- Sediment Ecology Research Group, School of Biology, Scottish Oceans Institute, University of St. Andrews, St. Andrews, United Kingdom
Saltmarshes are biogeomorphic ecosystems comprising halophytic plant communities typically located on low energy temperate coasts. Their distribution and structure are controlled by several key drivers, including sediment supply, type of vegetation, elevation, and local hydrodynamics. These dynamic systems are highly vulnerable and estimated to be experiencing annual losses of 1–2% globally. Past restoration efforts have largely implemented managed realignment strategies, however, examples of, and research on, conservation initiatives employing direct transplantation of saltmarsh vegetation into damaged or receding saltmarsh stands is less common. Here an example of transplantation restoration was investigated to understand its influence on sediment dynamics. Sediment settlement, deposition, and accretion rates of natural and restored vegetation (Bolboschoenus maritimus) and adjacent bare mudflats in a small estuary system were studied across consecutive seasons from summer 2015 to spring 2016 to examine the success of transplantation. Natural areas of B. maritimus were shown to be most effective at retaining deposited material, although experiencing the least amount of deposition (an average of 48 g/m2 per day), accreting by nearly 7.5 mm over the experimental period. Mudflat areas experienced the most deposition (an average of 322 g/m2 per day) whilst exhibiting the greatest erosion over the study, a decrease in level of 6 mm. Restored areas experience similar rates of deposition as their natural counterparts, however, did not retain this material as efficiently, presenting an erosion of 1.6 mm. The study indicates certain biogeomorphic processes have been altered within the restored area and beginning to reflect those of the natural area. However, the restored vegetation does not yet fully match the functionality of the natural B. maritimus stand, specifically where the natural stand displayed a net accretion of material the restored area did not. Such discrepancies may impact on the continued survival of the restoration site, which may have implications for the potential of transplanting to deliver ecosystem services, such as climate change mitigation through carbon burial.
Introduction
Saltmarshes are biogeomorphic intertidal ecosystems (Thorne et al., 2014; Schwarz et al., 2015, 2018; Baptist et al., 2016) comprised of halophytic plant communities (Burd, 1989) typically located in the low energy zones of temperate coasts (Allen and Pye, 1992). The current global extent of saltmarsh are estimated to be 54,951 km2 (Mcowen et al., 2017). The location of these coastal systems within intertidal zones produces a wide range of ecosystem services of value to society (Barbier et al., 2011; Beaumont et al., 2014; Costanza et al., 2014) and the natural world. Benefits such as, the provision of biodiversity, coastal protection (Costanza et al., 2008) through wave attenuation (Koch et al., 2009), the reduction of hard engineered defense costs (King and Lester, 1995) and climate change mitigation through sequestration and storage of carbon (Chmura et al., 2003; Donato et al., 2011; McLeod et al., 2011; Fourqurean et al., 2012; Burden et al., 2013).
However, coastal wetlands are, globally, among the most vulnerable and threatened ecosystems (Boorman, 2003; Craft et al., 2009; McLeod et al., 2011). Annually, it is estimated between 0.7 and 7% (McLeod et al., 2011) of intertidal wetlands are lost, of which saltmarshes experience 1 to 2% of loss (Duarte et al., 2008). Losses are driven by various factors, including direct anthropogenic influences, such as coastal development and altered sediment budgets (Boorman, 2003), and changing abiotic factors, such as relative sea-level rise (RSLR) which threatens to drown vegetation (Thorne et al., 2014; Crosby et al., 2016), where increased RSLR threatens to overcome plant tolerances to inundation in the absence of capacity to accrete on an existing marsh platform. However, it is possible accretion could keep pace or exceed RSLR (Reed et al., 1999; Craft et al., 2009; Kirwan et al., 2016) or landward retreat is possible (Schuerch et al., 2018). The loss of these habitats reduces their natural capital assets value and the beneficial services they deliver, as such investment in their conservation should be desired.
The restoration of saltmarshes has historically been achieved through managed realignment. The intentional flooding of previously claimed coastal areas comes with associated conflicts and risks; such as the loss of productive (valuable) land. Various approaches have been employed to facilitate realignment, including managed tidal regimes (Maris et al., 2007; Masselink et al., 2017), channel network design (Zeff, 1999), and sediment subsidization (Schrift et al., 2008). The continued development of restoration strategies to achieve a diverse range of efficient and economical approaches is crucial. The success of all restoration approaches requires a thorough understanding of the biogeomorphic feedbacks, such as between vegetation, sediment and tidal flow, which govern the development and persistence of a marsh area.
Saltmarsh systems are highly dynamic being controlled and influenced by a variety of abiotic (e.g., elevation, tidal flow, sediment supply) and biotic (e.g., vegetation structure, stem density) factors (van Proosdij et al., 2006). The interplay between these factors produce feedbacks which determine the state of the saltmarsh ecosystem at a given point in time—be that a steady-state dynamic equilibrium, or erosional or accreting condition (Baptist et al., 2016); ultimately this balance determine a marshes persistence or not, contributing to their vulnerability. A major factor in this interaction is sediment supply (van Proosdij et al., 2006), namely the erosion-accretion balance. Sediment deposition is governed by the complex interaction between the availability of sediment and the opportunity for this sediment to be deposited (van Proosdij et al., 2006); the interaction of these variables is worthy of further investigation, specifically the effects on sediment deposition resulting from restoration. Coastal vegetation such as mangroves, seagrasses, and saltmarshes encourage deposition to occur (Bos et al., 2007; Chen et al., 2007) by increasing surface roughness and slowing water flow due to presence of stems and leaves (Boorman, 2003; Bouma et al., 2005; Koch et al., 2009; Mudd et al., 2010; Potouroglou et al., 2017), they also decrease sediment resuspension (Boorman et al., 1998). The erosion-deposition balance is an important ecosystem function that determines the elevation of the marsh platform, facilitating successional development. Pioneer vegetation alters the erosion-deposition balance, through increased surface roughness and interception of suspended sediment particles, which encourages the vertical growth of the marsh platform within the tidal frame. The increased diversity of succession (Boorman, 2003) is typically associated with increases in structural complexity and feedbacks (Kirwan and Megonigal, 2013); through increased surface roughness encouraging accretion and lifting of the marsh platform allowing further plant species to colonize.
Restoration can be approached through direct transplanting of a local species (Sullivan, 2001) onto available mudflat or degraded saltmarsh areas, utilizing the “natural” process associated with pioneer establishment and development by altering the erosion-deposition balance. This encourages the spread of new swards and confers protection to existing marshes, relying upon natural lateral and vertical expansion following plantation. Direct transplanting is realistically achievable at a limited scale due to its labor-intensive process. However, transplanting regimes offer the opportunity to (re)vegetate areas of the existing intertidal zone, thus minimizing land-use conflict. The aim of restoration is to produce an ecologically functional area that replicates the natural system and helps to promote the health of an ecosystem under recognized threat. Such restoration through transplantation has taken place since the start of the millennium on the Eden Estuary (east coast of Scotland; Maynard, 2014). Here, Bolboschoenus maritimus plants are harvested from donor stands within the estuary and transplanted onto selected restoration sites (Maynard, 2014).
