- 1USDA Forest Service, Rocky Mountain Research Station, Reno, NV, United States
- 2U.S. Geological Survey, Western Ecological Research Center, Oakhurst, CA, United States
- 3U.S. Geological Survey, Forest and Rangeland Ecosystem Science Center, Boise, ID, United States
- 4USDA Natural Resources Conservation Service, West National Technology Support Center, Portland, OR, United States
- 5Numerical Terradynamic Simulation Group, University of Montana, Missoula, MT, United States
- 6W.A. Franke College of Forestry and Conservation, University of Montana, Missoula, MT, United States
Plant invasions can affect fuel characteristics, fire behavior, and fire regimes resulting in invasive plant-fire cycles and alternative, self-perpetuating states that can be difficult, if not impossible, to reverse. Concepts related to general resilience to disturbance and resistance to invasive plants provide the basis for managing landscapes to increase their capacity to reorganize and adjust following fire, while concepts related to spatial resilience provide the basis for managing landscapes to conserve resources and habitats and maintain connectivity. New, spatially explicit approaches and decision-tools enable managers to understand and evaluate general and spatial resilience to fire and resistance to invasive grasses across large landscapes in arid and semi-arid shrublands and woodlands. These approaches and tools provide the capacity to locate management actions strategically to prevent development of invasive grass-fire cycles and maintain or improve resources and habitats. In this review, we discuss the factors that influence fire regimes, general and spatial resilience to fire, resistance to invasive annual grasses, and thus invasive grass-fire cycles in global arid and semi-arid shrublands and woodlands. The Cold Deserts, Mediterranean Ecoregion, and Warm Deserts of North America are used as model systems to describe how and why resilience to disturbance and resistance to invasive annuals differ over large landscapes. The Cold Deserts are used to illustrate an approach and decision tools for prioritizing areas on the landscape for management actions to prevent development of invasive grass-fire cycles and protect high value resources and habitats and for determining effective management strategies. The concepts and approach herein represent a paradigm shift in the management of these ecosystems, which allows managers to use geospatial tools to identify resilience to disturbance and resistance to invasive plants in order to target conservation and restoration actions where they will provide the greatest benefits.
Introduction
Plant invasions are a global problem that affect ecosystems in a wide variety of ways. One of the most significant impacts to terrestrial ecosystems is when invasive plants affect fuel characteristics, fire behavior, and ultimately fire regimes (D'Antonio et al., 1992; Brooks et al., 2004). When invasive plants alter fire regimes in ways that promote their own persistence and dominance over native plant species, an invasive plant-fire cycle can establish (Brooks et al., 2004). The result is often alternative, self-perpetuating states that can be difficult, if not impossible, to reverse. These novel, alternative states are typically characterized by a general decline in resilience to disturbance and resistance to subsequent plant invasions that can spiral into an invasional meltdown (Simberloff and Von Holle, 1999).
One of the most effective ways to prevent landscapes from spiraling into decline is to prevent the initial development of invasive plant-fire cycles. Concepts related to general resilience to disturbance and resistance to invasive plants provide the basis for managing landscapes to increase their capacity to reorganize and adjust following fire and interacting disturbances and stressors, such as climate change (see Table 1 for definitions) (Chambers et al., 2014a, 2016; Curtin et al., 2014). Concepts related to spatial resilience provide the basis for managing landscapes to conserve resources and habitats and maintain connectivity (Holl and Aide, 2011; Rudnick et al., 2012). Coupling information on resilience to fire and resistance to invasive plants with spatial resilience enables managers to evaluate how the potential for recovery and likelihood of invasive plant-fire cycles differ across large landscapes and how these differences can affect high value resources and habitats.
Recently, new approaches and decision-tools have emerged that enable managers to understand and evaluate general and spatial resilience to fire and resistance to invasive grasses across large landscapes in arid and semi-arid shrublands and woodlands (Chambers et al., 2014a,c, 2017a,c; Ricca et al., 2018)1. These spatially explicit approaches and tools provide the capacity to quantify and visualize differences in resilience and resistance across landscapes in relation to high value resources and habitats, fire risk, and presence and abundance of invasive grasses. This has resulted in a new paradigm that allows managers to locate invasive species management and fire preparedness, suppression, and prevention activities strategically, where they are likely to have the greatest benefits for maintaining and improving resources and habitats (Chambers et al., 2014a, 2017a,c; Ricca et al., 2018)1.
Here we review our understanding of the factors that influence fire regimes, resilience to fire, resistance to invasive annual grasses, and thus development of invasive grass-fire cycles. We also discuss the factors that influence spatial resilience and the implications for high value resources and habitats. Our emphasis is on arid and semi-arid shrublands and woodlands in western North America. We use the Cold Deserts, Mediterranean Ecoregion, and Warm Deserts of North America as model systems to describe how and why resilience to disturbance and resistance to invasive annuals differ over large landscapes. We use the Cold Deserts to illustrate an approach and decision tools for prioritizing areas on the landscape for management actions to prevent development of invasive grass-fire cycles and protect high value resources and habitats.
Fire Regime Changes and Development of Invasive Grass-Fire Cycles
Fire regimes are characterized by patterns of fire seasonality, frequency, size, spatial continuity, intensity, type (crown fire, surface fire, or ground fire), and severity in a particular area or ecosystem (Agee, 1994; Sugihara et al., 2006). Fire regimes in arid and semi-arid shrublands and woodlands are highly variable because they occur over large environmental gradients and differ in vegetation composition (Brooks and Matchett, 2006; Chambers et al., 2014a). The primary environmental and vegetation characteristics that influence fire regimes are climate, topography, soils, vegetation types, and plant functional groups (Figure 1). Fire occurrence in any given year is a function of several switches—fuels (biomass), the conditioning of those fuels for burning, fire weather, and ignitions (Archibald et al., 2009; Bradstock, 2010) (Figure 1). Changes in fire regimes can result from changes in the composition of plant functional groups (Syphard et al., 2017; Bradley et al., 2018), the amount and conditioning of biomass for burning (Littell et al., 2009), and ignitions, both human and lightening caused (Fusco et al., 2016). Fire size and intensity is strongly influenced by fire weather and fire behavior (Bradstock, 2010). Increases in atmospheric CO2 concentrations that result in changes in climate and fire weather also have the potential to influence fire regimes (Littell et al., 2009; Abatzoglou and Kolden, 2013; Stavros et al., 2014) (Figure 1).
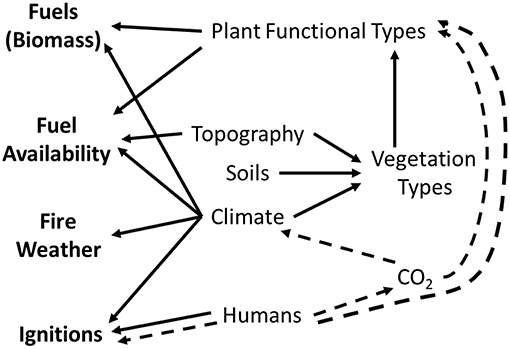
Figure 1. Influences of abiotic and biotic factors (climate, soils, vegetation types, plant functional types) on fire regimes via four “switches” (fuels, fuel availability, fire weather, and ignitions). Potential effects of changing climate, human activity, and atmospheric CO2 are indicated by dashed lines. Figure modified from Bradstock (2010).
Fires in more arid shrubland and woodland vegetation types with low amounts of widely dispersed fuels are typically fuel-limited, because the amount and continuity of fuels are generally insufficient for fire to spread. One or more years of above-normal precipitation is often required to create sufficient fuel for large wildfires to burn (Crimmins and Comrie, 2004; Littell et al., 2009; Pilliod et al., 2017). In contrast, fires in less arid shrubland and woodland vegetation types with higher amounts of densely-packed fuels are often flammability-limited in that they have enough fuel to support fires every summer, but may not be dry enough to burn (Littell et al., 2009; Abatzoglou and Kolden, 2013). In these systems, warmer and drier conditions are often required to decrease fuel moisture sufficiently for large wildfires to burn. These two conditions represent endpoints of a continuum across large landscapes.
Non-native grass invasions can alter plant functional group composition and structure within vegetation types and thus the amount and availability of fuels across broad environmental gradients. These grasses create fine fuels that are highly flammable and their invasion increases both fuel loads and fuel continuity in fuel-limited systems, even at relatively low abundances during their initial invasion (Bradley et al., 2018). Surface or near-surface fuels (primarily dead leaf material) facilitate the spread of fire in woodlands and shrublands around the globe, including Mediterranean shrublands of California (Syphard et al., 2017) and Chile (Gómez-González et al., 2011), Cold Desert shrublands in the western U.S. (Link et al., 2006), and most Australian vegetation types (Catchpole, 2002; Miller et al., 2010). Non-native grass invasions in arid and semi-arid shrublands and woodlands often result in invasive grass-fire cycles that support larger, more homogeneous, and more frequent fires (Syphard et al., 2017; Bradley et al., 2018). As a result of these invasions, fires are now becoming much more common in previously fuel-limited shrublands and woodlands where fires rarely occurred historically (Brooks et al., 2016). Fire frequencies that exceed the reproductive capacity of the dominant shrubs and trees can ultimately result in landscape conversion to invasive annual grass dominance (Miller et al., 2010; Pausas and Keeley, 2014), native or introduced forbs that are resilient to fire, or a mixture of both (Jones et al., 2018).
The amount and availability of invasive grass fuel varies across the landscape and is highly dependent on climatic/weather conditions. In arid and semi-arid shrublands and woodlands invaded by non-native grasses, wildfires tend to occur after one or more wet seasons or years and the accumulation of invasive grass fuels (Pilliod et al., 2017). Woody fuel loading and/or fine fuel loading interact with fire weather to influence the propensity for wildfires (Figure 2). As woody fuel loading and/or fine fuel loading increases, fuel packing ratios become more optimal, fuel continuity increases, and less severe fire weather is required for large wildfires. Invasive grasses increase fuel continuity and allow fires to burn under much lower fire weather severity than they would otherwise (Figure 2; Strand et al., 2014). Progressive increases in woody fuels due to management actions such as fire suppression also lowers the severity of fire weather required for large wildfires (e.g., Minnich, 2001), and can facilitate subsequent invasion of non-native grasses (Syphard et al., 2017). The length of the fire season and extreme fire weather conditions are projected to increase as the climate warms and may reduce the influence of fuel loads and continuity (Abatzaglou and Williams, 2016).
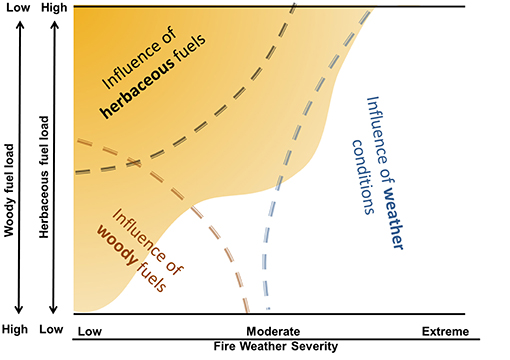
Figure 2. A conceptual model of the interaction of herbaceous and woody fuels with fire weather severity. Fuel composition is displayed on the y-axis and fire weather condition is displayed on the x-axis. Low fire weather severity is characterized by high fuel moistures, high relative humidity, low temperature, and low wind speeds, while extreme fire weather is characterized by the opposite conditions. As woody fuel loading or fine fuel loading increases, fuel packing ratios become more optimal, fuel continuity increases, and less severe fire weather is required for large wildfires. Annual grasses produce fine fuels, represented by the area in yellow in the upper left, that can fill interspaces between native fuels (shrubs and grasses). Extreme fire weather conditions, which are projected to increase in the future, can override the influence of fuel loads and continuity. Figure modified from Strand et al. (2014).
To determine if an invasive grass-fire cycle has established, it is necessary to: (1) document that a plant invasion has altered fuel bed characteristics; (2) demonstrate that these fuel bed changes alter the fire regime; and (3) show that the new regime promotes dominance of the fuels that drive the regime (D'Antonio et al., 1992). Rossiter et al. (2003) used indirect inference to test two assumptions of the invasive grass-fire model: (1) non-native grasses alter fuel load and ignitability; and (2) these changes increase frequency and/or intensity of fires (Rossiter et al., 2003). They showed that a perennial grass invader from Africa, Andropogon gayanus (Gamba grass), created fuel beds with seven times more biomass than those created by native Australian savanna species. This higher fuel load led to a fire that was eight times more intense than fires recorded in native fuel beds during the same time of year and produced the highest temperatures of any early dry season fire ever recorded in the Northern Territory, Australia. Although this study did not demonstrate that the invading species preferentially benefited from the fire behavior it created, numerous examples from other ecosystems suggest that African grasses typically benefit from frequent, moderate to high intensity fires (Brooks et al., 2004).
Resilience to Wildfire and Resistance to Invasive Grasses
General Resilience
The general resilience of ecosystems, or their broad ability to cope with disturbances (Folke et al., 2010) without changing regimes, differs among vegetation types and changes along environmental gradients in arid and semi-arid shrublands and woodlands. Ecosystem productivity and fuels generally increase over precipitation gradients. Seasonally arid vegetation types that produce more biomass have more frequent fires as illustrated for chaparral (Bond and Keeley, 2005) and sagebrush shrublands (Miller et al., 2013) in the western U.S., closed scrub to open mallee shrublands in Australia (Pausas and Bradstock, 2007; Bradstock, 2010; Miller et al., 2010), and Patagonian forests and shrublands (Mermoz et al., 2005). Areas with more frequent fires often have a higher proportion of plant functional types that are adapted to fire and thus capable of surviving and re-sprouting after fire (Mermoz et al., 2005; Pausas and Bradstock, 2007; Spasojevic et al., 2016). In relatively intact ecosystems, the combination of higher effective precipitation, greater productivity, and plant functional types adapted to fire typically results in greater resilience as indicated by smaller change in species composition following fire and more rapid return to the pre-fire community composition (Chambers et al., 2014a). Higher resource availability and plant productivity are associated with greater resilience to disturbance or recovery potential in the Cold Deserts (Condon et al., 2011; Davies et al., 2012; Chambers et al., 2014b, 2017b; Urza et al., 2017), Mediterranean Ecoregion (Corbin et al., 2007), and Warm Deserts (Brooks and Matchett, 2006).
The primary indicators of general resilience are environmental characteristics, including climate variables, topographic indices, and soil characteristics (Table 2). Ecosystem attributes and processes are also important factors in evaluating the general resilience of ecosystems (Table 2) and can include soil characteristics, land cover of vegetation types, productivity indices, species functional traits, and modeled attributes and processes, such as soil temperature and moisture regimes2 ecophysiological processes (Levine et al., 2016), and successional process after fire (Spasojevic et al., 2016). For example, in the four-corner region of the USA, remote sensing, climate data, and species trait databases were used in path analyses to evaluate whether functional diversity across a range of woodland and forest ecosystems influenced general resilience as indicated by the recovery of productivity after wildfires (Spasojevic et al., 2016). Longer term data and climate change projections make it possible to assess state changes over time and evaluate the potential for climate induced thresholds (Littell et al., 2009; Abatzoglou and Kolden, 2013).
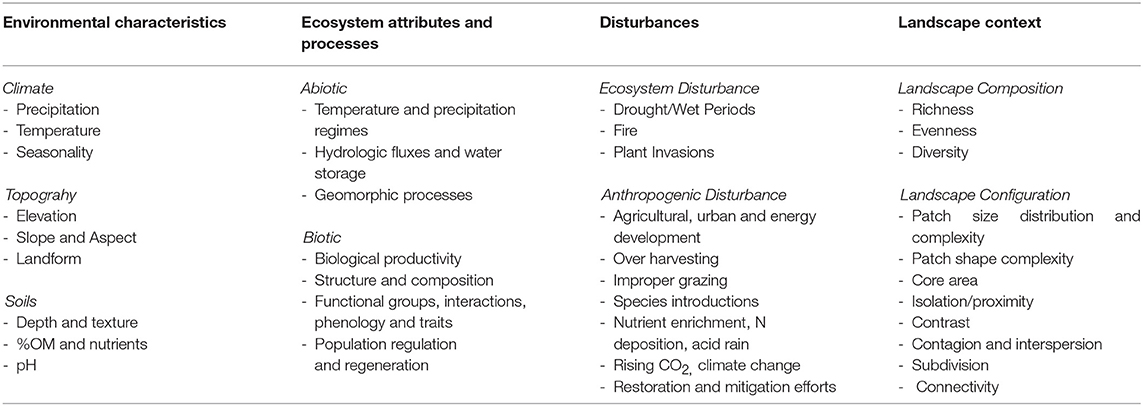
Table 2. The factors that contribute to general and spatial resilience to fire and resistance to invasive annual grasses and selected indicator variables for each factor.
Resistance to Invasive Grasses
The potential for invasive grasses to alter fire regimes and ecological resilience is strongly influenced by the system's resistance to the invasive grass. Resistance to invasive grasses is determined by the species' fundamental and realized niche (Chambers et al., 2014a). The fundamental niche is a function of a species' physiological and life history requirements for establishment, growth, and reproduction, and is highly dependent on a system's environmental characteristics. Factors such as elevation, slope, aspect, and soil characteristics determine soil temperature and water availability and affect expression of the fundamental niche of invasive grasses at plant community to landscape scales (Brooks et al., 2016). Changes in fire regimes that affect environmental factors, like soil temperature and moisture regimes, and ecosystem attributes, like biogeochemical cycling, can also influence expression of the fundamental niche (Germino et al., 2016).
