Further, populations of infectious Rhopalosiphum padi aphids grew faster on wheat infected with Barley yellow dwarf virus compared to virus-free plants, and the effect was stronger when plants were under drought stress compared to unstressed plants (Davis et al., 2015a). This study did not explore how non-infected aphids responded to drought, which may further alter the outcome of plant-insect vector interactions (Hale et al., 2003). There is evidence that pathogens can lower the fitness of their insect vectors (Donaldson and Gratton, 2007; Nachappa et al., 2011; de Oliveira et al., 2014), and pathogen-mediated vector traits can interact with drought-mediated changes in plants. This was illustrated in the case of infectious soybean aphids, which unlike their virus-free counterparts, were unaffected by water status of the plants (Castle and Berger, 1993). Overall, water deficit exposure appears to have no effect or improve performance of non-infectious aphids, but further research is necessary to validate this effect.
Plant-Mediated Effects of Phytopathogens on Their Vectors
Increasing evidence documents that phytopathogens manipulate the performance and behavior of their insect vectors by altering the chemical or physical properties of their shared host plant (Casteel and Falk, 2016; Eigenbrode et al., 2018; Mauck et al., 2018). These phytopathogen-induced vector and host plant phenotypes are typically thought to be conducive to pathogen dissemination in the environment (Mauck et al., 2018). For example, pathogen-induced injury and altered volatile emissions can increase the attractiveness of infected plants to vectors (Eigenbrode et al., 2002; Jiménez-Martínez et al., 2004; Belliure et al., 2005; Bosque-Pérez and Eigenbrode, 2011; Hodge et al., 2011; Shapiro et al., 2012; Ogada et al., 2013). However, the exact nature of the interaction likely depends on the mechanism of pathogen transmission (Castle and Berger, 1993; Mauck et al., 2012; Eigenbrode et al., 2018). Pathogens that require long, uninterrupted feeding periods for efficient transmission may benefit by improving host plant quality and enhancing vector settling behavior (Belliure et al., 2005; Ogada et al., 2013). Alternatively, pathogens that require only a brief feeding probe for transmission may promote their own proliferation by inducing deceptive visual or chemical cues to attract vectors to unpalatable or low-quality plants from which vectors quickly emigrate after an initial feeding attempt (Purcell and Almeida, 2005; Mauck et al., 2010). While it is likely that drought will alter the plant-mediated effects of pathogens on their vectors, we currently lack the necessary understanding of the mechanistic basis of plant virus manipulation to predict these complicated multi-stress outcomes (Mauck et al., 2019).
Insect Transmission of Pathogens Under Drought Stress
The efficiency of pathogen transmission by insects is likely to be influenced by drought stress. Water deficit can directly affect plant resistance to pathogens and vectors via the overlap and crosstalk in the pathways regulated by the key phytohormones. However, water deficit can also affect plant infection risk indirectly by altering vector behaviors or preferences that are critical to transmission and are otherwise manipulated by vector-borne pathogens to their benefit in unstressed plants (reviewed in Mauck et al., 2016). For example, a loss in plant cell turgor pressure may alter feeding behaviors, such as the number of times a vector probes a plant (Krugner and Backus, 2014; Nachappa et al., 2016). Furthermore, low turgor pressure may enhance the plant-to-plant movement of vectors as they search for acceptable hosts, thereby increasing the number of plants contacted by individual vectors. These recent studies support the idea that drought may transiently and indirectly increase plant susceptibility to insect-transmitted pathogens, particularly if water deficit is severe and stimulates vector movement and probing frequency.
In fact, there is accumulating evidence to support the indirect effects of drought-stressed host plants on pathogen transmission. Drought stress increased aphid transmission of Cauliflower mosaic virus (CMV) and Turnip mosaic virus to Brassica rapa (van Munster et al., 2017). However, water deficit had the opposite effect on the aphid-transmitted Turnip yellow virus (TuYV) in Arabidopsis, whereby transmission of TuYV was significantly reduced due to lower viral accumulation in aphids feeding on drought-stressed plants (Yvon et al., 2017). Nachappa et al. (2016) found that incidence and transmission of the aphid-transmitted Soybean mosaic virus were also lower in soybean exposed to drought compared to well-watered plants, likely owing to a decrease in time required for the aphids to access phloem in the stressed plants. Further, drought affected aphid transmission of CMV across Arabidopsis accessions with varying fitness responses to water deficit, and the authors concluded that the consequences of drought for transmission and virulence were driven by the growth traits of the host plants (Bergès et al., 2018).
