- 1School of Ocean Sciences, Bangor University, Bangor, United Kingdom
- 2Centre for Ecology and Hydrology, Bangor, United Kingdom
- 3Ocean and Earth Science, National Oceanography Centre Southampton, University of Southampton, Southampton, United Kingdom
- 4Marine Biological Association, Plymouth, United Kingdom
Grassland carbon capturing and storage (CCS) is thought to benefit from regulation of grazing. The impact is likely to depend on livestock density. Yet, few studies have tested this principle or evaluated the consistency of grazer-carbon relationships across multiple sites. We sampled four intertidal zones across 22 salt marshes along a 650 km stretch of coast in the UK to examine the impact of livestock density on globally important saltmarsh “blue carbon” stocks. Although there were marked impacts of grazing pressure on above ground vegetation composition, structure and biomass, there was no detectable relationship between grazing intensity and soil organic carbon, irrespective of tidal zone in the marsh or soil depth-layer analyzed. A substantial spatial variation in soil carbon was instead explained by contextual environmental variables. There was evidence that compensatory responses by vegetation, such as increased root growth, countered carbon loss from grazing impacts. Our work suggests that grazing effects on carbon stocks are minimal on broader scales in comparison with the influence of environmental context. The benefits of grazing management to carbon stores are likely to be highly context dependent.
Introduction
Environmental policies and management seek to optimize natural carbon capture and storage (CCS) across natural landscapes (Richards, 2004; Beaumont et al., 2014). Natural landscapes that are of most interest for carbon storage, such as peat bogs, upland heathland, mangroves and salt marshes, are often subject to livestock grazing that can influence CCS (Reeder and Schuman, 2002; Ostle et al., 2009; Donato et al., 2011; McSherry and Ritchie, 2013). Effective management of CCS relies both on understanding the nature of the relationship between CCS and intensity of land-use, and the consistency of this relationship across environmentally variable landscapes. While there are several ways to enhance the CCS potential of natural landscapes (Wallage et al., 2006; Ostle et al., 2009; Dung et al., 2016), adjustment of livestock grazing pressure is perhaps one of the easiest to manage and implement.
Natural and semi-natural grasslands, such as prairies, steppes and plains provide a valuable insight into the impact of livestock grazing on above- and below-ground processes (Kang et al., 2007). Globally, more than 40% of grasslands are grazed by livestock (Reid et al., 2008); thus, the regulation of stocking density provides an opportunity to positively influence carbon sequestration (Tanentzap and Coomes, 2012). While the sum of direct emissions of carbon dioxide and methane associated with livestock respiration, digestion and fecal decomposition increase with stocking density (Murray et al., 2001; Pinares-Patino et al., 2007), grazers also have strong above ground impacts on vegetation (Figures 1A,B) (McNaughton, 1979; Jensen, 1985; Facelli and Pickett, 1991; Olofsson and Oksanen, 2002; Cao et al., 2004), indirectly linking to below ground processes associated with CCS (De Deyn et al., 2008). The direction and magnitude of the impacts of livestock grazing are dictated by its intensity. Given the relationship between above and below ground processes (Bardgett and Wardle, 2003), it might be expected that an increase in grazing intensity will lead to a decrease in below ground soil carbon stocks. However, plant compensatory responses, such as increased root growth and increased carbon allocation to the roots with elevation of grazing pressure, can sometimes counter the above ground effects of grazers on carbon storage (Sollins et al., 1996; Derner et al., 2006; Tanentzap and Coomes, 2012). For example, light or moderate grazing intensity results in a fast growing, diverse plant community, increased root growth, and increased carbon allocation to the roots. In contrast, heavy grazing intensity results in minimal above ground biomass, low species richness and stunted root growth (Schuster, 1964; McNaughton, 1979; Jensen, 1985; Kiehl et al., 1996).
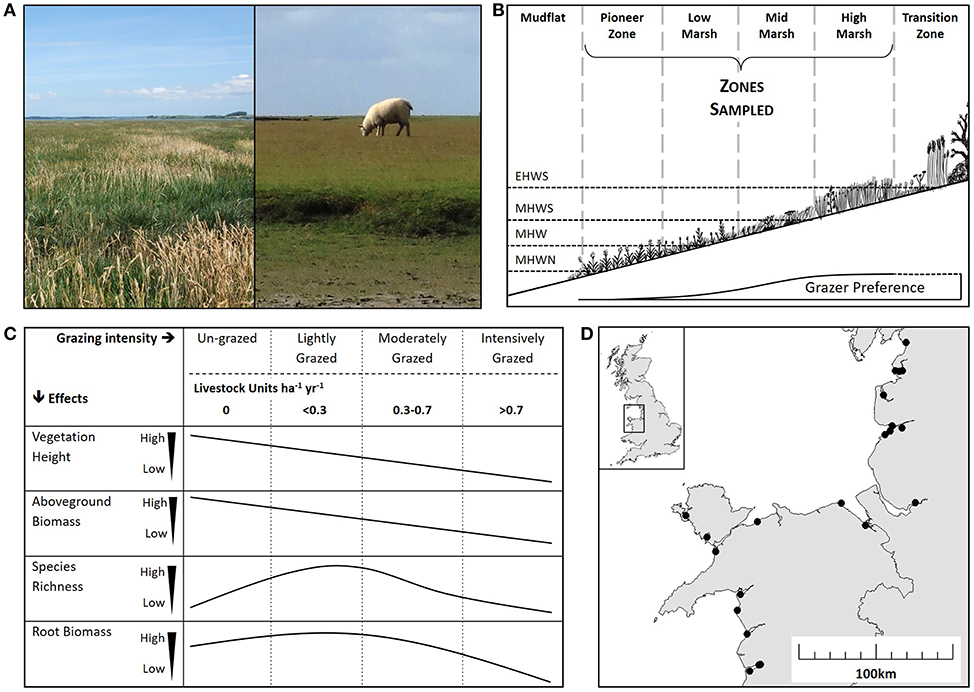
Figure 1. Salt marsh ecology and impacts of grazing. (A) The contrast between an un-grazed marsh (left) and an intensively grazed marsh (right). (B) A diagrammatic representation of a salt marsh showing zonation according to tidal inundation: extreme high-water spring (EHWS), mean high water spring (MHWS), mean high water (MHW) and mean high water neap (MHWN). Only the pioneer, low, mid and high zones were sampled in this study. Grazer preference is indicated showing decreased grazing activity in the lower zones. (C) Predicted effects of a range of grazing intensities on vegetation height, aboveground biomass, species richness and root biomass according to the literature (Stumpf, 1983; Jensen, 1985; Facelli and Pickett, 1991; McNaughton et al., 1998). Grazing is shown as “Grazing Intensity” category classified according to a Welsh Assembly Grazing Management Scheme (un-grazed, lightly grazed, moderately grazed and intensively grazed) and Livestock Units per hectare per year (LSU ha−1 yr−1). (D) Location of the 22 study sites along the coasts of west Wales and northwest England.