The realized niche is a subset of the fundamental niche and is determined largely by resource availability, biotic interactions with the plant community, and propagule pressure (Shea and Chesson, 2002). Niche opportunities can result when the life history of the invasive grass allows it to take advantage of “unused” resources within the plant community (Chambers et al., 2016; Germino et al., 2016). In many arid and semi-arid shrublands and woodlands, this is strongly influenced by the timing and amount of soil water storage, functional group dominance, and competitive interactions (Figure 3; Chambers et al., 2016). Native or desirable plant species that use similar resource pools and have resource use patterns that coincide with spatial or temporal aspects of the establishment and growth of invasive plant species are typically the most effective competitors (Leffler and Ryel, 2012). In systems that lack sufficient desirable plant species with resource use patterns similar to the invasive grass, reduced competition and higher resource availability can result in increased biomass, seed production, and spread of the invader (Chambers et al., 2007, 2017b; Olsson et al., 2012).
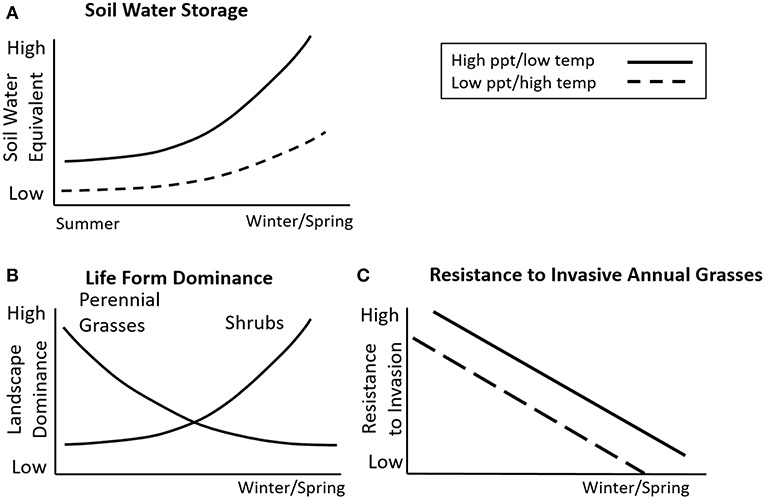
Figure 3. Changes in soil water storage, life form dominance and resistance to invasive annual grasses as seasonality of precipitation transitions from primarily summer to winter in the Cold Deserts of western North America. (A) Soil water storage increases as the proportion of winter/spring precipitation increases and these changes are relatively greater for areas with relatively high precipitation and low temperature. (B) Landscape dominance of perennial native grasses is highest with primarily summer precipitation; shrub dominance is greatest with primarily winter/spring precipitation. (C) Resistance to invasive annual grasses is higher in areas where soil water storage is low and perennial grasses dominate largely due to strong resource competition. Decreases in effective precipitation can increase resource fluctuations and lower resistance to invasive annual grasses. At more local scales, resistance also is influenced by resource availability and disturbance. Figure modified from Chambers et al. (2016).
Disturbances like improper livestock grazing (timing, duration and/or intensity), altered fire regimes, and stressors such as rapid climate change, rising CO2, and nitrogen deposition result in resource fluctuations that create niche opportunities and increase system invasibility (Davis et al., 2000; Davis and Pelsor, 2001; Shea and Chesson, 2002). The most common disturbances associated with decreased resilience and resistance in many arid and semi-arid shrublands and woodlands are improper livestock grazing and altered fire regimes (D'Antonio et al., 1992). Livestock grazing is a widespread land use in arid and semi-arid shrublands and woodlands that can alter plant functional types, biomass production, and thus fuel availability. Higher resource availability due to removal of perennial grasses and forbs by grazing can result in increases in woody species and fuel loads in shrublands and woodlands (Miller et al., 2013). Similarly, management actions, such as fire suppression, that reduce the range of natural variation (Holling and Meffe, 1996) can increase woody fuels in shrublands and woodlands that had shorter fire return intervals and lower levels of woody fuels historically (Minnich, 2001; Boyd et al., 2017). Larger and more severe fires that reduce abundance of woody species can create niche opportunities for invasive grasses.
Dispersal of invasive grass seeds and increased propagule pressure due to livestock grazing can result in increases in invasive grasses in interspaces among woody species and residual perennial grasses and forbs prior to wildfire (Reisner et al., 2013). Improper livestock grazing can also restrict native bunchgrasses to microsites under shrubs where fire intensity is greater and bunchgrass survival is less likely (Hulet et al., 2015). Higher or more contiguous fine fuel biomass can result in greater fire severity and extent, higher mortality of fire-intolerant trees, shrubs, and native grasses, and development of invasive grass-fire cycles (Pausas and Keeley, 2014). Biomass reduction of woody species for fuels management (mowing or removing shrubs, cutting down trees) can also increase resource availability and decrease resistance in areas that are climatically suited to invasive grasses, especially in sites that lack sufficient perennial natives for recovery (Prevey et al., 2010; Roundy et al., 2018).
Weather events and longer-term climate patterns can result in resource fluctuations that decrease resistance to invasion. Resource pulses due to weather events, such as above-average precipitation, can facilitate invasion where resource availability is greater than the capacity of the extant system to fully utilize the excess (Rejmanek, 1989; Davis et al., 2000). For example, extensive range expansion of Pennisetum ciliare (syn. Cenchrus ciliaris; buffelgrass) occurred in central Australia following periods of above-average rainfall in the mid-1970s and from 2000 to 2002 (Griffin et al., 1983; Friedel et al., 2006). This has been observed following El Niño years for Bromus rubens (red brome) in the Mojave Desert (Salo, 2005) and Bromus tectorum (cheatgrass) in salt desert vegetation types of the Cold Deserts (Meyer et al., 2001).
Progressive increases in CO2 concentrations and minimum temperatures over recent decades are likely resulting in increases in invasive annual grasses, but effects appear to depend on environmental characteristics and resource availability and to be context specific. Recent research indicates that addition of CO2 had positive effects on plant biomass in greenhouse studies (Ziska, 2005), no effect in a field study in the Wyoming Basin (Blumenthal et al., 2016), and depended on resource availability in the Mojave Desert (Nowak et al., 2004). Warming of minimum temperature by infrared heating had positive effects in areas of climate suitability for the invader (Campagnoni and Adler, 2014; Blumenthal et al., 2016), but warming by blocking convective cooling during days had no effect in areas at the limits of climate suitability (Larson et al., 2017).
Many of the same environmental characteristics and ecosystem attributes that determine resilience to wildfire influence resistance to invasive species (Table 2). Envelope or niche models are used to model the potential habitat of invasive plants and often serve as the basis for assessments of invasion risk. These models use distribution data for invasive plants in combination with environmental correlates (typically climatic factors) to model potential habitat across large landscapes (e.g., Jiménez-Valverde et al., 2011; Vilà and Ibáñez, 2011; Bradley et al., 2013). Bioclimatic envelope models have been developed for several invasive grasses in arid and semi-arid shrublands and woodlands, including P. ciliare in northwestern Mexico (Arriaga et al., 2004) and B. tectorum in the western US (Bradley, 2009).
Remote sensing image analysis is increasingly used as a tool for mapping invasive plant species (Bradley, 2014; He et al., 2015) including after fire (West et al., 2016). The distinct cover, morphology and/or seasonality of many invaded vs. native ecosystems allows invasive species to be detected remotely. Inter-annual variability in phenology has been used to identify annual grasses in desert ecosystems, including B. tectorum (Boyte and Wylie, 2016) and Eragrostis lehmanniana (Lehmann lovegrass) (Huang and Geiger, 2008). Accurately detecting small populations in the early stages of invasion is difficult, yet maps of heavily infested areas increase information about temporal and spatial patterns and predictors of invasion and provide another valuable tool for risk assessment (Bradley, 2014). Innovation in technology has allowed the coupling of remotely sensed data with machine learning algorithms to map fractional (i.e., continuous) cover of plant functional groups (Anderson et al., 2018; Jones et al., 2018) and invasives (West et al., 2016). This has increased the ability to detect the early presence of invasives and to map ecosystem transitions. Maps of plant functional group percent cover, including annual grasses and forbs, are currently available annually at 30 m resolution for the western U.S. from 1984 to 2017 (Jones et al., 2018), and maps of B. tectorum have been produced at 250-m resolution for a portion of the Cold Deserts since 2000 (e.g., Boyte and Wylie, 2016).
Spatial Resilience
Spatial resilience is a measure of how spatial attributes, processes, and feedbacks vary over space and time in response to disturbances and affect the ecological resilience of ecosystems within landscapes. It is determined by the composition, configuration, and functions of patches within landscapes and is closely related to resilience to fire and resistance to invasive grasses. Spatial resilience considers the distribution of vegetation types, spatial connectivity among landscape patches, and thus the ability of fire (Miller and Urban, 2000; Peters et al., 2004) and invasive plants (Bradley, 2010; González-Moreno et al., 2014; Basnou et al., 2015) to spread within a landscape. Effects of human activities on patch connectivity are key considerations in spatial resilience because they impact fire regimes, plant invasions, and thus resources needed to support habitats and species populations (Holl and Aide, 2011; Leu et al., 2011; Rappaport et al., 2015). The capacity exists to delineate system transitions by using fine resolution vegetation cover mapped across broad spatial and temporal scales (Jones et al., 2018).
At landscape scales, ignition and spread of wildfires result from complex interactions among topography, land cover, ignition sources, and weather (Figure 1). Wildfires start from a local epicenter (ignition point) and spread across landscapes as a function of the abundance and arrangement of disturbance-susceptible patches (Moreira et al., 2011). Fire spread rate can be facilitated or retarded by landscape heterogeneity. Thus, the spatial pattern of fire ignition and spread across landscapes are affected by fire proneness, i.e., the differential fire behavior in various land cover types that are not equally fire prone (e.g., Bajocco and Ricotta, 2008; Moreira et al., 2011).
Land use strongly affects fire risk by modifying vegetation structure and fuel loads, which, along with topography and weather, are the main drivers of fire intensity and rate of spread (Bradstock, 2010) (Figure 1). Thus, changes in land cover and land uses are directly linked to changes in landscape fuel patterns and fire risk (Moreira et al., 2011). Increased fire risk is expected where land use/land changes have promoted an increase in fuel loads, such as those resulting from expansion of trees into shrublands and shrubs into grasslands (Miller et al., 2013) or fuel continuity, such as those caused by annual grass invasions (Link et al., 2006). In contrast, other land uses or land cover changes can decrease fire risk when associated with the removal of biomass (e.g., targeted livestock grazing, fuel treatments) (Strand et al., 2014).
Wildland fire risk assessment and fuel management have become major activities in fire prone ecosystems as part of efforts to reduce the growing financial and ecological losses from wildfires (Ager et al., 2011; Scott et al., 2013; Chuvieco et al., 2014). Planners and fuel specialists routinely use simulation models to (1) characterize fire behavior under specific fuel and weather conditions, (2) examine potential effectiveness and ecological impacts of fuel treatment programs over a range of scales, from localized fuel types (5–50 ha) to large landscapes (1,000–50,000 ha), and (3) map fire risk to important social and ecological values (Collins et al., 2010; Ager et al., 2011). For example, wildfires are modeled to examine differences in wildfire probability and fire behavior across areas with high value conservation resources (national parks, species habitats) and to evaluate effects on wildlife habitat, soil erosion, and other factors (Scott et al., 2013). A wide variety of fire behavior models have been developed such as FlamMap, FARSITE, Behave, and FSIM along with supporting models and software to estimate appropriate weather, fuel moisture, and other input variables required to run the fire behavior models (see https://www.firelab.org/applications).
Complex interactions among climate, vegetation types, and human activity determine patterns of plant invasion across large landscapes. Land use related disturbances generally increase the likelihood of plant invasions (Gelbard and Belnap, 2003; Bradley, 2010). The risk of invasion can be evaluated based on spatial relationships among probabilities of grass invasion and land use variables, such as the distribution of roads, agriculture, and powerlines (Bradley, 2010; González-Moreno et al., 2014; Basnou et al., 2015). For example, spatial modeling was used to develop landscape-scale risk assessments associated with climate, land use variables, and invasion of B. tectorum for the State of Nevada in the U.S. (Bradley, 2010). In addition, relationships among climate, land use/land cover changes, and species invasions were evaluated for the Mediterranean region of Europe (González-Moreno et al., 2014; Basnou et al., 2015). Generalized linear models were used to examine effects of both current landscape structure and recent land use change from floristic surveys (species presence and relative abundance), climate and land cover variables, and human activity variables, and then to develop patch and landscape metrics of invasion.
Resilience and Resistance of Warm Deserts, Cold Deserts, and Mediterranean Ecoregion
The arid and semi-arid ecosystems represented by the Cold Deserts, Warm Deserts, and Mediterranean Ecoregion (Figure 4) exhibit a wide range of temperature and precipitation regimes, which influences resilience to wildfire, resistance to invasive grasses, and the tendency for grass-fire cycles to develop (see review in Brooks et al., 2016). Differences in the aridity, amount, seasonality, and predictability of precipitation, and onset of the dry season influence plant functional type dominance and have important consequences for both fire regimes and grass invasions (Figure 5A). Aridity increases across a north to south gradient, with the Mojave Basin and Range and Sonoran Basin and Range being the most arid. Summer precipitation (July, Aug, Sept) increases across a west to east gradient with the Columbia Plateau, Snake River Plain, Northern Basin and Range, and entire Mediterranean Ecoregion receiving mostly winter precipitation, and the Sonoran Basin and Range, Arizona/New Mexico Plateau, and Chihuahuan Deserts receiving mostly summer precipitation (Figure 5A). The Central Basin and Range and Mojave Basin and Range are transitional and receive varying amounts of winter and summer precipitation. Amount of precipitation received when temperature, and thus potential evapotranspiration is low influences the amount of water stored in deep soil layers, and therefore the balance between woody and herbaceous plant species in these ecoregions (Sala et al., 1997; Wilcox et al., 2012). Areas that receive more winter/spring precipitation typically have deeper soil water storage and a higher proportion of shrubs (Figure 6). In contrast, areas that receive predominantly summer precipitation have little deep-water storage and a higher proportion of perennial grasses (Figure 6). Water availability during the period when temperatures are favorable for plant growth influences the balance between C3 and C4 species with C3 species dominating in areas with cool, wet springs and C4 species tending to dominate in areas with warm, wet summers (Paruelo and Lauenroth, 1996; Sala et al., 1997).
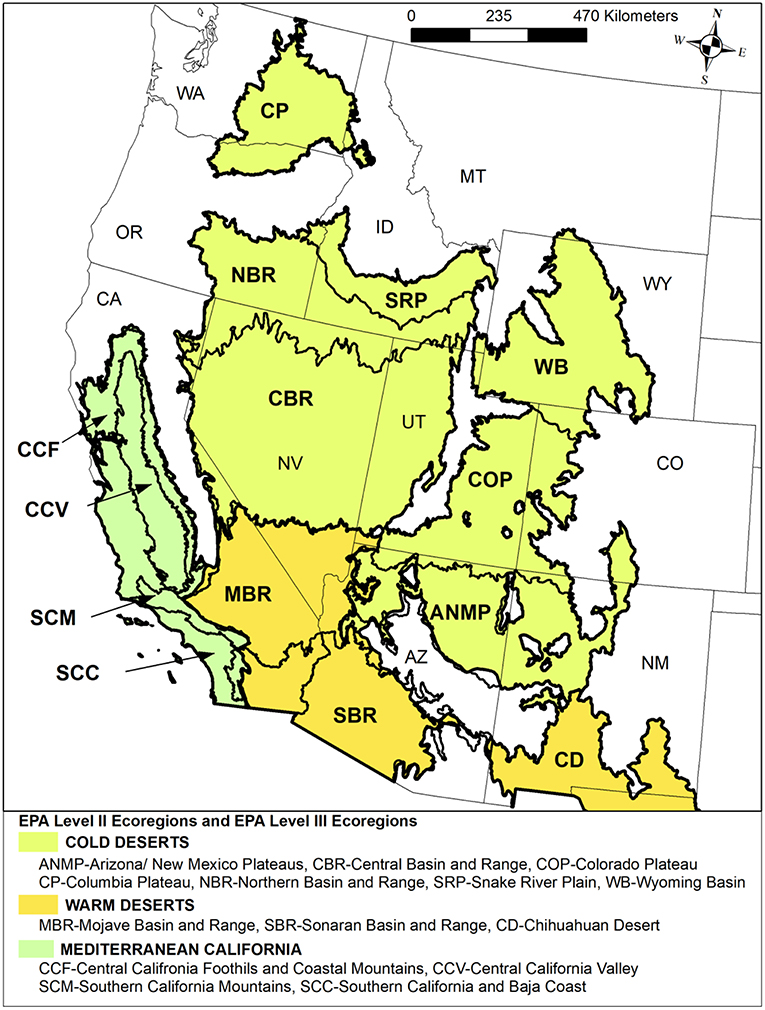
Figure 4. A map of the Cold Deserts, Mediterranean Ecoregion, and Warm Deserts Level II Ecoregions in North America and the Level III Ecoregions within them (U.S. Environmental Protection Agency [USEPA]., 2017).
