- 1Department of Entomology, Rutgers University, New Brunswick, NJ, United States
- 2Department of Environmental Systems Science, ETH Zürich, Zurich, Switzerland
- 3Department of Plant Biology, Rutgers University, New Brunswick, NJ, United States
Despite increasing knowledge about the impacts of pathogens on the interactions between plants and insect vectors, relatively little is known about their effects on other, non-vector, organisms. In cranberries, phytoplasma infection causes false blossom disease, which is transmitted by leafhoppers. We hypothesized that changes in plant chemistry induced by phytoplasma infection might affect the performance and feeding behavior not only of vectors but also of other phytophagous insects. To test this, we measured growth, survival, and the number of leaves damaged by larvae of three common non-vector herbivores: spotted fireworm (Choristoneura parallela Robinson), Sparganothis fruitworm (Sparganothis sulfureana Clemens), and gypsy moth (Lymantria dispar L.) on phytoplasma-infected and uninfected cranberries (Vaccinium macrocarpon Ait.). We also assessed the effects of phytoplasma infection on nutrients and phytochemistry related to defenses. In general, larvae of all three herbivore species grew 2–3 times bigger, and damaged 1.5–3.5 times more leaves, while feeding on infected vs. uninfected plants. Survival of Sparganothis fruitworm larvae was also ~1.5 times higher on infected plants, while spotted fireworm and gypsy moth larval survival was not affected. In a long-term (5-week) assay, gypsy moth larval survival and mass were enhanced when feeding on phytoplasma-infected leaves. Levels of important plant nutrients (e.g., N, P, K, Ca, S, Mn, Fe, B, Al, and Na) were higher in infected plants, while levels of defensive proanthocyanidins were reduced by 20–40% compared to uninfected plants. In contrast, levels of Mg were lower in infected plants, while concentrations of Cu, Zn, and defensive flavonols were not affected. Taken together, these findings suggest that phytoplasma infection enhances plant nutritional quality, while reducing plant defenses in cranberries. These effects, in turn, may explain the observed enhancement of non-vector herbivore performance, as well as the higher number of damaged leaves, on infected plants. Improved understanding of the ecology of pathogen-plant-herbivore interactions could aid efforts to enhance plant resistance and suppress disease transmission in agricultural settings.
Introduction
Insect-borne plant pathogens are common in both natural and agricultural ecosystems (Eigenbrode et al., 2018). These pathogens, which include viruses, bacteria, and fungi, often depend on insect herbivores as vectors for their transfer from infected to healthy, uninfected plants (Eigenbrode et al., 2018). Consequently, the epidemiology of these pathogens can be strongly influenced by the host selection and feeding behaviors of vector insects, which, in turn, are influenced by the levels of primary and secondary metabolites in plants (Gandon, 2018). Over the past two decades, many studies have demonstrated the ability of pathogens to affect vector behavior by altering features of host-plant chemistry (Stafford et al., 2011; Ingwell et al., 2012; Mauck et al., 2012; Eigenbrode and Bosque-Pérez, 2016; Mauck, 2016), including plant defense signals (Zhang et al., 2016; Carr et al., 2018), volatile emissions (Eigenbrode et al., 2002; Jiménez-Martínez et al., 2004; Mauck et al., 2010) and nutrition (e.g., leaf and/or phloem amino acid content) (Blua et al., 1994; McMenemy et al., 2012; Mauck et al., 2014). In addition to influencing host-plant interactions with vectors, such effects are likely to influence interactions with non-vector organisms, including other herbivores (e.g., Kersch-Becker and Thaler, 2014). Such effects on host-plant interactions with non-vectors may likely have broader implications for the ecology of biological communities and ecosystems, given increasing appreciation for the ecological significance of parasite effects on host phenotypes (Lefèvre et al., 2009). Yet, to date, relatively few studies have explored interactions among plant-pathogens, host plants, and non-vector organisms (but see Mauck et al., 2015).
Phytoplasmas are economically important bacterial plant pathogens, transmitted exclusively by insects in the order Hemiptera, that cause severe economic losses to agricultural crops worldwide (Bai et al., 2006; Weintraub and Jones, 2009). Common symptoms caused by phytoplasmas include stem proliferation referred to as witch's broom, changes of the flower structures to leaf-like structures (known as phyllody), yellowing (chlorosis), and stunting (Lee et al., 2000; Christensen et al., 2005). Phytoplasma infection can also alter diverse aspects of host plant chemistry (Oliveira et al., 2005; Musetti, 2009; Sugio et al., 2011a). For example, infection by phytoplasmas can alter carbohydrate and amino acid levels (Lepka et al., 1999; Tan and Whitlow, 2001), induce changes in volatile emissions (Mayer et al., 2008a,b; Orlovskis and Hogenhout, 2016), and affect defense signaling (Sugio et al., 2011b) in plants. Phytoplasma infection has also been shown to increase levels of plant secondary metabolites, including phenolic compounds and hydrogen peroxide (Junqueira et al., 2004; Musetti et al., 2004; Musetti, 2009). Previous work has shown that these changes in the phytochemistry of phytoplasma-infected plants can influence the behavior of insect vectors (e.g., leafhoppers and psyllids) (Weintraub and Beanland, 2006; Mayer et al., 2008a,b, 2011; Kaul et al., 2009; Maixner et al., 2014). For example, Beanland et al. (2000) showed that aster leafhoppers, Macrosteles quadrilineatus Forbes, live longer and have higher fecundity on asters [Callistephus chinensis (L.) Nees] infected by the aster yellows phytoplasma than on uninfected plants. Changes in host plant chemistry induced by phytoplasma infection may also have effects on non-vector herbivores, as host plants are usually shared by a community of insect herbivores that may be differentially influenced by pathogen infection (Barbosa, 1991). However, to our knowledge no previous study has investigated whether changes in plant chemistry due to phytoplasma infection affects the performance of non-vector herbivores.
In cranberries (Vaccinium macrocarpon Ait.), a crop native to North America, a phytoplasma pathogen causes false blossom, an economically-important disease that decreases crop productivity by sterilizing flowers (Chen, 1971; Polashock et al., 2017). This pathogen is transmitted exclusively by the blunt-nosed leafhopper (Limotettix vaccinii Van Duzee; Hemiptera: Cicadellidae) (Beckwith and Hutton, 1929; Dobroscky, 1931; De Lange and Rodriguez-Saona, 2015); however, many other herbivorous insects that do not transmit false blossom also feed on cranberries in the northeastern United States (USA), including many Lepidopteran species such as the spotted fireworm (Choristoneura parallela Robinson; Tortricidae), Sparganothis fruitworm (Sparganothis sulfureana Clemens; Tortricidae), and gypsy moth (Lymantria dispar L.; Erebidae) (Averill and Sylvia, 1998). All of these species feed on cranberry leaves, and spotted fireworm and Sparganothis fruitworm can also damage fruits (Averill and Sylvia, 1998).
The current study tested the hypothesis that changes in plant chemistry due to phytoplasma infection affect the performance of, and the amount of damaged leaves by, non-vector phytophagous insects in cranberries. Specifically, we asked the following questions: First, does performance (i.e., mass and survival) of non-vector insects (spotted fireworm, Sparganothis fruitworm, and gypsy moth), and the number of damaged leaves, differ between phytoplasma-infected and uninfected plants? We tested these three herbivore species because they have different co-evolutionary history with cranberries and could thus be affected differently by phytoplasma infection: spotted fireworm and Sparganothis fruitworm are both native to the USA while gypsy moth is an invasive pest (Averill and Sylvia, 1998). Second, does phytoplasma infection alter features of plant chemistry that affect the plant's suitability for herbivores, including nutrient levels and chemical defenses?