Thus, it appears that the effect of drought on vector feeding behavior and other fitness traits plays a key, albeit indirect, role in shaping the consequences of drought on transmission of vector-borne pathogens. It is also apparent that the interactions among the intensity and duration of drought, plant species, and vector and pathogen natural history traits are likely to be even more complex under field conditions.
Drought-Mediated Tripartite Interactions
Plants susceptible to insect-transmitted pathogens must contend simultaneously with the consequences of drought, herbivory, and pathogen infection and coordinate their defensive responses accordingly. Likewise, herbivores and pathogens employ strategies to overcome plant defenses and promote their own proliferation. The result is a complex web of direct and indirect effects among pathogens, vectors, and their shared host plants (Figure 1).
Predicting the outcomes of these complex interactions is a challenge as they are likely to be species-specific and driven by natural history traits and genetic diversity of each of the organisms. We speculate that changes in vector performance and behavior mediated by drought-induced modifications in plant primary and secondary metabolism are likely to increase plant disease risk. The overlap and crosstalk in the molecular responses of plants to biotic and abiotic stresses, however, will ultimately determine the outcome of tripartite interactions. Drought is likely to influence the overall number of vectors present in the environment and the likelihood that these vectors will encounter and feed upon host plants, which is the essential first-step in vector-borne pathogen transmission. However, the probability that an encounter between a vector and a host plant will result in infection is likely governed by the ability of the plant to mount a successful immune response while simultaneously countering the effects of herbivory and water limitation. Multiple comprehensive studies that integrate molecular, behavioral, and ecological studies within a single plant system are necessary in order to advance our understanding of the mechanisms and consequences of drought-mediated tripartite interactions.
Implications of Drought Stress for Suppression of Insect Vectors and Vector-Borne Pathogens
The tripartite interactions among plants, insect vectors, and pathogens take place within diverse communities, and the complex outcomes of these interactions can have broad implications for the management of agricultural systems. First, the ability to predict how deficit irrigation affects plant productivity, insect vectors, and the associated vector-borne pathogens will allow for effective exploitation of water as a means to suppress vectors and pathogens (Daane and Williams, 2003; Rousselin et al., 2017). Secondly, understanding the impact of drought stress on direct and indirect plant defenses will allow for predictions of the likelihood of pest outbreaks and proper remediation plans that incorporate irrigation as one of the pest management tactics. For example, drought may impair the competitive advantage of native herbivores during invasions by non-native herbivores, as was illustrated in wheat, where water deficit reduced the ability of the native aphid R. padi to inhibit the population growth of the invasive aphid, Metopolophium festucae Theobald subsp. cerealum (Foote et al., 2017). Incorporating irrigation to alter these interactions could lower the incidence and severity of outbreaks of non-native vectors and associated pathogens. Further, recruitment of natural enemies and their meaningful contribution to pest suppression can be affected by drought through altered attraction of predators to injured plants. The nearly universal drought-induced decrease in SA, which is important in indirect plant defenses, is likely to affect recruitment of predators through disrupted emission of volatiles (Martini and Stelinski, 2017).
Conclusions
Given the increases in incidence of drought across the globe, research exploring the mechanisms driving drought-mediated tripartite interactions on multiple levels of organization and across trophic levels is more critical than ever. Research should employ a species-specific approach to quantifying plant responses to multiple stresses and assessing their consequences from gene expression to the fitness and behavior of vectors, pathogens associated with them, and their natural enemies.
Author Contributions
AS and DF contributed equally to the conceptualization and composition of the manuscript.
Funding
This work was supported by the USDA NIFA TEX09638 to AS and USDA NIFA MO-HAPS0006 to DF.
Conflict of Interest Statement
The authors declare that the research was conducted in the absence of any commercial or financial relationships that could be construed as a potential conflict of interest.
Acknowledgments
The authors thank Punya Nachappa and Priya Voothuluru for discussion that inspired an earlier draft of this review.