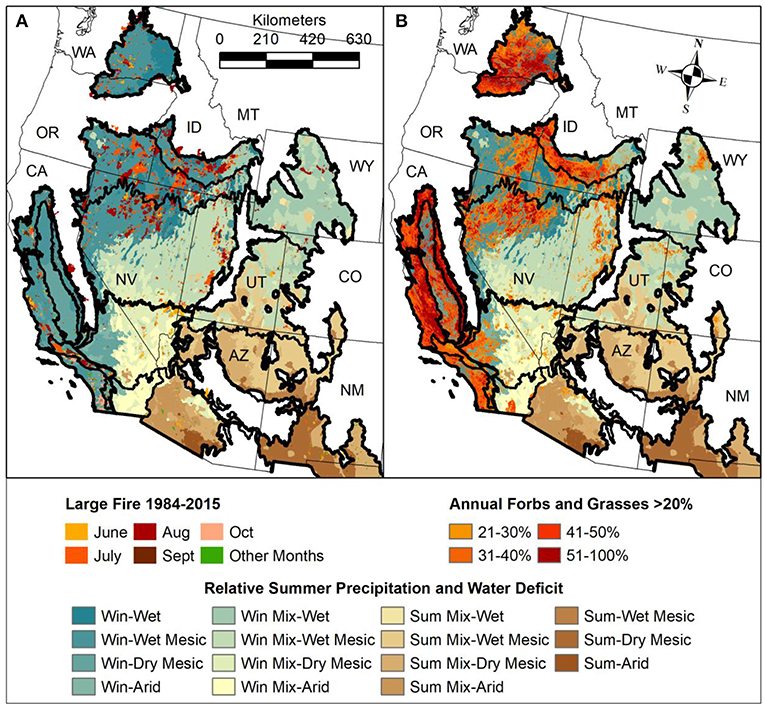
Figure 5. A generalized aridity index (Dobrowski et al., 2013) combined with the timing of precipitation (winter or summer) based on 30-year normal annual values (PRISM Climate Group, 2016) and overlaid with (A) large fires in the months of June, July, August, September, October, and other months (1984–2015; Monitoring Trends in Burn Severity [MTBS], 2018) and (B) percentage cover of annual forbs and grasses derived from the per-pixel maximum values from 2015–2017 (Jones et al., 2018).
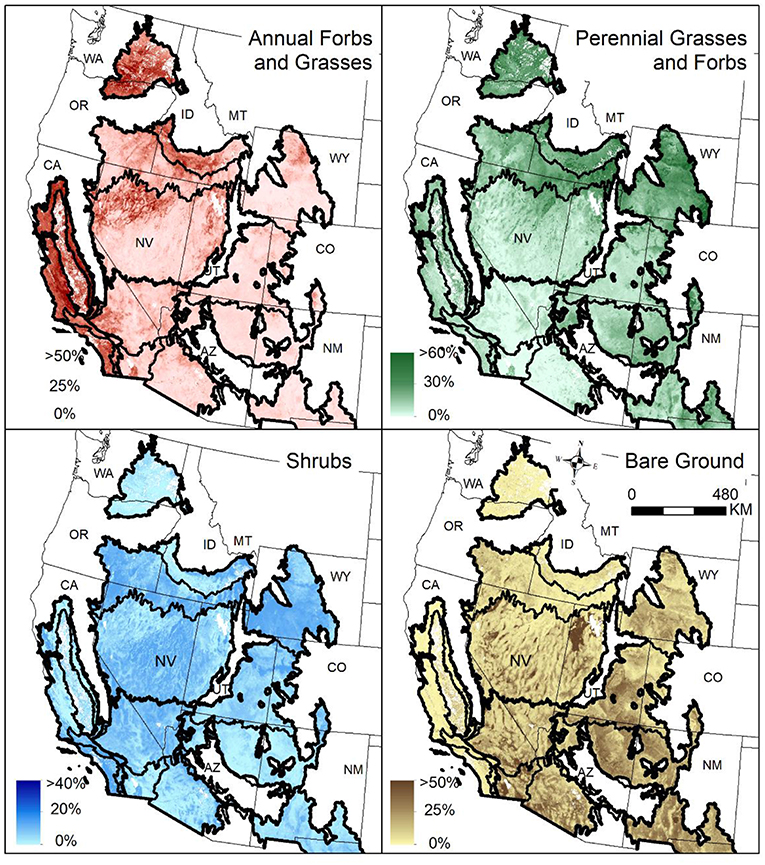
Figure 6. Continuous land cover maps of annual forbs and grasses, perennial forbs and grasses, shrubs, and bare ground averaged for 2017 (modified from Jones et al., 2018). Water, snow/ice, cropland, developed, and wetland areas have been excluded using the National Land Cover Database (NLCD) (2014).
The occurrence of large fires is related both to the degree of aridity and the timing of precipitation (Figure 5A). Lower aridity equates to higher vegetation productivity and thus greater amounts and continuity of fuels, which leads to more frequent fires in shrubland ecosystems (Bond and Keeley, 2005; Bradstock, 2010). More winter/spring precipitation typically results in longer fire seasons in which most fires burn in June, July and August. These fires vary in size but can exceed 100,000 ha (Geo MAC Wildland Fire Support., 2018). Dominance of summer precipitation typically results in shorter fire seasons in which most fires burn earlier in the year, before the onset of summer rains (Littell et al., 2009; Abatzoglou and Kolden, 2013). These fires are typically smaller. Large fires have burned in the northeastern Mojave Desert, where precipitation is a mix of winter and summer precipitation and is highly variable (Figure 5A) (Tagestad et al., 2016; Brooks et al., 2018).
The likelihood of conversion to invasive grasses is also related to the degree of aridity and timing of precipitation (Figure 5B). Resistance to invasive annual grasses is generally lowest in areas with wet winters but increases with aridity due to less favorable conditions for establishment, or increasing summer rainfall, which is associated with strong competition from native perennial grass species (Bradford and Lauenroth, 2006; Bradley, 2009). Large percentage cover of invasive annual grasses and forbs, repeated wildfires, and extensive human development likely explain relatively low cover of shrubs and perennial forbs and grasses in the less arid portions of the Mediterranean ecoregion (Syphard et al., 2017). Similar factors explain relatively low cover of shrubs and perennial forbs and grasses in the Snake River Plain and Northern Great Basin of the Cold Deserts (Knick et al., 2011; Balch et al., 2013).
Ecoregional Relationships
Prior sections show that effects of invasive grasses on fire regimes differ as a function of: (1) climatic regime and thus vegetation type; (2) plant functional groups and degree of fire adaptation of the vegetation type; (3) ecophysiological and life history characteristics of the invader; and (4) interactions with the dominant land uses and human developments. For planning and assessment, it is necessary to develop an understanding of how and why these factors differ in relation to relative resilience to fire and resistance to grass invasions. In the sections below, we discuss how these factors vary among the major vegetation types that comprise the Cold Deserts, Warm Deserts, and Mediterranean Ecoregion of the western United States.
Cold Deserts
General description
In the Cold Deserts, the dominant vegetation types occur along productivity gradients related to elevation and soil temperature and moisture regimes (Figure 7). Soil temperature regimes are predominantly warm (xeric) or cool (frigid) with small cold (cryic) areas at higher elevations and hot (thermic) areas in the south (Brooks et al., 2016). Soil moisture regimes range from winter moist (xeric; generally >30 cm annual PPT) to dry (aridic; generally <30 cm annual PPT) with large areas of dry and summer moist (aridic-ustic) in the Wyoming Basin and Colorado Plateau (Brooks et al., 2016). Salt desert vegetation types typically occur at lower elevations or in valley bottoms with drier soil moisture regimes and are dominated by members of the Chenopodiaceae, such as Atriplex spp. and Sarcobatus spp. (West, 1983a,b), but can include a diversity of shrub and grass species. Artemisia tridentata ssp. wyomingensis (Wyoming big sagebrush) and to a lesser degree A. tridentata ssp. tridentata (basin big sagebrush) types are found at low to mid elevations with warm and dry to warm and moist soil temperature and moisture regimes (West, 1983a,b; Miller et al., 2011). Artemisia tridentata ssp. vaseyana (mountain big sagebrush) and mountain brush (e.g., Symphoricarpos spp. [snowberry.], Purshia tridentata [antelope bitterbrush]) types occur at upper elevations with cool and moist to cold and moist regimes (West, 1983a,b; Miller et al., 2011). Associated species differ regionally, and the relative proportion of shrubs vs. herbaceous species can be highly dependent on grazing history and time since fire.
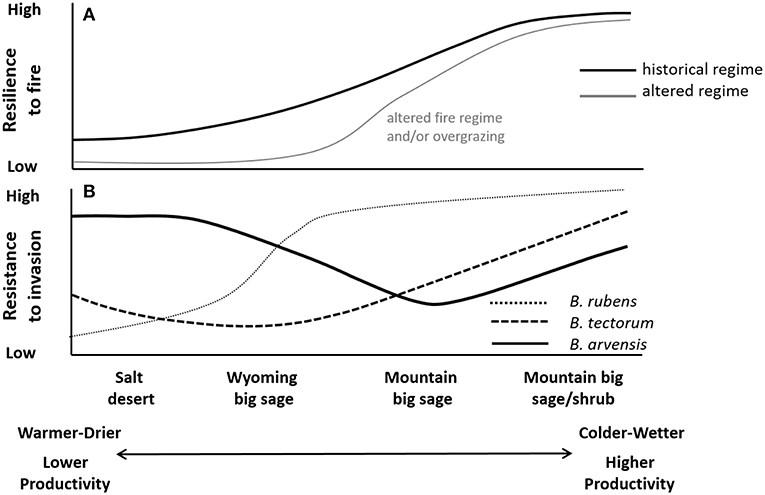
Figure 7. Hypothetical (A) resilience to historical and altered disturbance regimes (primary altered regime characteristic labeled in gray) and (B) resistance to common invasive annual grass species in the Cold Deserts. Adapted from Chambers et al. (2014a).
Resilience to fire
Resilience to fire increases along soil temperature, precipitation, and thus productivity gradients as observed in other arid and semi-arid shrublands (Figure 7) (Condon et al., 2011; Davies et al., 2012; Chambers et al., 2014a,b; Urza et al., 2017). In general, warmer and drier salt desert and Wyoming big sagebrush types have lower fuel biomass and availability and experienced few historical fires—fire return intervals varied regionally but were as long as 100 or more years (Miller et al., 2013). Consequently, these types have relatively low resilience to fire. In contrast, cooler and moister mountain big sagebrush and mountain big sagebrush/mountain shrub types are typically characterized by relatively high fuel biomass and availability and experienced more historical fires—fire return intervals also varied regionally but were as short as 10–12 years (Miller et al., 2013). These types have greater resilience to fire as indicated by more rapid post-fire recovery and smaller changes in species composition (Davies et al., 2012; Chambers et al., 2014b).
Resistance to invasion
The most problematic invasive grasses are winter annuals that are well-suited to those areas dominated by winter precipitation. These invasive annuals germinate in fall or spring, exhibit rapid growth, and are highly effective competitors for soil resources during spring to early summer (Chambers et al., 2007, 2016). Invasibility is closely related to soil climatic regimes as illustrated for the widespread invasive brome grasses, which are causing invasive grass-fire cycles (Figure 7) (Chambers et al., 2007, 2016, 2017b; Brooks et al., 2016). For example, germination, growth, or reproduction of B. tectorum is physiologically limited in relatively warm and dry salt desert sites at lower elevations by frequent, low precipitation years, constrained by low soil temperatures in mountain big sagebrush sites at high elevations, and optimal under relatively moderate temperature and water availability in Wyoming big sagebrush sites at mid elevations (Meyer et al., 2001; Chambers et al., 2007). In contrast, B rubens (red brome) is less cold tolerant (Bykova and Sage, 2012) and occurs primarily on warm and dry salt desert sites and Warm/Cold-desert transitional sites (Salo, 2005). Field brome (B. arvensis) is limited on warm and dry as well as cold sites, but can be relatively abundant on cool and moist sites (Baskin and Baskin, 1981). In the Wyoming basin and Colorado Plateau, where summer precipitation (ustic soil moisture regimes) and the relative abundance of perennial grasses is higher, invasive annual grasses appear less competitive (Figure 5) (Bradley, 2009; Bradley et al., 2018). None-the-less, these invasive grasses can persist following disturbance and are a rapidly emerging problem (Bradford and Lauenroth, 2006; Mealor et al., 2013) that may be further affected by climate change (Bradley et al., 2016). Other invasive annual grasses, such as medusahead (Taeniatherum caput-medusae) and North Africa grass (Ventenata dubia) are well-established in the Cold Deserts and appear to be expanding (Wallace et al., 2015), but their climatic tolerances are less well-studied.
Resistance in Cold Deserts is decreased by disturbances and stressors that increase dispersal, reduce perennial species cover and abundance, and elevate resource availability. The probability of B. tectorum presence is elevated significantly adjacent to agriculture, power lines, and roads (Bradley, 2010). Bromus tectorum presence is strongly associated with decreases in perennial native species, especially grasses and forbs, biological soil crusts, and distance between perennial herbaceous species (gaps) due to improper livestock grazing across a range of vegetation types Dettweiler-Robinson et al., 2013; Reisner et al., 2013.
Potential for development of invasive grass-fire cycles
The potential for invasive grass-fire cycles to develop is greatest in areas with low to moderate resilience to fire and resistance to invasive annual grasses. In fuel-limited salt desert and Wyoming big sagebrush types, invasion of non-native annual grasses and forbs can alter plant functional group composition within vegetation types and increase the amount and availability of fuels following high precipitation years (Littell et al., 2009; Abatzoglou and Kolden, 2013). Even small amounts of B. tectorum cover are associated with large increases in wildfire probability; B. tectorum has advanced the time of wildfire by 10 days in summer and increased the chances of ignition by humans (Bradley et al., 2018). Although abundance of these species is generally low in the Wyoming Basin and Colorado Plateau, abundance increases with wildfire (Knight et al., 2014), particularly in drier areas Floyd et al., 2006; Shinneman and Baker, 2009.
Warm Deserts
General description
Warm Deserts are the hottest and driest regions of the western United States. Soil temperature regimes are either hot or very hot (hyperthermic; >22°C) and soil moisture is mostly aridic, meaning that the soil is dry for at least half of the growing season and moist for <90 consecutive days (Brooks et al., 2016). There is a significant gradient in seasonality of precipitation, where a mix of summer and winter precipitation characterizes the northern and western areas and predominantly summer precipitation characterizes the southern and eastern areas. As a result, the Chihuahuan Deserts are characterized by shrublands and grasslands that can support relatively high perennial plant cover (20–30%), while the Mojave and Sonoran Deserts largely support shrublands where perennial cover of the most arid regions can be quite low (<7%). These differences are illustrated here for the Mojave Desert (Figure 8).
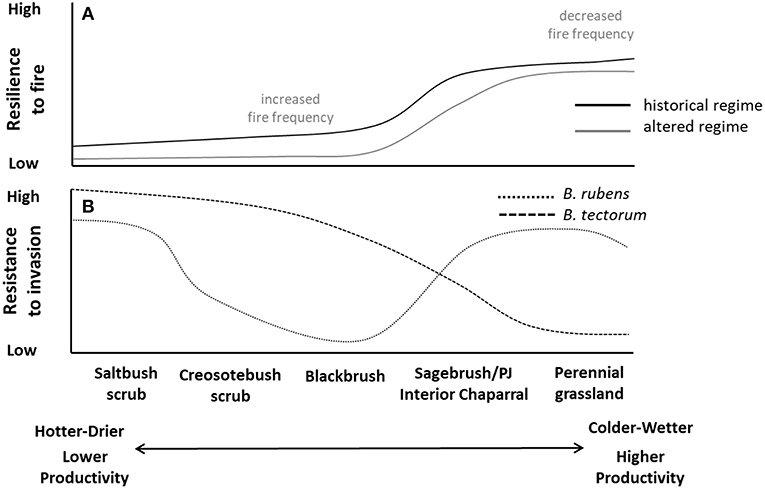
Figure 8. Hypothetical (A) resilience to historical and altered fire regimes (primary altered regime characteristic labeled in gray) and (B) resistance to common invasive annual grass species in the Mojave Desert. Adapted from Brooks et al. (2016).
Resilience to fire
High elevation and desert montane vegetation such as sagebrush steppe, interior (Arizona) chaparral, and pinyon-juniper woodlands of the Mojave and Sonoran Deserts have higher resilience due to more frequent fires historically and fire tolerant plant functional groups (Brooks et al., 2018) (e.g., Figure 8). Desert grasslands of the Sonoran and Chihuahuan deserts, and riparian vegetation throughout the Warm Deserts, also have fire tolerant plant functional groups and thus relatively high resilience. In contrast, vegetation types typical of hotter and/or drier conditions, such as creosote bush scrub and saltbush scrub, had fewer fires historically and have low resilience to fire.
Resistance to invasion
Bromus rubens is the most ubiquitous invasive annual grass across the Warm Desert region (Brooks et al., 2016). It occurs in all but the hottest and driest regions, and is most abundant in middle elevation creosote bush scrub and blackbrush shrubland, especially in moister microsites beneath shrubs, in rock crevices, and on north-facing slopes (Brooks and Berry, 2006; Brooks, 2009; Klinger et al., 2011). Schismus spp. (Mediterranean split-grass) is widespread at lower elevations where it can dominate in interspaces and areas beneath shrub canopies (Brooks, 2009). Bromus tectorum is much more restricted in its geographic distribution and is typically most abundant at higher elevations (Klinger et al., 2011). Localized areas of higher soil moisture, such as roadsides, riparian areas, and agricultural/urban areas can support high levels of both B. tectorum and B. diandrus (ripgut brome) (Brooks, 2009; Dudley, 2009).
Perennial invasive grasses are becoming increasingly prevalent, especially in the monsoonal regions of the Sonoran Desert. This region has higher minimum temperatures and summer rainfall which promote establishment and growth of P. ciliare, especially in shrublands (Marshal et al., 2012). Eragrostis lehmanniana (Lehmann lovegrass) is a perennial grass introduced for forage that is highly invasive and can dominate desert grasslands (Van Devender et al., 1997). Penniseum setaceum (purple fountaingrass) is a perennial grass introduced through ornamental horticulture that is currently expanding its range near urban areas in the Mojave Desert (Brooks, 2009).