Materials and Methods
Plant Preparation
Phytoplasma-infected and uninfected cranberries (V. macrocarpon c. “Crimson Queen”) were collected in November 2016 (at the dormant stage) and maintained at 10°C for about 3 months. Uninfected plants were taken from stolons provided by Integrity Propagation (http://integritypropagation.com/; Chatsworth, NJ, USA; this nursery regularly tests its plants to ensure they are free of any common cranberry viruses and phytoplasmas), while phytoplasma-infected plants were taken from a commercial cranberry field in Chatsworth, NJ, that was originally planted with material originated from Integrity Propagation. In February 2017, infected and uninfected plants were propagated clonally by stem cuttings (~7 cm each), that were transferred to individual 4 × 4-cm cells and placed in a greenhouse (20 ± 2°C, 70 ± 10% relative humidity [RH], and 15:9 light:dark [L:D]) for rooting. Plants were grown in a 50:50 v/v peat:sand mix, fertilized once a month from March till May with PRO-SOL 20-20-20 of nitrogen (N)-phosphorus (P)-potassium (K) All Purpose Plant Food (Pro Sol, Inc., Ozark, AL, USA) at a rate of 165 ppm N and were watered daily. After the cuttings developed roots, groups of five cuttings were transplanted into single pots (7 × 7 cm2). Plants were allowed to grow in the greenhouse until August 2017 when they were used in experiments. Prior to conducting the experiments, 10 plants (five plants from infected and uninfected plants) were randomly tested by DNA fingerprinting, using sequence characterized amplified region markers (Polashock and Vorsa, 2002), to verify that all plants were genetically the same. Another 10 plants (five plants from infected and uninfected plants) were randomly selected to test for phytoplasma infection by using a nested PCR assay (Lee et al., 2014). These tests showed that all plants belonged to the same genotype (Crimson Queen) and that only infected plants were positive for the presence of phytoplasma (data not shown). Visually, phytoplasma-infected plants did not show symptoms of any other cranberry disease except for those associated with false-blossom disease (e.g., bushy characters, short, and straight uprights; Figure 1). Therefore, our methods ensured that the plants were genetically similar, that uninfected plants were free from phytoplasma, and that growing conditions, propagation methods, and handling were uniform for uninfected and infected plants.
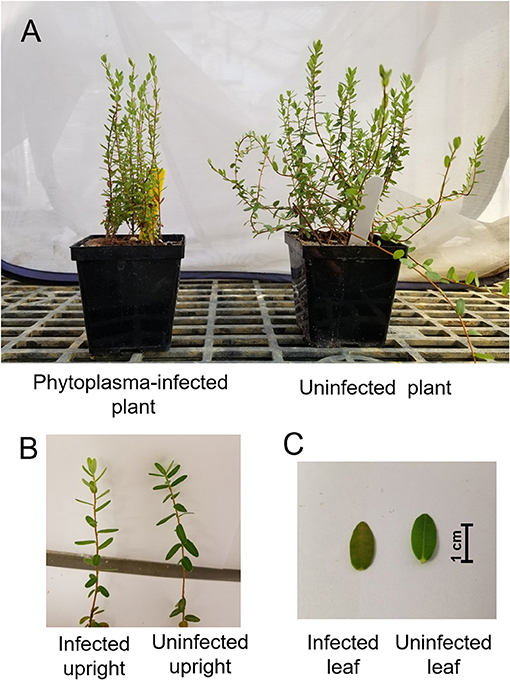
Figure 1. Morphological differences (A) and close-ups of uprights (B) and leaves (C) of phytoplasma-infected and uninfected cranberry (Vaccinium macrocarpon) plants.
For insect assays (see below), all five plants from each pot were used to study the performance of a single herbivore species. A total of 5, 6, and 9 pots of infected plants and the same number of pots of uninfected plants (N = 25, 30, 45 total plants each) were used to study the performance of spotted fireworm, Sparganothis fruitworm, and gypsy moth larvae, respectively. For plant chemistry analyses (see below), one plant was selected from each pot and a total of 10 and 15 uninfected plants and the same number of infected plants were used for nutrient and phenolic analyses, respectively. Different plants were used for the insect performance and chemical assays, and all material for plant chemistry analyses was harvested at the time of the performance assays (August 2017). All plants were at the vegetative stage when used.
Insects
Colonies of spotted fireworm, Sparganothis fruitworm, and gypsy moth were maintained at the Philip E. Marucci Entomology Laboratory (Chatsworth, NJ, USA) (24 ± 1°C, 65% RH, and 14:10 L:D). The spotted fireworm and Sparganothis fruitworm colonies originated from larvae collected from commercial cranberry bogs in Chatsworth, NJ (USA), and larvae were reared on the Stonefly Heliothis Diet (Ward's Scientific, Rochester, NY, USA). Gypsy moth eggs were obtained from the USDA APHIS (Massachusetts, USA), and larvae were reared on a wheat germ diet (Bell et al., 1981). Colonies were supplemented yearly with new individuals to reduce inbreeding depression. First instars were used for all experiments.
Insect Performance and Leaf Damage
To assess larval performance and amount of leaf damage on phytoplasma-infected and uninfected cranberry leaves, feeding experiments were conducted in a greenhouse at 22 ± 2°C, 70 ± 10% RH, and 15:9 L:D. One hundred infected and uninfected plants (total of 20 pots each; N = 200 plants total) were individually covered with 18 × 42 × 48-cm gauze bags (Temkin International; Springville, UT, USA). Each plant then received either three spotted fireworm or Sparganothis fruitworm 1st instars, or one gypsy moth 1st instar [N = 25 (5 pots), 30 (6 pots), and 45 (9 pots), respectively]. Plants were assigned randomly to treatments, and each plant was considered a replicate. Larval mortality and mass were assessed after 7 days (for gypsy moth) or 14 days (for spotted fireworm and Sparganothis fruitworm, whose larvae are smaller than gypsy moth larvae). The number of damaged leaves was estimated by counting the number of leaves with visible signs of larval feeding; note that leaves of phytoplasma-infected and uninfected cranberries have similar surface area (~0.4 cm2; Figure 1C).
An additional laboratory study was conducted to assess the long-term effects of feeding on phytoplasma-infected plants on gypsy moth larval performance (at 24 ± 1°C, 65% RH, and 14:10 L:D). Gypsy moth larvae (1st instars) were placed individually in 30 1-oz (29.57 ml) plastic cups (Maryland Plastic, Inc., Federalsburg, MD, USA) (i.e., one larva per cup). Fifteen larvae were fed uninfected cranberry leaves, while the other 15 larvae were fed phytoplasma-infected cranberry leaves (N = 15 replicates per plant type). Leaves (0.1 g) were replaced with new ones every 3 days; larval mortality and mass were recorded weekly for a total of 5 weeks.
Plant Nutrient Analysis
To explore the effects of phytoplasma infection on plant nutrients, leaves were taken from 10 randomly selected infected and uninfected plants (N = 10 replicates per plant type; 1 plant per pot), kept separately in paper bags, and allowed to dry. For each sample, leaves were randomly collected from different positions within the plant. Dried samples (1.5 g) were sent for nutrient analyses to the Penn State University Agricultural Analytical Service Laboratory (http://agsci.psu.edu/aasl). Total N was analyzed by combustion with an Elementar Vario Max N/C analyzer (Horneck and Miller, 1998), whereas P, K, calcium (Ca), magnesium (Mg), manganese (Mn), iron (Fe), copper (Cu), boron (B), aluminum (Al), zinc (Zn), sodium (Na), and sulfur (S) were analyzed by inductively coupled plasma emission spectroscopy (Huang and Schulte, 1985). For total N concentration, ground dried plant samples (at least 0.1 g) were combust at a high temperature. The gas from combustion was oxidized by copper oxide, then tungsten and Cu turned nitrogen oxide to nitrogen (N2) inside the Elementar Vario Max N/C analyzer. Total N concentrations are estimates from the proportion of electrical signal produced by thermal conductivity detector. For the other chemical elements, ground dried plant samples (0.25 g) were predigested in concentrated HNO3 from room temperature to 60°C for 30 min, followed by digestion with H2O2 at 90°C for 90 min. The sample solution was introduced into the spectrometer that detected the element emission and calculated its concentration.
