- Department of Biology, Georgetown University, Washington, DC, United States
Disruptive effects of climate change include range shifts, phenological mismatches among consumers and producers, and population declines. While these biological alterations have been widely documented, studies identifying specific mechanisms linking climate change to population declines are scarce. Extreme events, such as heatwaves can have devastating effects on living organisms and are increasing in frequency as Earth warms. Hence, understanding the effects of heatwaves on insects is necessary to inform conservation efforts and to develop predictions of population dynamics under future climate scenarios. Here, we experimentally evaluated the effects of heatwaves on the survival and phenology of the Baltimore Checkerspot (Euphydryas phaeton phaeton), a wetland butterfly with imperiled populations that has incorporated a novel host. We performed laboratory manipulations (implementing realistic temperature regimes) to assess the effect of heatwaves during summer and winter on the survival and phenology of E. p. phaeton. In addition, we analyzed historical temperature records to quantify the incidence of heatwaves within E. p. phaeton's range to assess their potential role in the decline of southeastern populations. We found that winter heatwaves with maximum temperatures of 20°C can have more devastating effects on survival than summer heatwaves (up to 41°C). Eggs endured acute heat stress during summer with no significant effects on phenology and survival; similarly, pre-overwintering larvae were robust to heatwave exposure, as only the most intense heatwave treatment reduced their survival (37% reduction compared to control conditions). By contrast, dormant larvae were the most vulnerable stage, as they lost from 2 to 6% of their body mass after a three-day summer heatwave. Furthermore, their exposure to winter heatwaves resulted in 75 to 100% mortality. Feeding on the native host provided higher resilience under thermal stress than feeding on the invasive, recently acquired host. Finally, both heatwave incidence and severity have increased in the southern range of E. p. phaeton in the period from 1894 to 2011. We show that warm winter days induced severe mortality, providing a mechanistic explanation of how climate change can trigger population declines in E. p. phaeton and other insects.
Introduction
Disruptive effects of climate change such as range shifts, phenological advances (Parmesan and Yohe, 2003; Parmesan, 2007), local extinctions (Parmesan, 2006), and phenological mismatches among consumers and producers (Gordo and Sanz, 2005; Parmesan, 2007; Yang and Rudolf, 2010; Ovaskainen et al., 2013) have been widely documented. Despite the growing number of studies linking altered phenological patterns (Parmesan, 2007) and decrements in arthropod abundance to altered temperature regimes (Lister and Garcia, 2018), studies identifying the specific mechanisms that result in insect decline (e.g., death, emigration) are scarcer (Cahill et al., 2012). Extreme events, such as heatwaves are becoming more common as a result of climate change (Hansen et al., 2012), and can have detrimental effects on both human populations (Xu et al., 2016) and ecosystem dynamics (Ummenhofer and Meehl, 2017). Temperature extremes can trigger changes in plant mortality, community composition and productivity; which in turn affect carbon cycling (Frank et al., 2015; Ummenhofer and Meehl, 2017). Arthropods are not exempt from susceptibility to extreme events; studies in the laboratory have shown that summer heat waves (5 days at constant 42°C) diminished male reproduction and sperm function in the flour beetle Triboleum castaneum not only in the generation experiencing them, but also in their offspring (Sales et al., 2018). Studies with lepidopterans showed that exposure to heatwaves of tropical butterflies resulted in prolonged development time, and a reduction of both pupal mass and immune function (Fischer et al., 2014). Furthermore, exposure of immature stages of the moth Plutella xylostella to high temperatures resulted in decreased adult lifespan, fecundity, and altered oviposition patterns (Zhang et al., 2015b). Despite experimental evidence of detrimental effects of temperature extremes on individuals, attribution of insect population declines to specific extreme events remains challenging, as it would require careful monitoring of both extreme events and insect populations. The frequency, intensity and duration of heatwaves are predicted to increase as Earth's mean temperature keeps rising (Meehl and Tebaldi, 2004; Perkins et al., 2012; Christidis et al., 2015). Thus, it is important to understand their effects on insects, which are experiencing generalized population declines (Hallmann et al., 2017) in which climate change has been identified as a key contributing factor (Lister and Garcia, 2018; Sánchez-Bayo and Wyckhuys, 2019).
The high temperatures that characterize summer heatwaves (~40°C) challenge insect physiological limits and can result in death by impaired metabolic activity (Addo-bediako et al., 2000; Chown, 2001). However, unseasonably warm temperatures throughout the year can also have deleterious effects, even if they fall well below the upper physiological limits of insects. For example, warm spells during winter can result in reduced overwinter survival and they can also trigger altered phenological patterns such as early onset of spring activities (Williams et al., 2015). Early spring activity increases the risk of exposure to harsh spring weather (Augspurger, 2013) and can trigger phenological mismatches among consumers and producers (Kudo and Ida, 2013). Food scarcity resulting from altered phenological patterns has been identified as the most important factor by which climate change results in local extinctions of animal populations (Cahill et al., 2012). Hence, unseasonal weather at any time of the year has the potential to be detrimental.
Deleterious effects of climate change on animal populations are often exacerbated by other environmental problems that affect the abundance and quality of food sources (Dirzo et al., 2014). Altered plant community composition, resulting from habitat destruction and colonization by invasive species, exposes herbivorous insects to novel hosts, often triggering host switches or expansions (Moran and Alexander, 2014). The incorporation of novel hosts into insect diets can have deleterious effects on insect populations because foliage quality often differs among novel and native hosts (Rosenwald et al., 2017). In addition, novel hosts have different phenological patterns; which in turn can affect the seasonality of their consumers (Batalden and Oberhauser, 2015); For oligophagous insects, host plant choice can drastically affect fitness, as it affects development time, fecundity (Awmack and Leather, 2002) and their relationships with predators (Bowers, 1980). Furthermore, feeding on high quality hosts can mitigate thermal stress (Diamond and Kingsolver, 2010). Thus, host quality is an important factor to consider when assessing the effects of climate change on herbivorous insects.
Here, we evaluate the effects of summer and winter heatwaves on the performance of a wetland, oligophagous butterfly, the Baltimore Checkerspot (Euphydryas phaeton phaeton, Drury Nymphalidae: Melitaeini). While northern populations of E. p. phaeton are stable (Bowers and Richardson, 2013), severe declines have been documented in the southern edge, where it is considered endangered (Durkin, 2009; Frye et al., 2013). Because E. p. phaeton have only one generation per year, temperature regimes experienced by each ontogenetic stage differ drastically (Figure 1). In our experiments, we matched abnormally warm periods with the corresponding ontogenetic stage that is most likely to experience them. Acknowledging differences in thermal sensitivity among ontogenetic stages is necessary to avoid biases when assessing species' vulnerability to climate change (Levy et al., 2015). In addition, we considered the effect of nutrition at mitigating thermal stress. North eastern populations of E. p. phaeton have two primary hosts: the native Chelone glabra L. (white turtlehead) and the invasive Plantago lanceolata L. (English plantain, Plantaginaceae), which was recently acquired as a host (Stamp, 1979). We performed a series of laboratory manipulations, implementing realistic temperature regimes, to determine the susceptibility to heatwaves of different ontogenetic stages of E. p. phaeton (eggs, active larvae, dormant larvae), and to assess the role of host plant at mitigating thermal stress. Finally, we analyzed historical temperature records from weather stations in two sites within E. p. phaeton's range to assess whether heatwave incidence, duration and intensity have recently increased.
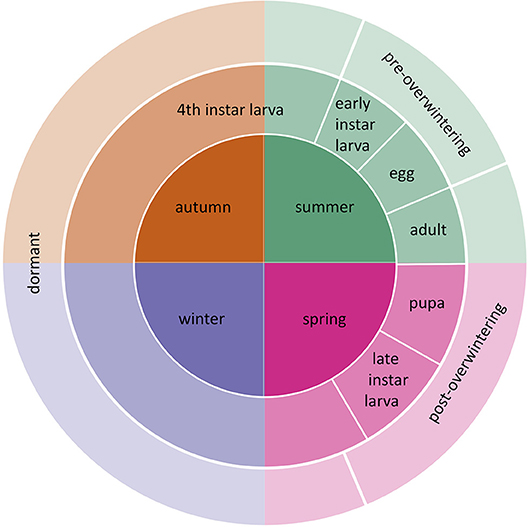
Figure 1. Life cycle of E. p. phaeton and the corresponding season when each stage occurs. Counterclockwise, eggs are laid in summer, they hatch into pre-overwintering larvae, which turn into dormant larvae upon molting into 4th instar. Larvae remain dormant during part of the summer, autumn, and winter. Post-overwintering larvae resume feeding and pupate the following spring and adults start the next life cycle.