There is the expectation that regulation of livestock density will benefit below ground carbon stocks (Frank et al., 1995; Conant et al., 2000; Derner et al., 2006; Yu and Chmura, 2010; Ward et al., 2016). Here we employ a multi-site study to explore whether grazing has detectable broad-scale impacts on above- and below-ground carbon stores in salt marshes, an ecosystem that is reputedly rich in carbon stocks (Duarte et al., 2013). So far, saltmarsh grazer-carbon relationships have only been explored by small scale studies and these have generated conflicting results from increases in soil C (Schuman et al., 1999; Derner et al., 2006; Yu and Chmura, 2010; Elschot et al., 2015a) to decreases in soil C (Morris and Jensen, 1998; Reader and Craft, 1999; Klumpp et al., 2009; Tanentzap and Coomes, 2012; Di Bella et al., 2015) or varied/no impacts (Meyer et al., 1995; Ford et al., 2012; Chen et al., 2015; Schipper et al., 2017) Large-scale studies are needed given that the likely cause for conflicting evidence is that grazing imapct on cabon storage is context dependent as suggested by recent systematic reviews (Conant and Paustian, 2002; McSherry and Ritchie, 2013; Davidson et al., 2017). In this study, we aim to empirically determine whether livestock grazing shows a significant impact on above- and below-ground salt marsh blue-carbon stocks in relation to several environmental parameters across a large biogeographical region.
Salt marshes are characterized by halophytic herbs, grasses and low shrubs that are periodically inundated with saline water, forming distinct plant communities zoned along the vertical stress-gradient of tidal inundation (Adam, 1990a) (Figure 1B). Salt marshes, along with mangroves and seagrass beds, are thought to provide longer-lasting and far denser “blue” carbon stores than most terrestrial systems, although salt marsh carbon stocks do vary considerably with marsh maturity, geomorphology and environmental setting (Sousa et al., 2010; Mcleud et al., 2011; Hayes et al., 2017; Himes-Cornell et al., 2018). Carbon stores are rich and long-lasting in marshes because they have high plant productivity (Middelburg et al., 1997) and sulfate rich, anaerobic sediments with slow organic deposition rates (Howarth, 1984; Valiela et al., 1985; Mueller et al., 2017) The low-energy, depositional characteristics of salt marshes also make them excellent carbon sinks (Chmura et al., 2003; Andersen et al., 2010) as much of the particulate material trapped by marshes is rich in externally produced organic matter (Van de Broek et al., 2018; Mueller et al., 2019).
Globally, many salt marshes are grazed by livestock for meat production and conservation management (Gedan et al., 2009). In un-grazed marshes the main inputs to soil carbon are sedimentation (Stumpf, 1983), degradation of root matter and plant litter deposition (Saunders et al., 2006), while the main outputs are gas emissions of carbon dioxide and methane (Morris and Whiting, 1986; Bartlett et al., 1987; Ford et al., 2012; Kingham, 2013) and sediment loss from wave and tidal erosion (Chalmers et al., 1985; Kingham, 2013). Introducing livestock is likely to have conflicting effects on saltmarsh properties and processes that affect CCS (Figure 1C) (Schuster, 1964; Jensen, 1985; Kiehl et al., 1996; McNaughton et al., 1998; Milotić et al., 2010). For example, above ground biomass and plant litter are likely to decrease under all grazing regimes (Bakker, 1985; Jensen, 1985; Kiehl et al., 1996). Consequently, less sediment, and associated carbon, would be trapped by vegetation during immersion (Neuhaus et al., 1999; Mueller et al., 2017). Conversely, soil compaction by livestock reduces soil pore size and induces anaerobic conditions (Tanner and Mamaril, 1958; Mueller et al., 2017), which in turn diminishes decomposition and carbon dioxide emission rates (Scanlon and Moore, 2000; Hussein and Rabenhorst, 2002; Elschot et al., 2015b). Grazer introduction of terrestrial sources of organic matter in to marshes may alter microbial community composition, which in turn can cause microbially-controlled pathways to allocate more carbon to medium- and long-term storage pools (Olsen et al., 2011; Mueller et al., 2017). In terrestrial wetlands anaerobic conditions result in microbial communities that increase methane emissions (Wang et al., 1996), but in salt marshes methane emissions are limited by abundant sulfate-reducing bacteria (Winfrey and Ward, 1983); therefore soil compaction at high stocking densities would be expected to reduce carbon loss by gas efflux in salt marshes.
We surveyed salt marshes across the west coast of the UK, covering a range of livestock densities, to investigate the broad-scale impacts of grazing intensity on salt marsh below-ground carbon stock. We expected grazing would reduce vegetation height and biomass (Figure 1C) and predicted that below ground carbon stock would significantly decrease at the highest stocking density, due to strong coupling between above and below ground processes. The study explored if grazer-induced loss of above-ground carbon input was counteracted by below-ground increased root growth at lower stocking densities (Figure 1C). Light and intense grazing were expected to increase and reduce plant diversity, respectively (Figure 1C). The study collected information on abiotic environmental variables to explore the influence of environmental context on salt marsh soil carbon, and to contrast the relative importance of context against the effects of grazer density.
Materials and Methods
Study Sites
Soil carbon stock was sampled in 22 salt marshes along a 650 km stretch of the west coast of the United Kingdom (Figure 1D; Table 1). Sites were in a single biogeographical saltmarsh region (Adam, 1990a); they were selected to incorporate a wide range of stocking densities with grazing regimes that were known and had been consistent for the past 30 years or more (Table 1). Although there were around 35–40 salt marshes within this region, marshes that were too small (<2 hectares), had a too much freshwater influence, or those where access was denied by landowners were excluded.
Livestock Units (LSU ha−1 yr−1) were used as a standardized measure of stocking density, where 1 LSU = 1 cow/6.6 sheep/33 geese (Almunia, 2009; Welsh Government, 2017). Management schemes in the UK stipulate grazing pressure by categorizing sites into a range of grazing intensities. Thus, sites were categorized into four grazing intensities based on Tir Gofal, an agri-environmental scheme in Wales (un-grazed; lightly grazed: <0.3 LSU ha−1 yr−1; moderately grazed: 0.3–0.7 LSU ha−1 yr−1; intensively grazed: >0.7 LSU ha−1 yr−1). Stocking densities (Table 1) and the Tir Gofal grazing categories were comparable to those observed in grazing studies from other salt marshes (Andresen et al., 1990; Berg et al., 1997; Neuhaus et al., 1999) and terrestrial grasslands (Rauzi and Hanson, 1966; Haveren, 1983; Graff et al., 2007; Proensky et al., 2016).