Here, we compare the potential sedimentation opportunity (quantity of sediment settling out of suspension) with actual sediment deposition and the resulting net elevation change (accretion); capturing tidal and seasonal influence on this relationship. The aim of the study was to assess if successful restoration activities display altered sediment processes then the adjacent un-vegetated mudflat, and further compare how the current processes differ with existing natural saltmarsh extents. It is hypothesized that, (1) restored areas will experience enhanced deposition than adjacent bare mudflats and (2) areas of vegetation, both natural and restored, will experience greater accretion than bare mudflats.
Materials and Methods
Field Location and Sampling Sites
The Eden Estuary (56°21′52″N, 2°50′27″W) is a small mesotidal pocket estuary on the east coast of Scotland, located between the larger Firth of Forth to the south and the Tay estuary to the north, and is situated within the Firth of Tay and Eden Estuary SAC and SPA, it is also designated as a SSSI, Ramsar site and Local Nature Reserve. The sedimentary composition with the estuary is dominated by particles classified as “fine sand” (125 and 250 μm) on the Wentworth scale (Wentworth, 1922), typically ranging between 150 and 200 μm.
The saltmarshes of the estuary are currently in an unfavorable condition due to coastal erosion and the pressure of extensive coastal defenses (SNH, 2011). Restoration efforts have been implemented to mitigate this degradation and since 2000, Maynard (2014) has been utilizing a “direct transplantation” approach. B. maritimus plants were harvested from donor marshes within the estuary and transplanted onto selected bare upper mudflat sites to encourage the spread of existing marsh as well as produce new swards. In the present study, four permanent sampling points were established in three area types (natural marsh, restored marsh, and natural mudflat; n = 12, referred to hence as “natural,” “restored,” and “mudflat”) to study the sediment dynamics therein (Figure 1). The study was conducted on the north shore of the estuary, within a confined extent, spanning ~400 m. The “natural” area comprised a robust dense mono-specific stand of B. maritimus, at an average elevation of 1.64 m, occupying the upper tidal limits of the intertidal zone. The “restored” area consisted of a mono-specific stand of well-established B. maritimus which was planted in 2010 at an average elevation of 1.76 m. The “mudflat” area was an unvegetated area running adjacent to the coast between the two extreme extents of the vegetated areas, at an average elevation of 1.22 m. Tidal inundation was calculated for each area from its known elevation (acquired by relative carrier phase positioning—Leica VIVA GS10 which has 5 mm horizontal error and 10 mm vertical error, using < 2 km baseline) and tide gauge data, contributed by the British Oceanographic Data Center (Leith−55° 59.39′N, 3° 10.902′W).
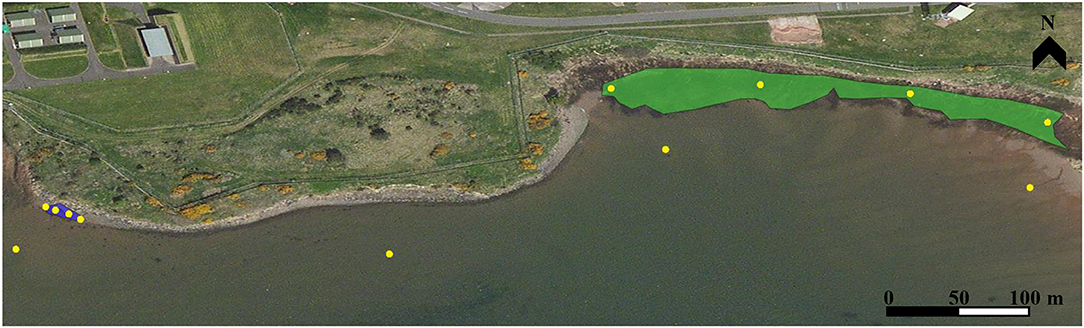
Figure 1. The north shore of the Eden Estuary, Scotland. The green polygon delineates the full extent of the “Natural” saltmarsh, an area of 450 m2, made up of a robust bed of B. maritimus. The blue polygon delineates the extent of the “Restored” area, a total of 100 m2, consisting of a planted mono-specific stand of B. maritimus. The “Mudflat” area consisted of the entire adjacent fringing edge running along the coast between the extreme ends of the natural and restored vegetated areas. The yellow points are the location of permanent sampling points.
Sampling Design
Sediment deposition and settlement were sampled on 16 occasions over a year period, on each occasion sampling took place at 12 permanent points. Sampling was carried out four times in each season; in summer from 16th to 23rd August 2015, autumn from 13th to 20th November 2015, winter from 10th to 17th February 2016 and spring from 23rd to 30th May 2016. The four sampling occasions in each season spanned across a spring to neap tidal change, beginning on a spring tide. On each occasion, sediment deposition and settlement traps were deployed during low tide and remained exposed for two flood tide events, after which they were retrieved. The next sampling occasion took place the following day at low tide, this was repeated four times each season. Sediment elevation measurements were taken once per season, following the sediment deposition and settlement sampling.
Sampling aimed to capture information on the sediment regime within the study area, where; sediment deposition was actual deposits onto the sediment surface during a sampling period, sediment settlement was the potential amount of sediment which could be deposited during a sampling period and accretion is the expression of the deposition-erosion balance evaluated through measurement of surface elevation change.
Sediment Deposition Measurement
Deposited sediment was measured using a “sediment trap” as described by Reed (1989), quantifying the passive deposition of material onto the surface of a pre-dry weighted, small pore sized (3–5 μm), paper filter disc; paper dried for 24 h at ~60°C. The study used a custom designed two-part plastic retaining system (Figure 2) to enhance filter paper retention and facilitate retrieval with minimal risk of sample disturbance. Traps used a 110 mm filter disc placed between 5 mm thick PVC base and a 0.25 mm thick acrylic retention ring with a 90 mm diameter opening (Figure 2). These were secured into the sediment with four 100 mm nails through corner holes. Following a 24 h exposure period, the complete trap was collected, and filter paper removed in the laboratory. Hydrobia sp. were occasionally present on the traps, these were removed using fine forceps, ensuring maximal retention of sediment. Papers were rinsed with distilled water to remove salt since initial testing indicated that the presence of salt prevented “ash-less” combustion of the filter paper. Rinsing was completed in two stages; with a first 100 ml wash, followed by a further 50 ml rinse. The filter papers and sediment were transferred to a dried, weighed and labeled aluminum foil dish (57 mm diameter x 13 mm deep); further distilled water rinsing ensured full sample transfer.