Phenolic Analysis
To explore the effects of phytoplasma infection on plant defenses, we measured flavonoid levels (i.e., proanthocyanidins and flavonols)—important secondary metabolites involved in plant defense against herbivores (Bernays and Chapman, 1994; Simmonds, 2001)—in phytoplasma-infected and uninfected plants. Leaves were randomly selected from each of 15 infected and 15 uninfected plants (1 plant per pot) and stored at −20°C before extraction (N = 15 replicates per plant type). Frozen samples were ground in liquid nitrogen, the ground material (~30 mg) was then placed in 2 ml Eppendorf tubes, and 0.6 ml of the extraction solution (80% acetone: 19.9% distilled water: 0.1% acetic acid) was added to each tube. Samples were vortexed for 5 min, followed by sonication for 10 min. After sonication, samples were centrifuged at 10,000 rpm for 5 min. The supernatant was transferred to a new Eppendorf tube, and the same procedure was repeated twice with the remaining sample by adding 0.6 ml of the extraction solution each time. The supernatants from these three extractions (~1.8 ml) were transferred to a 2 ml microcentrifuge tube and dried in a centrifugal vacuum for 24 h. The dried extracts were dissolved in 0.5 ml of 100% methanol and analyzed for quantification of flavonols and proanthocyanidins in a Waters Alliance high-pressure liquid chromatography (HPLC) system. HPLC conditions followed those described in Wang et al. (2017).
For flavonol analysis, a Gemini® 150 × 4.6 mm C18 110 Å, 5 μm LC column was used with water + 0.1% formic acid as solvent A and acetonitrile + 0.1% formic acid as solvent B. the elution gradient was 0–15% B from 0 to 1 min; 15–16% B from 1 to 5 min; 16% B from 5 to 10 min; 16–17% B from 10 to 25 min; 17% B from 25 to 28 min; 17–30% B from 28 to 30 min; 30–45% B from 30 to 38 min; 45–80% B from 38 to 40 min; 80–0% B from 40 to 43 min and 0% B from 43 to 50 min. Flow rate was 1 ml/min and injection volume was 10 μl. Compounds were detected in a photodiode array (PDA) detector at 366 nm. For proanthocyanidins, a Develosil® 250 × 4.6 mm 100 Diol-5, 5 μm LC column was used with 98% acetronitrile + 2% acetic acid as solvent A and 95% methanol + 3% water + 2% acetic acid as solvent B. The elution gradient was 0–10% B from 0 to 5 min; 10–12% B from 5 to 7 min; 12% B from 7 to 8 min; 12–13% B from 8 to 10 min; 13–20% B from 10 to 15 min; 20–40% B from 15 to 35 min; 40–0% B from 35 to 45 min and 0% B from 45 to 50 min. Flow rate was 1 ml/min and injection volume was 10 μl. Compounds were detected in fluorescence detector with excitation/emission wavelengths at 280/308 nm.
Statistical Analysis
Prior to analysis, all data were checked for normality and homoscedasticity. If needed, data were square root or log10 transformed to meet the assumptions for analysis of variance (ANOVA); otherwise, non-parametric Mann-Whitney U-tests were used. All parametric and non-parametric tests were performed using IBM® SPSS® version 24. Because each cranberry plant was considered a replicate, the mass and survival of spotted fireworm and Sparganothis fruitworm larvae from the same plants were averaged prior to statistical analysis. Differences in the masses of the spotted fireworm and gypsy moth larvae between uninfected and phytoplasma-infected plants were tested using Mann-Whitney U-tests, whereas differences in Sparganothis fruitworm larval mass were tested using a mixed model that included infection as a fixed factor and pot as a random factor. Larval survival of the spotted fireworm and Sparganothis fruitworm on uninfected and phytoplasma-infected plants was compared by Mann-Whitney U-tests, while gypsy moth larval survival was compared by a chi-square test. Differences in the number of leaves damaged by the spotted fireworm and gypsy moth between uninfected and phytoplasma-infected plants were compared by Mann-Whitney U-tests, whereas we used a mixed model to test for differences in the number of leaves damaged by Sparganothis fruitworm. For the long-term performance assay, we analyzed gypsy moth weekly survival using the Kaplan-Meyer survivorship curve and compared the weekly larval mass gained between infected vs. uninfected plants using one-way ANOVA.
Principal component analysis (PCA) was used to visualize differences in nutrient content and levels of proanthocyanidins and flavonols between uninfected and phytoplasma-infected plants (Minitab® version 18). Differences in the amounts of individual nutrients between uninfected and phytoplasma-infected plants were compared by one-way ANOVA, except for Mg, S, Cu, and Zn, which were analyzed using Mann-Whitney U-tests. Differences in total amounts and amounts of individual proanthocyanidins and flavonols between uninfected and phytoplasma-infected plants were compared by one-way ANOVA.
Results
Phytoplasma Infection Improves Larval Performance and Leaf Damage
Larval mass was consistently enhanced when feeding on phytoplasma-infected plants as compared to uninfected plants (Figure 2). Spotted fireworm (U = 118.5, p = 0.004; Figure 2A), Sparganothis fruitworm [F(1, 5.54) = 81.027, p < 0.001; Figure 2B], and gypsy moth (U = 62, p < 0.001; Figure 2C) larvae feeding on infected plants were 2, 1.9, and 3 times bigger, respectively, than those feeding on uninfected plants. Survival of Sparganothis fruitworm larvae was also ~1.5 times higher when feeding on infected plants (U = 239, p = 0.001; Figure 2A), whereas larval survival of spotted fireworm (U = 300, p = 0.797; Figure 2B) and gypsy moth (χ2 = 1.029, p = 0.31; Figure 2C) showed no significant effects.