Sampling Design
Sampling of each marsh was stratified using the four marsh vegetation zones at different shore elevations classically recognized in the salt marsh literature: pioneer, low, mid, and high marsh (Figure 1B) (Adam, 1990a). Some sites had fewer than four marsh zones due to embankments at the top end and/or erosion at the seaward margin (Table 1). Each zone was sampled in a 100 m wide area, using 10 randomly located 2 × 2 m quadrats. Above-ground plant measurements were sampled in all 10 quadrats and four of the quadrats were additionally sampled below ground. Table 1 shows the total number of quadrats per site.
Above and Below-Ground Sampling
Ten quadrats per zone were sampled above-ground for the following. Percentage cover per vegetation species was estimated by eye (Rodwell et al., 2000). Vegetation mean (n = 5) and maximum heights (n = 1) were observed according to Stewart et al. (2001). Vegetation biomass and litter biomass were sampled in a 25 × 50 cm area at the bottom left corner of each quadrat: live vegetation was cut at the soil level and dead litter was collected from the soil surface. Vegetation samples were then dried (80°C, 3 d). Four quadrats per zone were sampled below-ground for the following. One soil core (46 mm diameter, 46 cm deep) was taken centrally in the quadrat using a split tube corer with removable PVC liner that held the sediment until analysis. Three bulk density soil samples (4.8 cm diameter, 2.5 cm deep) were taken at the soil surface by each soil core and pooled. The mean (n = 5) soil surface compaction and subsurface soil strength were observed using a Geotest pocket penetrometer and a Pilcon shear vane, respectively. In the laboratory, the soil core was split lengthways into two halves: a half-core for root analysis and a half-core for soil organic carbon (SOC) analysis. The root core was divided into 5 cm depth segments; roots per segment were washed free of sediment over a 0.5 mm meshed metal sieve and dried (80°C, for 3 d). Sediment was extracted from the SOC half-core at five depths: 0–2, 5–7, 11–13, 22–24, and 44–46 cm; roots were manually removed and soil organic matter (SOM) was determined by loss on ignition (Ball, 1964; Schumacher, 2002) of 10 g homogenized sub-samples per depth. SOM was converted to SOC (%) by a quadratic formula (Craft et al., 1991). Bulk density samples (BD) had large roots and stones removed before being dried (105°C, 16 h), where-after SOC density (g C cm−3) was calculated (Emmet et al., 2008).
Observations of Environmental Contextual Predictor Variables
Data on abiotic indicators of environmental context were collated to examine the influence of environmental context on patterns of SOC. Sediment grain-size samples were taken alongside SOC samples (core depths: 0–2, 5–7, 11–13, 22–24, and 44–46 cm) and analyzed in a Malvern Mastersizer 2000 laser particle size analyser after H2O2 treatment (Robinson, 1927). Marsh geomorphological type was classified according to Allen (2000). Site wave exposure was calculated according to Burrows et al. (2008). Estuarine water quality variables (dissolved inorganic N, Orthophosphate, Silicates, pH, salinity, and suspended solids) were obtained from the June-2009 to May-2012 observational dataset held by the Environment Agency of Wales and north-west England.
Data Analysis
The study ran separate analyses for each of the four marsh zones (Figure 1B), as well as a single analysis with all zones incorporated. Single-zone analyses were included because substantial between-zone variation in environmental context made it difficult to detect grazing effects in the all-data analysis. Carbon in the top 12 cm of soils was assumed accumulated over the 30-years period for which we knew the grazing history of sites (Table 1), given the region's 3–4 mm y−1 vertical accretion rate (Shi, 1992; French and Spencer, 1993; Cundy and Croudace, 1996; Fox et al., 1999; Van der Wal et al., 2002; Shepherd et al., 2007). The grazing history of soils deeper than 12 cm was uncertain. The analysis took into consideration this depth-dependent variation in the temporal coupling of soil SOC with known grazing regimes: separate analyses were done for soil layers from 0 to 2, 0 to 10, and 0 to 50 cm. The top soil profile (0–2 cm) was regarded to be indicative of the present flux of material from above ground biomass (e.g., litter) to the below ground carbon pool. This layer was expected to show a tight coupling between above and below ground processes, and to be representative of the current grazing regime. The middle profile (0–10 cm) encompassed most of the root biomass and reflected the 30-years time scale for which the grazing regimes of marshes were known. This layer was expected to be less representative of the current above ground processes than the 0–2 cm depth profile, but to still show a weak coupling between above and below ground processes. The deepest profile (0–50 cm) was considered a metric of grazing in relation to the broader contextual influences. Some cores lacked the deepest soil layer (44–46 cm); missing points were estimated using regression techniques based on the remaining real-data points from the core.
Analyses explored the effects of grazing intensity on a categorical scale (un-grazed, light, medium and intense grazing), which are used by grazing management schemes, and on a continuous scale (LSU ha−1 yr−1) to look for threshold levels in carbon-grazing relationships. Analyses of Variance (ANOVA) or the non-parametric equivalent (Kruskal-Wallis multiple comparisons) were used to assess the impact of grazing as a categorical factor on plant height and biomass and on the individual depth profiles for root biomass and SOC. A p-value threshold of 0.05 was used throughout the analysis. A multivariate Permutational Analysis of Variance (PERMANOVA) (Anderson, 2005) was used to analyse the impact of grazing as a categorical factor on root biomass and SOC across all soil depth profiles, and an Analysis of Covariance (ANCOVA) was run on SOC with depth as a covariate to analyse the impact of grazing on the rate of SOC decrease with depth. A series of regression analyses were used to determine the impact of grazing as a continuous variable on both the aboveground plant characteristics and the individual depth profiles for both root biomass and SOC. The effects of factors grazing intensity (four categorical levels) and zone (four levels) on plant community composition was analyzed using 2-way PERMANOVA, followed by a similarity percentage test (SIMPER) to identify species that most strongly characterized compositional differences between grazing intensities (Primer statistical package: Clarke, 1993; Clarke and Warwick, 2001). The PERMANOVA was run with 9,999 permutations on a log transformed Bray-Curtis similarity matrix. False Discovery Rate (FDR) control p-values were calculated in Microsot Excel for all analyses to compensate for the large number of tests (Verhoeven et al., 2004) and partial eta squared effect sizes () were calculated in Microsoft Excel for all results where ≥0.0099 was a small effect, ≥0.0588 was a medium effect, and ≥0.1379 was a large effect (Cohen, 1988; Richardson, 2011). Finally, a mixed effects model was used to analyse the impact of multiple environmental and contextual factors (including grazing measured as LSU) on soil organic carbon (R Core Team, 2012). The model was run on the overall data set, the combined high marsh zone and mid marsh zone data (zones most used by grazers: Sharps et al., 2017), and the combined low zone and pioneer zone data (zones least likely to be influenced by grazers: Sharps et al., 2017).