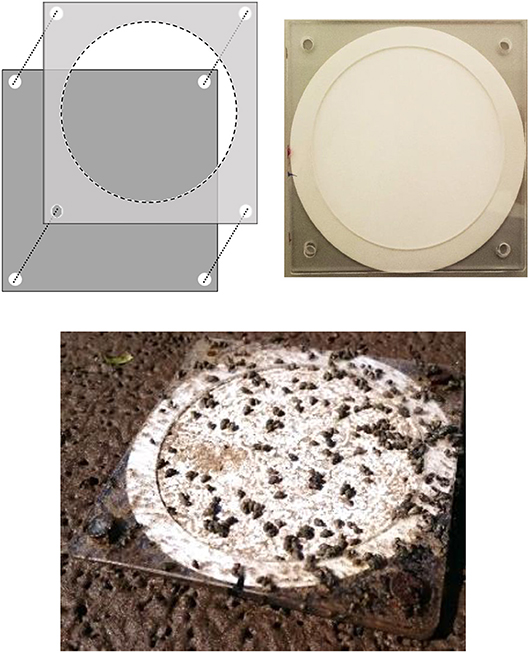
Figure 2. Top left - the deposit trap; upper light gray retention ring holds the filter paper onto the solid dark gray base plate. Four nails are pushed through the corners, affixing to the sediment. Top right - showing trap pre-deployment with filter paper installed. Bottom - showing trap in-situ post exposure with many Hydrobiid snails.
Sediment Settlement Measurement
Potential sediment for deposition was quantified as the material settling out of suspension during a sampling period, quantified using traps originally designed to measure larval settlement (Todd et al., 2006). Traps were ~300 mm tall, internally-baffled, tubes with a convex conical top and 10 mm aperture. At each sample point, a 300 by 68 mm pipe section was sunk into the sediment, leaving ~50 mm above of the surface (Figure 3). The settlement traps were filled with filtered sea water in the laboratory and affixed with cable ties into the support tubes with the collective aperture positioned 100 mm above the sediment surface (Figure 3). This placement and design was chosen to reduce disturbance of local hydrodynamics (Nolte et al., 2013), though the low height of the trap and uniform curved surface of the support cage, whilst minimizing the chance of colleting resuspended material; thus providing information on sediments settling toward the sediment surface.
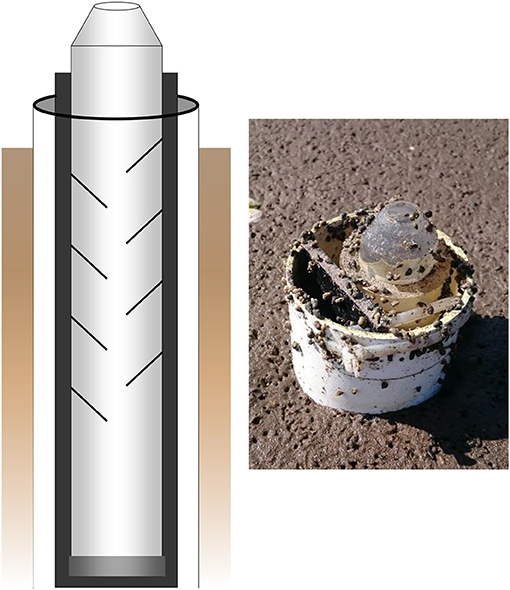
Figure 3. The settlement trap with internal baffles and conical opening. The column is attached to a backing plate (black). In the field this was placed into the sediment, sitting within the illustrated support tube.
Post exposure, tubes were collected and processed in the laboratory. Samples were emptied, by removal of the screw-on base. Traps were flushed with distilled water to remove material retained on baffles. Sediment settlement samples were centrifuged at 3,000 rpm for 15 min and sea water removed using a MORVAC filter pump. The samples were washed twice with distilled water as described above. Once washed, samples were transferred to dried, weighed and labeled aluminum dishes.
Sediment Dry Weight and Organic Content
Prepared deposit and settlement samples were dried for a minimum of 24 h at ~60°C to remove moisture. Dried samples were placed into a desiccator to cool and then weighed; sediment weight was calculated by removal of aluminum dish weight, and filter paper for deposit samples. Organic content was measured by Loss on Ignition (LOI). Samples were ashed in a muffle furnace at 450°C for 6 h, again samples were placed in a desiccator to cool then weighed; remaining sediment weight calculated by removal of aluminum boat weight, for deposit samples it was assumed the filter paper had been fully combusted.
Bed Height Measurements—SEB
Accretion rate was quantified using a modified Sedimentation Erosion Bar (SEB) (van Wijnen and Bakker, 2001; Nolte et al., 2013), which is based on the Surface Elevation Table as described by Boumans and Day (1993). Two 10 mm reinforced steel bars were sunk 1 m into the sediment 1.3 m apart, ~300 mm above of the surface. The “bar” was a 1.35 m long, 35 mm wide, 18 mm deep metal bar with holes aligned to fit the rods. Along the length of the bar, 11 equally spaced holes provide consistent measurement points. A “mm” graduated rod was used to measure the distance from the top of the bar to the sediment surface. During measurement trampling near the SEB was avoided so elevation change due natural processes alone were measured.
Elevation to Deposition Conversion
The possible amount of sediment (gain or loss) which could be expected to result in the measured change in sediment elevation was calculated. To estimate the equivalent sediment weight associated with the change in elevation a conversion was applied using surface layer bulk density values for the study area; vegetated area = 1.45 g/cm3 and mudflat = 1.30 g/cm3 (unpublished data, Taylor., 2017). The conversion assumed that the measured elevation change at each sampling point was representative of a 1 m2, applying the bulk density and elevation change to this area provided an estimate of sediment gained or lost.
Statistical Analysis
Data were analyzed using correlation, linear regression, and analysis of variance (ANOVA) tests to compare relationship between area types and the measured factors and analyses the drivers behind the measured factors.
Tidal inundation period differences between in each area were assessed using linear regression. The influence of tidal inundation on sediment deposition and settlement were then assessed using linear regression.
Correlation between all sediment deposition and settlement quantities at each sampling point for each sampling occasion were assessed. Linear regression was then used to assess how settlement rates and area type influenced measured sediment deposition quantity. The correlation between organic matter content and deposited quantity and settlement quantity were assessed for each sampling point on each sampling occasion.
Further assessment was then made toward understanding deposition rates in the study. ANOVA was used to compare deposition rates found in each area across the study, with Tukey's HSD post-hoc analysis employed to evaluate differences. ANOVA was then used to assess the influence of both area type and season on deposition.