Resistance to invasive grassed decreases along road corridors and near urban areas with high propagule pressure (Brooks, 2009) and where current or historic livestock grazing has reduced perennial vegetation cover (Brooks and Pyke, 2001; Brooks et al., 2007). Long-term reductions in resistance to invasion can be caused by repeated fires at higher elevations, or even single fires at lower elevations (Klinger and Brooks, 2017). Also, atmospheric nitrogen deposition downwind of urban or agricultural areas can increase soil nitrogen availability and biomass of invasive annual grasses and may elevate the potential for fire, and invasive grass dominance (Brooks, 2003; Allen et al., 2009; Rao and Allen, 2010; Rao et al., 2014).
Potential for a grass-fire cycle
In Mojave and Sonoran Deserts shrublands susceptibility to grass-fire cycles is greater where: (1) climatic regimes support native vegetation, which is not quite sufficient in amount, continuity, or flammability to have promoted periodic historical fires and thus to have evolved fire-tolerant traits; and (2) seasonal precipitation is sufficient to allow invasive annual grasses to establish, spread, and eventually dominate landscapes. For example, blackbrush scrub has insufficient fuels to carry fire under most conditions and exhibits slow recovery following fire, so resilience to fire tends to be low. High rainfall years can increase fine fuels from B. tectorum and B. rubens and result in large stand replacing fires, very slow recovery of native perennial shrubs, and rapid increases in Bromus spp. that ultimately cause reduced fire return intervals, extirpation of the dominant shrub species over large areas, and progressive dominance of the invasive annual grasses (Brooks et al., 2018; Klinger et al., 2018).
In grasslands of the eastern Sonoran and Chihuahuan Deserts, conditions that reduce the vigor of native perennial grasses (e.g., drought or excessive livestock grazing) can reduce resistance to invasive perennial grasses (Olsson et al., 2012). Some species, such E. lehmanniana, have differing phenology than the native perennial grasses and are shifting fire seasons in ways that negatively affect native species.
Mediterranean Ecoregion
General description
The Mediterranean region of California has relatively cool and wet winters and hot and dry summers (Keeley and Syphard, 2018). Soil temperature regimes are mostly hot (thermic) to warm (mesic) and moisture regimes are mostly dry (aridic) to winter moist (xeric). Compared to the Cold Deserts, the Mediterranean region has warmer temperatures; compared to the Warm Deserts, it has similar temperatures but moister conditions and receives a much higher percentage of precipitation in winter (Brooks et al., 2016). A strong productivity gradient exists where sage scrub is at the hotter and drier end and mixed conifer forest is at the cooler and wetter end (Figure 9). Perennial grasslands, oak savannas and woodlands, and chaparral occupy the middle of the gradient.
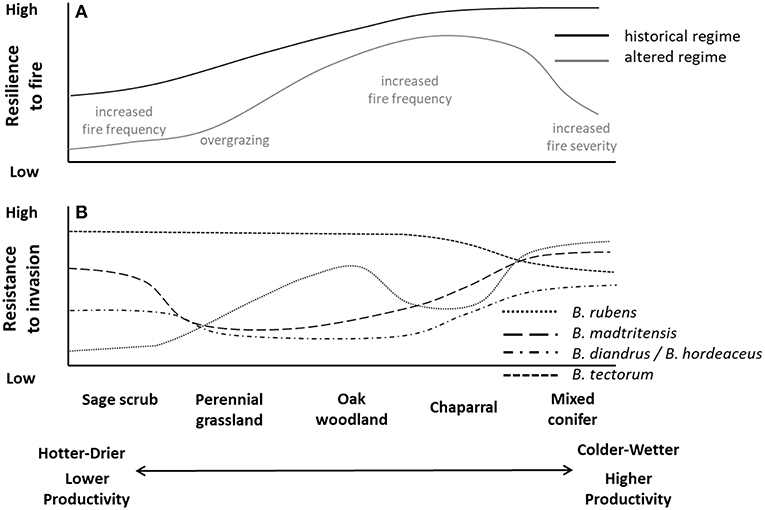
Figure 9. Hypothetical (A) resilience to historical and altered fire regimes (primary altered regime characteristic labeled in gray) and (B) resistance to common invasive annual grass species in the Mediterranean California ecoregion. Adapted from Brooks et al. (2016).
Resilience to fire
Resilience to fire is lower in hotter and drier areas and higher in cooler and wetter areas (Figure 9) (Keeley and Syphard, 2018). Only relict stands of saltbush scrub remain due to conversion to agriculture, low resistance to invasive annual grasses, and low resilience to fire (Wills, 2018). Sage scrub is still abundant where conditions are more mesic and constituent species are more fire tolerant, but it is less abundant in more arid areas where the constituent species are less fire tolerant (Borchert and Davis, 2018; Keeley and Syphard, 2018). Forest, woodlands, shrublands, and grasslands of the central coast are most resilient to fire due to the climatically moderating influences of the Pacific Ocean and a history of periodic fire (Borchert and Davis, 2018). Chaparral is highly resilient to fire, except under very short fire return intervals driven by annual grass invasions (Keeley and Syphard, 2018).
Resistance to invasion
The Mediterranean region has a long history of plant invasions dating to the mid-1700s and the Spanish missionary period (Heady, 1977). Many of the annual invasive grasses, like B. rubens, were originally introduced as seed contaminants in wheat and barley (Salo, 2005). Land use changes associated with human settlement, including widespread tilling associated with agriculture and extensive livestock grazing, reduced resistance of native ecosystems to invasion and resulted in spread of the invaders (Keeley and Syphard, 2018). Annual grasses in the genera Bromus and Avena are especially prevalent in Mediterranean regions (Klinger et al., 2011, 2018; Brooks et al., 2016). Invasion of perennial grass species, such as Cortaderia spp. (jubatagrass, pampasgrass), into wildland areas is facilitated by intentional introductions for ornamental horticulture (California Invasive Plant Council [Cal-IPC], 2018). High cover of native perennial species in chaparral tends to increase resistance to invasion, although fire can provide windows of lower resistance to invasion. Annual non-native forbs with potential to promote fires (e.g., Brassica spp.) are also abundant in these systems and typically have long-lived seedbanks that can persist for decades and then germinate following fire.
Potential for a grass-fire cycle
Two interrelated mechanisms can lead to grass-fire cycles in Mediterranean shrublands and reduce native shrubland resilience to fire and resistance to invasive grasses. Fire return intervals shifting from 20 to 50 years to 1–15 years can cause mortality of shrub seedlings and resprouting adults and significantly delay native shrub recovery (Keeley and Syphard, 2018). Also increased urbanization and conversion to agriculture can fragment shrubland patches, provide propagule sources for new invaders, and increase atmospheric nitrogen deposition—conditions that can increase fine fuels and fire frequency (Klinger et al., 2018). Grass-fire cycles have the highest potential to occur in more arid vegetation types such as saltbush scrub of the Central Valley and sage scrub of the interior valleys of southern California. Although the understory of oak savannas is now dominated by non-native annuals (>90% in many cases), these species have replaced mostly native herbaceous annuals with somewhat similar fuel characteristics and thus may not substantively change the fire regime.
Using a Multi-Scale, Resilience-Based Framework to Manage Invasive Grass-Fire Cycles
The extent of grass invasions and development of invasive grass-fire cycles around the globe indicate the need for strategic, multi-scale approaches that enable managers to determine where and how to invest limited fire management and restoration resources. An understanding of ecological resilience to disturbances like wildfire and resistance to invasive grasses can be used to facilitate regional planning and prioritize management actions such as fuels management, early detection and rapid response to new invasions (U. S. Department of the Interior [USDOI], 2016), fire suppression, and passive or active restoration (Chambers et al., 2014a, 2017a,b). Here, we provide information to apply the multi-scale, resilience-based framework described in1 to address invasive grass-fire cycles in arid and semiarid shrublands and woodlands.
The framework for prioritizing management actions to address invasive grass-fire cycles at landscape scales is based on (1) general resilience as indicated by environmental characteristics and ecosystem attributes and processes, (2) spatial resilience based on landscape composition and configuration, and thus capacity to support high value resources, and (3) interactions of general and spatial resilience with invasive annual grasses and fire. In the framework, a spatially explicit approach is used that enables managers to quantify and visualize differences in general and spatial resilience across the landscape in relation to cover of invasive annuals and fire risk. Assessments are typically conducted at the scale of one or more Level III ecoregions (Figure 4), and funding and human resources are allocated in a manner designed to maximize management investments. Here, we provide an example of how to use this framework for the Cold Deserts.
Steps in the Process
Develop the Management Objectives
Identifying appropriate management objectives and strategies in the context of long-term adaptive management programs is critical for long-term success. Adaptive management programs are designed to reduce uncertainty in the effectiveness of management actions by continually evaluating and adjusting management objectives and strategies to improve the effectiveness of management actions overtime. Adaptive management programs facilitate “learning by doing” and can help land managers and stakeholders examine the context, options, and probable outcomes of decisions through an explicit and repeatable process (Allen et al., 2011; Marcot et al., 2012; Thompson et al., 2013).
• Objectives for addressing invasive grass-fire cycles provide the basis for managing ecosystems to increase their capacity to reorganize and adjust to ongoing change while providing necessary ecosystem services. Key management objectives for the Cold Deserts typically include:
• Strategic location of firefighting resources to suppress wildfires in areas with high to very high fire risk and high value resources and habitats in intact salt desert and Wyoming big sagebrush types, and to prevent invasive grass fueled fires from burning through areas with high value resources and habitats in general;
• Prioritization of fuel treatments, specifically where, how much, and how to treat surface and canopy fuel to prevent uncharacteristic wildfires and protect high value resources and habitat;
• Prioritization of areas with little to no invasive plants for early detection and rapid response weed management strategies (U. S. Department of the Interior [USDOI], 2016), low to moderate levels of invasion for active weed management/restoration, and high levels of invasion for containment (Mealor et al., 2013);
• Prioritization of post-fire restoration treatments (soil stabilization, weed control, seeding) to focus on areas that will benefit most, specifically those areas that require soil surface stabilization or lack sufficient perennial natives to recover without treatments but still have the capacity to recover to a desirable state.
Develop Landscape Indicators of General Resilience and Resistance to Invasive Grasses
Information on the general resilience of ecosystems enables managers to: (1) evaluate differences in ecosystem responses to disturbance and recovery potentials across landscapes; (2) identify locations where ecosystems may exhibit critical transitions to novel alternative states in response to fire or other drivers; and (3) determine where conservation and restoration investments will have the greatest benefits1. Environmental characteristics (Table 2) are commonly used as indicators of general resilience and resistance to invasive plants because of their effects on ecosystem attributes and processes and plant invasions. In arid and semi-arid shrublands and woodlands, soil temperature and moisture regimes provide one of the most complete data sets for understanding and mapping potential resilience and resistance to invasive annual grasses. Soil temperature and moisture regimes are mapped for most of the region and are available through the USDA Natural Resources Conservation Service, Web Soil Survey (Figure 10A) (https://websoilsurvey.nrcs.usda.gov). In the Cold Deserts, the dominant vegetation (ecological) types have been characterized according to soil temperature and moisture regimes, general resilience to disturbance, and resistance to invasive annual grasses (Chambers et al., 2017a) based on recent research (Chambers et al., 2007, 2014b, 2017b; Condon et al., 2011; Davies et al., 2012; Urza et al., 2017) and expert input. State-and-transition models, which provide information on the alternative states, ranges of variability within states, and processes that cause plant community shifts within states as well as transitions among states, have been developed for the dominant vegetation types (Chambers et al., 2017a). To facilitate landscape analyses and prioritization, soil temperature and moisture regime subclasses have been used to categorize relative resilience to disturbance and resistance to invasive annual grasses as high, moderate, or low across the Cold Deserts (Figure 10B) (Maestas et al., 2016; Chambers et al., 2017a).
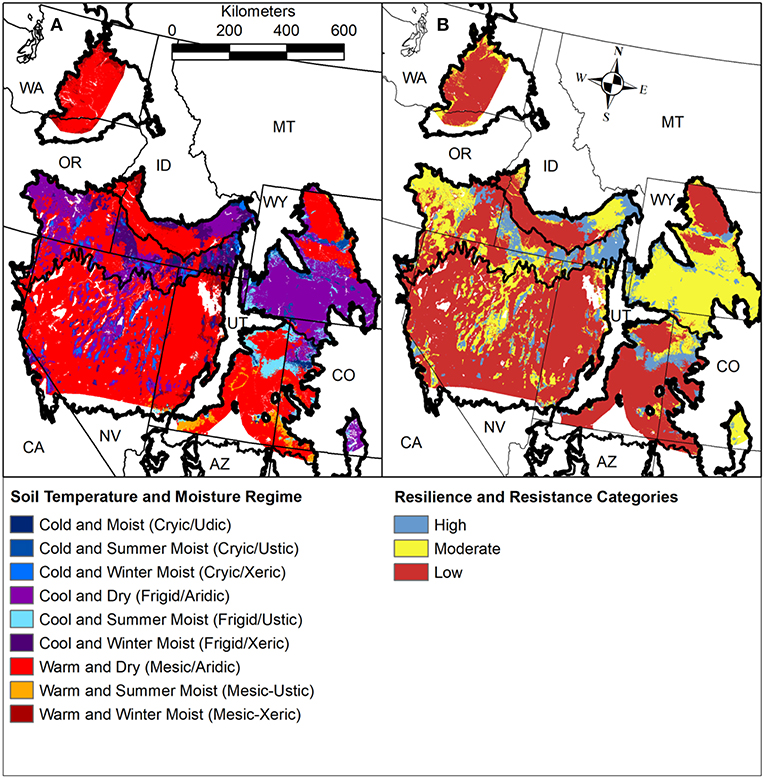
Figure 10. (A) Soil temperature and moisture regimes by moisture subclass derived from the Web Soil Survey for the Cold Deserts in western North America and (B) resilience and resistance categories developed from the soil temperature and moisture regimes (Maestas et al., 2016; Chambers et al., 2017a).
Develop an Understanding of Spatial Resilience
A understanding of spatial resilience in the context of landscapes provides the necessary information for creating functionally connected networks that provide ecosystem services and conserve resources and species. The landscape context provides information on (1) availability of resources and habitats to support species populations, (2) connectivity among resources and habitats, and (3) spatial constraints on ecological resilience and system recovery potential (Holl and Aide, 2011; Rudnick et al., 2012; McIntyre et al., 2014; Rappaport et al., 2015; Ricca et al., 2018). In the Cold Deserts, sagebrush ecosystems and the species that depend on them are threatened by progressive expansion of invasive annual grasses and development of grass-fire cycles and are a high priority for management (Knick et al., 2011; Miller et al., 2011; Davies et al., 2012). Landscape cover of sagebrush provides a regional metric of habitat availability and has been shown to be an important predictor of persistence of sagebrush obligate species (Rowland et al., 2006; Aldridge et al., 2008; Hanser et al., 2011; Wisdom et al., 2011; Knick et al., 2013).
Sage-grouse are broadly distributed species that occupy a diversity of environments containing sagebrush and have been managed as umbrella species for over 350 species of plants and animals that depend on sagebrush ecosystems (Suring et al., 2005; Knick et al., 2013).
Greater sage-grouse has been considered for listing under the U.S. Endangered Species Act several times and its status will be reevaluated in 2020 (U.S. Department of the Interior, Fish and Wildlife Service [USFWS], 2015). Here we use ecological minimum requirements underlying sage-grouse distributions (Knick et al., 2013; Chambers et al., 2014c) as a metric for evaluating spatial resilience in sagebrush ecosystems. Sagebrush landscape cover is derived from remotely sensed land cover data using a moving window analysis (Knick et al., 2013). Prior analyses show that percentage landscape cover of sagebrush around Greater sage-grouse leks (mating sites) is an indicator of the relative probability of lek persistence in different areas within the sagebrush biome (Aldridge et al., 2008; Wisdom et al., 2011; Knick et al., 2013). Greater sage-grouse lek persistence is low with 1 to 25% landscape cover of sagebrush, intermediate with 25 to 65%, and high with >65% (Chambers et al., 2014c). Although metrics more specific to sage-grouse have been developed, such as the probability of breeding bird habitat (Doherty et al., 2016), we use a modification of the three categories of landscape cover of sagebrush as a general metric of spatial resilience (Figure 11A). Intersecting the resilience and resistance index with the landscape cover of sagebrush categories provides information on sagebrush habitat availability and connectivity, potential for recovery following wildfire, and spatial constraints on recovery (Figure 11B).