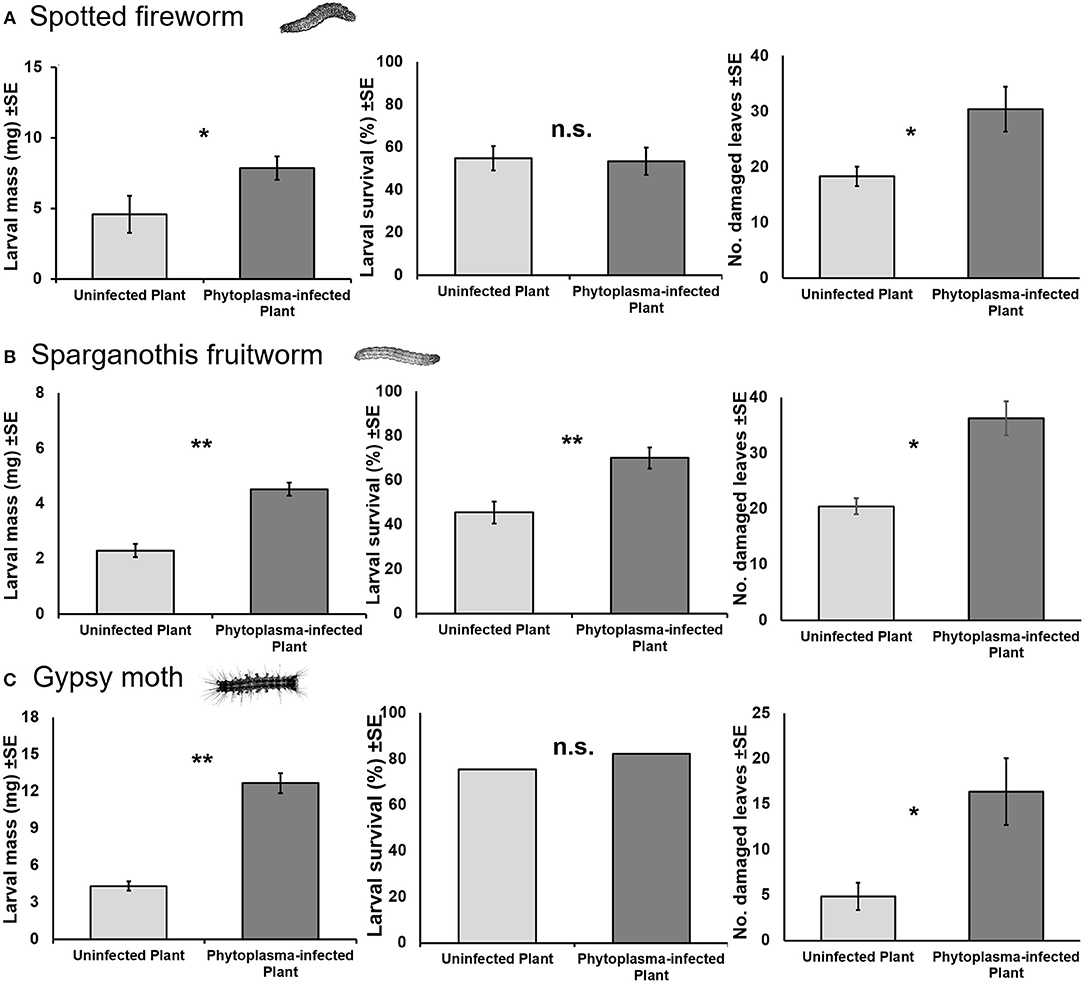
Figure 2. Mean (±SE) larval mass and survival of spotted fireworm (Choristoneura parallela) (A), Sparganothis fruitworm (Sparganothis sulfureana) (B), and gypsy moth (Lymantria dispar) (C), and number of damaged leaves, when fed on uninfected and phytoplasma-infected cranberry plants after 7 or 14 days. Asterisks indicate statistically significant differences (*p ≤ 0.05, **p ≤ 0.001). n.s., not significant (p > 0.05). N = 25, 30, and 35 for spotted fireworm, Sparganothis fruitworm, and gypsy moth, respectively.
The number of damaged leaves was also consistently higher on phytoplasma-infected plants compared to uninfected plants (Figure 2). Spotted fireworm (U = 162, p = 0.039; Figure 2A) and Sparganothis fruitworm [F(1, 5.09) = 18.898, p = 0.007; Figure 2B] larvae damaged ~1.6–1.7 times more infected than uninfected leaves. Similarly, gypsy moth (U = 12.5, p = 0.028; Figure 2C) larvae damaged 3.4 times more infected than uninfected leaves.
In the long-term performance assay, survival of gypsy moth larvae on phytoplasma-infected plants was significantly higher than on uninfected plants (χ2 = 7.995, p = 0.005; Figure 3A). Larvae fed on phytoplasma-infected leaves had higher mass than larvae fed on uninfected leaves [week 1: F(1, 16) = 12.308, p = 0.003; week 2: F(1, 13) = 10.305, p = 0.007; week 3: F(1, 12) = 6.714, p = 0.024; week 4: F(1, 11) = 22.190, p = 0.001] (Figure 3B). After 5 weeks, the mean mass of gypsy moth feeding on phytoplasma-infected leaves was 3.6 times that of larvae feeding on uninfected leaves [mean larval mass ± SE on infected plants = 137.07 ± 21.19 mg and on uninfected plants = 38.03 ± 17.36 mg; F(1, 11) = 10.694, p = 0.007].
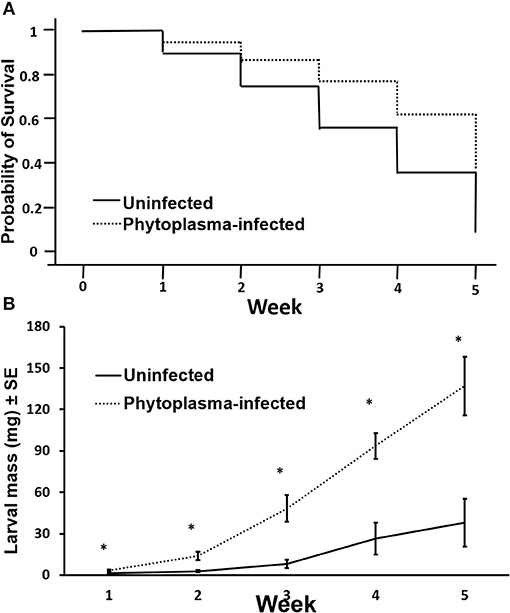
Figure 3. Survival (Kaplan-Meyer curve) (A) and mass (B) of gypsy moth (Lymantria dispar) larvae fed on uninfected and phytoplasma-infected cranberry leaves for 5 weeks. Asterisks indicate statistically significant differences (*p ≤ 0.05). N = 15.
Phytoplasma Infection Increases Plant Nutrients
The PCA revealed clear separation in nutrient composition between phytoplasma-infected and uninfected plants (Figure 4A). The first two components explained 74.7% of the total variation (53.1 and 21.5% for 1st and 2nd components, respectively). Additionally, levels of 10 (out of 13) individual nutrients were significantly higher in phytoplasma-infected than uninfected plants (Table 1). Levels of Mg were lower in infected than uninfected plants, whereas levels of Cu and Zn were not significantly affected by phytoplasma infection (Table 1).
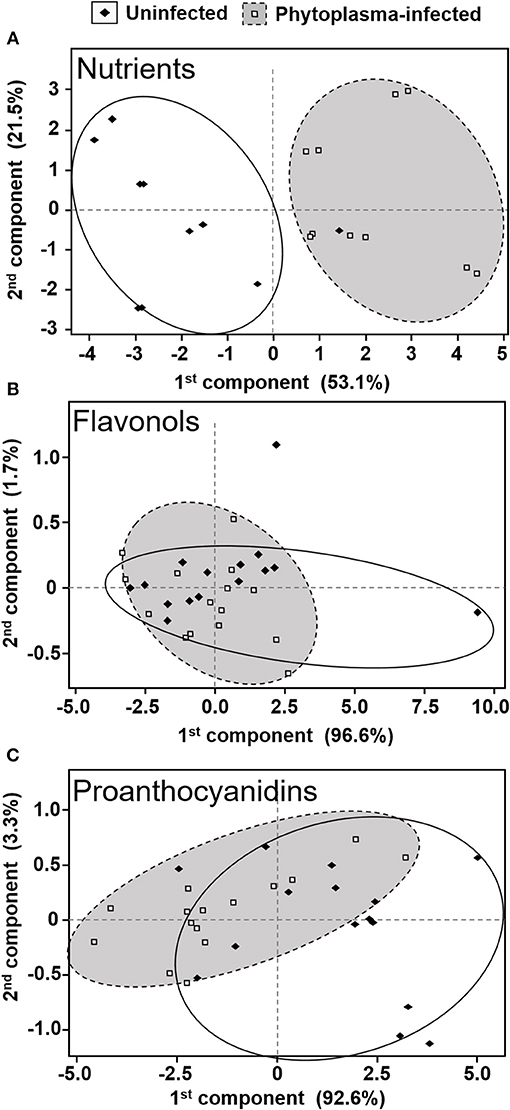
Figure 4. Score plots for principal components 1 and 2 from Principal Component Analysis (PCA) for differences in nutrient (A), flavonol (B), and proanthocyanidin (C) composition between uninfected and phytoplasma-infected cranberry leaves. Data are grouped into clusters, each cluster enclosing 80–100% of the data for a particular group.