Variables were log10 transformed where statistical tests outcomes breached the required assumptions, primarily non-normal distribution of test residuals. Deposition and settlement data were standardized to a 1 m2 area, corrected from their 63.62 and 0.78 cm2, respectively.
Results
Inundation Period
Over the sampling period there was a significant difference in estimated inundation time between the sites; total inundation times over the sampling period (16 sampling occasions) were, natural ≈ 116 ± 0.43 h, restored ≈ 109 ± 0.45 h, and mudflat ≈ 156 ± 0.24 h [F(2, 188) = 24.87, P < 0.001].
Tidal Inundation Effect on Deposition and Settlement
The influence of tidal inundation on the availability of potential sediment for deposition, as measured using the settlement tubes, was compared across the year. The dry weight of sediment and the inundation time for each point was examined to assess the relationship between these variables. Linear regression demonstrated a significant relationship (p < 0.001) between inundation period and the amount of settled sediment (r2 = 0.23). There was an average sediment settlement rate of 1.35 g per hour of inundation/m2.
Deposition rates were also compared against tidal inundation period, again assessing the total dry weight of sediment deposited and the total inundation period for each sampling point. A linear regression analysis showed there to be a significant relationship (p < 0.001, r2 = 0.17), as found with settlement rate. There was an average sediment deposition rate of 1.01 g per hour of inundation/m2.
Sediment Deposition and Settlement Rates
In some instances, the sediment deposited on the filter papers was below the level of detection (39 of 512 samples, 7.6%), and these were assigned a zero-deposition value. One deposit trap of the 194 deployed was lost during the study, due to interference by a wading bird.
There was a significant positive correlation between potential deposition (sediment settlement) and deposited material (rs = 0.672, p < 0.001). To account for possible sampling area effects (i.e., 1 cm diameter aperture vs. 9 cm diameter filter paper) data were standardized to 1 m2, averaged in each area type across each sampling occasion (n = 48). These standardized data displayed a similar significant positive correlation between potential (sediment settlement) and deposited material (rs = 0.759, p < 0.001), where deposition explained 55% of the variance [F(1, 46) = 58.57, p < 0.0001], with the relationship (log)deposited sediment weight per m2 = −3.65 + 0.9851*(log)settlement weight per m2.
This significant relationship between potential deposition and deposited material holds true within each area (Figure 4). A linear regression model assessed the influence of the interaction of settlement quantity, areas type and season on deposition [F(23, 24) = 5.345, p < 0.0001], which explained 68% of the variance in deposition; the significant interaction in the model was season:area type (p = 0.0028). Across seasons and areas, on average, more material was deposited on traps than collected in settling columns. An average difference of 0.16 g (115% more), 0.13 g (33% more), and 1.44 g (239% more) for natural, restored and mudflat areas was found, respectively.
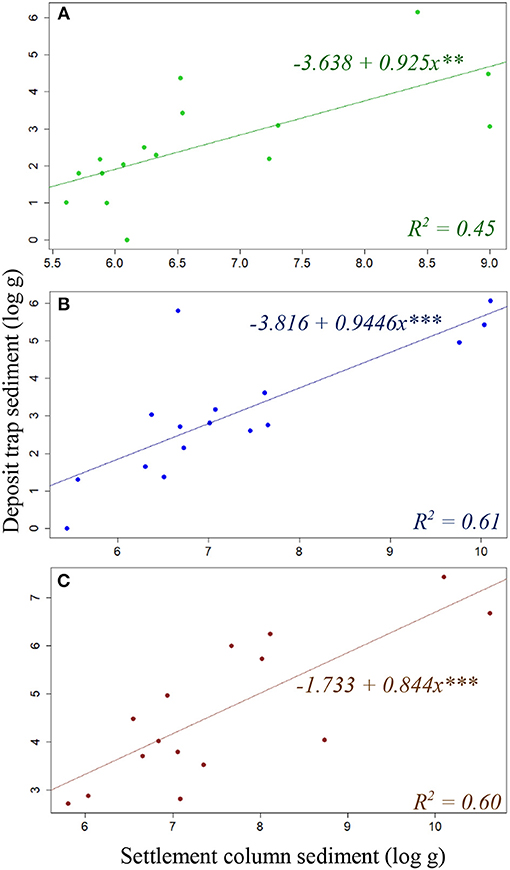
Figure 4. Sediment settlement and deposit dry weights across seasons for each area, corrected to 1 m2 equivalent, each point is averaged value of single sampling occasion. (A) Natural (green), (B) Restored (blue), (C) Mudflat (brown). Displaying linear regression trend line and equations for each area, significance codes: 0 “***”, 0.001 “**”.
When assessing the organic content (%) of deposits against the total amount of deposited sediment (dry weight, g) there was a weak positive correlation (rs = 0.195, p = 0.0068), however, for the amount of sediment caught (dry weight, g) in settlement traps there was a strong negative correlation with organic content (%) (rs = −0.48, p < 0.001). The percentage of organic matter present in sediment deposits differed between area and season (Figure 5). The natural area of B. maritimus had consistently higher organic content in deposited material than either the restored area or mudflat. The restored area although presenting lower organic content values than the natural vegetation, was generally greater than the adjacent mudflat, with an annual percentage average difference of nearly 3%. All areas displayed the same relationship with the seasons, organic content declining from a peak in summer through to winter then increasing again in spring.
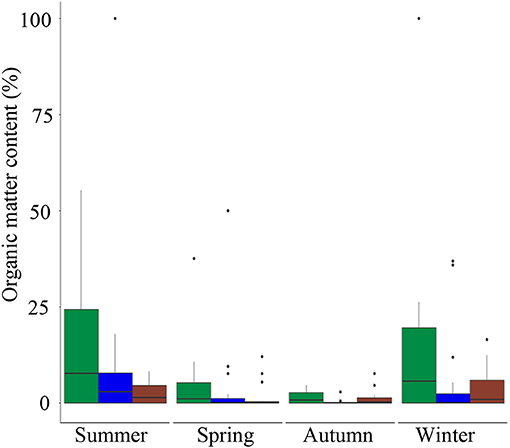
Figure 5. Percentage organic matter content measured in each area for each season. Colors represent natural area (green), restored area (blue) and the mudflat (brown). Boxes show inter-quartile range (IQR), horizontal line is the mean and whiskers extend to largest observation less than or equal to 1.5 * IQR beyond box limit.
Deposition Rates in Different Areas and Seasons
The rates of deposition measured between areas were significantly different [ANOVA F(2, 189) = 16.67, p < 0.001] (Figure 6). Tukey's HSD indicated significant differences between deposition rates over the mudflat and natural area (diff. = 3.27, p < 0.001) and mudflat and restored vegetation (diff. = 2.87, p < 0.001). There was no significant difference between natural and restored area deposition rates (p = 0.792). Overall sediment deposition rates between seasons were found to be significantly different [F(3, 187) = 7.99, p < 0.001]. However, a Tukey's HSD indicated that the only significant differences occur between spring and autumn (diff. = −2.30, p = 0.0019) and spring and winter (diff. = −2.68, p = 0.00108); all other comparisons between seasons did not significantly differ in their sediment deposition rates.