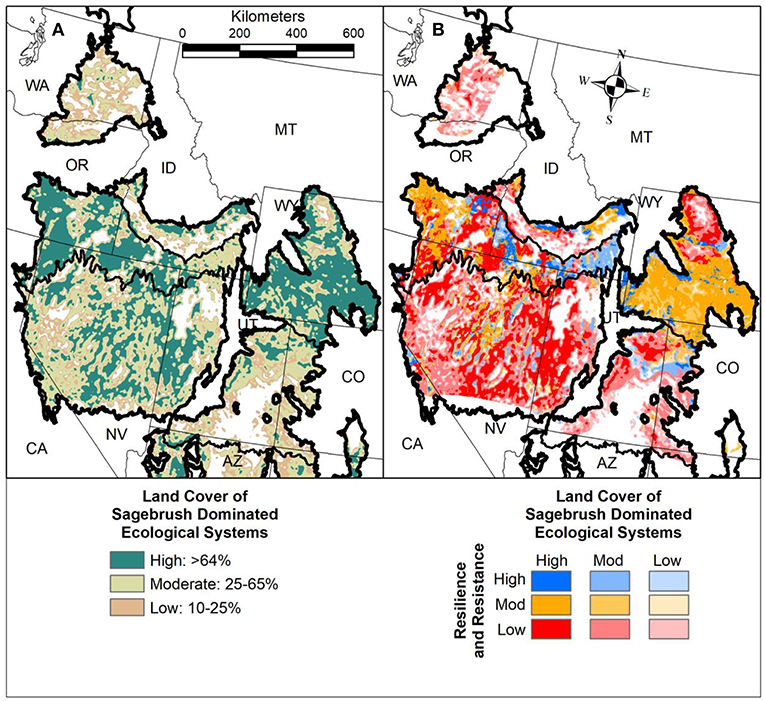
Figure 11. (A) Landscape cover of sagebrush in the Cold Deserts of western North America (low = 10–25%, moderate = 25–65%, high = >65%) (U.S. Department of the Interior, 2014). Categories of sagebrush landscape cover are based on ecological minimum requirements underlying sage-grouse distributions (Knick et al., 2013; Chambers et al., 2014c). Percentage of sagebrush within each of the categories was determined within a 5 km radius of each sagebrush pixel. (B) Landscape cover of sagebrush categories intersected with resilience and resistance categories developed from soil temperature and moisture regimes (Maestas et al., 2016; Chambers et al., 2017a).
Develop an Understanding of Fire Risk in Relation to Grass Invasions
Identifying fire risk in relation to grass invasions facilitates prioritization and selection of effective management strategies. Information on the probability of wildfire and land cover of invasive plants enables managers to: (1) identify vegetation types and areas on the landscape with the potential for transitions to less-desirable alternative states; (2) target management actions designed to reduce or mitigate wildfire and invasion; and (3) facilitate transformation to new states where disturbances and/or climate change are preventing return to desirable prior states. A large-fire risk assessment for the United States has been developed from modeled burn probabilities and fire size distributions based on weather data, spatial data on fuel structure and topography, historical fire data, and fire suppression effects (Finney et al., 2011), which was recently updated (Short et al., 2016). Also, cover estimates of annual forbs and grasses in the western United States were recently derived by combining over 30,000 vegetation field plots with satellite imagery, gridded meteorology, and abiotic land surface data (Figure 6) (Jones et al., 2018). Intersecting the resilience and resistance index, sagebrush landscape cover categories, and large fire risk provides spatially explicit information not only on the likelihood of large fires, but also on likely responses to those fires and effects on high value habitat (Figure 12A). Intersecting the resilience and resistance index, sagebrush landscape cover categories, and percentage land cover of annual forbs and grasses provides spatially explicit information on the current magnitude of invasion and thus the types of management actions most likely to be needed and effective, both pre- and post-fire (Figure 12B). These maps can be scaled down to local field offices or project areas to facilitate planning designed to locate management strategies where they will be most effective (Figure 13).
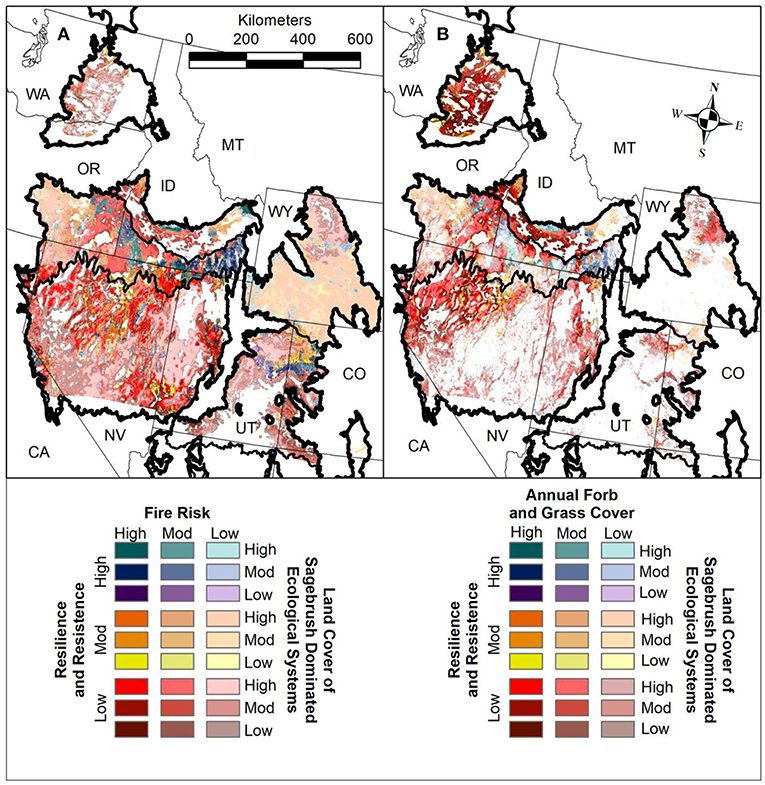
Figure 12. (A) Map illustrating relative fire risk for sagebrush dominated ecosystems in the Cold Deserts. Large fire risk (U.S. Department of Agriculture, Forest Service [USDA FS], 2018) is intersected with resilience and resistance categories developed from soil temperature and moisture regimes (Maestas et al., 2016; Chambers et al., 2017a), and landscape cover of sagebrush categories (low = <25%, moderate = 25–65%, high = >65%) based on ecological minimum requirements underlying sage-grouse distributions (Knick et al., 2013; Chambers et al., 2014c). (B) Map of annual forb and grass risk for sagebrush dominated ecosystems in the Cold Deserts. Continuous land cover of annual forbs and grasses (low = 10–20%; moderate = 20–40%; high = >40%) (Jones et al., 2018) is intersected with resilience and resistance categories developed from soil temperature and moisture regimes (Maestas et al., 2016; Chambers et al., 2017a) and landscape cover of sagebrush categories based on ecological minimum requirements underlying sage-grouse distributions (Knick et al., 2013; Chambers et al., 2014c).
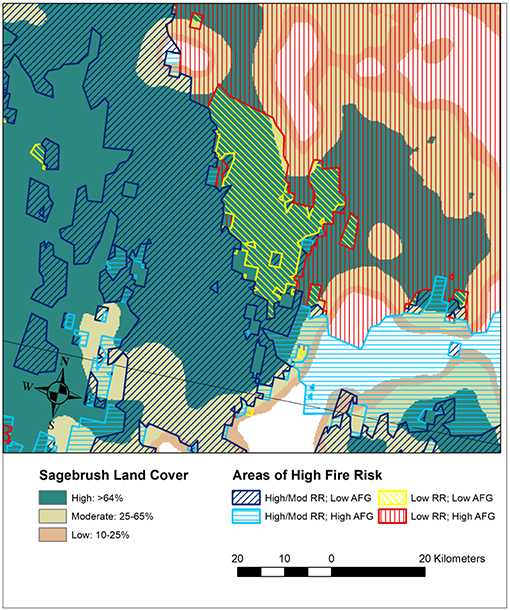
Figure 13. A map of an area on the Idaho/Nevada border that overlays relative sagebrush dominance with areas of high to very high fire risk that have (1) high to moderate resilience and resistance and low annual forb and grass cover, (2) high to moderate resilience and resistance and high annual forb and grass cover, (3) low resilience and resistance and low annual forb and grass cover, and (4) low resilience and resistance and high annual forb and grass cover. The geospatial data sources are described in Figure 12. Areas with high cover of sagebrush that have low resilience and resistance, high fire risk, and low cover of annual forbs and grasses are among the highest priorities for protective management strategies, such as conservation easements and early detection and rapid response management of invasive plants. Areas with moderate cover of sagebrush that have high to very high fire risk are areas to consider for treatments that will increase connectivity and resilience to wildfire, such as fuel treatments, fuel breaks, and seeding after wildfires. Areas with low cover of sagebrush may have limited ability to support desired resources and habitats and where associated with high levels of invasion or human development, fire prevention, preparedness, and suppression may be high priorities.
Areas with high to very high risk of large fires and high cover of annual grasses and forbs are typically locations where annual grass-fire cycles have developed (Figures 12A,B). These areas occur in the western part of the region with predominantly winter precipitation (Figure 5). In the western part of the region, many areas with low resilience and resistance and high landscape cover of sagebrush have high to very high risk of large fires and high cover of annual forbs and grasses (Figures 12A,B). In moderate and especially high resilience areas, the cover of annual forbs and grasses is generally lower. However, fire risk is not affected by resilience category and areas with both low and high resilience have high to very high fire risk. In the eastern part of the region, both large fire risk and cover of annual grasses and forbs is lower (Figures 12A,B). However, areas with moderate fire risk and moderate cover of annual grasses and forbs exist in areas with high landscape cover of sagebrush.
Management Applications
The resilience and resistance matrix is a decision-tool that provides the ability to consider resilience to wildfire and resistance to invasive grasses along with spatial resilience when prioritizing areas for management actions to prevent development of invasive grass-fire cycles at landscape scales (Table 3). The matrix allows managers to determine both the locations where management actions are likely to have the greatest benefits and the types of activities most likely to be effective. In the matrix, as resilience and resistance go from low to high (indicated by the lower to upper rows), the recovery potential increases as a function of the amount of change from the initial or desired state and the recovery time following disturbance. As landscape cover of sagebrush, a surrogate for spatial resilience, goes from low to high within these same systems (indicated by the columns), the capacity to support high value habitat and resources increases as a function of the size and shape of habitat and resource patches and their connectivity. Geospatial analyses and maps of landscape cover of sagebrush and relative resilience and resistance coupled with the risk of large fires and cover of annual forbs and grasses informs both management priorities and strategies within planning areas (Figure 13).
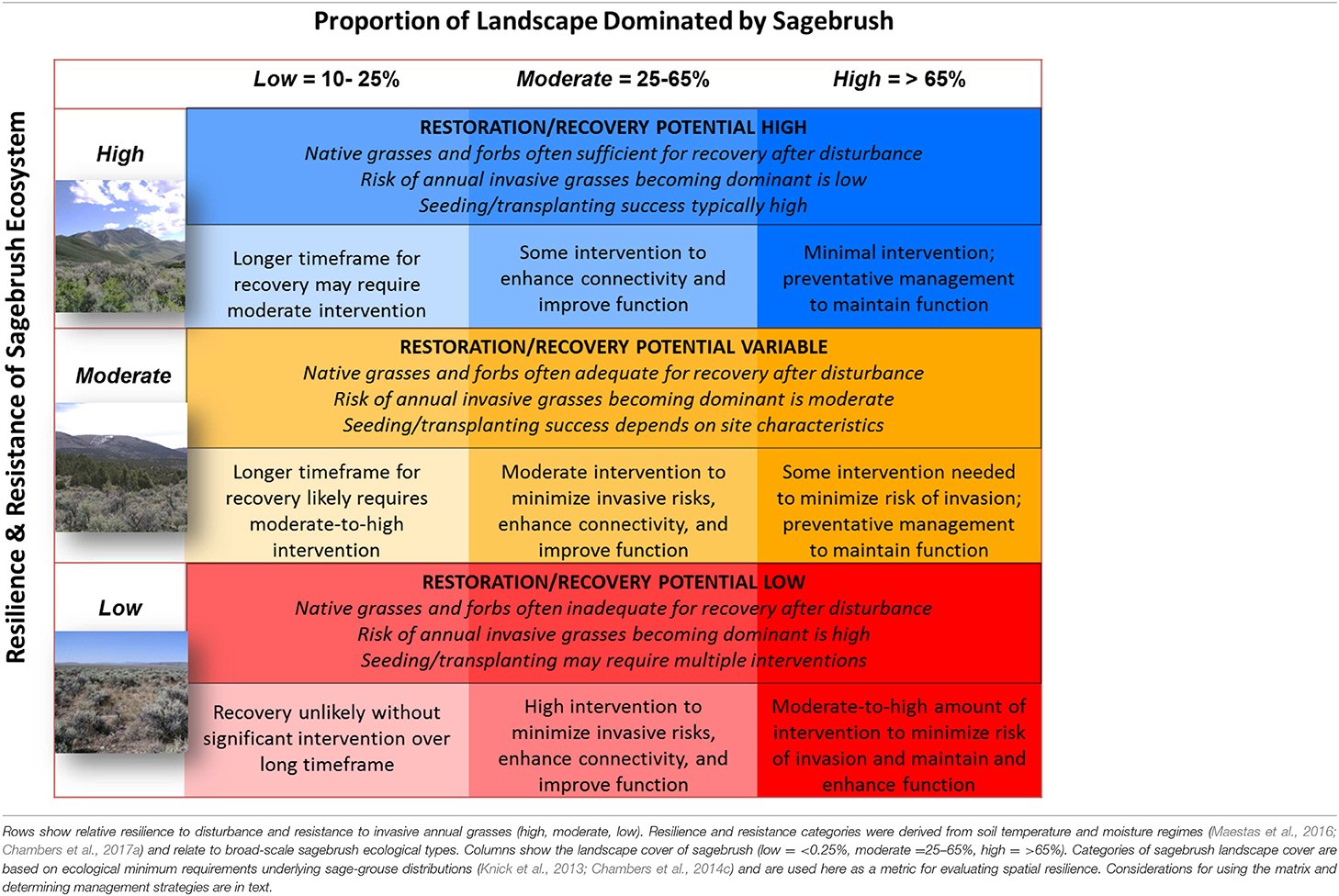
Table 3. Decision matrix for prioritizing areas for conservation and restoration investments and determining appropriate management strategies based on resilience concepts (Chambers et al., 2014a, 2017a)1.
The relative resilience to wildfire and resistance to invasive grasses strongly influences the response of an area to management strategies aimed at preventing or minimizing invasion and spread of non-native grasses and development of invasive grass-fire cycles (Chambers et al., 2014a,b, 2017a,c). Areas with high resilience and resistance often have the capacity to return to the prior or desired state with minimal investment following disturbances such as wildfire, while those with moderate resilience and resistance depend on both environment conditions and ecosystem attributes and require more detailed assessments to determine the most effective management strategies. Areas with low resilience and resistance are often among the most difficult to improve or restore and multiple management interventions may be required to obtain the desired state. In those areas where climate change effects are projected to be severe, management actions may need to help ecosystems transition to new climatic regimes (e.g., Millar et al., 2007; Halofsky et al., 2018a,b; Snyder et al., 2018).
The spatial resilience of an area is influenced by (1) resilience to disturbance and resistance to invasive grasses, which influence recovery potentials and the propensity to change states, and (2) anthropogenic developments, which fragment habitats, result in introductions of novel species, and can preclude return to prior states. An area with high landscape cover of sagebrush and high resilience to disturbance and resistance to invasive grasses may have relatively higher spatial resilience over time than one with low resilience to disturbance and resistance to invasive grasses. In contrast, an area with low landscape cover of sagebrush and high resilience to disturbance and resistance to invasive grasses may have similar spatial resilience to an area with low resilience to disturbance and resistance to invasive grasses if anthropogenic development, such as agriculture or oil and gas wells, is causing the loss of spatial resilience.
Areas with high landscape cover of sagebrush are high priorities for protective management across resilience and resistance categories, because they are more likely to be comprised of functioning ecosystems with relatively intact habitat and resource patches (Figures 12A,B) (Chambers et al., 2014b, 2017a,b). Areas with low resilience and resistance, high landscape cover of sagebrush, and high fire risk are among the highest priorities for protective management, because they have the highest risk of developing invasive grass-fire cycles and of undesirable state changes (Figure 13). In general, protective management strategies to reduce the risk of non-native grass invasions and altered fire regimes include: (1) reducing land use and new development and establishing conservation easements to minimize invasion vectors and corridors and human-caused fire starts; (2) ensuring that land uses and land treatments maintain or increase perennial native grasses and forbs—the plant functional types that enable recovery and compete with invasive annuals post-fire; (3) implementing early detection and rapid response strategies in areas at high risk of invasion or spread of invaders, and in association with developments and transportation/utility corridors (California Invasive Plant Council [Cal-IPC], 2012; Mealor et al., 2013) (4) prepositioning firefighting resources in areas of high fire risk, and managing fires to maintain resources an habitats; (5) implementing fuel treatments, including fuel breaks, in a manner that maintains or increases connectivity and prevents new invasions; and (6) seeding natives adapted to local conditions and a wide range of climatic conditions during post-fire restoration in areas where insufficient native perennials exist for unassisted recovery (Chambers et al., 2017a).
Areas with moderate landscape cover of sagebrush are often priorities for improving ecosystem functioning, habitat connectivity, and thus spatial resilience (Figures 12A,B, 13) (Chambers et al., 2014b, 2017a). Many of the same strategies apply as for areas with high landscape cover of sagebrush. Additional strategies may include: (1) implementing fuel treatments designed to increase connectivity and resilience to fire, such as removal of conifers expanding into sagebrush ecosystems; (2) thinning overly dense sagebrush stands and interseeding with perennial native grasses to improve habitat and increase resilience to fire (Huber-Sannwald and Pyke, 2005); (3) seeding or transplanting sagebrush and seeding a diverse mix of native species to reconnect intact habitats after wildfires (Pyke, 2011); and (4) managing livestock grazing and wild horse and burro numbers in a manner that increases treatment success. Consistent and repeated management interventions will likely be needed for these strategies to succeed in areas with low resilience and resistance, especially in areas with low to moderate cover of invasive forbs and grasses.