References
Ahmad, P., Rasool, S., Gul, A., Sheikh, S. A., Akram, N. A., Ashraf, M., et al. (2016). Jasmonates: Multifunctional roles in stress tolerance. Front. Plant Sci. 7:813. doi: 10.3389/fpls.2016.00813
Anderson, J. P., Badruzsaufari, E., Schenk, P. M., Manners, J. M., Desmond, O. J., Ehlert, C., et al. (2004). Antagonistic interaction between abscisic acid and jasmonate-ethylene signaling pathways modulates defense gene expression and disease resistance in Arabidopsis. Plant Cell 16, 3460–3479. doi: 10.1105/tpc.104.025833
Asselbergh, B., De Vleesschauwer, D., and Höfte, M. (2008). Global switches and fine-tuning—ABA modulates plant pathogen defense. Mol. Plant Microbe Interact. 21, 709–719. doi: 10.1094/MPMI-21-6-0709
Atkinson, N. J., Jain, R., and Urwin, P. E. (2015). “The Response of “Plants to simultaneous biotic and abiotic stress,” in Combined Stresses in Plants: Physiological, Molecular, and Biochemical Aspects, ed R. Mahalingam (Cham: Springer International Publishing), 181–201. doi: 10.1007/978-3-319-07899-1_9
Atkinson, N. J., Lilley, C. J., and Urwin, P. E. (2013). Identification of genes involved in the response of Arabidopsis to simultaneous biotic and abiotic stresses. Plant Physiol. 162, 2028–2041. doi: 10.1104/pp.113.222372
Atkinson, N. J., and Urwin, P. E. (2012). The interaction of plant biotic and abiotic stresses: from genes to the field. J. Exp. Bot. 63, 3523–3543. doi: 10.1093/jxb/ers100
Audenaert, K., Meyer, G. B. D., and Höfte, M. M. (2002). Abscisic acid determines basal susceptibility of tomato to Botrytis cinerea and suppresses salicylic acid-dependent signaling mechanisms. Plant Physiol. 128, 491–501. doi: 10.1104/pp.010605
Banfield-Zanin, J. A., and Leather, S. R. (2015). Reproduction of an arboreal aphid pest, Elatobium abietinum, is altered under drought stress. J. Appl. Entomol. 139, 302–313. doi: 10.1111/jen.12159
Bartels, D., and Sunkar, R. (2005). Drought and salt tolerance in plants. Crit. Rev. Plant Sci. 24, 23–58. doi: 10.1080/07352680590910410
Belliure, B., Janssen, A., Maris, P. C., Peters, D., and Sabelis, M. W. (2005). Herbivore arthropods benefit from vectoring plant viruses. Ecol. Lett. 8, 70–79. doi: 10.1111/j.1461-0248.2004.00699.x
Bergès, S. E., Vile, D., Vazquez-Rovere, C., Blanc, S., Yvon, M., Bédiée, A., et al. (2018). Interactions between drought and plant genotype change epidemiological traits of Cauliflower mosaic virus. Front. Plant Sci. 9:703. doi: 10.3389/fpls.2018.00703
Bonhomme, L., Valot, B., Tardieu, F., and Zivy, M. (2012). Phosphoproteome dynamics upon changes in plant water status reveal early events associated with rapid growth adjustment in maize leaves. Mol. Cell. Proteomics 11, 957–972. doi: 10.1074/mcp.M111.015867
Bosque-Pérez, N. A., and Eigenbrode, S. D. (2011). The influence of virus-induced changes in plants on aphid vectors: insights from luteovirus pathosystems. Virus Res. 159, 201–205. doi: 10.1016/j.virusres.2011.04.020
Bostock, R. M. (2005). Signal crosstalk and induced resistance: straddling the line between cost and benefit. Annu. Rev. Phytopathol. 43, 545–580. doi: 10.1146/annurev.phyto.41.052002.095505
Boyer, J. S. (1982). Plant productivity and environment. Science 218, 443–448. doi: 10.1126/science.218.4571.443
Bray, E. A., Bailey-Serred, J., and Weretilnyk, E. (2000). “Responses to abiotic stresses,” in Biochemistry and Molecular Biology of Plants (Rockville, MD: American Society of Plant Physiologists), 1158–1203.