Methods
Study System
Euphydryas phaeton are univoltine butterflies native to Eastern North America. Two subspecies have been described: E. p. phaeton (Drury), in the east, and E. p. ozarkae (Masters), in the montane southwestern part of their range (Robertson, 2015). Adults mate and lay eggs in large clusters during summer, eggs hatch within 3 weeks, larvae feed until the third instar, molt, and fourth instar larvae remain in a state of low metabolic activity from late-summer until the following spring, when they pupate and emerge as adults. Thus, eggs and young larvae (1st to 3rd instar) develop while exposed to hot summer temperatures. By contrast, 4th instar larvae experience a broad temperature range throughout their dormancy, which lasts about 8 months, from the end of summer to the following spring, when they complete development (Figure 1). Northern populations of E. p. phaeton have two oviposition hosts; the native C. glabra, and the invasive P. lanceolata, which was introduced from Eurasia and was first reported in North America about 190 years ago (Cavers et al., 1980). Euphydryas p. ozarkae has an additional oviposition host, the hemiparasitic Aureolaria flava, L. Farw. (Smooth Yellow Foxglove, Orobanchaceae, (Robertson, 2015). The incorporation of P. lanceolata to E. p. phaeton's diet has allowed for colony persistence in areas where C. glabra is absent or disappearing (Bowers and Richardson, 2013); however, feeding on P. lanceolata has some potential costs including higher predation risk due to enhanced palatability to birds (Bowers, 1980), lower growth rate and food efficiency conversion (Bowers et al., 1992) and the risk of death by haying due to grass management practices (Bowers and Richardson, 2013). Herbivore host preferences do not necessarily track foliage quality, as generalists often use hosts according to their relative abundance and not their nutritional value (Mason et al., 2011). Changes in abundance of P. lanceolata due to land management practices has already lead to local extinctions of a closely related species, E. editha (Singer and Parmesan, 2018). While northern populations of E. p. pheaton use P. lanceolata as both oviposition and secondary (post-diapause) host (Bowers and Richardson, 2013), populations in Maryland, USA have been reported to use P. lanceolata solely as a secondary host (Pers. Comm. Jen Selfridge). For our experiments, we maintained a captive colony of E. p. phaeton with founders from Cape Cod, MA, a population that feeds primarily on P. lanceolata. This colony is supplemented with eggs collected from wild females of the same population every year; we indicate in each assay whether wild-caught or lab-reared individuals were included.
Experiment Design
To evaluate the effects of heatwaves on E. p. phaeton throughout their lifecycle, we performed two sets of experimental manipulations, simulating summer and winter conditions. We used growth chambers (models 136 VL and 130 VL, Percival Scientific, Perry, IA, USA) to expose individuals to either typical or heatwave conditions during the season they naturally occur. Because E. p. phaeton lay eggs in clusters, we were able to control for genetic variation in many of our experiments by splitting clutches of siblings onto the different treatments of a given assay. We exposed eggs, pre-overwintering (1st to 3rd instar) and dormant larvae (4th instar) to summer heatwaves and dormant larvae to winter heatwaves. We used different individuals in each assay to avoid potential cumulative effects of repetitive heatwave exposure. In all treatments, temperature oscillated daily approximating a sinusoidal function. We used generalized linear and mixed effects models (GLM & GLMM) to compare performance estimates (e.g., survival, development time, larval mass) among experimental conditions. GLMMs allowed us to incorporate both the fixed effects of interest (e.g., temperature regime) as well as random effects (e.g., of clutch). We modeled survival over a specific interval as a Bernoulli trial and thus used a binomial distribution and logit link function; we modeled development time as the count of days to complete a given life stage and thus used a Poisson distribution with a log link function(Abarca et al., 2018). Finally, to analyze larval mass we implemented a gamma distribution with an inverse function, as mass data exhibited this distribution. We performed all analyses in R version 3.4.3 (R Core, 2017), using package lme4 (Bates et al., 2015). For model validation of GLM and GLMM, we visually inspected residuals and tested the significance of predictors using type II deviance test, implemented in the Anova function of R package car (Fox and Weisberg, 2011).
Summer Heatwave Experiments
To assess the effects of summer heatwaves we performed three assays, each for a different ontogenetic stage: eggs, active larvae (2nd instar) and dormant larvae (4th instar).We implemented a typical summer temperature regime in the southeastern range of 26 ± 5°C (mean ± amplitude), which was maintained for control individuals and interrupted by a 3-day heatwave with either a large 31 ± 10°C or a small 36 ± 5°C amplitude, resulting in three treatments: control (26 ± 5°C), low (31 ± 10°C) and high (36 ± 5°C). Note that the maximum temperature of both heatwave treatments was 41°C (Figure 2).
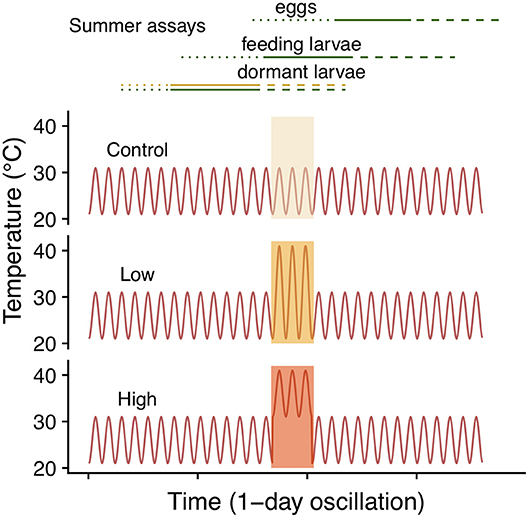
Figure 2. Summer heatwave temperature regimes. Lines in the top panel correspond to the different sets of clutches included in each of the three summer assays. Lines designate eggs (dotted), feeding (solid) and dormant (dashed) larvae. Colors indicate host, green for C. glabra and yellow for (P. lanceolata), only the dormant larvae assay included both hosts.
To assess the effect of heatwave exposure on eggs, we evenly split six clutches, from six different wild-caught females (egg mass size varied from 180 to 300 eggs) and assigned siblings to each of the three treatments. Eggs were laid in an oviposition cage in the field (Cape Cod MA, early July, 2018) and transferred to laboratory control conditions 1 day after oviposition. Exposure to heatwave treatments (control, low, high) occurred 4 days later (5-day old eggs). After heatwave exposure, all clutches were maintained on C. glabra under control (26 ± 5) conditions until the end of August. We recorded hatching success (proportion of eggs that hatched), egg and larval development time (to hatch and to 4th instar, respectively) and survival to the onset of dormancy (4th instar). We assessed the effects of temperature regime on egg and larval development time and survival to dormancy onset using GLMMs. We compared development time among temperature regimes using two models, one from oviposition to eclosion and one from hatching to the onset of dormancy (4th instar), both using a Poisson error distribution (for the count of number of days needed) and log link function (Table 1). We used a binomial error distribution and logit link function in modeling survival to the onset of dormancy. In all models we included temperature regime as a fixed factor and clutch as a random factor.