In areas with low landscape cover of sagebrush, the ability to increase spatial resilience and capacity to support desired resources and habitats may be limited by environmental factors, level of invasion, or amount of human development (Figures 12A,B, 13). These areas typically require higher levels of intervention over longer-timeframes. Where associated with high levels of invasion or human development, fire prevention, preparedness, and suppression may be high priorities. These areas are often sources of invasive plants and vectors for their spread (Gelbard and Belnap, 2003; Bradley, 2010) and of human-caused fire ignitions (Fusco et al., 2016). Management strategies include: (1) reducing fuels and suppressing fires to protect both human developments and remaining habitat; (2) using integrated weed management strategies (California Invasive Plant Council [Cal-IPC], 2012; Mealor et al., 2013); (3) educating stakeholders and the public about the risk of weed invasions and invasive grass-fire cycles as well as the importance of natural resources and species habitats; and (4) implementing restoration/rehabilitation activities designed to reduce the spread of invasive plants and decrease fire risk.
n topographically diverse sagebrush landscapes, resilience to disturbance, resistance to invasion, and spatial resilience often varies not only across ecoregions but also across planning and project areas. To step landscape scale assessments and prioritizations down to local scales, it is necessary to evaluate the specific conditions that exist within the area, develop the appropriate objectives, and determine the best management strategies using the highest resolution geospatial data available. Planning areas occur over continuums of environmental conditions, such as effective precipitation, have differing land use histories and species compositions (Johnstone et al., 2016), and may be projected to experience different climate change effects. They also have different resource and habitat values and socio-economic concerns and constraints. Careful assessment of these factors and of past responses to both disturbances and management treatments helps ensure that management strategies are implemented in a manner that will maximize conservation and restoration investments. Rigorous monitoring of management outcomes related to clearly defined objectives provides the scientific basis for adjusting policies or management actions in response to dynamic conditions.
Author Contributions
JC, MB, MG, and JM conceived the idea and developed the manuscript outline. JC led the writing of the manuscript. MB, MG, and JM contributed critically to the draft. DB, MJ, and BA contributed data and developed the map products. All authors gave final approval for publication.
Funding
Author support was provided by USDA Forest Service, Rocky Mountain Research Station, U.S. Geological Survey, and USDA Natural Resources Conservation Service.
Disclaimer
Any use of trade, firm, or product names is for descriptive purposes only and does not imply endorsement by the US Government.
Conflict of Interest Statement
The authors declare that the research was conducted in the absence of any commercial or financial relationships that could be construed as a potential conflict of interest.
Acknowledgments
We thank Stanley G. Kitchen, David A. Pyke, Edith B. Allen, and Eddie Van Etten for insightful and helpful reviews of this manuscript.
Footnotes
1. ^Article in production for Frontiers: Chambers, J. C., Allen, C. R., and Cushman, S. A. (2019). Operationalizing the concepts of resilience and resistance for managing species and ecosystems at risk. Front. Ecol. Evol.
2. ^Article in production for Frontiers: Bradford, J. B., Schlaepfer, D. R., Lauenroth, W. K., Palmquist, K. A., Chambers, J. C., Maestas, J. D., et al. (2019). 21st century changes in soil temperature and moisture regimes in North American drylands. Front. Ecol. Evol.
References
Abatzaglou, J. T., and Williams, A. P. (2016). Impact of anthropogenic climate change on wildfire across western US forests. PNAS 113, 11770–11775. doi: 10.1073/pnas.1607171113
Abatzoglou, J. T., and Kolden, C. A. (2013). Relationships between climate and macroscale area burned in the western United States. Inter. J. Wildland Fire. 22, 1003–1020. doi: 10.1071/WF13019
Agee, J. K. (1994). Fire and Weather Disturbances in Terrestrial Ecosystems of the Eastern Cascades. General Technical Reports PNW-GTR-320. Portland, OR: U.S. Department of Agriculture, Forest Service, Pacific Northwest Research Station.
Ager, A. A., Vaillant, N. M., and Finney, M. A. (2011). Integrating fire behavior models and geospatial analysis for wildland fire risk assessment and fuel management planning. J. Combust. 19:572452. doi: 10.1155/2011/572452
Aldridge, C. L., Nielsen, S. E., Beyer, H. L., Boyce, M. S., Connelly, J. W., Knick, S. T., et al. (2008). Range-wide patterns of greater sage-grouse persistence. Divers. Distrib. 14, 983–994. doi: 10.1111/j.1472-4642.2008.00502.x
Allen, C. R., Fontaine, J. J., Pope, K. L., and Gamestani, A. S. (2011). Adaptive management for a turbulent future. J. Env. Manag. 92, 1339–1345. doi: 10.1016/j.jenvman.2010.11.019
Allen, E. B., Rao, L. E., Steers, R. J., Bytnerowicz, A., and Fenn, M. E. (2009). “Impacts of atmospheric nitrogen deposition on vegetation and soils in Joshua Tree National Park,” in The Mojave Desert: Ecosystem Processes and Sustainability, eds R. H. Webb, L. F. Fenstermaker, J. S. Heaton, D. L. Hughson, E. V. McDonald, and D. M. Miller (Las Vegas, NV: University of Nevada), 78–100.
Anderson, K. E., Glenn, N. F., Spaete, L. P., Shinneman, D. J., Pilliod, D. S., Arkle, R. S., et al. (2018). Estimating vegetation biomass and cover across large plots in shrub and grass dominated drylands using terrestrial lidar and machine learning. Ecol. Indic. 84, 793–802. doi: 10.1016/j.ecolind.2017.09.034
Archibald, S., Roy, D. P., vanWilgen, B. W., and Scholes, R. J. (2009). What limits fire? An examination of drivers of burnt area in Southern Africa. Glob. Chang. Biol. 15, 613–630. doi: 10.1111/j.1365-2486.2008.01754.x
Arriaga, L., Castellanos, A. E., Moreno, E., and Jesús Alarcón. (2004). Potential ecological distribution of alien invasive species and risk assessment: a case study of buffel grass in arid regions of Mexico. Conserv. Biol. 18, 1504–1514. doi: 10.1111/j.1523-1739.2004.00166.x
Bajocco, S., and Ricotta, C. (2008). Evidence of selective burning in Sardinia (Italy): which land-cover classes do wildfires prefer? Landscape Ecol. 23, 241–248. doi: 10.1007/s10980-007-9176-5
Balch, J. K., Bradley, B. A., D'Antonio, C. M., and Gómez-Dans, J. (2013). Introduced annual grass increases regional fire activity across the arid western USA (1980–2009). Glob. Chang. Biol. 19, 173–183. doi: 10.1111/gcb.12046
Baskin, J. M., and Baskin, C. C. (1981). Ecology of germination and flowering in the weedy winter annual grass Bromus japonicus. J Range Manag. 34, 369–372.
Basnou, C., Iguzquiza, J., and Pino, J. (2015). Examining the role of landscape structure and dynamics in alien plant invasion from urban Mediterranean coastal habitats. Landscape Urban Plan. 136, 156–164. doi: 10.1016/j.landurbplan.2014.12.001
Blumenthal, D. M., Kray, J. A., Ortmans, W., Ziska, L. H., and Pendall, E. (2016). Cheatgrass is favored by warming but not CO2 enrichment in a semi-arid grassland. Glob. Chang. Biol. 22, 3026–3038. doi: 10.1111/gcb.13278
Bond, W. J., and Keeley, J. E. (2005). Fire as a global ‘herbivore': the ecology and evolution of flammable ecosystems. Trends Ecol. Evol. 20, 387–394. doi: 10.1016/j.tree.2005.04.025
Borchert, M. I., and Davis, F. W. (2018). “Central Coast Bioregion,” in Fire in California's Ecosystems, 2nd Edn, Revised, eds J. W. van Wagtendonk, N. G. Sugihara, S. L. Stephens, A. E. Thode, K. E. Shaffer, and J. Fites-Kaufman (Berkeley, CA: University of California Press), 299–318.
Boyd, C. S., Kerby, J. D., Svejcar, T. J., Bates, J. D., Johnson, D. D., et al. (2017). The sage-grouse habitat mortgage: effective conifer management in space and time. Rangeland Ecol. Manag. 70, 141–148. doi: 10.1016/j.rama.2016.08.012
Boyte, S., and Wylie, B. K. (2016). Near-real-time cheatgrass percent cover in the Northern Great Basin, USA, 2015. Rangelands 38, 278–284. doi: 10.1016/j.rala.2016.08.002
Bradford, J. B., and Lauenroth, W. K. (2006). Controls over invasion of Bromus tectorum: the importance of climate, soil, disturbance and seed availability. J. Veg. Sci. 17, 693–704. doi: 10.1111/j.1654-1103.2006.tb02493.x
Bradley, B. A. (2009). Regional analysis of the impacts of climate change on cheatgrass invasion shows potential risk and opportunity. Glob. Chang. Biol. 15, 196–208. doi: 10.1111/j.1365-2486.2008.01709.x
Bradley, B. A. (2010). Assessing ecosystem threats from global and regional change: Hierarchical modeling of risk to sagebrush ecosystems from climate change, land use and invasive species in Nevada, USA. Ecography 33, 198–208. doi: 10.1111/j.1600-0587.2009.05684.x
Bradley, B. A. (2014). Remote detection of invasive plants: a review of spectral, textural and phenological approaches. Biol. Invasions. 16:1411–1425. doi: 10.1007/s10530-013-0578-9
Bradley, B. A., Blumenthal, D. M., Wilcove, D. S., and Ziska, L. H. (2013). Predicting plant invasions in an era of global change. Trends Ecol. Evol. 25, 310–318. doi: 10.1016/j.tree.2009.12.003
Bradley, B. A., Curtis, C. A., and Chambers, J. C. (2016). “Chapter 9. Bromus response to climate and projected changes with climate change,” in Exotic Brome-grasses in Arid and Semiarid Ecosystems of the Western US: Causes, Consequences, and Management Implications, eds M. J. Germino, J. C. Chambers, and C. S. Brown (New York, NY: Springer: Series on Environmental Management), 257–274.
Bradley, B. A., Curtis, C. A., Fusco, E. J., Abatzoglou, J. T., Balch, J. K., Dadashi, S., et al. (2018). Cheatgrass (Bromus tectorum) distribution in the intermountain Western United States and its relationship to fire frequency, seasonality, and ignitions. Biol. Invasions. 20, 1493–1506. doi: 10.1007/s10530-017-1641-8
Bradstock, R. A. (2010). A biogeographic model of fire regimes in Australia: current and future implications. Glob. Ecol. Biogeogr. 19, 145–158. doi: 10.1111/j.1466-8238.2009.00512.x
Brooks, M. L. (2003). Effects of increased soil nitrogen on the dominance of alien annual plants in the Mojave Desert. J. Appl. Ecol. 40, 344–353. doi: 10.1046/j.1365-2664.2003.00789.x
Brooks, M. L. (2009). “Spatial and temporal distribution of non-native plants in upland areas of the Mojave Desert,” in The Mojave Desert: Ecosystem Processes and Sustainability, eds R. H. Webb, L. F. Fenstermaker, J. S. Heaton, D. L. Hughson, E. V. McDonald, and D. M. Miller (Las Vegas, NV: University of Nevada), 101–124.
Brooks, M. L., and Berry, K. H. (2006). Dominance and environmental correlates of alien annual plants in the Mojave Desert. J. Arid Environ. 67, 100–124. doi: 10.1016/j.jaridenv.2006.09.021
Brooks, M. L., Brown, C. S., Chambers, J. C., D'Antonio, C. M., Keeley, J. E., and Belnap, J. (2016). “Exotic annual Bromus invasions: comparisons among species and ecoregions in the Western United States,” in Exotic Brome-grasses in Arid and Semiarid Ecosystems of the Western US: Causes, Consequences, and Management Implications. eds M. J. Germino, J. C. Chambers, C. S. Brown (New York, NY: Springer: Series on Environmental Management), 11–60.
Brooks, M. L., D'Antonio, C. M., Richardson, D. M., Grace, J. B., Keeley, J. E., DiTomaso, et al. (2004). Effects of invasive alien plants on fire regimes. Bioscience 54, 677–688. doi: 10.1641/0006-3568(2004)054[0677:EOIAPO]2.0.CO;2
Brooks, M. L., Esque, T. C., and Duck, T. (2007). “Creosotebush, blackbrush, and interior chaparral shrublands,” in Fire Ecology and Management of the Major Ecosystems of Southern Utah, eds S. Hood and M. Miller (Fort Collins, CO: U.S. Department of Agriculture, Forest Service, Rocky Mountain Research Station, RMRS-GTR-202), 97–110.
Brooks, M. L., and Matchett, J. R. (2006). Spatial and temporal patterns of wildfires in the Mojave Desert, 1980–2004. J. Arid Environ. 67, 148–164. doi: 10.1016/j.jaridenv.2006.09.027
Brooks, M. L., Minnich, R., and Matchett, J. R. (2018). “Southeastern Deserts Bioregion,” in Fire in California's Ecosystems, 2nd Edition, Revised, eds. J. W. van Wagtendonk, N. G. Sugihara, S. L. Stephens, A. E. Thode, K. E. Shaffer, and J. Fites-Kaufman (Berkeley, CA: University of California Press), 353–378.
Brooks, M. L., and Pyke, D. (2001). “Invasive plants and fire in the deserts of North America,” in Proceedings of the Invasive Species Workshop: The Role of Fire In the Control and Spread of Invasive Species. Fire Conference 2000: The First National Congress on Fire Ecology, Prevention and Management, eds K. Galley and T. Wilson (Tallahassee, FL: Tall Timbers Research Station, Misc. Publ. No. 11), 1–14.
Bykova, O., and Sage, R. F. (2012). Winter cold tolerance and the geographic range separation of Bromus tectorum and Bromus rubens, two severe invasive species in North America. Glob. Chang. Biol. 18, 3654–3663. doi: 10.1111/gcb.12003
California Invasive Plant Council [Cal-IPC] (2012). Preventing the Spread of Invasive Plants: Best Management Practices for Land Managers, 3rd Edn. Cal-IPC Publication 2012-03. Berkeley, CA: California Invasive Plant Council.
California Invasive Plant Council [Cal-IPC]. (2018). Plants A to Z. California Invasive Plant Council. Available online at: https://www.cal-ipc.org (accessed May 15, 2019).
Campagnoni, A., and Adler, P. B. (2014). Warming, competition, and Bromus tectorum population growth across an elevation gradient. Ecosphere 5:121. doi: 10.1890/ES14-00047.1
Catchpole, W. (2002). “Fire properties and burn patterns in heterogeneous landscapes,” in Flammable Australia: The Fire Regimes and Biodiversity of a Continent, eds R.A. Bradstock, J. E. Williams and A. M. Gill (Cambridge: Cambridge University Press), 50–75.
Chambers, J. C., Beck, J. L., Bradford, J. B., Bybee, J., Campbell, S., Carlson, J., et al. (2017a). Science Framework for Conservation and Restoration of the Sagebrush Biome: Linking the Department of the Integrated Rangeland Fire Management Strategy to Long-Term Strategic Conservation Actions. Part One. Science Basis and Applications. RMRS-GTR-360. Fort Collins, CO: U.S Department of Agriculture, Forest Service, Rocky Mountain Research Station, P. 213.
Chambers, J. C., Board, D. I., Roundy, B. A., and Weisberg, P. J. (2017b). Removal of perennial herbaceous species affects response of cold desert shrublands to fire. 28., 975–984 J. Veg. Science. doi: 10.1111/jvs.12548
Chambers, J. C., Bradley, B. A., Brown, C. S., D'Antonio, C., Germino, M. J., Grace, J. B., et al. (2014a). Resilience to stress and disturbance, and resistance to Bromus tectorum L. invasion in the cold desert shrublands of western North America. Ecosystems 7, 360–375. doi: 10.1007/s10021-013-9725-5
Chambers, J. C., Germino, M. J., Belnap, J., Brown, C. S., Schupp, E. W., and St. Clair, S. B. (2016). “Plant community resistance to invasion by Bromus species—The role of community attributes,” in Exotic Brome-Grasses in Arid and Semiarid Ecosystems of the Western US: Causes, Consequences, and Management Implications, eds M. J. Germino, J. C. Chambers, C. S. Brown (New York, NY: Springer: Series on Environmental Management), 275–306.
Chambers, J. C., Maestas, J. D., Pyke, D. A., Boyd, C., Pellant, M., and Wuenschel, A. (2017c). Using resilience and resistance concepts to manage persistent threats to sagebrush ecosystems and Greater sage-grouse. Rangeland Ecol. Manag. 70, 149–164. doi: 10.1016/j.rama.2016.08.005
Chambers, J. C., Miller, R. F., Board, D. I., Pyke, D. A., Roundy, B. A., Grace, J. B., et al. (2014b). Resilience and resistance of sagebrush ecosystems: implications for state and transition models and management treatments. Rangeland Ecol. Manag. 67, 440–454. doi: 10.2111/REM-D-13-00074.1
Chambers, J. C., Pyke, D. A., Maestas, J. D., Pellant, M., Boyd, C. S., Campbell, S. B., et al. (2014c). Using Resistance and Resilience Concepts to Reduce Impacts of Invasive Annual Grasses and Altered Fire Regimes on the Sagebrush Ecosystem and Greater Sage-Grouse: A Strategic Multi-scale Approach. RMRS-GTR-326. Fort Collins, CO: U.S Department of Agriculture, Forest Service, Rocky Mountain Research Station, 73.