Phytoplasma Infection Lowers Proanthocyanidin Content
Six flavonols were identified and quantified from cranberry leaves: quercetin-3-galactoside, quercetin-3-glucoside, quercetin-3-xyloside, quercetin-3-arabinopyranoside, quercetin-3-arabinofuranoside, and quercetin-3-rhamnoside. The PCA revealed a high degree of overlap in flavonol composition between uninfected and phytoplasma-infected cranberry plants, with the first two components explaining 98.3% of total variation (Figure 4B). There were also no significant differences in the levels of total amounts and of all individual flavonols between uninfected and phytoplasma-infected plants (all p > 0.05; Table 2).
Monomeric and oligomeric proanthocyanidins from cranberry leaves were separated by their degree of polymerization (DP) into DP1, DP2, DP3, DP4, DP5, DP6, and DP7+ (polymeric size more than 6). The PCA for the proanthocyanidin polymers shows the first two components explaining 95.9% of the variation, with the first component explaining 92.6% of the variation and separating most of the data into two distinct groups (i.e., phytoplasma-infected and uninfected plants; Figure 4C). Phytoplasma infection reduced the concentrations of all the above-mentioned polymers by 20–40% [DP1: F(1, 28) = 13.523, p = 0.001; DP2: F(1, 28) = 9.404, p = 0.005; DP3: F(1, 28) = 9.463, p = 0.005; DP4: F(1, 28) = 13.596, p = 0.001; DP5: F(1, 28) = 8.345, p = 0.007; DP6: F(1, 28) = 12.266, p = 0.002; DP7+: F(1, 28) = 25.165, p < 0.001; Total: F(1, 28) = 17.627, p < 0.001) compared with uninfected plants (Figure 5).
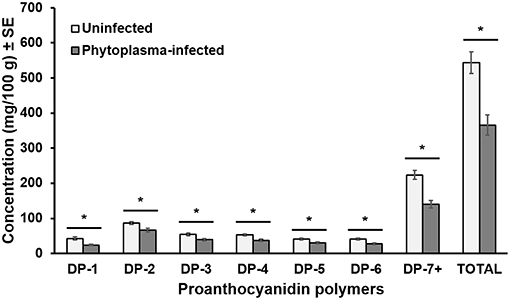
Figure 5. Mean (±SE) amounts of proanthocyanidin polymers in uninfected and phytoplasma-infected cranberry leaves. DP, degree of polymerization. An asterisk indicates statistically significant differences (*p ≤ 0.05). N = 20.
Discussion
The performance of larvae from three lepidopteran species was strongly enhanced by phytoplasma infection relative to uninfected plants. Spotted fireworm, Sparganothis fruitworm, and gypsy moth larvae all grew significantly bigger and also damaged significantly more leaves when fed on infected plants. This increase in the number of leaves damaged may reflect greater larval consumption and/or more frequent larval movement from leaf to leaf due to phytoplasma infection. Though not recorded, a higher biomass in the phytoplasma treatment could also mean that the caterpillars were already in another (later) instar than the ones in the uninfected treatment. Faster growth on phytoplasma-infected plants could provide a fitness advantage as the insects are then not exposed as long to predators and entomopathogens. Previous studies on phytoplasma infection have reported positive (Sugio et al., 2011a; Maixner et al., 2014), negative (Mayer et al., 2011), and neutral effects (Vega et al., 1995) on the performance of insect vectors. There are, however, fewer data on the impact of plant bacterial infections on non-vector species. One study found that the non-vector Peregrinus maidis (Ashmead) (Homoptera: Delphacidae) feeding on corn infected with corn stunt spiroplasma had decreased body mass but increased fecundity (Vega et al., 1995). To our knowledge, this study is the first to document the (positive) effects of phytoplasma infection on non-vector insect herbivores in a cropping system.
The enhancement of non-vector herbivore performance observed in the present study may reflect a pathogen manipulation of the host plant to enhance vector transmission. Because phytoplasmas persist and replicate inside the vector (Hogenhout et al., 2008; Maejima et al., 2014), they may benefit from prolonged vector feeding on infected plants, which in turn may increase the likelihood that vectors will acquire the pathogen. In the current system, we have previously observed that the performance of blunt-nosed leafhoppers, which serve as phytoplasma vectors, was enhanced on infected, relative to uninfected, cranberries (NP, unpublished data). This is consistent with previous findings that persistently transmitted plant viruses tend to enhance host-plant quality for aphid vectors (e.g., Mauck et al., 2012). The current data reveal similar effects on the performance of non-vector herbivores. It remains unknown whether the phytoplasma itself affects, or persists inside, non-vector insects.
To explore the effects of false blossom disease on cranberry chemistry, we examined the effects of infection on constitutive levels of plant nutrients and chemical defenses. With respect to nutrition status, we found that levels of most of the plant mineral concentrations examined were increased in phytoplasma-infected cranberry plants compared to uninfected plants. Previous studies have shown that plant nutrient modulations induced by phytoplasma can vary greatly (e.g., Zhao and Liu, 2009; Al-Ghaithi et al., 2016). In our study, N levels were elevated in phytoplasma-infected cranberry plants. Higher N levels have not only been reported to enhance pathogen infection, growth, and reproduction (Mitchell et al., 2003), but also the growth, development, and fecundity of herbivorous insects (Mattson, 1980; Awmack and Leather, 2002; Chen et al., 2004). In fact, higher plant N concentrations have previously been shown to enhance the growth rates of many Lepidopteran larvae (Chen et al., 2004; Coley et al., 2006). Some of the other nutrients we found to be elevated in phytoplasms-infected plants, including K, are also important for both pathogens and insects (Amtmann et al., 2008). These nutrient changes in cranberries due to phytoplasma infection may thus benefit both pathogens and herbivores.
We also compared levels of several plant defense compounds between phytoplasma-infected and uninfected cranberry plants. Although phytoplasma infection did not affect flavonol levels, it significantly reduced concentrations of proanthocyanidins; these are polyphenolic compounds found in many vascular plants that play an important role in anti-microbial defense but can also act as herbivore deterrents and thus reduce insect feeding (Fisk, 1980; Bernays, 1981; Van Huynh and Bevington, 2014). This result is consistent with previous studies reporting that plant chemical defenses are compromised by pathogen infection (Junqueira et al., 2004; Rusjan et al., 2012). Thus, the increased performance and feeding of non-vector insects on phytoplasma-infected cranberry plants may be due in part to this reduction in plant defenses. In addition to providing plants with protection against pathogens and insect herbivores (Koskimäki et al., 2009), the presence of these and other phenolic compounds have been suggested to have benefits for human health (Côté et al., 2010), making higher quantities of these compounds in cultivated fruits desirable (Gallardo et al., 2018); however, it was not possible to assess effects on fruit chemistry in our study system as false-blossom plants are sterile. Furthermore, since we measured only proanthocyanidin and flavonol levels, further investigation is needed to determine whether other secondary metabolites are differentially affected by phytoplasma infection in cranberry.
There are at least two possible conflicting scenarios via which phenotypic changes in plant chemistry due to phytoplasma infection might arise as the result of a plant-pathogen arms-race. First, phytoplasmas could trigger a defense response (i.e., systemic acquired resistance; Sticher et al., 1997) in plants to suppress the infection: the “induced plant defense” hypothesis. Under this scenario we would expect an increase in levels of secondary metabolites and/or increased resistance against herbivores. However, our data for false blossom disease in cranberries does not support this hypothesis. Alternatively, phytoplasmas could manipulate the plant defense responses for its own benefit and the benefit of the vector: the “vector manipulation” hypothesis (Ingwell et al., 2012). Under this scenario the positive effects on vectors could also conceivably be a side effect of manipulation to enhance pathogen performance. In this case, we would expect reduced secondary metabolites and/or increased nutrient content. These predictions are more consistent with our observations in cranberry. Furthermore, as the current results demonstrate, these effects may enhance host plant quality not only for the leafhopper vector (NP, unpublished data), but also for other herbivores, an observation with potential implications for pest management such as an increase in chemical control measures to manage these pests.