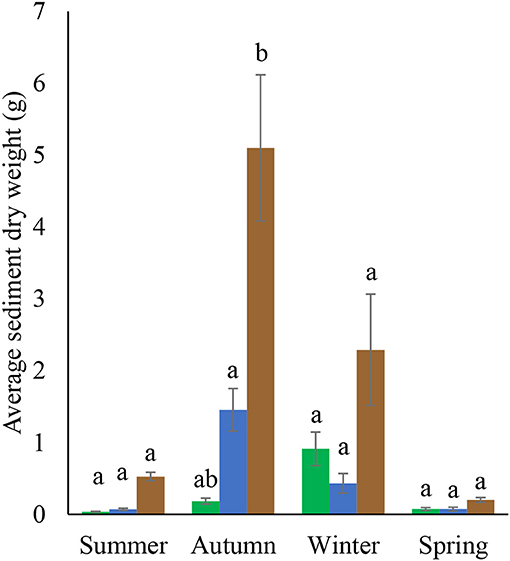
Figure 6. Averaged sediment deposition (dry weight in grams) quantities measured in each area during each season. The average in each area was calculated from samples collected at four points on four occasions (n = 16 per area). Letters show significant differences between sediment deposition across each area and season. Colors represent natural area (green), restored area (blue), and the mudflat (brown).
The influence of area type and season on deposition rates were assessed together to understand which of these factors was most influential in terms of sediment deposition rates. An ANOVA of deposition rate with season and area type (as independent variables) explained 34% of the variance in deposition rates measured [F(11, 179) = 8.31, p < 0.001]. The natural stand typically exhibited significantly different deposition rates across seasons (summer p = 0.021, autumn p = 0.030, spring p = 0.009). The restored site shows significant differences in spring (p < 0.001) and winter (p = 0.001), and mudflat areas were significantly different in autumn (p = 0.012).
Comparison of Sediment Elevation Changes
The natural area was the only area which displayed a net positive elevation change during the study (Figure 7), an average of 7.5 mm increase. The restored and mudflat areas both experienced a net lowering in elevation of 1.6 and 6.0 mm, respectively (Figure 7). Measured elevation changes highlighted a discontinuity between rates of deposition and accretion. A comparison of potential total dry weight depositional accumulation and converted elevation changes to equivalent sediment weights shows large differences between potential accretion and actual retention (Table 1). There was a pronounced difference between vegetated and mudflat areas in terms of sediment retention during the study. Mudflats attracted over four times more deposition than the vegetated zones, though mudflat rate of loss was greater than for both vegetated areas (Table 1). The natural areas experienced the least potential accretion input (lowest measured deposition rate) (Figure 6) but retained more material (reduced erosion rates) than other areas; retaining an estimated 6.3% of deposited material (Table 1). A comparison of the calculated difference between an assumed constant-deposition-no-erosion and converted actual elevation change shows areas of vegetation to be more similar than the un-vegetated mudflat area, which displayed the greatest difference of an estimated 118 kg/m2 discrepancy over the study (Table 1).
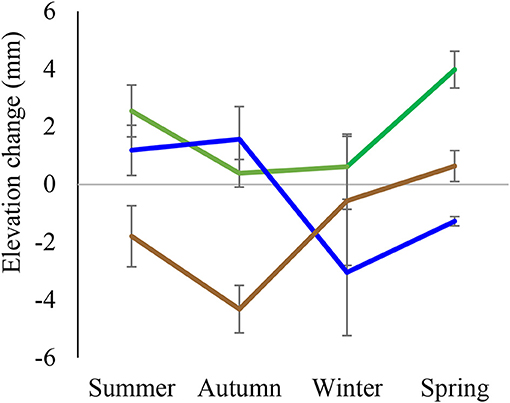
Figure 7. Average changes in sediment elevation between seasons measured in each of the study areas; Natural = green, Restored = blue, and Mudflat = brown. Four Sediment Elevation Bar (SEB) in each area were measured once per season, each SEB consisted of 11 measurments, data shown is average change across each area. Standard error bars taken from across measurments at each site in each season.
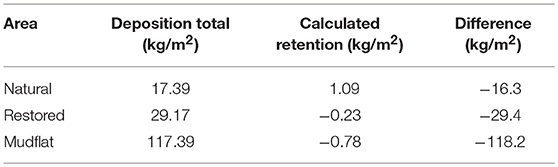
Table 1. Estimate of sediment deposition dry weight taken from deposition rate data (assuming a constant accretion and full sediment retention) and actual elevation change (applying a bulk density conversion to generate a dry weight) and the difference in assumed constant deposited accretion and actual accumulation rates.
Discussion
Saltmarsh restoration and conservation is of increasing importance under the present threat of loss and increasing future risk (Boorman, 2003; Craft et al., 2009; McLeod et al., 2011). The restoration of saltmarsh areas through direct transplanting offers possible opportunities to diversify conservation initiatives beyond typical managed realignment projects. This study shows that restored marshes have the potential to trap sediment as effectively as natural analogs, however restored marshes failed to retain that sediment sufficiently to raise elevation at equivalent rates to natural marshes by the end of the study. It was hypothesized that restored areas would experience enhanced deposition over the adjacent bare mudflat, however, the study showed that this was not the case, with both vegetated (natural and restored) areas experiencing less sediment deposition than the mudflat. It was further hypothesized that the vegetated areas would experience greater rates of accretion, which is partly correct, with the only increase in elevation found in the natural area and the restored area experiencing less elevation loss than the mudflat. The study took advantage of a valuable opportunity to study the effects of saltmarsh restoration as compared to its natural donor marsh within a relatively spatially discreet area. The study was restricted to the estuarine area where restoration and natural stands were present, ideally exposed to similar environmental conditions. However, this restriction limited site availability, so preventing site-level replication and resulting in the mudflat being ~40 cm lower than the vegetated areas which may influence the sediment dynamics studied; these limitations are acknowledged but are not considered to undermine the value of this comparative study.