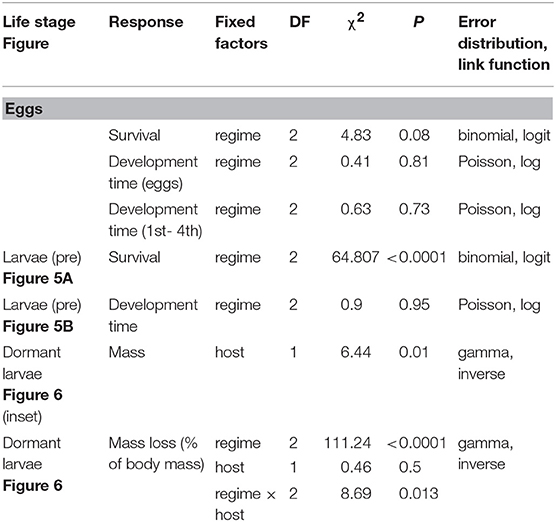
Table 1. GLMM summaries for effects of heatwave exposure during summer on survival, development time and mass of E. phaeton at different ontogenetic stages: eggs, pre-overwintering (pre), and dormant larvae. All models included clutch as a random intercept.
To assess the effects of summer heatwaves on active, pre-overwintering larvae, we followed a similar approach. We split five clutches from five different wild females into three groups, one for each summer temperature regime; we maintained neonates on C. glabra and exposed 7-day old larvae (2nd instar) to the corresponding regime (control, low, high). We recorded development time and survival to the onset of dormancy (4th instar). We used GLMM analogous to those described above to compare survival and development time among temperature regimes (see Table 1).
Finally, to test whether exposure of dormant larvae to summer heatwaves results in mass loss, we weighed 4th instar larvae from each of four clutches before and after exposure to each of the three summer treatments (control, low and high). Larvae included in this assay had fed on either C. glabra (2 clutches) or P. lanceolata (2 clutches). We split groups of siblings among the three treatments for an initial sample size of 240; however, a group from one of the clutches was lost to causes independent from experimental treatments, leaving a final sample size of 220. We kept caterpillars from each sibling group together because larvae are gregarious in nature; our observations in the lab showed that when separated and maintained individually, they engage in abnormal behaviors which could result in mass loss, such as excessive movement and silking. While keeping larvae together was necessary to reduce stress, it prevented the identification of individual caterpillars. Thus, to calculate mass loss, we ranked larvae by weight and assumed the rankings were consistent before and after treatment (that is, the heaviest caterpillar before the heatwave would also be the heaviest individual after the heatwave). We compared percent mass loss among treatments with a GLMM including clutch as a random effect and temperature regime, host, and their interaction as fixed effects, using a gamma error distribution (inverse link function; see Table 1). We built a similar model to compare initial mass among caterpillars from different hosts (C. glabra vs. P. lanceolata), including host as explanatory variable and initial mass as the response variable (Table 1).
Winter Heatwave Experiment
To evaluate the effect of winter heatwaves on E. p. phaeton survival, we subjected dormant larvae (4th instar) to each of three winter regimes: cold (0 ± 5°C), mild (4 ± 5°C), or heatwaves (4 ± 5°C interrupted every 10 days by a two-day period at 15 ± 5°C; Figure 3). For this assay we used groups of siblings (N = 12 groups), and groups of larvae from multiple clutches (N = 5 groups). These 17 groups of larvae did not participate in any other assays, and were raised under uniform laboratory conditions feeding on either P. lanceolata (4 groups) or C. glabra (13 groups) during their whole pre- overwintering stage (instars 1 to 4). We kept all larvae at realistic temperatures for a gradual transition from fall to winter conditions. Total sample size by regime was: cold: N = 714; mild: N = 719; heatwaves: N = 719 larvae. Exposure to these winter temperature regimes started on December 15th 2017 and ended on March 16th 2018, when all larvae were supplied with P. lanceolata foliage and exposed to uniform spring conditions (Figure 3). We counted the number of individuals who survived to spring (were alive and moving on March 19th) and who initiated feeding and growing (had fed and were still alive on March 28th). We compared survival among treatments as a Bernoulli trial (binomial error distribution, logit link function) within a GLM for each date in which survival was assessed (see Table 2).
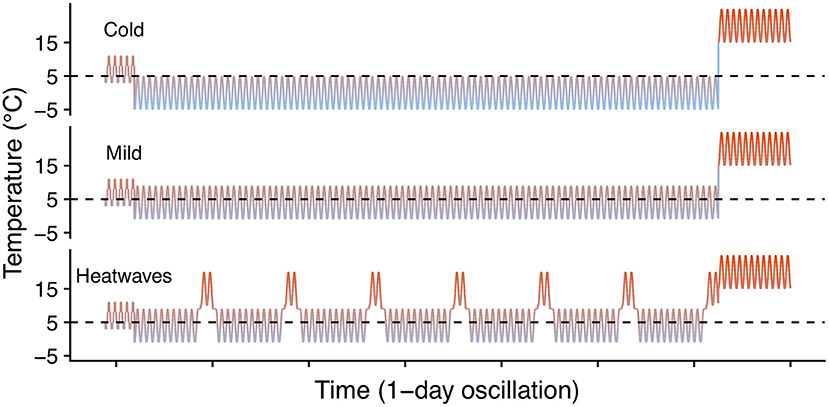
Figure 3. Winter heatwave temperature regimes. Dashed lines indicate 5°C to facilitate visual comparison among regimes.
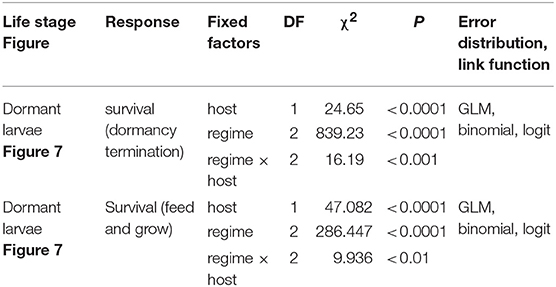
Table 2. GLM summaries for effects of heatwave exposure during winter on survival of pre-overwintering larvae.
Host Plant and Thermal Stress
To test whether host plant choice can affect E. p. phaeton performance under thermal stress, we exposed pre-overwintering larvae to stressfully low temperatures while feeding on either P. lanceolata or C. glabra following a fully factorial design. We split each of five laboratory- reared egg masses of at least 200 viable eggs into groups of 50 hatchlings and assigned them to the four treatments resulting from the combination of two hosts (C. glabra, P. lanceolata) and two spring temperature regimes (cold vs. warm spells): All individuals were kept at a base regime of 20 ± 5°C and were exposed to either warm (4 days at 22.5 ± 2.5°C) or cold spells (4 days at 12.5 ± 2.5°C) twice during larval development (Figure 4). Upon the onset of dormancy (molt to 4th instar), we transferred all larvae to 26 ± 5°C and weighed them using a balance (Mettler Toledo MX5). We recorded development time and survival to the end of August (2 months after reaching 4th instar). We compared development time and survival among treatments using GLMMs as described above (see Table 3 for error distributions and link functions). While pre-overwintering larvae are not likely to experience temperatures as low as our regimes, this assay allowed us to both assess the role of host plant at mitigating thermal stress, and to obtain groups of caterpillars of variable size (body mass). Having a gradient of larval body sizes allowed us to test whether dormant caterpillar mass affects the likelihood of survival to the end of summer. To do this, we performed a logistic regression including the mean mass per group (N = 16 groups) as independent variable and survival to the end of August (survivors/total) as the dependent variable (Table 3).
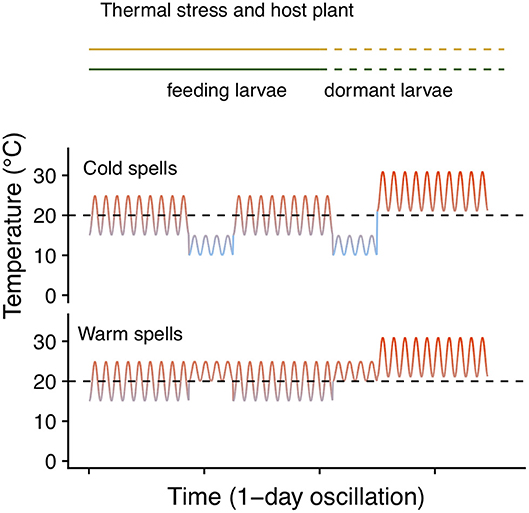
Figure 4. Temperature regimes of the thermal stress and host plant assay. Lines in the top indicate the timing at which feeding (solid) and dormant (dashed) larvae experienced each regime. Dashed black lines indicate 20°C to facilitate comparison.