Results
Grazing had significant impacts on above ground vegetation, as expected. Plant height (ANOVA, factor Grazing Intensity: F3, 9 = 17.67, p < 0001, = 0.494), above ground biomass (ANOVA, factor Grazing Intensity: F3, 9 = 6.96, p = 0.040, = 0.181) and plant litter biomass (K-WMC: H3 = 135.12, p < 0001) all significantly decreased with increasing grazing intensity regardless of whether grazing was treated as a categorical (grazing intensity category) or continuous (LSU) variable (Figure 2). There was no significant effect of grazing on plant species richness (ANOVA, factor Grazing Intensity: F3,9 = 0.05, p = 0.387, = 0.072), although community composition did change with grazing intensity in the mid marsh [PERMANOVA, factor Grazing Intensity: Pseudo F(3) = 2.071, P(perm) = 0.029]. A SIMPER analysis found that communities of un-grazed marshes were generally highly variable and dominated by one of several community types (Festuca rubra, Atriplex portulacoides or a diverse Plantago maritima/Puccinellia maritima community). Light and moderately grazed marshes were less variable and were consistently dominated by Puccinellia maritima, a stress-tolerant, low marsh species. Intensively grazed marshes were characterized by a low-diversity Festuca rubra sward, which changed little between each marsh. Grazing also impacted surface soil compaction (ANOVA, factor: Grazing—F3, 18 = 6.39, p = 0.003, ηp2 = 0.355), with soil compaction increasing with increasing grazing intensity.
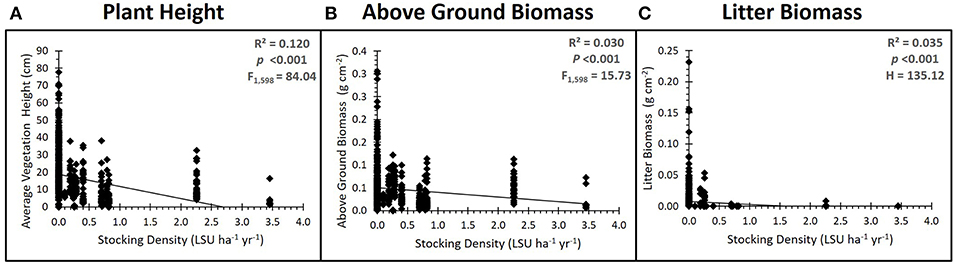
Figure 2. Grazing impacts on above ground vegetation. Results of the regression analysis showing the relationship between grazing intensity (stocking density, LSU ha−1) and (A) average vegetation height, (B) above ground biomass and (C) litter biomass. All significantly decreased with an increase in stocking density, with litter biomass reducing to almost zero as soon as grazers are introduced.
Grazing had no single or interactive effects on below ground soil organic carbon (SOC) [PERMANOVA, factor Grazing Intensity: Pseudo F = 1.18, P(perm) = 0.327] or root biomass [PERMANOVA, factor Grazing Intensity: Pseudo F = 1.35, P(perm) = 0.235] for any of the sediment depths analyzed, regardless of whether grazing was treated as a categorical or continuous variable (Figure 3) (Supplementary Information S1: Regression Table). The rate of decline in SOC with soil depth did not vary significantly between grazing intensities (ANCOVA, factor Grazing Intensity: F3, 1093 = 0.64, p = 0.597, ηp2 = 0.009), although SOC did significantly decrease with depth (Figure 4) (ANCOVA, factor Depth: F1, 1093 = 219.96, p < 0.001, = 0.166). There was no overall (all zones) relationship between root biomass and stocking density (LSU), except for a significant positive relation of root biomass with LSU in the surface soils of the high marsh, suggesting a possible plant compensatory response in this zone (Supplementary Information S1: regression table).
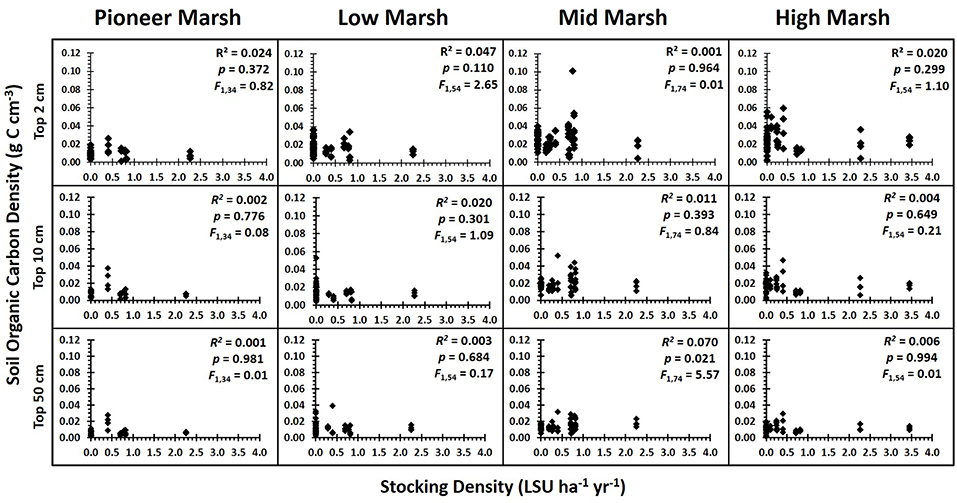
Figure 3. Soil organic carbon density vs. grazing intensity by zone for each of the depths analyzed. Results of the regression analysis showing the relationship between soil organic carbon density and grazing intensity (stocking density, LSU ha−1) for each zone and for each of the depth profiles analyzed: top 2 cm, top 10 cm, and top 50 cm. With the false discovery rate corrections (Verhoeven et al., 2004), there were no significant impacts of grazing on soil carbon (Supplementary Information S1: regression table).
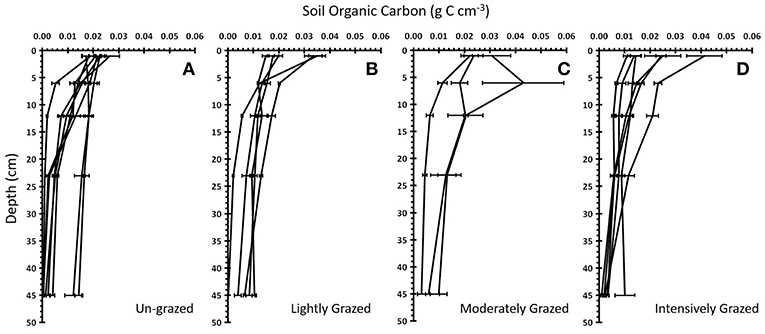
Figure 4. Soil organic carbon with depth for four different grazing intensities. The mean ± standard error soil organic carbon vs. soil depth for each marsh within each “Grazing Intensity” category: (A) un-grazed (n = 8 marshes), (B) lightly grazed (n = 5), (C) moderately grazed (n = 3), and (D) intensively grazed (n = 6). Each line represents a single marsh.