Casteel, C. L., and Falk, B. W. (2016). “Plant virus-vector interactions: more than just for virus transmission,” in Current Research Topics in Plant Virology, eds A. Wang and X. Zhou (Cham: Springer International Publishing), 217–240. doi: 10.1007/978-3-319-32919-2_9
Castle, S. J., and Berger, P. H. (1993). Rates of growth and increase of Myzus persicae on virus-infected potatoes according to type of virus-vector relationship. Entomol. Exp. Applic. 69, 51–60. doi: 10.1111/j.1570-7458.1993.tb01727.x
Daane, K. M., and Williams, L. E. (2003). Manipulating vineyard irrigation amounts to reduce insect pest damage. Ecol. Applic. 13, 1650–1666. doi: 10.1890/02-5328
Davis, T. S., Bosque-Pérez, N. A., Foote, N. E., Magney, T., and Eigenbrode, S. D. (2015a). Environmentally dependent host–pathogen and vector–pathogen interactions in the Barley yellow dwarf virus pathosystem. J. Appl. Ecol. 52, 1392–1401. doi: 10.1111/1365-2664.12484
Davis, T. S., Bosque-Pérez, N. A., Popova, I., and Eigenbrode, S. D. (2015b). Evidence for additive effects of virus infection and water availability on phytohormone induction in a staple crop. Front. Ecol. Evol. 3:114. doi: 10.3389/fevo.2015.00114
de Oliveira, C. F., Long, E. Y., and Finke, D. L. (2014). A negative effect of a pathogen on its vector? A plant pathogen increases the vulnerability of its vector to attack by natural enemies. Oecologia 174, 1169–1177. doi: 10.1007/s00442-013-2854-x
Donaldson, J. R., and Gratton, C. (2007). Antagonistic effects of soybean viruses on soybean aphid performance. Environ. Entomol. 36, 918–925. doi: 10.1093/ee/36.4.918
Eigenbrode, S. D., Bosque-Pérez, N. A., and Davis, T. S. (2018). Insect-borne plant pathogens and their vectors: ecology, evolution, and complex interactions. Annu. Rev. Entomol. 63, 169–191. doi: 10.1146/annurev-ento-020117-043119
Eigenbrode, S. D., Ding, H., Shiel, P., and Berger, P. H. (2002). Volatiles from potato plants infected with potato leafroll virus attract and arrest the virus vector, Myzus persicae (Homoptera: Aphididae). Proc. R. Soc. Lond. Ser. B Biol. Sci. 269, 455–460. doi: 10.1098/rspb.2001.1909
Erb, M., Balmer, D., Lange, E. S. D., Merey, G. V., Planchamp, C., Robert, C., et al. (2011). Synergies and trade-offs between insect and pathogen resistance in maize leaves and roots. Plant Cell Environ. 34, 1088–1103. doi: 10.1111/j.1365-3040.2011.02307.x
Fan, J., Hill, L., Crooks, C., Doerner, P., and Lamb, C. (2009). Abscisic acid has a key role in modulating diverse plant-pathogen interactions. Plant Physiol. 150, 1750–1761. doi: 10.1104/pp.109.137943
Florencio-Ortiz, V., Sellés-Marchart, S., Zubcoff-Vallejo, J., Jander, G., and Casas, J. L. (2018). Changes in the free amino acid composition of Capsicum annuum (pepper) leaves in response to Myzus persicae (green peach aphid) infestation. A comparison with water stress. PLoS ONE 13:e0198093. doi: 10.1371/journal.pone.0198093
Foote, N. E., Davis, T. S., Crowder, D. W., Bosque-Pérez, N. A., and Eigenbrode, S. D. (2017). Plant water stress affects interactions between an invasive and a naturalized aphid species on cereal crops. Environ. Entomol. 46, 609–616. doi: 10.1093/ee/nvx071
Fournier, F., Boivin, G., and Stewart, R. K. (1995). Effect of Thrips tabaci (Thysanoptera: Thripidae) on yellow onion yields and economic thresholds for its management. J. Econ. Entomol. 88, 1401–1407. doi: 10.1093/jee/88.5.1401