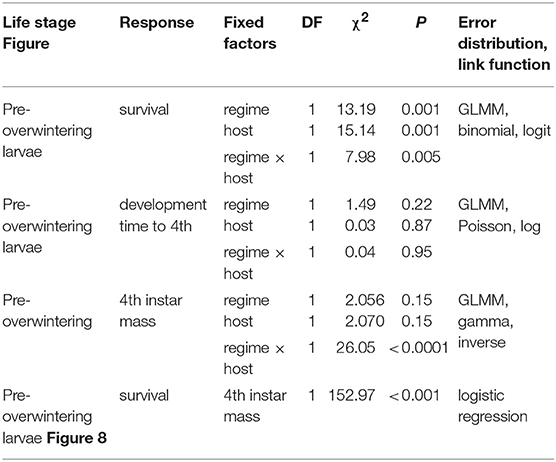
Table 3. GLMM and logistic regression summaries for the combined effects of cold stress and host plant on survival and development time of pre-overwintering larvae.
Historical Temperature Analysis
To determine whether heatwave incidence and intensity have recently increased within E. p. phaeton's range, we gathered daily temperature data from two weather stations, one in the northeastern part of the range (located at Brockton, MA: 42.04756 N,−71.00819W) and one in the southeastern part of the range (located on the eastern shore at Chestertown, MD: 39.2166N,−76.0519W). We selected these weather stations because they had the longest periods recorded (1894 to 2011) within the regions of interest. We defined heatwave after Russo et al. (2014), as three or more consecutive days with a maximum temperature above the 90th percentile of a reference period. To set the reference periods we divided each season (spring, summer, autumn, winter) into 6 sub-seasons of 15 or 16 days and calculated the 90th percentile value for location and sub-season using historical data (1894 to 1913). We subsequently used these reference values to identify heatwaves and quantify their yearly incidence. To test whether heatwave incidence (number of heatwaves), intensity (maximum temperature during a heatwave) and duration (mean heatwave length by season) have changed over time, we performed two sets of GLMs: one per region, for each response variable (incidence, intensity, and duration) including year and season as predictors (Table 4). We used a Poisson distribution (log link function) to analyze counts of heatwave incidence and a Gamma distribution (inverse link function) for heatwave intensity. To analyze heatwave duration, we used a quasipoisson distribution (log link function) because mean heatwave length is the average of multiple day counts, so it was not discrete.
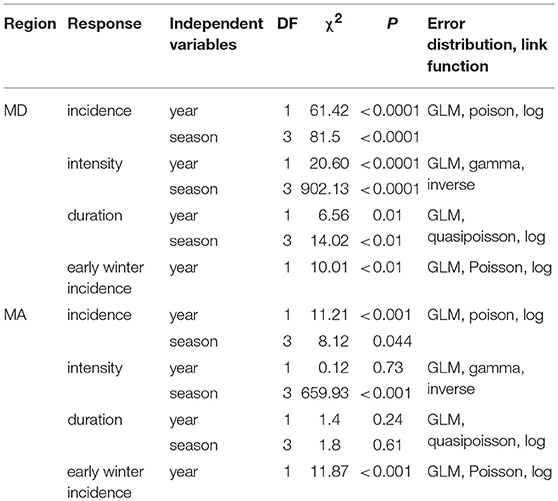
Table 4. GLM summaries for the analysis of heatwave incidence, intensity and duration in MD and MA during the period of 1894 to 2011.
We found that winter warm spells, characterized by maximum temperatures of 20°C resulted in severe mortality of E. p. phaeton larvae in the laboratory. Thus, we separately assessed the incidence of heatwaves during the first part of winter (excluding most of March), hereafter “early winter.” This period reflects when host plant foliage is less likely to be available. For this analysis, we counted the heatwaves occurring from day 353 (December 21st) of each year to day 63 (March 4th) of the following year. To assess whether “early winter” heatwave frequency has increased over time we performed two GLMs, one per region, including year as predictor (Table 4).
Results
Summer Heatwave Experiments
Exposure of eggs to summer heatwaves of a maximum temperature of 41°C did not have a significant effect on survival to the onset of dormancy (Table 1), or development time to both hatching and the onset of dormancy (Table 1). More than 90% of the eggs hatched, and about 50% of individuals survived to the onset of dormancy (4th instar) regardless of experimental treatment. Eggs from all temperature regimes hatched about 14 days after being laid (14 [median], 1 [interquartile interval]) and larvae entered dormancy about a month after oviposition (30, 3). By contrast, exposure of 2nd instar larvae (7-day old) to the high heatwave regime (36 ± 5°C) resulted in a 37% decrease in survivorship when compared to those under control and low heatwave conditions (Table 1, Figure 5A); however, there were no significant differences in development time (Table 1, Figure 5B) as larvae from all summer regimes entered dormancy about 17 days after hatching.
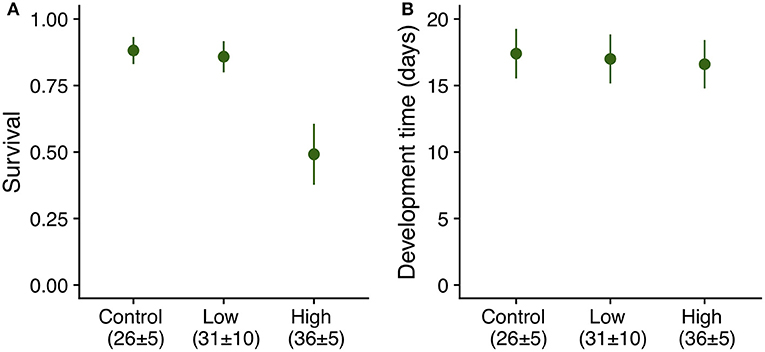
Figure 5. (A) Survival (LS means ± SE) and (B) development time (LS means ± SE) from hatching to 4th instar of E. phaeton individuals exposed to summer heatwave regimes as 2nd instar (7-day old) larvae. There were significant differences among treatments in survival, but not in development time (Table 1).
Larvae fed on the native C. glabra were 12% larger (7.26 mg [median], 1.52 [interquartile interval]) at the onset of dormancy than those fed on P. lanceolata (Figure 6). Furthermore, host plant mediated the effects of heatwave exposure on dormant 4th instar larvae. Individuals maintained under control conditions lost about 3% of their body mass regardless of host plant. However; under heatwave conditions, larvae feeding on P. lanceolata lost up to 6% of their mass, and only 3% when feeding on C. glabra (Table 1, Figure 6).
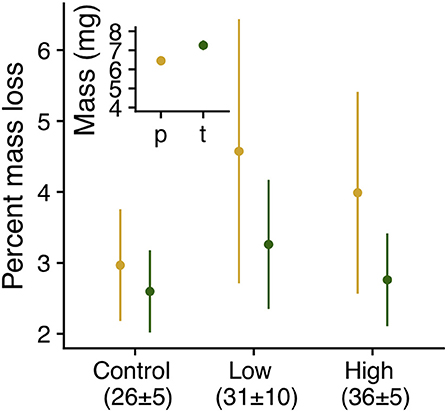
Figure 6. Mass (inset, LS means ± SE , χ2 = 6.44, P = 0.01) and percent mass lost (interaction: χ2 = 8.69, P = 0.013, host: χ2 = 0.46, P = 0.5, regime: χ2 = 111.23, P < 0.0001) by dormant E. phaeton larvae during exposure to summer heatwaves. Larvae had previously fed on C. glabra (c, green) or P. lanceolata (p, yellow).
Winter Heatwave Experiment
Exposure to winter heatwaves resulted in severe mortality, as < 15% of larvae subjected to this treatment survived to resume activity the following spring (Figure 7, Table 2). By contrast individuals exposed to the cold treatment had much higher survivorship to both resuming activity the following spring (March 19th, 87%) and to feed and grow (March 28th, 50%). Individuals in the mild winter treatment exhibited intermediate survivorship (~ 40% and 25%). Notably, the likelihood of winter survival was influenced by host plant use during the pre-overwintering period; the probability of survival was consistently larger for larvae that had fed on C. glabra (Table 2, Figure 7). No larvae that had fed on P. lanceolata during the pre-overwintering period and were exposed to winter heatwaves survived.