SOC (ANOVA, factor: Zone—F3, 18 = 5.09, p < 0.001, ηp2 = 0.193) and root biomass (ANOVA factor: Zone—F3, 18 = 41.47, p < 0.001, ηp2 = 0.365) did differ between marsh zones: both were higher in the mid and high zones than in the low marsh and pioneer zone. A Tukey HSD test showed that there was significantly less SOC in the top 10 cm of soil of the pioneer (x ± SD = 0.009 ± 0.007) and low marsh (0.014 ± 0.008) zones than in the mid (0.019 ± 0.008) and high (0.017 ± 0.008) marsh zones. SOC in the top 50 cm of soils was lower in the pioneer zone (0.007 ± 0.005) than in the other three zones (low: 0.012 ± 0.007; mid: 0.014 ± 0.005; high: 0.012 ± 0.005). A similar pattern was found for root biomass where root biomass in the top 10 cm was significantly lower in the pioneer zone (0.003 ± 0.005) than in the other three zones (low: 0.011 ± 0.007; mid: 0.015 ± 0.011; high: 0.021 ± 0.015) and was significantly higher in the high marsh than in the other three zones. This pattern was expected as older, higher marsh zones have had longer to accumulate organic matter.
The substantial spatial variation in SOC (Figure 3) was due to consistent among-marsh differences in SOC (ANOVA, factor Marsh: F18, 18 = 7.95, p < 0.001, ηp2 = 0.315) and root biomass (ANOVA, factor Marsh: F3, 18 = 7.14, p < 0.001, ηp2 = 0.392), suggesting that contextual differences among marshes had a greater influence on carbon stocks than grazing. A linear mixed effects model found that SOC in the top 10 cm of soil had a significant association with plant community composition (F16, 181 = 3.10, p < 0.001), but this relationship generally did not translate down to the deeper soil layers (F16, 181 = 1.17, p = 0.292). SOC in the deeper soil profiles of the higher marsh zones showed a significant association with marsh morphology (F5, 12 = 3.92, p = 0.024), tidal range (F1, 12 = 11.86, p = 0.005) with more SOC in marshes with higher tidal ranges, and wave fetch (F1, 12 = 21.69, p = 0.001) with higher SOC in more sheltered marshes. SOC in the deeper profiles in the lower marsh zones was significantly associated with soil grain size, with less SOC in coarse sediments (F1, 69 = 29.38, p < 0.001) (Table 2).
Discussion
Our study shows that clear above ground effects of grazing do not necessarily translate to below ground effects on soil carbon stocks on the broad-scale. We found no detectable effect of grazing on carbon density (g C cm−3), irrespective of which soil depth or marsh zones were considered, or whether or not grazing was considered a categorical or continuous predictor of carbon. The expectation of coupled above to below-ground effect of grazing negates the many complex interactions that vegetation and carbon may have with livestock. Grazing has complex influences on grassland abiotic (e.g., compaction) and biotic (e.g., vegetation biomass and structure) conditions that influence carbon capture and storage (Andresen et al., 1990; Bardgett and Wardle, 2003; Bhogal et al., 2010). Increased stocking density can also initiate compensatory responses by the vegetation community (Holland et al., 1996; Tanentzap and Coomes, 2012) and Tanentzap and Coomes (2012) showed that a small reduction of soil carbon storing in the early years after grazer addition tailed off to no effect of grazing when study systems had sufficient time to develop compensatory responses to herbivory. We found that livestock grazing weakly stimulated the root content in high marsh soils, suggesting that there is some compensation by the species in the vegetation community, thereby mitigating any negative impact of grazing on soil carbon stocks (Tanentzap and Coomes, 2012). Similarly, changes in the microbial communities on grazed marshes may also have increased SOC due to the introduction of terrestrial organic matter from the livestock (Mueller et al., 2017). Alternatively, carbon turnover in microbial communities may have been slower, due to lower redox levels under grazed regimes, resulting in reduced microbial exo-enzymes and therefore slower decomposition rates and greater carbon accumulation in medium- and long-term storage pools (Olsen et al., 2011; Mueller et al., 2017).
In salt marshes, above ground plant adaptation to grazing occurs within a few years to a decade of introduction of grazers (Kuijper and Bakker, 2004). Our sites had been exposed to the same grazing regimes for a minimum of 30 years prior to the study—long enough for plant compensation to take place. We do not dismiss that grazers might have diminished the annual carbon storage at some point; for instance, in the early years following grazer introduction, when some studies have found that grazing can impact on carbon storing (Yu and Chmura, 2010; Sammul et al., 2012). However, over time, this early reduction of soil carbon stocks is likely to have been diluted into insignificance by decades of compensation by the plant community (Tanentzap and Coomes, 2012).
Across a large biogeographical region, variation in environmental drivers had a stronger influence on carbon stocks than livestock density. Salt marshes are at the interface between terrestrial and marine environments (Adam, 1990b) and are subjected to greater spatial and temporal variation in environmental conditions, and hence more disturbance, than many grazed terrestrial grasslands (Adam, 1990c). Environmental context determines much of the external carbon inputs to salt marshes. For instance, sedimentation rates are linked to the local tidal regime (Stumpf, 1983) and allochthonous organic matter, that imported with marine sediment rather than created on the salt marsh, can contribute considerably to SOC stocks where tall vegetation traps sediment (Van de Broek et al., 2018; Mueller et al., 2019). Arguably, the impact of grazing on saltmarsh carbon is comparatively weak relative to the influence of sharp background environmental gradients (Grime, 1974; Nolte et al., 2013). The assumption that carbon capture and storage benefits from management of grazing might well be tenuous in naturally variable and disturbed systems, such as salt marshes.
The observed lack of grazer effects on carbon stock is consistent with the findings of a few smaller-scale grazer presence/absence studies (Meyer et al., 1995; Ford et al., 2012), but it disagrees with studies made in North America (Reader and Craft, 1999; Yu and Chmura, 2010). A recent systematic review showed grazer effects on carbon have only been demonstrated in the USA (Davidson et al., 2017), where marshes are structurally and functionally different too much of the rest of the world (Catterjsse and Hampel, 2006; Bakker et al., 2015). In the USA much of SOC is derived from prolific within-marsh plant production, whilst in Europe the majority of carbon is externally derived through sedimentation (Catterjsse and Hampel, 2006; Bakker et al., 2015). Thus, carbon storing in European type marshes may be more strongly influenced by extrinsic processes that regulate sedimentation than intrinsic processes that influence the vegetation, such as grazing pressure. This deduction is corroborated by the observation made here that environmental context explained the majority of spatial variation in carbon stock, whereas the effects of grazing could not be detected.