Chambers, J. C., Roundy, B. A., Blank, R. R., Meyer, S. E., and Whittaker, A. (2007). What makes great basin sagebrush ecosystems invasible by Bromus tectorum? Ecol. Monogr. 77, 117–145. doi: 10.1890/05-1991
Chuvieco, E., Aguado, I., Jurdao, S., Pettinari, M. L., Yebra, M., Salas, J., et al. (2014). Integrating geospatial information into fire risk assessment. Inter. J. Wildland Fire 23, 606–619. doi: 10.1071/WF12052W
Collins, B. M., Stephens, S.L., Moghaddas, J.J., and Battles, J. (2010). Challenges and approaches in planning fuel treatments across fire-excluded forested landscapes. J. For. 108, 24–31. doi: 10.1093/jof/108.1.24
Condon, L., Weisberg, P. L., and Chambers, J. C. (2011). Abiotic and biotic influences on Bromus tectorum invasion and Artemisia tridentata recovery after fire. Inter. J. Wildland Fire. 20, 1–8. doi: 10.1071/WF09082
Corbin, J., Dyer, A., and Seabloom, E. (2007). “Competitive interactions,” in California Grasslands: Ecology and Management, eds M. R. Stromberg, J. D. Corbin, and C. M. D'Antonio (Berkely, CA: University of California Press), 156–168.
Crimmins, M. A., and Comrie, A. C. (2004). Interactions between antecedent climate and wildfire variability across southeastern Arizona. Int. J. Wildland Fire. 13, 455–466. doi: 10.1071/WF03064
Curtin, C. G., and Parker, J. P. (2014). Foundations of resilience thinking. Conserv. Biol. 28, 912–923. doi: 10.1111/cobi.12321
D'Antonio, C. M., and Thomsen, M. (2004). Ecological resistance in theory and practice. Weed Technol. 18, 1572–1577. doi: 10.1614/0890-037X(2004)018[1572:ERITAP]2.0.CO;2
D'Antonio, C. M., and Vitousek, P. M. (1992). Biological invasions by exotic grasses, the grass/fire cycle, and global change. Ann. Rev. Ecol. Syst. 23, 63–87. doi: 10.1146/annurev.es.23.110192.000431
Davies, G. M., Bakker, J. D., Dettweiler-Robinson, E., Dunwiddie, P. W., Hall, S. A., and Downs, J. (2012). Trajectories of change in sagebrush-steppe vegetation communities in relation to multiple wildfires. Ecol. Appl. 22, 1562–1577. doi: 10.1890/10-2089.1
Davis, M. A., Grime, J. P., and Thompson, K. (2000). Fluctuating resources in plant communities: a general theory of invasibility. J. Ecol. 88, 528–534. doi: 10.1046/j.1365-2745.2000.00473.x
Davis, M. A., and Pelsor, M. (2001). Experimental support for a resource-based mechanistic model of invasibility. Ecol. Lett. 4, 421–428. doi: 10.1046/j.1461-0248.2001.00246.x
Dettweiler-Robinson, E, Bakker, J. D., and Grace, J. B. (2013). Controls of biological soil crust cover and composition shift with succession in sagebrush shrub-steppe. J. Arid Environ. 94, 96–104. doi: 10.1016/j.jaridenv.2013.01.013
Dobrowski, S. Z., Abatzoglou, J., Swanson, A. K., Greenberg, J. A., Mynsberge, A. R., Holden, Z. A., et al. (2013). The climate velocity of the contiguous United States during the 20th century. Global Change Biol. 19, 241–251. doi: 10.1111/gcb.12026
Doherty, K. E., Evans, J. S., Coates, P. S., Juliusson, L. M., and Fedy, B. C. (2016). Importance of regional variation in conservation planning: a rangewide example of the Greater Sage-Grouse. Ecosphere 7:e01462. doi: 10.1002/ecs2.1462
Dudley, T.L. (2009). Invasive plants in Mojave Desert riparian areas,” in The Mojave Desert: Ecosystem Processes and Sustainability, eds R. H. Webb, L. F. Fenstermaker, J. S. Heaton, D. L. Hughson, E. V. McDonald, and D. M. Miller (Las Vegas, NV: University of Nevada), 125–155.
Finney, M. A., McHugh, C. W., Grenfell, I. C., Riley, K. L., and Short, K. C. (2011). A simulation of probabilistic wildfire risk components for the continental United States. Stoch. Environ. Res. Risk Assess. 25, 973–1000. doi: 10.1007/s00477-011-0462-z
Floyd, M. L., Hanna, D., and Romme, W. H. (2006). Historical and recent fire regimes in piñon-juniper woodlands on Mesa Verde, Colorado, USA. Forest Ecol. Manag. 198, 269–289. doi: 10.1016/j.foreco.2004.04.006
Folke, C., Carpenter, S. R., Walker, B., Scheffer, M., Chapin, R., and Rockström, J. (2010). Resilience thinking: integrating resilience, adaptability and transformability. Ecol. Soc. 15:20. Available online at: http://www.ecologyandsociety.org/vol15/iss4/art20/
Friedel, M. H., Puckey, H., O'Malley, C., Waycott, M., Smyth, A.nita, and Miller, G. (2006). Buffel Grass: Both Friend and Foe: An Evaluation of the Advantages and Disadvantages of Buffel Grass Use, and Recommendations for Future Research: A Report to the Desert Knowledge Cooperative Research Centre on the Dispersal, Impact and Management of Buffel Grass (Cenchrus ciliaris) in Desert Australia. Alice Springs, NT: Desert Knowledge, CRC Press.
Fusco, E. J., Abatzoglou, J. T., Balch, J. K., Finn, J. T., and Bradley, B. A. (2016). Quantifying the human influence on fire ignition across the western USA. Ecol. Appl. 26, 2390–2401. doi: 10.1002/eap.1395
Gelbard, J. L., and Belnap, J. (2003). Roads as conduits for exotic plant invasions in a semiarid landscape. Conserv. Biol. 17, 420–432. doi: 10.1046/j.1523-1739.2003.01408.x
Geo MAC Wildland Fire Support. (2018). Geospatial Multi-Agency Coordination. Available online at: https://www.geomac.gov (accessed May 15, 2019).
Germino, M. J., Belnap, J., Stark, J. M., Allen, E. B., and Rau, B. M. (2016). “Ecosystem impacts of exotic annual invaders in the genus Bromus,” in Exotic Brome-grasses in Arid and Semiarid Ecosystems of the Western US: Causes, Consequences, and Management Implications, eds M. J. Germino, J. C. Chambers, C. S. Brown (New York, NY: Springer: Series on Environmental Management), 61–98.
Gómez-González, S., Torres-Díaz, C., Valencia, G., Torres-Morales, P., Cavieres, L. A., and Pausas, J. G. (2011). Anthropogenic fires increase alien and native annual species in the chilean coastal matorral. Divers. Distrib. 17, 58–67. doi: 10.1111/j.1472-4642.2010.00728.x
González-Moreno, P., Diez, J. M., Ibáñez, I., Font, X., and Vilà, M. (2014). Plant invasions are context-dependent: multiscale effects of climate, human activity and habitat. Divers. Distrib. 20, 720–731. doi: 10.1111/ddi.12206
Griffin, G., Price, N. F., and Portlock, H. F. (1983). Wildfires in the central Australian rangelands 1970–1980. J. Environ. Manag. 17, 311–323.
Halofsky, J. E., Peterson, D. L., Dante-Wood, S. K., Hoang, L., Ho, J. J., and Joyce, L. A. (2018a). Climate Change Vulnerability and Adaptation in the Northern Rocky Mountains, Parts 1 and 2. General Technical Reports. RMRS GTR- 374. Fort Collins, CO: U.S. Department of Agriculture, Forest Service, Rocky Mountain Research Station, 475.
Halofsky, J. E., Peterson, D. L., Ho, J. J., Little, N., and Joyce, L. A. (2018b). Climate Change Vulnerability and Adaptation in the Intermountain Region, Parts 1 and 2. General Technical Reports RMRS-GTR-375. Fort Collins, CO: U.S. Department of Agriculture, Forest Service, Rocky Mountain Research Station, 509.
Hanser, S. E., Leu, M., Knick, S. T., and Aldridge, C. L. (2011). Sagebrush Ecosystem Conservation and Management: Ecoregional Assessment Tools and Models for the Wyoming Basins. Lawrence, KS: Allen Press.
He, K. S., Bradley, B. A., Cord, A. F., Rocchini, D., Tuanmu, M., Schmidtlein, S., et al. (2015). Will remote sensing shape the next generation of species distribution models? Rem. Sens. Ecol. Conserv. 1, 4–18. doi: 10.1002/rse2.7
Heady, H. F. (1977). “Valley grassland,” in Terrestrial Vegetation of California, eds M. G. Barbourr, and J. Major (New York, NY: Wiley-Interscience), 491–514.
Hirota, M., Holmgren, M., Van Nes, E. H., and Scheffer, M. (2011). Global resilience of tropical forest and savanna to critical transitions. Science 334, 232–235. doi: 10.1126/science.1210657
Holl, K. D., and Aide, T. M. (2011). When and where to actively restore ecosystems? Forest Ecol. Manag. 261, 1558–1563. doi: 10.1016/j.foreco.2010.07.004
Holling, C. S. (1973). Resilience and stability of ecological systems. Annu. Rev. Ecol. Syst. 4, 1–23.
Holling, C. S., and Meffe, G. K. (1996). Command and control and the pathology of natural resource management. Conserv. Biol. 10, 28–337. doi: 10.1046/j.1523-1739.1996.10020328.x
Huang, C. Y., and Geiger, E. L. (2008). Climate anomalies provide opportunities for large-scale mapping of non-native plant abundance in desert grasslands. Diversity Distrib. 14, 875–884. doi: 10.1111/j.1472-4642.2008.00500.x
Huber-Sannwald, E., and Pyke, D. A. (2005). Establishing native grasses in a big sagebrush–dominated site: an intermediate restoration step. Restor. Ecol. 13, 292–301. doi: 10.1111/j.1526-100X.2005.00037.x
Hulet, A., Boyd, C. S., Davies, K. W., and Svejcar, T. J. (2015). Prefire (preemptive management to decrease fire-induced bunchgrass mortality and reduce reliance on postfire seeding. Rangeland Ecol. Manag. 68, 437–444. doi: 10.1016/j.rama.2015.08.001
Jiménez-Valverde, A., Peterson, A. T., Soberón, J., Overton, J. M., Aragón, P., and Lobo, J. M. (2011). Use of niche models in invasive species risk assessments. Biol. Invasions. 13, 2785–2797. doi: 10.1007/s10530-011-9963-4
Johnstone, J. F., Allen, C. D., Franklin, J. F., Frelich, L. E., Harvey, B. J., Higuera, P. E., et al. (2016). Changing disturbance regimes, ecological memory, and forest resilience. Front. Ecol. Environ. 14, 369–378. doi: 10.1002/fee.1311
Jones, M. O., Allred, B. W., Naugle, D. E., Maestas, J. D., Donnelly, P., et al. (2018). Innovation in rangeland monitoring: annual, 30 m, plant functional type percent cover maps for U.S. rangelands, 1984–2017. Ecosphere 9:e02430. doi: 10.1002/ecs2.2430
Keeley, J. E., and Syphard, A. D. (2018). “South Coast Bioregion,” in Fire in California's Ecosystems, 2nd Edn, Revised, eds J. W. van Wagtendonk, N. G. Sugihara, S. L. Stephens, A. E. Thode, K. E. Shaffer, and J. Fites-Kaufman (Berkeley, CA: University of California Press), 319–352.
Klinger, R. C., and Brooks, M. L. (2017). Alternative pathways to landscape transformation: invasive grasses, burn severity and fire frequency in arid ecosystems. J. Ecol. 105, 1521–1533. doi: 10.1111/1365-2745.12863
Klinger, R. C., Brooks, M. L., Frakes, N., Matchett, J. R., and McKinley, R. (2011). “Chapter 6: Vegetation trends following the 2005 Southern Nevada Complex Fire,” in Southern Nevada Complex Emergency Stabilization and Rehabilitation Final Report, eds K. L. Bauer, M. L. Brooks, L. A. DeFalco, L. Derasary, K. Drake, N. Frakes, et.al (Ely, NV: U.S. Department of the Interior, Bureau of Land Management), 118–194.
Klinger, R. C., Brooks, M. L., and Randall, J. M. (2018). “Fire and invasive plants,” in Fire in California's Ecosystems: 2nd Edn, Revised, eds J. W. van Wagtendonk, N. G. Sugihara, S. L. Stephens, A. E. Thode, K. E. Shaffer, and J. Fites-Kaufman (Berkeley, CA: University of California Press), 459–476.
Knick, S. T., Hanser, S. E., Miller, R. F., Pyke, D. A., Wisdom, M. J., and Finn, S. P. (2011). “Ecological influence and pathways of land use in sagebrush,” in Greater Sage-Grouse—Ecology and Conservation of a Landscape Species and Its Habitats, Studies in Avian Biology 38. eds S. T. Knick and J. W. Connelly (Berkeley, CA: University of California Press), 203–251.
Knick, S. T., Hanser, S. E., and Preston, K. L. (2013). Modeling ecological minimum requirements for distribution of greater sage-grouse leks: implications for population connectivity across their western range, U.S.A. Ecol. Evol. 3, 1539–1551. doi: 10.1002/ece3.557
Knight, D. H., Jones, G. P., Reiners, W. A., and Romme, W. H. (2014). Mountains and Plains: The Ecology of Wyoming landscapes, 2nd Edn. New Haven, CT: Yale University Press, 404.
Larson, C. D., Lehnhoff, E. A., and Rew, L. J. (2017). A warmer and drier climate in the northern sagebrush biome does not promote cheatgrass invasion or change its response to fire. Oecologia 185, 763–774. doi: 10.1007/s00442-017-3976-3
Leffler, A. J., and Ryel, R. J. (2012). “Resource pool dynamics: conditions that regulate species interactions and dominance,” in Invasive Plant Ecology and Management. Linking Processes to Practice, eds T. A. Monaco and R. L. Sheley (Cambridge, MA: CAB International), 57–78.
Leu, M., Hanser, S. E., and Knick, S. T. (2011). The human footprint in the West: a large-scale analysis of anthropogenic impacts. Ecol. Appl. 18, 1119–1139. doi: 10.1890/07-0480.1
Levine, N. M., Zang, K., Longo, M., Baccini, A., Phillips, O. L., Lewis, S. L., et al. (2016). Ecosystem heterogeneity determines the ecological resilience of the Amazon to climate change. PNAS 113, 793–797. doi: 10.1073/pnas.1511344112
Link, S. O., Keeler, C. W., Hill, R. W., and Hagen, E. (2006). Bromus tectorum cover mapping and fire risk. Inter. J. Wildland Fire 15, 113–119. doi: 10.1071/WF05001
Littell, J. S., McKenzie, D., Peterson, D. L., and Westerling, A. L. (2009). Climate and wildfire area burned in the western U.S. ecoprovinces, 1916-2003. Ecol. Appl. 19, 1003–1021. doi: 10.1890/07-1183.1
Maestas, J. D., Campbell, S. B., Chambers, J. C., Pellant, M., and Miller, R. F. (2016). Tapping soil survey information for rapid assessment of sagebrush ecosystem resilience and resistance. Rangelands 38, 120–128. doi: 10.1016/j.rala.2016.02.002
Marcot, B. G., Thompson, M. P., Runge, M. C., Thompson, F. R., McNulty, S., Cleaves, D., et al. (2012) Recent advances in applying decision science to managing national forests. Forest Ecol. Manag. 285, 123–132. doi: 10.1016/j.foreco.2012.08.024
Marshal, V. M., Lewis, M. M., and Ostendorf, L. B. (2012). Buffel grass (Cenchrus ciliaris) as an invader and threat to biodiversity in arid environments: a review. J. Arid Environ. 78, 1–12. doi: 10.1016/j.jaridenv.2011.11.005
McIntyre, N. E., Wright, C. K, Swain, S., Hayhoe, K., Liu, G., Schwartz, F. W., et al. (2014). Climate forcing of wetland landscape connectivity in the Great Plains. Front. Ecol. Environ. 12, 59–64. doi: 10.1890/120369
Mealor, B. A., Mealor, R. D., Kelley, W. K., Bergman, W. K., Bergman, D. L., Burnett, S. A., et al. (2013). Cheatgrass Management Handbook: Managing an Invasive Annual Grass in the Rocky Mountain Region. Laramie, WY: University of Wyoming; Fort Collins, CO: Colorado State University, 131.
Mermoz, M., Kitzberger, T., and Veblen, T. T. (2005). Landscape influence on occurrence and spread of wildfires in Patagonian forests and shrublands. Ecology, 86, 2705–2715. doi: 10.1890/04-1850
Meyer, S. E., Garvin, S. C., and Beckstead, J. (2001). “Factors mediating cheatgrass invasion of intact salt desert shrubland,” in Shrubland Ecosystem Genetics and Biodiversity: Proceedings, eds D. E. McArthur and D. J. Fairbanks (Ogden, UT: U.S. Department of Agriculture, Forest Service, Rocky Mountain Research Station, Proc RMRS-P-21), 224–232.
Millar, C. I., Stephenson, N. L., and Stephens, S. L. (2007). Climate change and forests of the future: managing in the face of uncertainty. Ecol. Appl. 17, 2145–2151. doi: 10.1890/06-1715.1
Miller, C., and Urban, D. L. (2000). Connectivity of forest fuels and surface fire regimes. Landscape Ecol. 15, 145–154. doi: 10.1023/A:1008181313360
Miller, G., Friedel, M., Adam, P., and Chewings, V. (2010). Ecological impacts of buffel grass (Cenchrus ciliaris L.) invasion in central Australia – does field evidence support a fire-invasion feedback? Rangeland J. 32, 353–365. doi: 10.1071/RJ09076
Miller, R. F., Chambers, J. C., Pyke, D. A., Pierson, F. B., and Williams, J. C. (2013). Fire Effects on Vegetation and Soils in the Great Basin Region and the Role of Site Characteristics. General Technical Reports RMRS-GTR-308. Fort Collins, CO: U.S. Department of Agriculture, Forest Service, Rocky Mountain Research Station, 136.