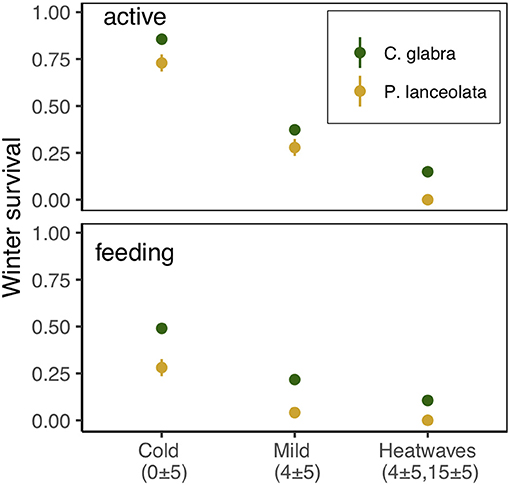
Figure 7. Survival of dormant E. phaeton larvae exposed to cold, mild, or heatwave winter regimes at two different times, March 19th, 2018 (active) and March 28th, 2018 (feeding).
Host Plant and Thermal Stress
We found no effect of temperature regime, host, or their interaction on development time of pre-overwintering larvae (1st to 4th instar), as it took them 18 days (median; interquartile range = 2) to reach the dormancy stage regardless of host (Table 3). By contrast, all three factors significantly affected caterpillar survival to the end of summer (Table 3), as caterpillars feeding on C. glabra and experiencing warm spells had larger survival odds (75%) than those in all other host and temperature combinations (50%; Table 3).
Analyses of pre-overwintering dormant larval mass showed that for larvae experiencing cold spells, those feeding on C. glabra were 20% larger than those feeding on P. lanceolata at the end of summer (a significant effect of the host by temperature regime interaction, but not of each factor separately; Table 3). Finally, there was a significant relationship between larval mass and summer survival, as colonies that had larger mean larval mass also had a higher proportion of survivors, Table 3, Figure 8).
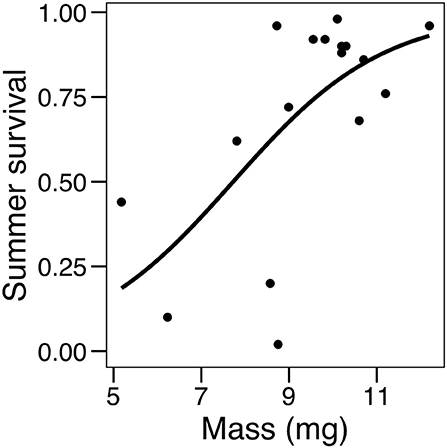
Figure 8. Logistic regression showing the effect of mean larval mass on summer survival (proportion of dormant larvae surviving to the end of August).
Historical Temperature Analysis
We found that the incidence of heatwaves has increased since 1894 in both MA and MD (Figure 9, Table 4). Heatwave intensity (Figure 10, Table 4) and duration (Table 4) exhibited a significant increase in MD only. In Maryland, heatwave intensity (maximum temperature during a heatwave) during summer varied between 30 to 40°C and during winter between 13.9 and 30.6°C. In MA ranges were 30 to 40°C in summer and 8.3 to 28.9°C in winter. Most heatwaves had a duration of 3 to 5 days in both regions. When early winter (excluding most of March) was considered separately, annual incidence of heatwaves during the first part of winter was consistently under 4 per winter in MA, but in MD increased from 2 in the 1890's to 5 in recent years (Table 4).
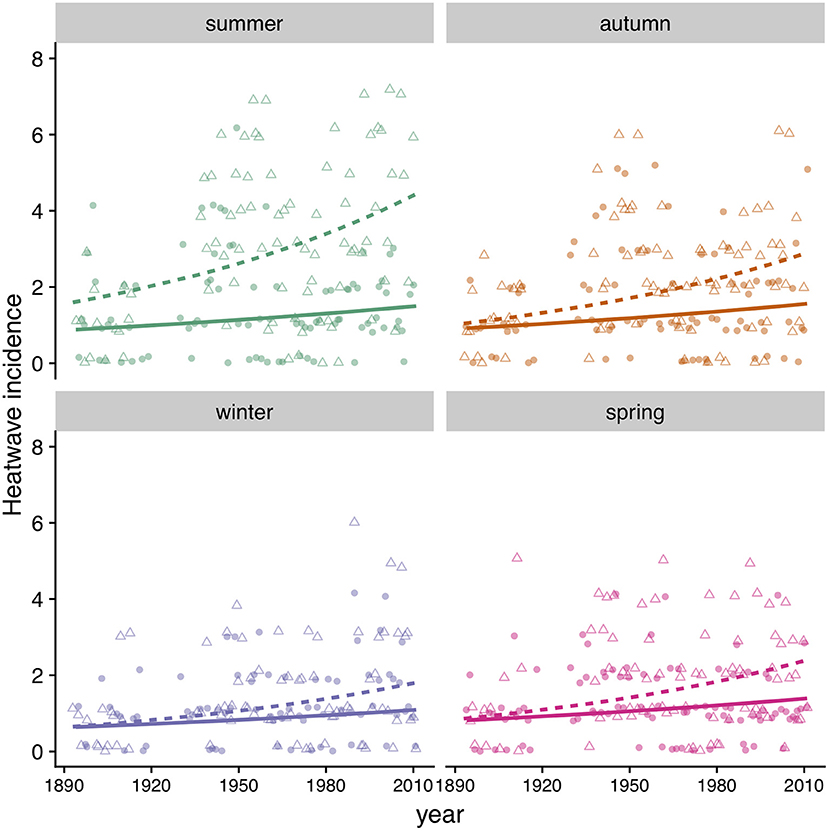
Figure 9. Heatwave incidence (count) by season in MD (triangles, dashed line) and MA (circles, solid line). Symbols correspond to data points and lines to model predictions (Table 4).
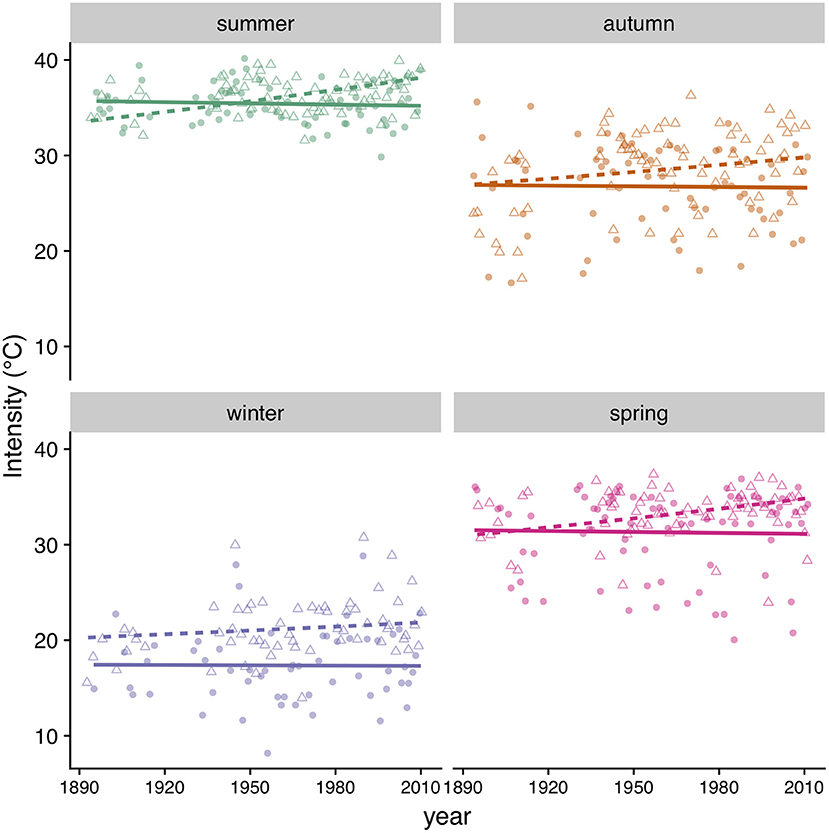
Figure 10. Heatwave intensity (maximum temperature) by season in MD (triangles, dashed line) and MA (circles, solid line). Symbols correspond to data points and lines to model predictions (Table 4).