Guo, H., Sun, Y., Peng, X., Wang, Q., Harris, M., and Ge, F. (2016). Up-regulation of abscisic acid signaling pathway facilitates aphid xylem absorption and osmoregulation under drought stress. J. Exp. Bot. 67, 681–693. doi: 10.1093/jxb/erv481
Haider, M. S., Kurjogi, M. M., Khalil-Ur-Rehman, M., Fiaz, M., Pervaiz, T., Jiu, S., et al. (2017). Grapevine immune signaling network in response to drought stress as revealed by transcriptomic analysis. Plant Physiol. Biochem. 121, 187–195. doi: 10.1016/j.plaphy.2017.10.026
Hale, B. K., Bale, J. S., Pritchard, J., Masters, G. J., and Brown, V. K. (2003). Effects of host plant drought stress on the performance of the bird cherry-oat aphid, Rhopalosiphum padi (L.): a mechanistic analysis. Ecol. Entomol. 28, 666–677. doi: 10.1111/j.1365-2311.2003.00563.x
Harb, A., Krishnan, A., Ambavaram, M. M. R., and Pereira, A. (2010). Molecular and physiological analysis of drought stress in Arabidopsis reveals early responses leading to acclimation in plant growth. Plant Physiol. 154, 1254–1271. doi: 10.1104/pp.110.161752
Hodge, S., Hardie, J., and Powell, G. (2011). Parasitoids aid dispersal of a nonpersistently transmitted plant virus by disturbing the aphid vector. Agric. For. Entomol. 13, 83–88. doi: 10.1111/j.1461-9563.2010.00493.x
Howe, G. A., Lightner, J., and Ryan, C. A. (1996). An octadecanoid pathway mutant (JL5) of tomato is compromised in signaling for defense against insect attack. Plant Cell 8, 2067–2077. doi: 10.1105/tpc.8.11.2067
Huberty, A. F., and Denno, R. F. (2004). Plant water stress and its consequences for herbivorous insects: a new synthesis. Ecology 85, 1383–1398. doi: 10.1890/03-0352
Huot, O. B., and Tamborindeguy, C. (2017). Drought stress affects Solanum lycopersicum susceptibility to Bactericera cockerelli colonization. Entomol. Exp. Appl. 165, 70–82. doi: 10.1111/eea.12627
Inbar, M., Doostdar, H., and Mayer, R. T. (2001). Suitability of stressed and vigorous plants to various insect herbivores. Oikos 94, 228–235. doi: 10.1034/j.1600-0706.2001.940203.x
Ingram, J., and Bartels, D. (1996). The molecular basis of dehydration tolerance in plants. Annu. Rev. Plant Biol. 47, 377–403. doi: 10.1146/annurev.arplant.47.1.377
Jiménez-Martínez, E. S., Bosque-Pérez, N. A., Berger, P. H., Zemetra, R. S., Ding, H., and Eigenbrode, S. D. (2004). Volatile cues influence the response of Rhopalosiphum padi (Homoptera: Aphididae) to Barley yellow dwarf virus–infected transgenic and untransformed wheat. Environ. Entomol. 33, 1207–1216. doi: 10.1603/0046-225X-33.5.1207
Joern, A., and Mole, S. (2005). The plant stress hypothesis and variable responses by blue grama grass (Bouteloua gracilis) to water, mineral nitrogen, and insect herbivory. J. Chem. Ecol. 31, 2069–2090. doi: 10.1007/s10886-005-6078-3
Kazan, K. (2015). Diverse roles of jasmonates and ethylene in abiotic stress tolerance. Trends Plant Sci. 20, 219–229. doi: 10.1016/j.tplants.2015.02.001
Koornneef, A., and Pieterse, C. M. (2008). Cross talk in defense signaling. Plant Physiol. 146, 839–844. doi: 10.1104/pp.107.112029
Krugner, R., and Backus, E. A. (2014). Plant water stress effects on stylet probing behaviors of Homalodisca vitripennis (Hemiptera: Cicadellidae) associated with acquisition and inoculation of the bacterium Xylella fastidiosa. J. Econ. Entomol. 107, 66–74. doi: 10.1603/EC13219
Kunkel, B. N., and Brooks, D. M. (2002). Cross talk between signaling pathways in pathogen defense. Curr. Opin. Plant Biol. 5, 325–331. doi: 10.1016/S1369-5266(02)00275-3