In conclusion, our study demonstrates that phytoplasma-induced changes in cranberry, including increasing mineral nutrient status and lowering defenses, facilitate non-vector herbivore performance and leaf damage. However, many additional questions remain about the relationship between phytoplasma, the host plant, and herbivorous insects. For instance, our study assessed herbivore performance on relatively young (i.e., < 6 months old rooted clones) tissues; additional research is needed to evaluate the effects of phytoplasma infection on herbivore population and community dynamics in cranberry with varying tissue maturities and determine whether phytochemistry levels change with the plant's ontogeny such as at the reproductive (flowering) stage. In our study, changes in phytochemistry due to phytoplasma infection were only investigated before the insects fed on the plants; thus, further investigation is needed to determine whether and how levels of primary and secondary metabolites are subsequently affected by herbivore feeding. However, at least in the short term, we document a positive effect of phytoplasma infection on herbivore performance that was seen through most of the larval development period for gypsy moth. Future transcriptomic and gene expression studies may also provide us with more details on the mechanisms that underlie host plant manipulation by phytoplasmas. Indeed, the mechanisms by which phytoplasmas and other pathogens with small genomes (Marcone et al., 1999) are able to manipulate their hosts to influence interactions with insect vectors is a topic of emerging interest (Musetti, 2009). Addressing these gaps in our existing knowledge will not only provide information to control the spread of important agricultural pathogens (by inducing defenses that could suppress them), but also give us a clearer view of this complex tripartite host plant-herbivore-pathogen relationship in the ecosystem.
Author Contributions
NP, MCM, NV, and CR-S conceived the ideas and designed methodologies. NP and YW collected the data. NP analyzed the data. NP and CR-S led writing of the manuscript. All authors contributed critically to the drafts and gave final approval for publication.
Funding
This work was supported by a Thai Royal scholarship to NP, hatch projects Nos. NJ08140 and NJ08252 to CR-S, and funds from the New Jersey Blueberry and Cranberry Research Council, Inc., Cape Cod Cranberry Growers Association, and Ocean Spray Cranberries, Inc. to CR-S.
Conflict of Interest Statement
The authors declare that the research was conducted in the absence of any commercial or financial relationships that could be construed as a potential conflict of interest.
Acknowledgments
We thank Vera Kyryczenko-Roth for supplying insects for studies and assistance with nutrient analysis, Robert Holdcraft for help with figures, Brittany Dodson for editing services, and two reviewers for helpful comments on an earlier draft.
References
Al-Ghaithi, A. G., Hanif, M. A., Al-Busaidi, W. M., and Al-Sadi, A. M. (2016). Increased sodium and fluctuations in minerals in acid limes expressing witches' broom symptoms. SpringerPlus 5:418. doi: 10.1186/s40064-016-2049-0
Amtmann, A., Troufflard, S., and Armengaud, P. (2008). The effect of potassium nutrition on pest and disease resistance in plants. Physiol. Plant. 133, 682–691. doi: 10.1111/j.1399-3054.2008.01075.x
Averill, A. L., and Sylvia, M. M. (1998). Cranberry Insects of the Northeast: A Guide to Identification, Biology and Management. Amherst, MA: University of Massachusetts Extension.
Awmack, C. S., and Leather, S. R. (2002). Host plant quality and fecundity in herbivorous insects. Annu. Rev. Entomol. 47, 817–844. doi: 10.1146/annurev.ento.47.091201.145300
Bai, X., Zhang, J., Ewing, A., Miller, S. A., Jancso Radek, A., Shevchenko, D. V., et al. (2006). Living with genome instability: the adaptation of phytoplasmas to diverse environments of their insect and plant hosts. J. Bacteriol. 188, 3682–3696. doi: 10.1128/JB.188.10.3682-3696.2006
Barbosa, P. (1991). “Plant pathogens and nonvector herbivores,” in Microbial Mediation of Plant-Herbivore Interactions, eds P. Barbosa, V. A. Krischik, and C. G. Jones (New York, NY: John Wiley and Sons, Inc.), 341–382.
Beanland, L., Hoy, C. W., Miller, S. A., and Nault, L. R. (2000). Influence of aster yellows phytoplasma on the fitness of aster leafhopper (Homoptera: Cicadellidae). Ann. Entomol. Soc. Am. 93, 271–276. doi: 10.1603/0013-8746(2000)093[0271:IOAYPO]2.0.CO;2
Beckwith, C. S., and Hutton, S. B. (1929). Cranberry false blossom and the blunt-nosed leafhopper. N. Jersey Agric. Exp. Station Bull. 491, 1–16.
Bell, R. A., Owens, C. D., Shapiro, M., and Tardif, J. R. (1981). “Development of mass rearing technology,” in The Gypsy Moth: Research Toward Integrated Pest Management, eds C. C. Doane and M. L. McManus. (Washington, DC: U.S. Department of Agriculture, Forest Service), 599–633.
Bernays, E. A. (1981). Plant tannins and insect herbivores: an appraisal. Ecol. Entomol. 6, 353–360. doi: 10.1111/j.1365-2311.1981.tb00625.x
Bernays, E. A., and Chapman, R. E. (1994). Host-Plant Selection by Phytophagous Insects. Boston, MA: Springer US. doi: 10.1007/b102508
Blua, M. J., Perring, T. M., and Madore, M. A. (1994). Plant virus-induced changes in aphid population development and temporal fluctuations in plant nutrients. J. Chem. Ecol. 20, 691–707. doi: 10.1007/BF02059607
Côté, J., Caillet, S., Doyon, G., Sylvain, J. F., and Lacroix, M. (2010). Bioactive compounds in cranberries and their biological properties. Crit. Rev. Food Sci. Nutr. 50, 666–679. doi: 10.1080/10408390903044107
Carr, J. P., Murphy, A. M., Tungadi, T., and Yoon, J.-Y. (2018). Plant defense signals: players and pawns in plant-virus-vector interactions. Plant Sci. 279, 87–95. doi: 10.1016/j.plantsci.2018.04.011
Chen, T. A. (1971). Mycoplasmalike organisms in sieve tube elements of plants infected with blueberry stunt and cranberry false blossom. Phytopathology 61, 233–236. doi: 10.1094/Phyto-61-233
Chen, Y., Lin, L., Wang, C., Yeh, C., and Hwang, S. (2004). Response of two Pieris (Lepidoptera: Pieridae) species to fertilization of a host plant. Zool. Stud. 43, 778–786.
Christensen, N. M., Axelsen, K. B., Nicolaisen, M., and Schulz, A. (2005). Phytoplasmas and their interactions with hosts. Trends Plant Sci. 10, 526–535. doi: 10.1016/j.tplants.2005.09.008
Coley, P. D., Bateman, M. L., and Kursar, T. A. (2006). The effects of plant quality on caterpillar growth and defense against natural enemies. Oikos 115, 219–228. doi: 10.1111/j.2006.0030-1299.14928.x
De Lange, E. S., and Rodriguez-Saona, C. (2015). Blunt-Nosed Leafhopper: A Vector of Cranberry False Blossom Disease. Rutgers Coorperative Extension. Factsheet 1248. Availableonline at: https://njaes.rutgers.edu/fs1248/ (accessed November 11, 2015).
Dobroscky, I. D. (1931). Studies on cranberry false blossom disease and its insect vector. Contrib. Boyce Thompson Inst. 3, 59–83.