Discussion
This study identifies winter heatwaves as a potential factor contributing to the decline of southeastern populations of E. p. phaeton. Winter heatwaves resulted in severe larval mortality in the laboratory and historical temperature records show an increment in the incidence, intensity and duration of these events within the southeastern range of E. p. phaeton, where population declines have been observed (Durkin, 2009; Frye et al., 2013). Thus, we conclude that climate change, and more specifically, winter heatwaves, should be considered a serious threat to E. p. phaeton populations. Our results are consistent with predictions that increased temperature variation in mid-latitudes would negatively impact insects inhabiting these regions (Kingsolver et al., 2013). Careful monitoring of overwintering E. p. phaeton populations would be necessary to attribute demographic changes to specific heatwave events in the wild.
We found dormant larvae (4th instar) to be particularly vulnerable to heatwaves. These larvae rely on biomass accumulated during early instars to persist for a period of over 7 months in which they experience a broad range of thermal conditions. Exposure of dormant larvae to summer heatwaves resulted in mass loss (Figure 6), which can increase pre-winter mortality, as the odds of larval survival to the end of summer decreased with size (Figure 8). Hence, summer heatwaves can result in fewer and smaller larvae surviving until the onset of winter. Because biomass is an important predictor of larval overwinter success (Smith, 2002), summer heatwaves can also result in increased larval susceptibility to unfavorable winter conditions. Furthermore, winter heatwaves drastically reduced survival of dormant caterpillars (Figure 7). Dormant larvae exposed to warm temperatures during winter activate and move around; thus, mortality was probably due to energy reserve depletion resulting from increased metabolic activity. This phenomenon has been observed in multiple taxa including vertebrates (Williams et al., 2015) and gallflies (Irwin and Lee, 2003). By contrast, eggs (which are only present during summer) showed no negative effects on survival or phenology after exposure to summer heatwaves. The egg stage of E. phaeton has a long duration compared to other summer butterflies whose eggs often hatch after 2 to 10 days (James and Nunnallee, 2011); for example, eggs of Epargyreus clarus hatch after ~ 5 days in summer temperature regimes (Abarca et al., 2018) and Papilio polyxenes eggs hatch after ~ 6 days (Blau, 1981) and it is also more resilient to heatwaves than other lepidopterans; exposure to heatwaves resulted in increased egg mortality in both Ostrinia furnacalis (Zhou et al., 2018) and Manduca sexta (Potter et al., 2011). Pre-overwintering larvae were somewhat resilient to heatwaves, as neither of the regimes (low, high) affected their phenology (Figure 5). However, the high regime (36 ± 5 °C) did result in reduced survival. This regime was characterized by a relatively high minimum temperature of 31°C, which is not characteristic of the MD area, where minimum summer heatwave temperatures range within 21°C and 27°C. Minimum temperatures during heatwaves have been found to have larger effects on mortality than maximum temperatures (Hajat et al., 2002). Note that while summer heatwaves had little to no effect on the survival and phenology of E. p. phaeton eggs and early-instar larvae; we cannot discard the occurrence of sublethal effects, such as reduced fertility (Addo-bediako et al., 2000; Zhang et al., 2015a) and immune function (Chown, 2001) which have been reported to occur in response to heatwaves.
The winter temperature regimes we implemented were conservative compared to actual winter temperatures recorded in the MD area. In the laboratory, we exposed larvae to a heatwave regime with a maximum temperature of 20°C. Maximum winter heatwave temperatures commonly exceed this threshold in MD, but not in MA (Figure 9). Thus, the winter conditions MD caterpillars currently experience in the wild are likely to be harsher than our heatwave regime. Current E. p. phaeton populations in MD are limited to the northern Piedmont, west to the Appalachian Plateau (Pers. Comm. Jen Selfridge) where temperatures are presumably lower than the Chestertown weather station used in this study, which is more representative of Coastal Plain, valleys and urban areas. This distribution pattern is consistent with what would be expected under a scenario in which winter heatwaves are a main mortality source in the wild. Further, niche models identify temperature as a main driver of this species distribution (Czachura et al., in preparation) and climate change as a potential threat (Frye et al., 2013). Given the imperiled status of E. p. phaeton in MD, we used individuals from a MA population in our experiments. This constitutes a potential limitation of our study, as MD populations could be more resilient to heatwaves than those in MA due to local adaptation. However, we argue that it is unlikely for MD populations to be robust to winter heatwaves because maximum thermal physiological limits are well conserved within taxa (Chown, 2001). In addition, the heatwave effects we found on survival were so dramatic (survival of < 15 %), that even if twice as many MD individuals survived irregular winters, populations would still experience severe losses. Future experiments would elucidate whether northern and southern populations of E. p. phaeton significantly differ in their thermal thresholds and susceptibility to winter heatwaves.
We found that host plant had an important role at mediating the effects of thermal stress. Larvae feeding on C. glabra attained larger sizes, were less vulnerable to mass loss during summer heatwaves and, more importantly, all survivors under the winter heatwave regime had fed on C. glabra. This is consistent with previous work showing that host plant identity (Diamond and Kingsolver, 2010; Abarca et al., 2018) and nutrient content (Andersen et al., 2009), can mediate insect responses to thermal stress; and that resource acquisition before overwintering significantly affects survival (Pullin, 1987). For example, Vallières et al. (2015) showed that both population of origin and host plant species mediated the effect of fall heatwaves on the survival of the hemlock looper (Lambdina fiscellaria).Thus, the costs of feeding on suboptimal hosts are increased under thermal stress, which may be the reason why P. lanceolata has not been adopted as a primary host (oviposition) by E. p. phaeton populations in MD, where pre-overwintering larvae have been reported to feed exclusively on C. glabra. Future studies would investigate this possibility. Additionally, heatwave incidence and intensity are likely to increase throughout E. p. phaeton's range, and the reliance of northern populations on the more widespread novel host, P. lanceolata, may become maladaptive under future thermal regimes. Generalist herbivores often use hosts according to their relative abundance and not their nutritional value (Mason et al., 2011); the intersection of limited C. glabra availability and climate change imposes a significant threat to E. p. phaeton's long-term persistence. Finally, it is important to note that warmer temperatures can reduce host quality (Bauerfeind and Fischer, 2013), which is a potential effect of summer heatwaves that we did not investigate but that can further imperil not only E. p. phaeton but other arthropod populations.
We showed that winter heatwaves, characterized by temperatures well under physiological limits can have more devastating effects than summer heatwaves characterized by maximum temperatures that are physiologically stressful. Our results are consistent with others showing that irregular winter patterns are detrimental (Bale and Hayward, 2010), particularly for species that overwinter as larvae (Williams et al., 2012). Populations of E. p. phaeton in MD face multiple threats such as habitat destruction, urbanization and host plant (C. glabra) scarcity (Frye et al., 2013) that can be mitigated through conservation efforts. We recommend those efforts to consider local thermal regimes and be concentrated in relatively cool areas, were winter heatwaves are less likely to result in severe overwinter mortality.
Data Availability
All datasets generated for this study are included in the Supplementary Files as Data Sheets 1–7.
Author Contributions
MA, LR, and EL conceived and designed the experiments. LR and MA collected colony founders. MA and EL carried out the experiments and analyzed data. MA wrote the manuscript with input and edits from EL and LR.
Funding
Funding for this project was generously provided by Georgetown University.
Conflict of Interest Statement
The authors declare that the research was conducted in the absence of any commercial or financial relationships that could be construed as a potential conflict of interest.
Acknowledgments
We thank Greg Breed for his help developing E. phaeton rearing techniques and Douglas Taron for advice on caterpillar husbandry. We thank Madeline Lee & Declan Mirabella for their assistance in the laboratory and with data management. We also thank Jennifer Selfridge (former J Frye) for her kind support and information on the current status of E. p. phaeton populations in MD.