Larsson, S., and Björkman, C. (1993). Performance of chewing and phloem-feeding insects on stressed trees. Scand. J. Forest Res. 8, 550–559. doi: 10.1080/02827589309382801
Lee, S. C., and Luan, S. (2012). ABA signal transduction at the crossroad of biotic and abiotic stress responses. Plant Cell Environ. 35, 53–60. doi: 10.1111/j.1365-3040.2011.02426.x
Liu, X., Song, Y., Xing, F., Wang, N., Wen, F., and Zhu, C. (2016). GhWRKY25, a group I WRKY gene from cotton, confers differential tolerance to abiotic and biotic stresses in transgenic Nicotiana benthamiana. Protoplasma 253, 1265–1281. doi: 10.1007/s00709-015-0885-3
Martini, X., and Stelinski, L. L. (2017). Drought stress affects response of phytopathogen vectors and their parasitoids to infection- and damage-induced plant volatile cues. Ecol. Entomol. 42, 721–730. doi: 10.1111/een.12439
Mauch-Mani, B., and Mauch, F. (2005). The role of abscisic acid in plant–pathogen interactions. Curr. Opin. Plant Biol. 8, 409–414. doi: 10.1016/j.pbi.2005.05.015
Mauck, K., Bosque-Pérez, N. A., Eigenbrode, S. D., De Moraes, C. M., and Mescher, M. C. (2012). Transmission mechanisms shape pathogen effects on host–vector interactions: evidence from plant viruses. Funct. Ecol. 26, 1162–1175. doi: 10.1111/j.1365-2435.2012.02026.x
Mauck, K. E., Chesnais, Q., and Shapiro, L. R. (2018). “Evolutionary determinants of host and vector manipulation by plant viruses,” in Advances in Virus Research Environmental Virology and Virus Ecology, ed C. M. Malmstrom (Cambridge, MA: Academic Press), 189–250. doi: 10.1016/bs.aivir.2018.02.007
Mauck, K. E., De Moraes, C. M., and Mescher, M. C. (2016). Effects of pathogens on sensory-mediated interactions between plants and insect vectors. Curr. Opin. Plant Biol. 32, 53–61. doi: 10.1016/j.pbi.2016.06.012
Mauck, K. E., Kenney, J., and Chesnais, Q. (2019). Progress and challenges in identifying molecular mechanisms underlying host and vector manipulation by plant viruses. Curr. Opin. Insect Sci. 33, 7–18. doi: 10.1016/j.cois.2019.01.001
Mauck, K. E., Moraes, C. M. D., and Mescher, M. C. (2010). Deceptive chemical signals induced by a plant virus attract insect vectors to inferior hosts. Proc. Natl. Acad. Sci. U.S.A. 107, 3600–3605. doi: 10.1073/pnas.0907191107
Mcvean, R. I. K., and Dixon, A. F. G. (2001). The effect of plant drought-stress on populations of the pea aphid Acyrthosiphon pisum. Ecol. Entomol. 26, 440–443. doi: 10.1046/j.1365-2311.2001.00341.x
Melotto, M., Underwood, W., Koczan, J., Nomura, K., and He, S. Y. (2006). Plant stomata function in innate immunity against bacterial invasion. Cell 126, 969–980. doi: 10.1016/j.cell.2006.06.054
Mittler, R., and Blumwald, E. (2010). Genetic engineering for modern agriculture: challenges and perspectives. Annu. Rev. Plant Biol. 61, 443–462. doi: 10.1146/annurev-arplant-042809-112116
Mohr, P. G., and Cahill, D. M. (2007). Suppression by ABA of salicylic acid and lignin accumulation and the expression of multiple genes, in Arabidopsis infected with Pseudomonas syringae pv. tomato. Funct. Integr. Genomics 7, 181–191. doi: 10.1007/s10142-006-0041-4
Muñoz-Espinoza, V. A., López-Climent, M. F., Casaretto, J. A., and Gómez-Cadenas, A. (2015). Water stress responses of tomato mutants impaired in hormone biosynthesis reveal abscisic acid, jasmonic acid and salicylic acid interactions. Front. Plant Sci. 6:997. doi: 10.3389/fpls.2015.00997