Eigenbrode, S. D., and Bosque-Pérez, N. A. (2016). “Chemical Ecology of Aphid-Transmitted Plant Viruses,“ in Vector-Mediated Transmission of Plant Pathogens, ed J. K. Brown (St. Paul, MN: The American Phytopathological Society Press), 3–19. doi: 10.1094/9780890545355.001
Eigenbrode, S. D., Bosque-Pérez, N. A., and Davis, T. S. (2018). Insect-borne plant pathogens and their vectors: ecology, evolution, and complex interactions. Annu. Rev. Entomol. 63, 169–191. doi: 10.1146/annurev-ento-020117-043119
Eigenbrode, S. D., Ding, H., Shiel, P., and Berger, P. H. (2002). Volatiles from potato plants infected with potato leafroll virus attract and arrest the virus vector, Myzus persicae (Homoptera: Aphididae). Proc. Biol. Sci. 269, 455–460. doi: 10.1098/rspb.2001.1909
Fisk, J. (1980). Effects of HCN, phenolic acids and related compounds in Sorghum bicolor on the feeding behaviour of the planthopper Perregrinus maidis. Entomol. Exp. Appl. 27, 211–222. doi: 10.1111/j.1570-7458.1980.tb02968.x
Gallardo, K. R., Klingthong, P., Zhang, Q., Polashock, J., Atucha, A., Zalapa, J., et al. (2018). Breeding trait priorities of the cranberry industry in the United States and Canada. HortScience 53, 1467–1474. doi: 10.21273/HORTSCI13219-18
Gandon, S. (2018). Evolution and manipulation of vector host choice. Am. Nat. 192, 23–34. doi: 10.1086/697575
Hogenhout, S. A., Oshima, K., Ammar, E.-D., Kakizawa, S., Kingdom, H. N., and Namba, S. (2008). Phytoplasmas: bacteria that manipulate plants and insects. Mol. Plant Pathol. 9, 403–423. doi: 10.1111/j.1364-3703.2008.00472.x
Horneck, D. A., and Miller, R. O. (1998). “Determination of total nitrogen in plant tissue,” in Handbook of Reference Methods for Plant Analysis, ed Y. Kalra (New York, NY: CRC Press), 75–83.
Huang, C. Y. L., and Schulte, E. E. (1985). Digestion of plant tissue for analysis by ICP emission spectroscopy. Commun. Soil Sci. Plant Anal. 16, 943–958. doi: 10.1080/00103628509367657
Ingwell, L. L., Eigenbrode, S. D., and Bosque-Pérez, N. A. (2012). Plant viruses alter insect behavior to enhance their spread. Sci. Rep. 2:578. doi: 10.1038/srep00578
Jiménez-Martínez, E. S., Bosque-Pérez, N. A., Berger, P. H., Zemetra, R. S., Ding, H., and Eigenbrode, S. D. (2004). Volatile cues influence the response of Rhopalosiphum padi (Homoptera: Aphididae) to barley yellow dwarf virus–infected transgenic and untransformed wheat. Environ. Entomol. 33, 1207–1216. doi: 10.1603/0046-225X-33.5.1207
Junqueira, A., Bedendo, I., and Pascholati, S. (2004). Biochemical changes in corn plants infected by the maize bushy stunt phytoplasma. Physiol. Mol. Plant Pathol. 65, 181–185. doi: 10.1016/j.pmpp.2005.01.005
Kaul, C., Seitz, A., Maixner, M., and Johannesen, J. (2009). Infection of Bois-Noir tuf-type-I stolbur phytoplasma in Hyalesthes obsoletus (Hemiptera: Cixiidae) larvae and influence on larval size. J. Appl. Entomol. 133, 596–601. doi: 10.1111/j.1439-0418.2009.01406.x
Kersch-Becker, M. F., and Thaler, J. S. (2014). Virus strains differentially induce plant susceptibility to aphid vectors and chewing herbivores. Oecologia 174, 883–892. doi: 10.1007/s00442-013-2812-7
Koskimäki, J. J., Hokkanen, J., Jaakola, L., Suorsa, M., Tolonen, A., Mattila, S., et al. (2009). Flavonoid biosynthesis and degradation play a role in early defence responses of bilberry (Vaccinium myrtillus) against biotic stress. Eur. J. Plant Pathol. 125:629. doi: 10.1007/s10658-009-9511-6
Lee, I.-M., Davis, R. E., and Gundersen-Rindal, D. E. (2000). Phytoplasma: phytopathogenic Mollicutes. Annu. Rev. Microbiol. 54, 221–255. doi: 10.1146/annurev.micro.54.1.221
Lee, I.-M., Polashock, J., Bottner-Parker, K. D., Bagadia, P. G., Rodriguez-Saona, C., Zhao, Y., et al. (2014). New subgroup 16SrIII-Y phytoplasmas associated with false-blossom diseased cranberry (Vaccinium macrocarpon) plants and with known and potential insect vectors in New Jersey. Eur. J. Plant Pathol. 139, 399–406. doi: 10.1007/s10658-014-0396-7
Lefèvre, T., Lebarbenchon, C., Gauthier-Clerc, M., Missé, D., Poulin, R., and Thomas, F. (2009). The ecological significance of manipulative parasites. Trends Ecol. Evol. 24, 41–48. doi: 10.1016/j.tree.2008.08.007
Lepka, P., Stitt, M., Moll, E., and Seemüller, E. (1999). Effect of phytoplasmal infection on concentration and translocation of carbohydrates and amino acids in periwinkle and tobacco. Physiol. Mol. Plant Path. 55, 59–68. doi: 10.1006/pmpp.1999.0202
Maejima, K., Oshima, K., and Namba, S. (2014). Exploring the phytoplasmas, plant pathogenic bacteria. J. Gen. Plant Pathol. 80, 210–221. doi: 10.1007/s10327-014-0512-8
Maixner, M., Albert, A., and Johannesen, J. (2014). Survival relative to new and ancestral host plants, phytoplasma infection, and genetic constitution in host races of a polyphagous insect disease vector. Ecol. Evol. 4, 3082–3092. doi: 10.1002/ece3.1158
Marcone, C., Neimark, H., Ragozzino, A., Lauer, U., and Seemüller, E. (1999). Chromosome sizes of phytoplasmas composing major phylogenetic groups and subgroups. Phytopathology 89, 805–810. doi: 10.1094/PHYTO.1999.89.9.805
Mattson, W. J. (1980). Herbivory in relation to plant nitrogen content. Annu. Rev. Ecol. Syst. 11, 119–161. doi: 10.1146/annurev.es.11.110180.001003
Mauck, K., Bosque-Pérez, N. A., Eigenbrode, S. D., Moraes, C. M., and Mescher, M. C. (2012). Transmission mechanisms shape pathogen effects on host–vector interactions: evidence from plant viruses. Funct. Ecol. 26, 1162–1175. doi: 10.1111/j.1365-2435.2012.02026.x
Mauck, K. E. (2016). Variation in virus effects on host plant phenotypes and insect vector behavior: what can it teach us about virus evolution? Curr. Opin. Virol. 21, 114–123. doi: 10.1016/j.coviro.2016.09.002
Mauck, K. E., De Moraes, C. M., and Mescher, M. C. (2010). Deceptive chemical signals induced by a plant virus attract insect vectors to inferior hosts. Proc. Natl. Acad. Sci. U.S.A. 107, 3600–3605. doi: 10.1073/pnas.0907191107
Mauck, K. E., De Moraes, C. M., and Mescher, M. C. (2014). Biochemical and physiological mechanisms underlying effects of Cucumber mosaic virus on host-plant traits that mediate transmission by aphid vectors. Plant Cell Environ. 37, 1427–1439. doi: 10.1111/pce.12249
Mauck, K. E., Smyers, E., De Moraes, C. M., and Mescher, M. C. (2015). Virus infection influences host plant interactions with non-vector herbivores and predators. Funct. Ecol. 29, 662–673. doi: 10.1111/1365-2435.12371
Mayer, C. J., Vilcinskas, A., and Gross, J. (2008a). Pathogen-induced release of plant allomone manipulates vector insect behavior. J. Chem. Ecol. 34, 1518–1522. doi: 10.1007/s10886-008-9564-6
Mayer, C. J., Vilcinskas, A., and Gross, J. (2008b). Phytopathogen lures its insect vector by altering host plant odor. J. Chem. Ecol. 34, 1045–1049. doi: 10.1007/s10886-008-9516-1
Mayer, C. J., Vilcinskas, A., and Gross, J. (2011). Chemically mediated multitrophic interactions in a plant-insect vector-phytoplasma system compared with a partially nonvector species. Agr. Forest Entomol. 13, 25–35. doi: 10.1111/j.1461-9563.2010.00495.x
McMenemy, L. S., Hartley, S. E., MacFarlane, S. A., Karley, A. J., Shepherd, T., and Johnson, S. N. (2012). Raspberry viruses manipulate the behaviour of their insect vectors. Entomol. Exp. Appl. 144, 56–68. doi: 10.1111/j.1570-7458.2012.01248.x
Mitchell, C. E., Reich, P. B., Tilman, D., and Groth, J. V. (2003). Effects of elevated CO2, nitrogen deposition, and decreased species diversity on foliar fungal plant disease. Global Change Biol. 9, 438–451. doi: 10.1046/j.1365-2486.2003.00602.x
Musetti, R. (2009). “Biochemical changes in plants infected by phytoplasmas,” in Phytoplasmas: Genomes, Plant Hosts and Vectors, eds P. Weintraub, and P. Jones (Wallingford: CABI UK), 132–146. doi: 10.1079/9781845935306.0132
Musetti, R., di Toppi, L. S., Ermacora, P., and Favali, M. A. (2004). Recovery in apple trees infected with the apple proliferation phytoplasma: an ultrastructural and biochemical study. Phytopathology 94, 203–208. doi: 10.1094/PHYTO.2004.94.2.203
Oliveira, E., Oliveira, C. M., Magalhâes, P. C., Andrade, C. L. T., and Hogenhout, S. (2005). Spiroplasma and phytoplasma infection reduce kernel production, and nutrient and water contents of several but not all maize cultivars. Maydica 50, 171–178.