Miller, R. F., Knick, S. T., Pyke, D. A., Meinke, C. W., Hanser, S. E., Wisdom, M. J., et al. (2011). “Characteristics of sagebrush habitats and limitations to long-term conservation,” in Greater Sage-Grouse—Ecology and Conservation of a Landscape Species and Its Habitats, Studies in Avian Biology 38, eds S. T. Knick and J. W Connelly (Berkeley, CA: University of California Press), 145–185.
Minnich, R. A. (2001). An integrated model of two fire regimes. Conserv. Biol. 15, 1549–1553. doi: 10.1046/j.1523-1739.2001.01067.x
Monitoring Trends in Burn Severity [MTBS]. (2018). A Multiagency Program Designed to Consistently Map the Burn Severity and Perimeters of Fires Across all Lands of the United States from 1984 and beyond. Available online at: https://www.mtbs.gov/ (accessed May 15, 2019).
Moreira, F., Viedma, O., Arianoutsou, M., Curt, T., Koutsias, N., Rigolot, E., et al. (2011). Landscape wildfire interactions in southern Europe: implications for landscape management. J. Environ. Manag. 92, 2389–2402. doi: 10.1016/j.jenvman.2011.06.028
National Land Cover Database [NLCD]. (2014). Percent Developed Imperviousness 2014. 2011 Edition, amended 2014. Metadata. Sioux Falls, SD: U.S. Department of the Interior, U.S. Geological Survey.
Nowak, R. S., Ellsworth, D. S., and Smith, S. D. (2004). Functional responses of plants to elevated atmospheric CO2: do photosynthetic and productivity data from FACE experiments support early predictions? New Phyt. 162, 253–280. doi: 10.1111/j.1469-8137.2004.01033.x
Olsson, A. D., Betancourt, J., McClaran, M. P., and Marsh, S. E. (2012). Sonoran Desert Ecosystem transformation by a C4 grass without the grass/fire cycle. Divers. Distrib. 18, 10–21. doi: 10.1111/j.1472-4642.2011.00825.x
Paruelo, J. M., and Lauenroth, W. (1996). Relative abundance of plant functional types in grasslands and shrublands of North America. Ecol. Appl. 6, 1212–1224. doi: 10.2307/2269602
Pausas, J. G., and Bradstock, R. A. (2007). Fire persistence traits of plants along a productivity and disturbance gradient in Mediterranean shrublands of south-east Australia. Glob. Ecol. Biogeogr. 16, 330–340. doi: 10.1111/j.1466-8238.2006.00283.x
Pausas, J. G., and Keeley, J. E. (2014). Abrupt climate-independent fire regime changes. Ecosystems 17, 1109–1120. doi: 10.1007/s10021-014-9773-5
Peters, D. P., Pielke, R. A., Bestelmeyer, B. T., Allen, C. D., Munson-McGee, S., and Havstad, K. M. (2004). Cross-scale interactions, nonlinearities, and forecasting catastrophic events. Proc. Nat. Acad. Sci. U S A. 101, 15130–15135. doi: 10.1073/pnas.0403822101
Pilliod, D. S., Welty, J. L., and Arkle, R. S. (2017). Refining the cheatgrass–fire cycle in the Great Basin: precipitation timing and fine fuel composition predict wildfire trends. Ecol. Evol. 7, 8126–8151. doi: 10.1002/ece3.3414
Prevey, J. S., Germino, M. J., Huntley, N. J., and Inouye, R. S. (2010). Exotic plants increase and native plants decrease with loss of foundation species in sagebrush steppe. Plant Ecol. 201, 39–51. doi: 10.1007/s11258-009-9652-x
PRISM Climate Group (2016). Oregon State University. Available online at: http://prism.oregonstate.edu (accessed May 15, 2019).
Pyke, D. A. (2011). “Restoring and rehabilitating sagebrush habitats,” in Greater Sage-Grouse—Ecology and Conservation of a Landscape Species and Its Habitats, Studies in Avian Biology 38. eds S. T. Knick and J. W. Connelly (Berkeley, CA: University of California Press), 531–548.
Rao, L. E., and Allen, E. B. (2010). Combined effects of precipitation and nitrogen deposition on native and invasive winter annual production in California deserts. Oecologia. 162, 1035–1046. doi: 10.1007/s00442-009-1516-5
Rao, L. E., Matchett, J. R., Brooks, M. L., Johnson, R. F., Minnich, R. A., and Allen, E. B. (2014). Relationships between annual plant productivity, nitrogen deposition and fire size in low-elevation California desert scrub. Int. J. Wildland Fire. 24, 48–58. doi: 10.1071/WF13152
Rappaport, D. I., Tambosi, L. R., and Metzger, J. P. (2015). A landscape triage approach: combining spatial and temporal dynamics to prioritize restoration and conservation. J. Appl. Ecol. 52, 590–601. doi: 10.1111/1365-2664.12405
Reisner, M. D., Grace, J. B., Pyke, D. A., and Doescher, P. S. (2013). Conditions favouring Bromus tectorum dominance of endangered sagebrush steppe ecosystems. J. Appl. Ecol. 50, 1039–1049. doi: 10.1111/1365-2664.12097
Rejmanek, M. (1989). “Invasibility of plant communities,” in Ecology of Biological Invasion: A Global Perspective, eds J. A. Drake, F. Di Castri, R. H., Groves, F. J. Kruger, H. A. Mooney, M. Rejmanek, et al. (New York, NY, Wiley and Sons), 369–388.
Ricca, M. A., Coates, P. S., Gustafson, K. B., BruPee, B. E., Chambers, J. C., Lisius, S., et al. (2018). Using indices of species distribution, resilience, and resistance as an ecological currency for conservation planning of Greater sage-grouse. Ecol. Appl. 28, 878–896. doi: 10.1002/eap.1690
Rossiter, N. A., Setterfield, S. A., Douglas, M. M., and Hutley, L. B. (2003). Testing the grass-fire cycle: alien grass invasion in the tropical savannas of northern Australia. Divers. Distrib. 9, 169–176. doi: 10.1046/j.1472-4642.2003.00020.x
Roundy, B. A., Chambers, J. C., Pyke, D. A., Miller, R. F., Tausch, R. J., Schupp, E. W., et al. (2018). Resilience and resistance in sagebrush ecosystems are associated with seasonal soil temperature and water availability. Ecosphere 9:02417. doi: 10.1002/ecs2.2417
Rowland, M. M., Wisdom, M. J., Suring, L. H., and Meinke, C. W. (2006). Greater sage-grouse as an umbrella species for sagebrush-associated vertebrates. Biol. Conserv. 129 , 323–335. doi: 10.1016/j.biocon.2005.10.048
Rudnick, D. A., Ryan, S. J., Beier, P., Cushman, S. A., Dieffenback, F., Epps, C. W., et al. (2012). The Role of Landscape Connectivity in Planning and Implementing Conservation and Restoration priorities. Report No. 16. Ecological Society of America (Washington, DC).
Sala, O., Lauenroth, W., and Golluscio, R. (1997). “Plant functional types in temperate semi-arid regions,” in Plant Functional Types, eds. T. M. Smith and F. I. Woodward (Cambridge, MA: Cambridge University Press), 217–233.
Salo, L. F. (2005). Red brome (Bromus rubens subsp. madritensis) in North America: possible modes for early introductions, subsequent spread. Biol. Invasions. 7, 165–180. doi: 10.1007/s10530-004-8979-4
Scott, J. H., Thompson, M. P., and Calkin, D. E. (2013). A Wildfire Risk Assessment Framework for Land and Resource Management. General Technical Reports RMRS-GTR-315. Fort Collins, CO: U.S. Department of Agriculture, Forest Service, Rocky Mountain Research Station, 83.
Seidl, R., Spies, T. A., Peterson, D. L., Stephens, S. L., and Hick, J. A. (2016). Searching for resilience: addressing the impacts of changing disturbance regimes on forest ecosystem services. J. Appl. Ecol. 53, 120–129. doi: 10.1111/1365-2664.12511
Shea, K., and Chesson, P. (2002). Community ecology theory as a framework for biological invasions. Trends Ecol. Evol. 17, 170–176. doi: 10.1016/S0169-5347(02)02495-3
Shinneman, D. J., and Baker, W. L. (2009). Environmental and climatic variables as potential drivers of post-fire cover of cheatgrass (Bromus tectorum) in seeded and unseeded semiarid ecosystems. Inter. J. Wildland Fire. 18, 191–202. doi: 10.1071/WF07043
Short, K. C., Finney, M. A., Scott, J. H., Gilbertson-Day, J. W., and Grenfell, I. C. (2016). Spatial Dataset of Probabilistic Wildfire Risk Components for the Conterminous United States. Forest Service Research Data Archive. Fort Collins, CO: U.S. Department of Agriculture, Forest Service, Rocky Mountain Research Station.
Simberloff, D., and Von Holle, B. (1999). Positive interactions of nonindigenous species: invasional meltdown? Biol. Invasions 1, 21–32.
Snyder, K. A., Evers, L., Chambers, J. C., Dunham, J., Bradford, J. G., and Loik, M. E. (2018). Effects of changing climate on the hydrological cycle in Cold Desert ecosystems of the Great Basin and Columbia Plateau. Rangeland Ecol. Manag. 72, 1–12. doi: 10.1016/j.rama.2018.07.007
Spasojevic, M. J., Bahlai, C. S., Bradley, B. A., Butterfield, J., Tuanmu, M. M., STla, S., et al. (2016). Scaling up the diversity–resilience relationship with trait databases and remote sensing data: the recovery of productivity after wildfire. Glob. Chang. Biol. 22, 1421–1432. doi: 10.1111/gcb.13174
Stavros, E. N., Abatzaglou, J. T., Mckenzie, D., and Larkin, N. K. (2014). Regional projections of the likelihood of very large wildland fires under a changing climate in the contiguous Western United States. Climate Change. 126, 455–468. doi: 10.1007/s10584-014-1229-6
Strand, E. K., Launchbaugh, K. L., Limb, R. F., and Torell, L. A. (2014). Livestock grazing effects on fuel loads for wildland fire in sagebrush dominated ecosystems. J. Rangeland Appl. 1, 35–57. doi: 10.1007/s004420100737
Sugihara, N. G., van Wagtendonk, J. W., and Fites-Kaufman, J. (2006). “Fire as an ecological process,” in Fire in California's Ecosystems, eds N. G. Sugihara, J. W. van Wagtendonk, K. E. Shaffer (Berkeley, CA: University of California Press), 58–74.
Suring, L. H., Rowland, M. M., and Wisdom, M. J. (2005). “Identifying species of conservation concern,” in, Habitat Threats in the Sagebrush Ecosystem: Methods of Regional Assessment and Applications in the Great Basin, eds M. J. Wisdom, M. M. Rowland, and L. H. Suring (Lawrence, KS: Alliance Communications Group), 150–162.
Syphard, A. D., Keeley, J. E., and Abatzaglou, J. T. (2017). Trends and drivers of fire activity vary across California aridland ecosystems. J. Arid Environ. 144, 110–122. doi: 10.1016/j.jaridenv.2017.03.017
Tagestad, J., Brooks, M., Cullinan, V., Downs, J., and McKinley, R. (2016). Precipitation regime classification for the Mojave Desert: implications for fire occurrence. J. Arid Environ. 124, 388–397. doi: 10.1016/j.jaridenv.2015.09.002
Thompson, M. P., Marcot, B. G., Thompson, F. R., McNulty, S., Fischer, L. A., Runge, M. C., et al. (2013). The Science of Decision Making: Applications for Sustainable Forest and Grassland Management in the National Forest System. General Technical Reports GTR-WO-88. Washington DC: USDA, Forest Service.
U.S. Department of the Interior Geological Survey. (2014). LANDFIRE. 3.0. Existing Vegetation Type Layer. Updated December 17, 2014 (Washington, DC). Available online at: https://www.landfire.gov/evt.php (accessed May 15, 2019).
U.S. Environmental Protection Agency [USEPA]. (2017). Level II and level III Ecoregions of the Continental United States. Corvallis, OR: U.S EPA-National Health and Environmental Research Laboratory, Map scale 1:750,000. Available online at: https://www.epa.gov/eco-research/ecoregions-north-america (accessed May 15, 2019).
Urza, A., Weisberg, P. J., Chambers, J. C., Board, D. I., Dhaemers, J., and Flake, S. (2017). Post-fire vegetation response at the woodland-shrubland interface is mediated by the pre-fire community. Ecosphere 8:e01851. doi: 10.1002/ecs,2.1851
U. S. Department of Agriculture Forest Service [USDA FS]. (2018). Continuous 2018 Wildfire Hazard Potential: GIS Data and Maps. Available online at: https://www.firelab.org/document/continuous-2018-whp-gis-data-and-maps (accessed May 15, 2019).
U. S. Department of the Interior [USDOI] (2016). Safeguarding America's Lands and Waters From Invasive Species: A National Framework for Early Detection and Rapid Response. Washington DC: U.S. Department of the Interior, 55. Available online at: https://www.doi.gov/sites/doi.gov/files/National%20EDRR%20Framework.pdf (accessed May 15, 2019).
U. S. Department of the Interior Fish Wildlife Service [USFWS]. (2015). Endangered and Threatened Wildlife and Plants; 12-Month Finding on a Petition to List the Greater Sage-Grouse (Centrocercus urophasianus) as an Endangered or Threatened Species. Proposed Rule. Fed. Register 80, 59858-59942. Available online at: http://www.gpo.gov/fdsys/pkg/FR-2015-10-02/pdf/2015-24292.pdf (accessed May 15, 2019).
Van Devender, T. R., Felger, R. S., and Burquez, A.M. (1997). “Exotic plants in the sonoran desert region, Arizona and Sonora,” in 1997 Symposium Proceedings (California Exotic Pest Council). Available online at: https://www.cal-ipc.org/resources/symposium/archive/1997-cal-ipc-symposium/ (accessed May 15, 2019).
Vilà, M., and Ibáñez, I. (2011). Plant invasions in the landscape. Landscape Ecol. 26, 461–472. doi: 10.1007/s10980-011-9585-3
Wallace, J. M., Pavek, P. L. S., and Prather, T. S. (2015). Ecological Characteristics of Ventenata dubia in the Intermountain Pacific Northwest. Invasive Plant Sci. Manag. 8, 57–71. doi: 10.1614/IPSM-D-14-00034.1
West, A. M., Evangelista, P. H., Jarnevich, C. S., Kumara, S., Swallow, A., Luizza, M. W., et al. (2016). Using multi-date satellite imagery to monitor invasive grass species distribution in post-wildfire landscapes: An iterative, adaptable approach that employs open-source data and software. Int. J. Appl. Earth Obs. 59, 135–146. doi: 10.1016/j.jag.2017.03.009
West, N. E. (1983a). “Intermountain salt-desert shrubland,” in Temperate Deserts and Semi-Deserts, ed N.E. West (Amsterdam: Elsevier Publishing Company), 375–378.
West, N. E. (1983b). “Great Basin-Colorado Plateau sagebrush semi-desert,” in Temperate Deserts and Semi-Deserts, ed N. E. West (Amsterdam: Elsevier Publishing Company), 331–350.
Wilcox, B. P., Turnbull, L., Young, M. H., Williams, C. J., Ravi, S., Seyfried, M. S., et al. (2012). Invasion of shrublands by exotic grasses: ecohydrological consequences in cold versus warm deserts. Ecohydrology 5, 160–173. doi: 10.1002/eco.247
Wills, R. (2018). “Central Valley's Ecoregion,” in Fire in California's Ecosystems: 2nd Edition, Revised, ed J. W. van Wagtendonk, N. G. Sugihara, S. L. Stephens, A. E. Thode, K. E. Shaffer, and J. Fites-Kaufman (Berkeley, CA: University of California Press), 279–298.
Wisdom, M. J., Meinke, C. W, Knick, S.T., and Schroeder, M. A. (2011). “Factors associated with extirpation of sage-grouse,” in Greater Sage-Grouse—Ecology Conservation of a Landscape Species Its Habitats, Studies in Avian Biology 38, ed S. T. Knick J. W. Connelly. (Berkeley, CA: University of California Press), 451–474.
Keywords: non-native invasive grasses, fire regimes, resilience to fire, resistance to invasive plants, spatial resilience, high value resources, prioritization, management strategies
Citation: Chambers JC, Brooks ML, Germino MJ, Maestas JD, Board DI, Jones MO and Allred BW (2019) Operationalizing Resilience and Resistance Concepts to Address Invasive Grass-Fire Cycles. Front. Ecol. Evol. 7:185. doi: 10.3389/fevo.2019.00185
Received: 15 February 2019; Accepted: 08 May 2019;
Published: 06 June 2019.
Edited by:
Anouschka R. Hof, Wageningen University and Research, NetherlandsReviewed by:
Edith B. Allen, University of California, Riverside, United StatesEddie Van Etten, Edith Cowan University, Australia
Copyright © 2019 Chambers, Brooks, Germino, Maestas, Board, Jones and Allred. This is an open-access article distributed under the terms of the Creative Commons Attribution License (CC BY). The use, distribution or reproduction in other forums is permitted, provided the original author(s) and the copyright owner(s) are credited and that the original publication in this journal is cited, in accordance with accepted academic practice. No use, distribution or reproduction is permitted which does not comply with these terms.
*Correspondence: Jeanne C. Chambers, amVhbm5lLmNoYW1iZXJzQHVzZGEuZ292