Nachappa, P., Culkin, C. T., Saya, P. M., Han, J., and Nalam, V. J. (2016). Water stress modulates soybean aphid performance, feeding behavior, and virus transmission in soybean. Front Plant Sci 7:552. doi: 10.3389/fpls.2016.00552
Nachappa, P., Shapiro, A. A., and Tamborindeguy, C. (2011). Effect of ‘Candidatus Liberibacter solanacearum' on fitness of its insect vector, Bactericera cockerelli (Hemiptera: Triozidae), on tomato. Phytopathology 102, 41–46. doi: 10.1094/PHYTO-03-11-0084
Nguyen, D., Rieu, I., Mariani, C., and van Dam, N. M. (2016). How plants handle multiple stresses: hormonal interactions underlying responses to abiotic stress and insect herbivory. Plant Mol. Biol. 91, 727–740. doi: 10.1007/s11103-016-0481-8
Ogada, P. A., Maiss, E., and Poehling, H.-M. (2013). Influence of tomato spotted wilt virus on performance and behaviour of western flower thrips (Frankliniella occidentalis). J. Appl. Entomol. 137, 488–498. doi: 10.1111/jen.12023
Paris, H., Stoffella, P., and Powell, C. (1993). Sweet-potato whitefly, drought stress, and leaf silvering of squash. Hortscience 28, 157–158. doi: 10.21273/HORTSCI.28.2.157
Pons, X., and Tatchell, G. M. (1995). Drought stress and cereal aphid performance. Ann. Appl. Biol. 126, 19–31. doi: 10.1111/j.1744-7348.1995.tb05000.x
Prasch, C. M., and Sonnewald, U. (2013). Simultaneous application of heat, drought, and virus to Arabidopsis plants reveals significant shifts in signaling networks. Plant Physiol. 162, 1849–1866. doi: 10.1104/pp.113.221044
Purcell, A. H., and Almeida, R. P. P. (2005). “Insects as vectors of disease agents,” in Encyclopedia of Plant and Crop Science, ed M. Dekker (London: Tayler & Francis). doi: 10.1081/E-EPCS-120010496
Ramanjulu, S., and Bartels, D. (2002). Drought- and desiccation-induced modulation of gene expression in plants. Plant Cell Environ. 25, 141–151. doi: 10.1046/j.0016-8025.2001.00764.x
Ramegowda, V., and Senthil-Kumar, M. (2015). The interactive effects of simultaneous biotic and abiotic stresses on plants: mechanistic understanding from drought and pathogen combination. J. Plant Physiol. 176, 47–54. doi: 10.1016/j.jplph.2014.11.008
Rousselin, A., Bevacqua, D., Sauge, M.-H., Lescourret, F., Mody, K., and Jordan, M.-O. (2017). Harnessing the aphid life cycle to reduce insecticide reliance in apple and peach orchards. A review. Agron. Sustain. Dev. 37:38. doi: 10.1007/s13593-017-0444-8
Sconiers, W. B., and Eubanks, M. D. (2017). Not all droughts are created equal? The effects of stress severity on insect herbivore abundance. Arthropod Plant Interact. 11, 45–60. doi: 10.1007/s11829-016-9464-6
Shah, J. (2003). The salicylic acid loop in plant defense. Curr. Opin. Plant Biol. 6, 365–371. doi: 10.1016/S1369-5266(03)00058-X
Shapiro, L., Moraes, C. M. D., Stephenson, A. G., and Mescher, M. C. (2012). Pathogen effects on vegetative and floral odours mediate vector attraction and host exposure in a complex pathosystem. Ecol. Lett. 15, 1430–1438. doi: 10.1111/ele.12001
Simpson, K. L. S., Jackson, G. E., and Grace, J. (2012). The response of aphids to plant water stress – the case of Myzus persicae and Brassica oleracea var. capitata. Entomol. Exp. Applic. 142, 191–202. doi: 10.1111/j.1570-7458.2011.01216.x
Skinner, R. H. (1996). Response of Bemisia argentifolii (Homoptera: Aleyrodidae) to water and nutrient stressed cotton. Environ. Entomol. 25, 401–406. doi: 10.1093/ee/25.2.401