Deposition and Elevation Trends
Rates of deposition within vegetated areas (both natural and restored) were shown to be lower than those measured on the mudflat (Figure 6). The similar deposition rates of the restored and natural areas suggest that, although higher in the tidal frame and so experiencing different tidal inundation, the restored area was functionally similar from the perspective of deposition rates and the organic content of deposits. This study focused on deposition onto the sediment platform, but it is possible that sediment intercepted and retained by vegetative structures eventually enhance overall sediment surface deposition and accretion, which is not purposefully measured here. Over the study period the natural stand of vegetation showed an increased sediment elevation (Figure 7), showing a consistent trend of accretion, with the mudflat displaying the greatest loss in elevation of 6.0 mm (Figure 7); highlighting a discontinuity between deposition rates and the resulting changes in sediment elevation. The studied restored area was well-established and appears to be persisting, but does not appear to have achieved the same sediment retention capacity, possibly a result of the limited size of the sward so reducing the conferred protection to sediment resuspension or a product of its different exposure within the tidal frame. However, the study indicates the restored area has shifted from the more dynamic state of the adjacent mudflats (Bouma et al., 2016), which experienced significantly greater deposition and the greatest net erosion. Vegetated areas were shown to be accretionary or substantially less erosional than the unvegetated mudflat area. The restored site, which previously is assumed to closely reflect the bare mudflat condition, now occupies a “middle ground” between the dynamic mudflat and the accretional natural vegetation.
Settlement, Deposition, and Erosion/Accretion Relationships
Analysis suggests that area type has limited influence over the settlement-deposition relationship, as indicated by the similar regression relationships for each area; perhaps driven by broader scale forces, such as tidal flow. The two vegetated areas show that higher proportions of the “potential sediment” supply are deposited at any given time point. The slightly lower explained variance in the natural area suggests different factors could influence the relationship between potential deposition and realized deposition in this area. It is likely that characteristics such as elevation (flood height) and vegetative structure should be considered in understanding the settlement-deposition relationship. The link between settlement (potential deposition) and actual deposition is one factor which can influence the persistence saltmarsh system; however, this study suggests that resulting accretion rates could play a greater role.
The deposition-erosion balance reported in the restored area differs from that of the local natural area, such that there was a net loss of material over the study, a trend which may have the greatest adverse effect on the persistence of the restored area. If the trend of elevation loss recorded in this short-term study reflect that of the continued long-term situation of the marsh area, coupled with RSLR, could leave such areas vulnerable to drowning. It will be important to monitor these interactions and feedbacks and assess how these alter as the restoration matures and expands laterally. The differing mechanisms behind sediment accretion acting within the natural and restored area are not readily revealed by this study. Investigation into drivers of sediment retention in each of the areas studied could facilitate improved restoration approaches, to deliver resilient sites which maintain themselves within a tidal frame; such as tidal range (inundation frequency) (van Wijnen and Bakker, 2001; Mudd et al., 2009; Kirwan et al., 2010), suspended sediment concentrations (Kirwan et al., 2010), and elevation (Crooks et al., 2002).
Organic Matter Input Trends
The data suggested there is little input of organic matter into this benthic system via settling sediment, where the suspended load seems dominated by material lower in organic content, however, the organic content of deposited material was higher. This proportionally greater organic matter content measured in deposits indicates the depositional processes favored the retention of organic matter relative to the potential deposition material. In the case of the natural area, where higher organic content coupled with net vertical accretion, there is potential for such vegetated intertidal areas to act as sites of carbon burial. The study showed the restored area to also retain higher quantities of organic matter in deposits, though experienced a net loss in elevation. If the development of the restored area led to the net accretion of sediment, this would likely deliver ecosystem services, such as carbon sequestration (Burden et al., 2013).
The seasonal relationship with organic matter highlights the possible importance of allochthonous material in the estuary. In the “growing” months of summer and spring there is a larger quantity of organic matter being deposited in all areas; likely a result of increased organic matter availability from plant growth, both within the estuary and from alluvial-terrestrial inputs. However, the change between the seasons is far greater in the vegetated areas, with a maximum difference of 14.2% in the natural area and 10.3% in the restored area; compared to a 2.7% change on the mudflat. This variance illustrates the functional capacity of vegetative structures, which encourage the deposition and retention of finer organic particles through flow retardation. This characteristic may a provide crucial feedback mechanism as the restored area continues to develop, increasing the organic content of the sediment bed during critical growth phases and so enhancing growth capacity.
Conclusion
It is not yet clear how the current sedimentary interactions will affect the long-term survival and evolution of this site, specifically the persistence of the restored area. Although the data suggests the site of restoration is experiencing a different biogeomorphic situation compared to the bare mudflat, which is assumed to reflect its previous state, this situation does not yet mirror its natural counterpart. This study illustrates the subtleties of saltmarsh development and feedbacks, highlighting the importance of site selection in restoration activities. The “established” appearance of the restored planted saltmarsh disguises its possible vulnerability in dynamic sedimentary interactions leading to net erosion across the site.
The high cost/effort to size ratio of direct planting makes it important to minimize the risks of failure of any project through a continued improvement of our understanding of interactions between vegetation and sediment resulting from restoration (Maynard, 2014). This study has highlighted the possible vulnerability of restored areas, which may visually appear established, but whose processes do not indicate a robust ecosystem; specifically, net elevation loss during the study. The delay for restoration to achieve comparable ecological functionality to natural areas is important to consider when carrying out restoration projects and may require additional effort once established to ensure their persistence; for example, increased planting effort to continue natural expansion. Such information could advance approaches and maximize the delivery of conservation objectives which are crucial to prevent the loss of these systems.
Author Contributions
BT carried out collection and analysis of samples used toward completion of this research and completed the written output of this article. DP and JB provided significant input into the planning and design of the research and valuable insight and development during the creation of the manuscript.
Funding
The authors received funding from the MASTS pooling initiative (The Marine Alliance for Science and Technology for Scotland) and Scottish Natural Heritage (SNH), their support is gratefully acknowledged. MASTS is funded by the Scottish Funding Council (grant reference HR09011) and contributing institutions.
Conflict of Interest Statement
The authors declare that the research was conducted in the absence of any commercial or financial relationships that could be construed as a potential conflict of interest.
Acknowledgments
Thanks to the UK Tide Gauge Network for tide date, provided through the British Oceanographic Data Center. Thanks also goes to Dr. Clare Maynard for her past and continuing efforts to restore the saltmarshes of the Eden Estuary, on which this study is based. Finally, thank you to SNH and Fife Coast and Countryside Trust for their helpful input and permissions to carry out the research.
References
Allen, J. R. L., and Pye, K. (1992). Saltmarshes: Morphodynamics, Conservation and Engineering Significance. Cambridge: Cambridge University Press.