Orlovskis, Z., and Hogenhout, S. A. (2016). A bacterial parasite effector mediates insect vector attraction in host plants independently of developmental changes. Front. Plant Sci. 7:885. doi: 10.3389/fpls.2016.00885
Polashock, J. J., Caruso, F. L., Averill, A. L., and Schilder, A. C. (2017). Compendium of Blueberry, Cranberry, and Lingonberry Diseases and Pests. St. Paul, MN: The American Phytopathological Society Press. doi: 10.1094/9780890545386
Polashock, J. J., and Vorsa, N. (2002). Development of SCAR markers for DNA fingerprinting and germplasm analysis of American cranberry. J. Am. Soc. Horti. Sci. 127, 677–684. doi: 10.21273/JASHS.127.4.677
Rusjan, D., Veberič, R., and Mikulič-Petkovšek, M. (2012). The response of phenolic compounds in grapes of the variety ‘Chardonnay' (Vitis vinifera L.) to the infection by phytoplasma Bois noir. Eur. J. Plant Pathol. 133, 965–974. doi: 10.1007/s10658-012-9967-7
Simmonds, M. S. (2001). Importance of flavonoids in insect–plant interactions: feeding and oviposition. Phytochemistry 56, 245–252. doi: 10.1016/S0031-9422(00)00453-2
Stafford, C. A., Walker, G. P., and Ullman, D. E. (2011). Infection with a plant virus modifies vector feeding behavior. Proc. Natl. Acad. Sci. U.S.A. 108, 9350–9355. doi: 10.1073/pnas.1100773108
Sticher, L., Mauch-Mani, B., and Métraux, J. P. (1997). Systemic acquired resistance. Annu. Rev. Phytopathol. 35, 235–270. doi: 10.1146/annurev.phyto.35.1.235
Sugio, A., Kingdom, H. N., MacLean, A. M., Grieve, V. M., and Hogenhout, S. A. (2011a). Phytoplasma protein effector SAP11 enhances insect vector reproduction by manipulating plant development and defense hormone biosynthesis. Proc. Natl. Acad. Sci. U.S.A. 108, E1254–E1263. doi: 10.1073/pnas.1105664108
Sugio, A., MacLean, A. M., Kingdom, H. N., Grieve, V. M., Manimekalai, R., and Hogenhout, S. A. (2011b). Diverse targets of phytoplasma effectors: from plant development to defense against insects. Annu. Rev. Phytopathol. 49, 175–195. doi: 10.1146/annurev-phyto-072910-095323
Tan, P. Y., and Whitlow, T. (2001). Physiological responses of Catharanthus roseus (periwinkle) to ash yellows phytoplasmal infection. N. Phytol. 150, 757–769. doi: 10.1046/j.1469-8137.2001.00121.x
Van Huynh, A., and Bevington, M. J. (2014). MALDI-TOF MS analysis of proanthocyanidins in two lowland tropical forest species of Cecropia: a first look at their chemical structures. Molecules 19, 14484–14495. doi: 10.3390/molecules190914484
Vega, F. E., Barbosa, P., Kuo-Sell, H. L., Fisher, D. B., and Nelsen, T. C. (1995). Effects of feeding on healthy and diseased corn plants on a vector and on a non-vector insect. Experientia 51, 293–299. doi: 10.1007/BF01931115
Wang, Y., Johnson-Cicalese, J., Singh, A. P., and Vorsa, N. (2017). Characterization and quantification of flavonoids and organic acids over fruit development in American cranberry (Vaccinium macrocarpon) cultivars using HPLC and APCI-MS/MS. Plant Sci. 262, 91–102. doi: 10.1016/j.plantsci.2017.06.004
Weintraub, P. G., and Beanland, L. (2006). Insect vectors of phytoplasmas. Annu. Rev. Entomol. 51, 91–111. doi: 10.1146/annurev.ento.51.110104.151039
Weintraub, P. G., and Jones, P. (2009). Phytoplasmas: Genomes, Plant Hosts and Vectors. Wallingford: CABI. doi: 10.1079/9781845935306.0000
Zhang, C., Ding, Z., Wu, K., Yang, L., Li, Y., Yang, Z., et al. (2016). Suppression of jasmonic acid-mediated defense by viral-inducible microRNA319 facilitates virus infection in rice. Mol. Plant. 9, 1302–1314. doi: 10.1016/j.molp.2016.06.014
Keywords: Vaccinium macrocarpon, spotted fireworm, Sparganothis fruitworm, gypsy moth, host manipulation, nutrients, flavonoids
Citation: Pradit N, Mescher MC, Wang Y, Vorsa N and Rodriguez-Saona C (2019) Phytoplasma Infection of Cranberries Benefits Non-vector Phytophagous Insects. Front. Ecol. Evol. 7:181. doi: 10.3389/fevo.2019.00181
Received: 24 December 2018; Accepted: 06 May 2019;
Published: 22 May 2019.
Edited by:
Claude Becker, Gregor Mendel Institute of Molecular Plant Biology (GMI), AustriaReviewed by:
Sybille B. Unsicker, Max Planck Institute for Chemical Ecology, GermanyYuanyuan Song, Fujian Agriculture and Forestry University, China
Copyright © 2019 Pradit, Mescher, Wang, Vorsa and Rodriguez-Saona. This is an open-access article distributed under the terms of the Creative Commons Attribution License (CC BY). The use, distribution or reproduction in other forums is permitted, provided the original author(s) and the copyright owner(s) are credited and that the original publication in this journal is cited, in accordance with accepted academic practice. No use, distribution or reproduction is permitted which does not comply with these terms.
*Correspondence: Cesar Rodriguez-Saona, Y3JvZHJpZ3VlekBuamFlcy5ydXRnZXJzLmVkdQ==