Stout, M. J., Thaler, J. S., and Thomma, B. P. H. J. (2006). Plant-mediated interactions between pathogenic microorganisms and herbivorous arthropods. Annu. Rev. Entomol. 51, 663–689. doi: 10.1146/annurev.ento.51.110104.151117
Tariq, M., Wright, D. J., Rossiter, J. T., and Staley, J. T. (2012). Aphids in a changing world: testing the plant stress, plant vigour and pulsed stress hypotheses. Agric. For. Entomol. 14, 177–185. doi: 10.1111/j.1461-9563.2011.00557.x
Thaler, J. S., Agrawal, A. A., and Halitschke, R. (2010). Salicylate-mediated interactions between pathogens and herbivores. Ecology 91, 1075–1082. doi: 10.1890/08-2347.1
Thaler, J. S., Karban, R., Ullman, D. E., Boege, K., and Bostock, R. M. (2002). Cross-talk between jasmonate and salicylate plant defense pathways: effects on several plant parasites. Oecologia 131, 227–235. doi: 10.1007/s00442-002-0885-9
Urano, K., Maruyama, K., Ogata, Y., Morishita, Y., Takeda, M., Sakurai, N., et al. (2009). Characterization of the ABA-regulated global responses to dehydration in Arabidopsis by metabolomics. Plant J. 57, 1065–1078. doi: 10.1111/j.1365-313X.2008.03748.x
van Munster, M., Yvon, M., Vile, D., Dader, B., Fereres, A., and Blanc, S. (2017). Water deficit enhances the transmission of plant viruses by insect vectors. PLoS ONE 12:e0174398. doi: 10.1371/journal.pone.0174398
Walling, L. L. (2000). The myriad plant responses to herbivores. J. Plant Growth Regul. 19, 195–216. doi: 10.1007/s003440000026
Wei, T., Deng, K., Wang, H., Zhang, L., Wang, C., Song, W., et al. (2018). Comparative transcriptome analyses reveal potential mechanisms of enhanced drought tolerance in transgenic Salvia miltiorrhiza plants expressing AtDREB1A from Arabidopsis. Int. J. Mol. Sci. 19:E827. doi: 10.3390/ijms19030827
Yan, J., Lipka, A. E., Schmelz, E. A., Buckler, E. S., and Jander, G. (2015). Accumulation of 5-hydroxynorvaline in maize (Zea mays) leaves is induced by insect feeding and abiotic stress. J. Exp. Bot. 66, 593–602. doi: 10.1093/jxb/eru385
Yasuda, M., Ishikawa, A., Jikumaru, Y., Seki, M., Umezawa, T., Asami, T., et al. (2008). Antagonistic interaction between systemic acquired resistance and the abscisic acid–mediated abiotic stress response in Arabidopsis. Plant Cell 20, 1678–1692. doi: 10.1105/tpc.107.054296
Keywords: herbivory, pathogen transmission, plant-insect interactions, plant-pathogen interactions, water deficiency, climate change
Citation: Szczepaniec A and Finke D (2019) Plant-Vector-Pathogen Interactions in the Context of Drought Stress. Front. Ecol. Evol. 7:262. doi: 10.3389/fevo.2019.00262
Received: 28 March 2019; Accepted: 24 June 2019;
Published: 09 July 2019.
Edited by:
Lora A. Richards, University of Nevada, Reno, United StatesReviewed by:
Muthappa Senthil-Kumar, National Institute of Plant Genome Research (NIPGR), IndiaKarsten Mody, Darmstadt University of Technology, Germany
Copyright © 2019 Szczepaniec and Finke. This is an open-access article distributed under the terms of the Creative Commons Attribution License (CC BY). The use, distribution or reproduction in other forums is permitted, provided the original author(s) and the copyright owner(s) are credited and that the original publication in this journal is cited, in accordance with accepted academic practice. No use, distribution or reproduction is permitted which does not comply with these terms.
*Correspondence: Adrianna Szczepaniec, YXN6Y3plcGFuaWVjJiN4MDAwNDA7dGFtdS5lZHU=