Our expectations of negative impacts of grazing on soil carbon stocks were based on evidence from a combination of single-site, experimental studies (Schuster, 1964; Klumpp et al., 2009; Yu and Chmura, 2010; Ford et al., 2012) and meta-data analyses from both terrestrial grasslands and coastal wetlands (Chmura et al., 2003; Tanentzap and Coomes, 2012). None of these studies incorporated a full range of grazing intensities, as we did here; nor did they view the influence of grazing in the context of natural variation. Our study does not imply that grazing has no effect on carbon stocks; rather it shows that the effect of grazing was insufficiently strong or consistent to be detectable above the broad-scale influences of environmental variation and compensation by vegetation. This finding implies that management schemes that stipulate grazing regimes across multiple sites are unlikely to generate spatially consistent effects on carbon stocks. Furthermore, our study only looked at carbon stocks and not carbon sequestration rates. The carbon stocks in this study are a product of accumulated inputs and outputs of organic and inorganic carbon from autochthonous and allochthonous sources, and while there was no impact of grazing on the overall carbon stocks, grazing may affect carbon sequestration rates and mechanisms. There are still many good reasons to manage grazing, such as safeguarding the provision of other ecosystem services, including wave attenuation (Möller et al., 1999), sea defense (King and Lester, 1995; Möller et al., 2007); habitat management (Norris et al., 1997) and biodiversity conservation (Adler et al., 2001). It may be that the regulation of grazing intensity is of particular benefit to above-ground functioning of saltmarshes, such as the provisioning of wildlife habitat and the regulation of gaseous fluxes, and less beneficial to below-ground processes, such as carbon storing.
Author Contributions
MS, AG, RH, and SH contributed toward experimental conception design. RH and MS selected the study sites and collected the field data. RH analyzed all laboratory samples and MS and RH conducted the data analysis with inputs from SH. RH wrote the first draft of the manuscript and all authors contributed to revisions.
Funding
Funding was provided from Knowledge Economy Skills Scholarships (KESS) as a European Social Fund, and from the Natural Environmental Research Council C-SIDE grant (NERC: NE/RO10846/1 R28R32).
Conflict of Interest Statement
The authors declare that the research was conducted in the absence of any commercial or financial relationships that could be construed as a potential conflict of interest.
Acknowledgments
We thank the Centre for Ecology and Hydrology (CEH), Bangor for providing project support and field and laboratory facilities throughout the project. We would like to thank Inma Roberts, Steve Hughes, Hilary Ford, and Jan Hiddink for help and advice on practical and analytical techniques throughout the project. Thanks for fieldwork assistance: Marc Brouard, Gareth Harvey, Jennifer Harvey, Fred Maier, Damian Smith, Caroline Lucien, Kate Batchelor, Cat Spinney, Dan Hewitt, Vicki Foster, and in particular Matt Lundquist, Aoife Ni Neachtain, and Cai Ladd.
Supplementary Material
The Supplementary Material for this article can be found online at: https://www.frontiersin.org/articles/10.3389/fevo.2019.00151/full#supplementary-material
References
Adam, P. (1990b). “General features of saltmarshes and their environment,” in Saltmarsh Ecology (Cambridge: Cambridge University Press), 1–71.
Adam, P. (1990c). “Coping with the environment,” in Saltmarsh Ecology (Cambridge: Cambridge University Press), 207–308.
Adler, P. B., Raff, D. A., and Lauenroth, W.K. (2001). The effect of grazing on spatial heterogeneity of vegetation. Oecologia. 128:4. doi: 10.1007/s004420100737
Allen, J. R. L. (2000). Morphodynamics of Holocene salt marshes: a review sketch from the Atlantic and Southern North Sea coasts of Europe. Quaternary Sci. Rev. 19:12. doi: 10.1016/S0277-3791(99)00034-7
Almunia, J. (2009). Commission of the European Communities. Commission Regulation (EC): Implementing Regulation (EC) No 1166/2008 of the European Parliament and of the Council on Farm Structure Surveys and the Survey on Agricultural Production Methods, as Regards Livestock Unit Coefficients and Definitions of the Characteristics. Report number: 1200/2009. Brussels: European Union.
Andersen, T. J., Svinth, S., and Prejrup, M. (2010). Temporal variation of accumulation rates on a natural salt marsh in the 20th century — the impact of sea level rise and increased inundation frequency. Mar. Geol. 279:1–4. doi: 10.1016/j.margeo.2010.10.025
Anderson, M. J. (2005). PERMANOVA–Permutational Multivariate Analysis of Variance. Aukland: Department of Statistics, University of Aukland.
Andresen, H., Bakker, J. P., Brongers, M., Heydenmann, B., and Irmler, U. (1990). Long-term changes of salt marsh communities by cattle grazing. Vegetatio. 898:2. doi: 10.1007/BF00032166
Bakker, J. P. (1985). The impact of grazing on plant communities, plant populations and soil conditions on salt marshes. Vegetatio. 62:1–3. doi: 10.1007/BF00044766
Bakker, J. P., Nielsen, K. J., Alberti, J., Chan, F., Hacker, S. D., Iribarne, O. O., et al. (2015). “Bottom-up and top-down interactions in coastal interface systems,” in Trophic Ecology: Bottom-up and Top-Down Interactions Across Aquatic and Terrestrial Systems, eds T. C. Hanley, and K. J. La Pierre (Cambridge, UK: Cambridge University Press), 157–200.