Baptist, M. J., de Groot, A. V., and van Duin, W. E. (2016). Contrasting biogeomorphic processes affecting salt-marsh development of the Mokbaai, Texel, The Netherlands. Earth Surf. Process. Landforms 41, 1241–1249. doi: 10.1002/esp.3949
Barbier, E. B., Hacker, S. D., Kennedy, C., Koch, E. W., Stier, A. C., and Silliman, B. R. (2011). The value of estuarine and coastal ecosystem services. Ecol. Monogr. 81, 169–193. doi: 10.1890/10-1510.1
Beaumont, N. J., Jones, L., Garbutt, A., Hansom, J. D., and Toberman, M. (2014). The value of carbon sequestration and storage in coastal habitats. Estuar. Coast. Shelf Sci. 137, 32–40. doi: 10.1016/j.ecss.2013.11.022
Boorman, L. (2003). Saltmarsh review: an overview of coastal saltmarshes, their dynamic and sensitivity characteristics for conservation and management. JNCC Rep. 334:132.
Boorman, L. A., Garbutt, A., and Barratt, D. (1998). The role of vegetation in determining patterns of the accretion of salt marsh sediment. Sediment. Process. 139, 389–399. doi: 10.1144/GSL.SP.1998.139.01.29
Bos, A. R., Bouma, T. J., de Kort, G. L. J., and van Katwijk, M. M. (2007). Ecosystem engineering by annual intertidal seagrass beds: sediment accretion and modification. Estuar. Coast. Shelf Sci. 74, 344–348. doi: 10.1016/j.ecss.2007.04.006
Bouma, T. J., De Vries, M. B., Low, E., Kusters, L., Herman, P. M. J., Tánczos, I. C., et al. (2005). Flow hydrodynamics on a mudflat and in salt marsh vegetation: identifying general relationships for habitat characterisations. Hydrobiologia 540, 259–274. doi: 10.1007/s10750-004-7149-0
Bouma, T. J., van Belzen, J., Balke, T., van Dalen, J., Klaassen, P., Hartog, A. M., et al. (2016). Short-term mudflat dynamics drive long-term cyclic salt marsh dynamics. Limnol. Oceanogr. 61, 2261–2275. doi: 10.1002/lno.10374
Boumans, R., and Day, J. W. (1993). High precision measurements of sediment elevation in shallow coastal areas using a sedimentation-erosion table. Estuaries 16, 375–380. doi: 10.2307/1352509
Burd, F. (1989). The Saltmarsh Survey of Great Britain - An Inventory of British Saltmarshes. Nature Conservancy Council, Peterborough. doi: 10.1007/s13398-014-0173-7.2
Burden, A., Garbutt, R. a., Evans, C. D., Jones, D. L., and Cooper, D. M. (2013). Carbon sequestration and biogeochemical cycling in a saltmarsh subject to coastal managed realignment. Estuar. Coast. Shelf Sci. 120, 12–20. doi: 10.1016/j.ecss.2013.01.014
Chen, S.-N., Sanford, L. P., Koch, E. W., Shi, F., and North, E. W. (2007). A nearshore model to investigate the effects of seagrass bed geometry on wave attenuation and suspended sediment transport. Estuar. Coasts 30, 296–310. doi: 10.1007/BF02700172
Chmura, G., Anisfeld, S., Cahoon, D. R., and Lynch, J. (2003). Global carbon sequestration in tidal, saline wetland soils. Global Biogeochem. Cycles 17:1111. doi: 10.1029/2002GB001917
Costanza, R., de Groot, R., Sutton, P., van der Ploeg, S., Anderson, S. J., Kubiszewski, I., et al. (2014). Changes in the global value of ecosystem services. Glob. Environ. Change 26, 152–158. doi: 10.1016/j.gloenvcha.2014.04.002
Costanza, R., Pérez-Maqueo, O., Martinez, M. L., Sutton, P., Anderson, S. J., and Mulder, K. (2008). The value of coastal wetlands for hurricane protection. AMBIO 37, 241–248. doi: 10.1579/0044-7447(2008)37[241:TVOCWF]2.0.CO;2
Craft, C., Clough, J., Ehman, J., Joye, S., Park, R., Pennings, S., et al. (2009). Forecasting the effects of accelerated sea-level rise on tidal marsh ecosystem services. Front. Ecol. Environ. 7, 73–78. doi: 10.1890/070219
Crooks, S., Schutten, J., Sheern, G. D., Pye, K., and Davy, A. J. (2002). Drainage and elevation as factors in the restoration of Salt Marsh in Britain. Restor. Ecol. 10, 591–602. doi: 10.1046/j.1526-100X.2002.t01-1-02036.x
Crosby, S. C., Sax, D. F., Palmer, M. E., Booth, H. S., Deegan, L. A., Bertness, M. D., et al. (2016). Salt marsh persistence is threatened by predicted sea-level rise. Estuar. Coast. Shelf Sci. 181, 93–99. doi: 10.1016/j.ecss.2016.08.018
Donato, D. C., Kauffman, J. B., Murdiyarso, D., Kurnianto, S., Stidham, M., and Kanninen, M. (2011). Mangroves among the most carbon-rich forests in the tropics. Nat. Geosci. 4, 293–297. doi: 10.1038/ngeo1123
Duarte, C. M., Dennison, W. C., Orth, R. J. W., and Carruthers, T. J. B. (2008). The charisma of coastal ecosystems: addressing the imbalance. Estuar. Coasts 31, 233–238. doi: 10.1007/s12237-008-9038-7
Fourqurean, J. W., Duarte, C. M., Kennedy, H., Marbà, N., Holmer, M., Mateo, M. A., et al. (2012). Seagrass ecosystems as a globally significant carbon stock. Nat. Geosci. 5, 505–509. doi: 10.1038/ngeo1477
King, S. E., and Lester, J. N. (1995). The value of salt marsh as a sea defence. Mar. Pollut. Bull. 30, 180–189. doi: 10.1016/0025-326X(94)00173-7
Kirwan, M. L., Guntenspergen, G. R., D'Alpaos, A., Morris, J. T., Mudd, S. M., and Temmerman, S. (2010). Limits on the adaptability of coastal marshes to rising sea level. Geophys. Res. Lett. 37:L23401. doi: 10.1029/2010GL045489
Kirwan, M. L., and Megonigal, J. P. (2013). Tidal wetland stability in the face of human impacts and sea-level rise. Nature 504, 53–60. doi: 10.1038/nature12856
Kirwan, M. L., Temmerman, S., Skeehan, E. E., Guntenspergen, G. R., and Faghe, S. (2016). Overestimation of marsh vulnerability to sea level rise. Nat. Clim. Change 6, 253–260. doi: 10.1038/nclimate2909
Koch, E. W., Barbier, E. B., Silliman, B. R., Reed, D. J., Perillo, G. M. E., Hacker, S. D., et al. (2009). Non-linearity in ecosystem services: temporal and spatial variability in coastal protection. Front. Ecol. Environ. 7, 29–37. doi: 10.1890/080126
Maris, T., Cox, T., Temmerman, S., De Vleeschauwer, P., Van Damme, S., De Mulder, T., et al. (2007). Tuning the tide: creating ecological conditions for tidal marsh development in a flood control area. Hydrobiologia 588, 31–43. doi: 10.1007/s10750-007-0650-5
Masselink, G., Hanley, M. E., Halwyn, A. C., Blake, W., Kingston, K., Newton, T., et al. (2017). Evaluation of salt marsh restoration by means of self-regulating tidal gate – Avon estuary, South Devon, UK. Ecol. Eng. 106, 174–190. doi: 10.1016/j.ecoleng.2017.05.038
Maynard, C. (2014). Restoring the Degraded Shoreline. St. Andrews; Boca Raton, FL: University of St. Andrews.