Supplementary Material
The Supplementary Material for this article can be found online at: https://www.frontiersin.org/articles/10.3389/fevo.2019.00193/full#supplementary-material
Data Sheet 1. Historical temperature records.
Data Sheet 2. Summer heatwave experiments: eggs.
Data Sheet 3. Summer heatwave experiments: feeding larvae.
Data Sheet 4. Summer heatwave experiments: dormant larvae.
Data Sheet 5. Winter heatwave: dormant larvae.
Data Sheet 6. Host plant and thermal stress: phenology and survival.
Data Sheet 7. Host plant and thermal stress: caterpillar mass.
References
Abarca, M., Larsen, E. A., Lill, J. T., Weiss, M., Lind, E., and Ries, L. (2018). Inclusion of host quality data improves predictions of herbivore phenology. Entomol. Exp. Appl. 8, 1–13. doi: 10.1111/eea.12715
Addo-bediako, A., Chown, S. L., and Gaston, K. J. (2000). Thermal tolerance, climatic variability and latitude. Proc. R. Soc. B Biol. Sci. 267, 739–745. doi: 10.1098/rspb.2000.1065
Andersen, L. H., Kristensen, T. N., Loeschcke, V., Toft, S., and Mayntz, D. (2009). Protein and carbohydrate composition of larval food affects tolerance to thermal stress and desiccation in adult Drosophila melanogaster. J. Insect Physiol. 56, 336–340. doi: 10.1016/j.jinsphys.2009.11.006
Augspurger, C. K. (2013). Reconstructing patterns of temperature, phenology, and frost damage over 124 years: Spring damage risk is increasing. Ecology 94, 41–50. doi: 10.1890/12-0200.1
Awmack, C. S., and Leather, S. R. (2002). Host Plant Quality and Fecundity in Herbivorous Insects. Annu. Rev. Entomol. 47, 817–844. doi: 10.1146/annurev.ento.47.091201.145300
Bale, J. S., and Hayward, S. A. L. (2010). Insect overwintering in a changing climate. J. Exp. Biol. 213, 980–994. doi: 10.1242/jeb.037911
Batalden, R. V., and Oberhauser, K. S. (2015). “Potential changes in eastern North American monarch migration in response to an introduced milkweed, Asclepias curassavica,” in Monarchs in a Changing World: Biology and Conservation of an Iconic Insect, eds. K. S. Oberhauser, K. R. Nail, and S. Altizer (Ithaca, New York: Cornell University press), 215–222.
Bates, D., Mächler, M., Bolker, B., and Walker, S. (2015). Fitting linear mixed-effects models using lme4. J. Stat. Softw. 67, 1–48. doi: 10.18637/jss.v067.i01
Bauerfeind, S. S., and Fischer, K. (2013). Increased temperature reduces herbivore host-plant quality. Glob. Change Biol. 19, 3272–3282. doi: 10.1111/gcb.12297
Blau, W. S. (1981). Life history variation in the black swallowtail butterfly. Oecologia 48, 116–122. doi: 10.1007/BF00346997
Bowers, M. D. (1980). Unpalatability as a defense strategy of Euphydryas phaeton (Lepidoptera: Nymphalidae). Evolution 34, 586–600. doi: 10.1111/j.1558-5646.1980.tb04846.x
Bowers, M. D., and Richardson, L. L. (2013). Use of two oviposition plants in populations of Euphydryas phaeton Drury (Nymphalidae). J. Lepid. Soc. 67, 299–300. doi: 10.18473/lepi.v67i4.a7
Bowers, M. D., Stamp, N. E., and Collinge, S. K. (1992). Early stage of host range expansion by a specialist herbivore, euphydryas phaeton (Nymphalidae). Ecology 73, 526–536. doi: 10.2307/1940758
Cahill, A. E., Aiello-Lammens, M. E., Fisher-Reid, M. C., Hua, X., et al. (2012). How does climate change cause extinction? Proc. R. Soc. B Biol. Sci. 280, 20121890–20121890. doi: 10.1098/rspb.2012.1890
Cavers, P. B., Bassett, I. J., and Crompton, C. W (1980). The biology of canadian weeds. 47. plantago lanceolata L. Can. J. Plant Sci. 60, 1269–1282. doi: 10.4141/cjps80-180
Chown, S. L. (2001). Physiological variation in insects: hierarchical levels and implications. J. Insect Physiol. 47, 649–660. doi: 10.1016/S0022-1910(00)00163-3
Christidis, N., Jones, G. S., and Stott, P. A. (2015). Dramatically increasing chance of extremely hot summers since the 2003 European heatwave. Nat. Clim. Chang. 5, 46–50. doi: 10.1038/nclimate2468
Diamond, S. E., and Kingsolver, J. G. (2010). Environmental dependence of thermal reaction norms : host plant quality can reverse the temperature-size rule. Am. Nat. 175, 1–10. doi: 10.1086/648602
Dirzo, R., Young, H. S., Galetti, M., Ceballos, G., Isaac, N. J. B., and Collen, B. (2014). Defaunation in the Anthropocene. Science 345, 401–406. doi: 10.1126/science.1251817
Durkin, P. M. (2009). Efforts to restore the baltimore checkerspot (Euphydryas phaeton) in Maryland. News Lepid. Soc. 51, 3–5.
Fischer, K., Klockmann, M., and Reim, E. (2014). Strong negative effects of simulated heat waves in a tropical butterfly. J. Exp. Biol. 217, 2892–2898. doi: 10.1242/jeb.106245
Fox, J., and Weisberg, S. (2011). An R companion to Applied Regression. Thousand Oaks, CA: Sage Available online at: http://socserv.socsci.mcmaster.ca/jfox/Books/Companion
Frank, D., Reichstein, M., Bahn, M., Thonicke, K., Frank, D., Mahecha, M. D., et al. (2015). Effects of climate extremes on the terrestrial carbon cycle: Concepts, processes and potential future impacts. Glob. Chang. Biol. 21, 2861–2880. doi: 10.1111/gcb.12916
Frye, J., Durkin, P., Gibbs, D., Gibbs, R., and Lustig, M. (2013). Conservation and Management of the Baltimore Checkerspot (Euphydryas phaeton Drury) in Maryland: Strategies for Statewide Monitoring and for Wetland Restoration, Captive Breeding and Release in the Piedmont Region. Annapolis.
Gordo, O., and Sanz, J. J. (2005). Phenology and climate change: a long-term study in a Mediterranean locality. Oecologia 146, 484–495. doi: 10.1007/s00442-005-0240-z
Hajat, S., Kovats, R. S., Atkinson, R. W., and Haines, A. (2002). Impact of hot temperatures on death in London: A time series approach. J. Epidemiol. Commun. Health 56, 367–372. doi: 10.1136/jech.56.5.367
Hallmann, C. A., Sorg, M., Jongejans, E., Siepel, H., Hofland, N., Schwan, H., et al. (2017). More than 75 percent decline over 27 years in total flying insect biomass in protected areas. PLoS ONE 12, 1–21. doi: 10.1371/journal.pone.0185809
Hansen, J., Sato, M., and Reto, R. (2012). Perception of climate change. Proc. Natl. Acad. Sci. 109, E2415–E2423. doi: 10.1073/pnas.1205276109
Irwin, J. T., and Lee, R. E. (2003). Cold winter microenvironments conserve energy and improve overwintering survival and potential fecundity of the goldenrod gall fly, Eurosta solidaginis. Oikos 100, 71–78. doi: 10.1034/j.1600-0706.2003.11738.x
James, D. G., and Nunnallee, D. (2011). Life Histories of Cascadia Butterflies. 1st ed. Corvallis, OR: Oregon State University Press.