Ball, D. F. (1964). Loss-on-ignition as an estimate of organic matter and organic carbon in non-calcareous soils. J. Soil Sci. 15:1. doi: 10.1111/j.1365-2389.1964.tb00247.x
Bardgett, R. D., and Wardle, D. A. (2003). Herbivore-mediated linkages between aboveground and belowground communities. Ecology. 84:9. doi: 10.1890/02-0274
Bartlett, K. B., Bartlett, D. S., Harriss, R. C., and Sebacher, D. I. (1987). Methane emissions along a salt marsh salinity gradient. Biogeochemistry. 4:3. doi: 10.1007/BF02187365
Beaumont, N. J., Jones, L., Garbutt, A., Hansom, J. D., and Toberman, M. (2014). The value of carbon sequestration and storage in coastal habitats. Estuar. Coast. Shelf Sci. 137, 32–40. doi: 10.1016/j.ecss.2013.11.022
Berg, G., Esselink, P., Groeneweg, M., and Kiehl, K. (1997). Micropatterns in Festuca rubra dominated salt-marsh vegetation induced by sheep grazing. Plant Ecol. 132:1. doi: 10.1023/A:1009727804007
Bhogal, A., Nicholson, F. A., Young, I., Sturrock, C., Whitmore, A. P., and Chambers, B. J. (2010). Effects of recent and accumulated livestock manure carbon additions on soil fertility and quality. Eur. J. Soil Sci. 62:1. doi: 10.1111/j.1365-2389.2010.01319.x
Burrows, M. T., Harvey, R., and Robb, L. (2008). Wave exposure indices from digital coastlines and the prediction of rocky shore community structure. Mar. Ecol. Prog. Ser. 353, 1–12. doi: 10.3354/meps07284
Cao, G., Tang, Y., Mo, W., Wang, Y., Li, Y., and Zhao, X. (2004). Grazing intensity alters soil respiration in an alpine meadow on the Tibetan plateau. Soil Biol. Biochem. 36:2. doi: 10.1016/j.soilbio.2003.09.010
Catterjsse, A., and Hampel, H. (2006). European intertidal marshes: a review of their habitat functioning and value for aquatic organisms. Mar. Ecol. Prog. Ser. 324, 293–307. doi: 10.3354/meps324293
Chalmers, A. G., Wiegert, R. G., and Wolf, P. L. (1985). Carbon balance in a salt marsh: interactions of diffusive export, tidal deposition and rainfall-caused erosion. Estuar. Coast. Shelf Sci. 21:6. doi: 10.1016/0272-7714(85)90071-X
Chen, W., Huang, D., Liu, N., Zhang, Y., Badgery, W. B., Wang, X., et al. (2015). Improved grazing management may increase soil carbon sequestration in temperate steppe. Sci. Rep. 5:10892. doi: 10.1038/srep10892
Chmura, G. L., Anisfeld, S. C., Cahoon, D. R., and Lynch, J. C. (2003). Global carbon sequestration in tidal, saline wetland soils. Global Biogeochem. Cy. 17:4. doi: 10.1029/2002GB001917
Clarke, K. R. (1993). Non-parametric multivariate analyses of changes in community structure. Aust. J. Ecol. 18:1. doi: 10.1111/j.1442-9993.1993.tb00438.x
Clarke, K. R., and Warwick, R. M. (2001). Change in Marine Communities: An Approach to Statistical Analysis and Interpratation. 2nd Edn. Plymouth: PRIMER-E.
Cohen, J. (1988). Statistical Power Analysis for the Behavioural Sciences. Hillside, NJ: Lawrence Erlbaum.
Conant, R., Paustian, K., and Elliott, E. T. (2000). Grassland management and conversion into grassland: effects on soil carbon. Ecol. Appl. 11:2. doi: 10.1890/1051-0761(2001)011[0343:GMACIG]2.0.CO;2
Conant, R. T., and Paustian, K. (2002). Potential soil carbon sequestration in overgrazed grassland ecosystems. Global Biogeochem. Cy. 16:4. doi: 10.1029/2001GB001661
Craft, C. B., Seneca, E. D., and Broome, S. W. (1991). Loss on ignition and kjeldahl digestion for estimating organic carbon and total nitrogen in estuarine marsh soils: calibration with dry combustion. Estuar. Coast. 14:2. doi: 10.2307/1351691
Cundy, A. B., and Croudace, I. W. (1996). Sediment accretion and recent sea-level rise in the Solent, Southern England: inferences from radiometric and geochemical studies. Estuar. Coast. Shelf Sci. 43:4. doi: 10.1006/ecss.1996.0081
Davidson, K. E., Fowler, M. S., Skov, M. W., Doerr, S. Y., Beaumont, N., and Griffin, J. N. (2017). Meta-analysis reveals livestock grazing alters multiple ecosystem properties and services in salt marshes. J. Appl. Ecol. 54:5. doi: 10.1111/1365-2664.12892
De Deyn, G. B., Cornelissen, J. H. C., and Bardgett, R. D. (2008). Plant functional traits and soil carbon sequestration in contrasting biomes. Ecol. Lett. 11:5. doi: 10.1111/j.1461-0248.2008.01164.x
Derner, J. D., Boutton, T. W., and Briske, D. D. (2006). Grazing and ecosystem carbon storage in the North American great plains. Plant Soil. 280:1–2. doi: 10.1007/s11104-005-2554-3
Di Bella, C. E., Rodríguez, A. M., Jacobo, E., Golluscio, R. A., and Taboada, M. A. (2015). Impact of cattle grazing on temperate coastal salt marsh soils. Soil Use Manage. 31:2. doi: 10.1111/sum.12176
Donato, D. C., Kauffman, J. B., Murditarso, D., Kurnianto, S., Stidham, M., and Kannien, M. (2011). Mangroves among the most carbon-rich forests in the tropics. Nat. Geosci. 4, 293–297. doi: 10.1038/ngeo1123
Duarte, C. M., Losada, I. J., Hendriks, I. E., Mazarrasa, I., and Marbà, N. (2013). The role of coastal plant communities for climate change mitigation and adaptation. Nat. Clim. Change. 3:11. doi: 10.1038/nclimate1970
Dung, L. V., Tue, N. T., Nhuan, M. T., and Omori, K. (2016). Carbon storage in a restored mangrove forest in Can Gio Mangrove Forest Park, Mekong Delta, Vietnam. Forest Ecol. Manag. 380, 31–40. doi: 10.1016/j.foreco.2016.08.032
Elschot, K., Bakker, J., Temmerman, S., van de Koppel, J., and Bouma, T. (2015a). Ecosystem engineering by large grazers enhances carbon stocks in a tidal salt marsh. Mar. Ecol. Prog. Ser. 537, 9–21. doi: 10.3354/meps11447
Elschot, K., Bakker, J., Temmerman, S., van de Koppel, J., and Bouma, T. (2015b). “Experimental evidence for top-down control of carbon stocks in a tidal salt marsh,” In. Effects of Vegetation Patterns and Grazers on Tidal Marshes. Ph.D. thesis (Groningen: Rijksuiversiteit Groningen).
Emmet, B. A., Frogbrook, Z. L., Chamberlain, P. M., Griffiths, R., Pickup, R., Poskitt, J., et al. (2008). Countryside Survey Technical Report No. 3/07. Centre for Ecology and Hydrology, Natural Environment Research Council.