McLeod, E., Chmura, G., and Bouillon, S. (2011). A blueprint for blue carbon: toward an improved understanding of the role of vegetated coastal habitats in sequestering CO2. Front. Ecol. 9, 552–560. doi: 10.1890/110004
Mcowen, C. J., Weatherdon, L. V., Bochove, J.-W., Van Sullivan, E., Blyth, S., Zockler, C., et al. (2017). A global map of saltmarshes. Biodivers. Data J. 5:e11764. doi: 10.3897/BDJ.5.e11764
Mudd, S., Howell, S., and Morris, J. (2009). Impact of dynamic feedbacks between sedimentation, sea-level rise, and biomass production on near-surface marsh stratigraphy and carbon accumulation. Estuar. Coast. Shelf Sci. 82, 377–389. doi: 10.1016/j.ecss.2009.01.028
Mudd, S. M., D'Alpaos, A., and Morris, J. T. (2010). How does vegetation affect sedimentation on tidal marshes? Investigating particle capture and hydrodynamic controls on biologically mediated sedimentation. J. Geophys. Res. Earth Surf. 115:F03029. doi: 10.1029/2009JF001566
Nolte, S., Koppenaal, E. C., Esselink, P., Dijkema, K. S., Schuerch, M., De Groot, A. V., et al. (2013). Measuring sedimentation in tidal marshes: a review on methods and their applicability in biogeomorphological studies. J. Coast. Conserv. 17, 301–325. doi: 10.1007/s11852-013-0238-3
Potouroglou, M., Bull, J. C., Krauss, K. W., Kennedy, H. A., Fusi, M., Daffonchio, D., et al. (2017). Measuring the role of seagrasses in regulating sediment surface elevation. Sci. Rep. 7:11917. doi: 10.1038/s41598-017-12354-y
Reed, D. J. (1989). Patterns of sediment deposition in subsiding coastal salt marshes, Terrebonne Bay, Louisiana: the role of winter storms. Estuaries 12, 222–227. doi: 10.1007/BF02689700
Reed, D. J., Spencer, T., Murray, A. L., French, J. R., and Journal, S. (1999). Marsh surface sediment deposition and the role of tidal creeks: implications for created and managed coastal marshes. J. Coast. Conserv. 5, 81–90.
Schrift, A. M., Mendelssohn, I. A., and Materne, M. D. (2008). Salt marsh restoration with sediment-slurry amendments following a drought-induced large-scale disturbance. Wetlands 28, 1071–1085. doi: 10.1672/07-78.1
Schuerch, M., Spencer, T., Temmerman, S., Kirwan, M. L., Wolff, C., Lincke, D., et al. (2018). Future response of global coastal wetlands to sea-level rise. Nature 561, 231–234. doi: 10.1038/s41586-018-0476-5
Schwarz, C., Bouma, T. J., Zhang, L. Q., Temmerman, S., Ysebaert, T., and Herman, P. M. J. (2015). Interactions between plant traits and sediment characteristics influencing species establishment and scale-dependent feedbacks in salt marsh ecosystems. Geomorphology 250, 298–307. doi: 10.1016/j.geomorph.2015.09.013
Schwarz, C., Gourgue, O., van Belzen, J., Zhu, Z., Bouma, T. J., van de Koppel, J., et al. (2018). Self-organization of a biogeomorphic landscape controlled by plant life-history traits. Nat. Geosci. 11, 672–677. doi: 10.1038/s41561-018-0180-y
Sullivan, G. (2001). “Establishing vegetation in restored and created coastal wetlands,” in Handbook for Restoring Tidal Wetlands, ed B. Zedler (Boca Raton, FL: CRC Press, 119–156.
Thorne, K. M., Elliott-Fisk, D. L., Wylie, G. D., Perry, W. M., and Takekawa, J. Y. (2014). Importance of biogeomorphic and spatial properties in assessing a tidal salt marsh vulnerability to sea-level rise. Estuar. Coasts 37, 941–951. doi: 10.1007/s12237-013-9725-x
Todd, C. D., Phelan, P. J. C., Weinmann, B. E., Gude, A. R., Andrews, C., Paterson, D. M., et al. (2006). Improvements to a passive trap for quantifying barnacle larval supply to semi-exposed rocky shores. J. Exp. Mar. Biol. Ecol. 332, 135–150. doi: 10.1016/j.jembe.2005.11.013
van Proosdij, D., Davidson-Arnott, R. G. D., and Ollerhead, J. (2006). Controls on spatial patterns of sediment deposition across a macro-tidal salt marsh surface over single tidal cycles. Estuar. Coast. Shelf Sci. 69, 64–86. doi: 10.1016/j.ecss.2006.04.022
van Wijnen, H. J., and Bakker, J. P. (2001). Long-term surface elevation change in salt marshes: a prediction of marsh response to future sea-level rise. Estuar. Coast. Shelf Sci. 52, 381–390. doi: 10.1006/ecss.2000.0744
Wentworth, C. K. (1922). A scale of grade and class terms for clastic sediments. J. Geol. 30, 377–392. doi: 10.1086/622910
Keywords: saltmarsh, sediment deposition, accretion, seasonal, restoration
Citation: Taylor BW, Paterson DM and Baxter JM (2019) Sediment Dynamics of Natural and Restored Bolboschoenus maritimus Saltmarsh. Front. Ecol. Evol. 7:237. doi: 10.3389/fevo.2019.00237
Received: 14 December 2018; Accepted: 07 June 2019;
Published: 26 June 2019.
Edited by:
Martin Wiggers Skov, Bangor University, United KingdomReviewed by:
Cai Ladd, Bangor University, United KingdomJordi F. Pagès, University of Barcelona, Spain
Copyright © 2019 Taylor, Paterson and Baxter. This is an open-access article distributed under the terms of the Creative Commons Attribution License (CC BY). The use, distribution or reproduction in other forums is permitted, provided the original author(s) and the copyright owner(s) are credited and that the original publication in this journal is cited, in accordance with accepted academic practice. No use, distribution or reproduction is permitted which does not comply with these terms.
*Correspondence: Benjamin W. Taylor, Ynd0LmJlbnRheWxvciYjeDAwMDQwO2dtYWlsLmNvbQ==