Kingsolver, J. G., Diamond, S. E., and Buckley, L. B. (2013). Heat stress and the fitness consequences of climate change for terrestrial ectotherms. Funct. Ecol. 27, 1415–1423. doi: 10.1111/1365-2435.12145
Kudo, G., and Ida, T. Y. (2013). Early onset of spring increases the phenological mismatch between plants and pollinators. Ecology 94, 2311–2320. doi: 10.1890/12-2003.1
Levy, O., Buckley, L. B., Keitt, T. H., Smith, C. D., Boateng, K. O., Kumar, D. S., et al. (2015). Resolving the life cycle alters expected impacts of climate change. Proc. R. Soc. B 282, 1813. doi: 10.1098/rspb.2015.0837
Lister, B. C., and Garcia, A. (2018). Climate-driven declines in arthropod abundance restructure a rainforest food web. Proc. Natl. Acad. Sci. U.S.A. 115, E10397–E10406. doi: 10.1073/pnas.1722477115
Mason, P. A., Wilkes, S. R., Lill, J. T., and Singer, M. S. (2011). Abundance trumps quality: Bi-trophic performance and parasitism risk fail to explain host use in the fall webworm. Oikos 120, 1509–1518. doi: 10.1111/j.1600-0706.2011.19053.x
Meehl, G. A., and Tebaldi, C. (2004). More intense, more frequent, and longer lasting heat waves in the 21st century. Science 305, 994–997. doi: 10.1126/science.1098704
Moran, E. V., and Alexander, J. M. (2014). Evolutionary responses to global change: lessons from invasive species. Ecol. Lett. 17, 637–649. doi: 10.1111/ele.12262
Ovaskainen, O., Skorokhodova, S., Yakovleva, M., Sukhov, A., Kutenkov, A., Kutenkova, N., et al. (2013). Community-level phenological response to climate change. Proc. Natl. Acad. Sci. U. S. A. 110, 13434–13439. doi: 10.1073/pnas.1305533110
Parmesan, C. (2006). Ecological and evolutionary responses to recent climate change. Annu. Rev. Ecol. Evol. Syst. 37, 637–669. doi: 10.1146/annurev.ecolsys.37.091305.110100
Parmesan, C. (2007). Influences of species, latitudes and methodologies on estimates of phenological response to global warming. Glob. Chang. Biol. 13, 1860–1872. doi: 10.1111/j.1365-2486.2007.01404.x
Parmesan, C., and Yohe, G. (2003). A globally coherent fingerprint of climate change impacts across natural systems. Nature 421, 37–42. doi: 10.1038/nature01286
Perkins, S. E., Alexander, L. V., and Nairn, J. R. (2012). Increasing frequency, intensity and duration of observed global heatwaves and warm spells. Geophys. Res. Lett. 39, 1–5. doi: 10.1029/2012GL053361
Potter, K. A., Davidowitz, G., and Arthur Woods, H. (2011). Cross-stage consequences of egg temperature in the insect Manduca sexta. Funct. Ecol. 25, 548–556. doi: 10.1111/j.1365-2435.2010.01807.x
Pullin, A. S. (1987). Adult feeding time, lipid accumulation, and overwintering in Aglais urticae and Inachis io (Lepidoptera: Nymphalidae). J. Zool. 211, 631–641. doi: 10.1111/j.1469-7998.1987.tb04476.x
R Core, T. (2017). R: A Language and Environment for Statistical Computing. Vienna: R Foundation for Statistical Computing.
Robertson, S. M. (2015). Life history, oviposition characteristics, and management of the Ozark Baltimore Checkerspot (Euphydryas phaeton ozarke).
Rosenwald, L. C., Lill, J. T., Lind, E. M., and Weiss, M. R. (2017). Dynamics of host plant selection and host-switching by silver-spotted skipper caterpillars. Arthropod. Plant. Interact. 11, 833–842. doi: 10.1007/s11829-017-9538-0
Russo, S., Dosio, A., Graversen, R. G., Sillmann, J., Carrao, H., Dunbar, M. B., et al. (2014). Magnitude of extreme heat waves in present climate and their projection in a warming world. J. Geophys. Res. 119, 12500–12512. doi: 10.1002/2014JD022098
Sales, K., Vasudeva, R., Dickinson, M. E., Godwin, J. L., Lumley, A. J., Michalczyk, Ł., et al. (2018). Experimental heatwaves compromise sperm function and cause transgenerational damage in a model insect. Nat. Commun. 9, 1–11. doi: 10.1038/s41467-018-07273-z
Sánchez-Bayo, F., and Wyckhuys, K. A. G. (2019). Worldwide decline of the entomofauna: a review of its drivers. Biol. Conserv. 232, 8–27. doi: 10.1016/j.biocon.2019.01.020
Singer, M. C., and Parmesan, C. (2018). Lethal trap created by adaptive evolutionary response to an exotic resource. Nature 557, 238–241. doi: 10.1038/s41586-018-0074-6
Smith, R. J. (2002). Effect of larval body size on overwinter survival and emerging adult size in the burying beetle, Nicrophorus investigator. Can. J. Zool. 80, 1588–1593. doi: 10.1139/z02-151
Stamp, N. E. (1979). New oviposition plant for Euphydryas phaeton (Nymphalidae). J. Lepid. Soc. 33, 203–204.
Ummenhofer, C. C., and Meehl, G. A. (2017). Extreme weather and climate events with ecological relevance: a review. Philos. Trans. R. Soc. B Biol. Sci. 372. doi: 10.1098/rstb.2016.0135
Vallières, R., Rochefort, S., Berthiaume, R., Hébert, C., and Bauce, É. (2015). Effect of simulated fall heat waves on cold hardiness and winter survival of hemlock looper, Lambdina fiscellaria (Lepidoptera: Geometridae). J. Insect Physiol. 73, 60–69. doi: 10.1016/j.jinsphys.2014.12.001
Williams, C. M., Hellmann, J., and Sinclair, B. J. (2012). Lepidopteran species differ in susceptibility to winter warming. Clim. Res. 53, 119–130. doi: 10.3354/cr01100
Williams, C. M., Henry, H. A. L., and Sinclair, B. J. (2015). Cold truths: How winter drives responses of terrestrial organisms to climate change. Biol. Rev. 90, 214–235. doi: 10.1111/brv.12105
Xu, Z., FitzGerald, G., Guo, Y., Jalaludin, B., and Tong, S. (2016). Impact of heatwave on mortality under different heatwave definitions: a systematic review and meta-analysis. Environ. Int. 89–90, 193–203. doi: 10.1016/j.envint.2016.02.007
Yang, L. H., and Rudolf, V. H. W. (2010). Phenology, ontogeny and the effects of climate change on the timing of species interactions. Ecol. Lett. 13, 1–10. doi: 10.1111/j.1461-0248.2009.01402.x
Zhang, W., Chang, X. Q., Hoffmann, A. A., Zhang, S., and Ma, C. S. (2015a). Impact of hot events at different developmental stages of a moth: the closer to adult stage, the less reproductive output. Sci. Rep. 5, 1–9. doi: 10.1038/srep10436
Zhang, W., Rudolf, V. H. W., and Ma, C. S (2015b). Stage-specific heat effects: timing and duration of heat waves alter demographic rates of a global insect pest. Oecologia 179, 947–957. doi: 10.1007/s00442-015-3409-0
Keywords: novel host, dormancy, climate change, baltimore checkerspot, Euphydryas phaeton, invasive, Plantago lanceolata
Citation: Abarca M, Larsen EA and Ries L (2019) Heatwaves and Novel Host Consumption Increase Overwinter Mortality of an Imperiled Wetland Butterfly. Front. Ecol. Evol. 7:193. doi: 10.3389/fevo.2019.00193
Received: 02 March 2019; Accepted: 13 May 2019;
Published: 04 June 2019.
Edited by:
Lora A. Richards, University of Nevada, Reno, United StatesReviewed by:
Fernando Colchero, University of Southern Denmark, DenmarkShu-Jun Wei, Beijing Academy of Agricultural and Forestry Sciences, China
Copyright © 2019 Abarca, Larsen and Ries. This is an open-access article distributed under the terms of the Creative Commons Attribution License (CC BY). The use, distribution or reproduction in other forums is permitted, provided the original author(s) and the copyright owner(s) are credited and that the original publication in this journal is cited, in accordance with accepted academic practice. No use, distribution or reproduction is permitted which does not comply with these terms.
*Correspondence: Mariana Abarca, bWE3ODJAZ2VvcmdldG93bi5lZHU=