Facelli, J. M., and Pickett, S. T. A. (1991). Plant litter: its dynamics and effects on plant community structure. Bot. Rev. 57:1. doi: 10.1007/BF02858763
Ford, H., Garbutt, A., Jones, L., and Jones, D. L. (2012). Methane, carbon dioxide and nitrous oxide fluxes from a temperate salt marsh: grazing management does not alter global warming potential. Estuar. Coast. Shelf Sci. 113. doi: 10.1016/j.ecss.2012.08.002
Fox, W. M., Johnson, M. S., Jones, S. R., Leah, R. T., and Copplestone, D. (1999). The use of sediment cores from stable and developing salt marshes to reconstruct the recent historical contamination profiles in the Mersey estuary, UK. Mar. Environ. Res. 47:4. doi: 10.1016/S0141-1136(98)00123-8
Frank, A. B., Tanaka, D. L., Hofmann, L., and Follett, R. F. (1995). Soil carbon and nitrogen of the northern great plains grasslands as influenced by long-term grazing. J. Range Manage. 48:5. doi: 10.2307/4002255
French, J. R., and Spencer, T. (1993). Dynamics of sedimentation in a tide-dominated backbarrier salt marsh, Norfolk, UK. Mar. Geol. 110:3–4. doi: 10.1016/0025-3227(93)90091-9
Gedan, K. B., Silliman, B. R., and Bertness, M. D. (2009). Centuries of human driven change in salt marsh ecosystems. Annu. Rev. Mar. Sci. 1, 117–141. doi: 10.1146/annurev.marine.010908.163930
Graff, P., Aguiar, M. R., and Chaneton, E. J. (2007). Shifts in positive and negative plant interactions along a grazing intensity gradient. Ecology. 88:1. doi: 10.1890/0012-9658(2007)88[188:SIPANP]2.0.CO;2
Grime, J. P. (1974). Vegetation classification by reference to strategies. Nature. 250, 26–31. doi: 10.1038/250026a0
Haveren, B. P. (1983). Soil bulk density as influenced by grazing intensity and soil type on a shortgrass prairie site. J. Range Manage. 36:5. doi: 10.2307/3898346
Hayes, M. A, Jesse, A., Hawke, B., Baldock, J., Tabet, B., Lockington, D., et al. (2017). Dynamics of sediment carbon stocks across intertidal wetland habitats of Moreton Bay, Australia. Glob. Change Biol. 23:10. doi: 10.1111/gcb.13722
Himes-Cornell, A., Pendleton, L., and Atiyah, P. (2018). Valuing ecosystem services from blue forests: a systematic review of valuation of salt marshes, sea grass beds and mangrove forests. Ecosyst. Serv. 30, 36–48. doi: 10.1016/j.ecoser.2018.01.00
Holland, J. N., Cheng, W., and Crossley, D. A. J. (1996). Herbivore-induced changes in plant carbon allocation: assessment of below-ground C fluxes using carbon-14. Oecologia. 107:1. doi: 10.1007/BF00582238
Howarth, R. W. (1984). The ecological significance of sulfur in the energy dynamics of salt marsh and coastal marine sediments. Biogeochemistry. 1:1. doi: 10.1007/BF02181118
Hussein, A. H., and Rabenhorst, M. C. (2002). Modelling of nitrogen sequestration in coastal marsh soils. Soil Sci. Soc. Am. J. 66:1. doi: 10.2136/sssaj2002.3240
Jensen, A. (1985). The effect of cattle and sheep grazing on salt-marsh vegetation at Skallingen, Denmark. Vegetatio. 60:1. doi: 10.1007/BF00053910
Kang, L., Han, X., Zhang, Z., and Sun, O. J. (2007). Grassland ecostystems in China: review of current knowledge and research advancement. Philos. Trans. Roy. Soc. B. 362:1482. doi: 10.1098/rstb.2007.2029
Kiehl, K., Eischeid, I. E., Gettner, S., and Walter, J. (1996). Impact of different sheep grazing intensities on salt marsh vegetation in Northern Germany. J. Veg. Sci. 7, 99–106. doi: 10.2307/3236421
King, S. E., and Lester, J. N. (1995). The value of salt marsh as a sea defence. Mar. Pollut. Bull. 30:3. doi: 10.1016/0025-326X(94)00173-7
Kingham, R. J. (2013). The Broad-Scale Impacts of Livestock Grazing on Saltmarsh Carbon Stocks. Ph.D. thesis. Bangor: Bangor University.
Klumpp, K., Fontaine, S., Attard, E., Le Roux, X., Gleixner, G., and Soussana, J.-F. (2009). Grazing triggers soil carbon loss by altering plant roots and their control on soil microbial community. J. Ecol. 97:5. doi: 10.1111/j.1365-2745.2009.01549.x
Kuijper, D., and Bakker, J. P. (2004). Large-Scale Effects of a Small Herbivore on Salt-Marsh Vegetation Succession, A Comparative Study. Ph.D. thesis. Groningen: University of Groningen.
Mcleud, E., Chmura, G. L., Bouilln, S., Salm, R., Björk, M., Duarte, C. M., et al. (2011). A blueprint for blue carbon: towards an improved understanding of the role of vegetated costal habitats in sequestering CO2. Front. Ecol. Environ. 9:4. doi: 10.1890/110004
McNaughton, S. J. (1979). Grazing as an optimization process: grass-ungulate relationships in the Serengeti. Am. Nat. 113:5. doi: 10.1086/283426
McNaughton, S. J., Banyikwa, F. F., and McNaughton, M. M. (1998). Root biomass and productivity in a grazing ecosystem: the Serengeti. Ecology. 79:2. doi: 10.1890/0012-9658(1998)079[0587:RBAPIA]2.0.CO;2
McSherry, M. E., and Ritchie, M. E. (2013). Effects of grazing on grassland soil carbon: a global review. Glob. Change Biol. 19:5. doi: 10.1111/gcb.12144
Meyer, H., Fock, H., Haase, A., Reinke, H. D., and Tulowitzki, I. (1995). Structure of the invertebrate fauna in salt marshes of the Wadden Sea coast of Schleswig-Holstein influenced by sheep-grazing. Helgoländer Meeresun. 49, 1–4. doi: 10.1007/BF02368383
Middelburg, J. J., Nieuwenhuize, J., Lubberts, R. K., and van de Plassche, O. (1997). Organic carbon isotope systematics of coastal marshes. Estuar. Coast. Shelf Sci. 45:5. doi: 10.1006/ecss.1997.0247
Milotić, T., Erfanzadeh, R., Pétillon, J., Maelfait, J.-P., and Hoffman, M. (2010). Short-term impact of grazing by sheep on vegetation dynamics in a newly created salt-marsh site. Grass Forage Sci. 65, 121–132. doi: 10.1111/j.1365-2494.2009.00725.x
Möller, I., Spencer, T., French, J. R., Legget, D. J., and Dixon, M. (1999). Wave transformation over salt marshes: a field and numerical modelling study from North Norfolk, England. Estuar. Coast. Shelf Sci. 49, 411–426. doi: 10.1006/ecss.1999.0509
Möller, I., Spencer, T., French, J. R., Leggett, D. J., and Dixon, M. (2007). The sea-defence value of salt marshes: field evidence from North Norfolk. Water Environ. J. 15:2. doi: 10.1111/j.1747-6593.2001.tb00315.x
Morris, J. T., and Jensen, A. (1998). The carbon balance of grazed and non-grazed Spartina anglica saltmarshes at Skallingen, Denmark. J. Ecol. 86:2. doi: 10.1046/j.1365-2745.1998.00251.x
Morris, J. T., and Whiting, G. J. (1986). Emission of gaseous carbon dioxide from salt-marsh sediments and its relation to other carbon losses. Estuar. Coast. 9:1. doi: 10.2307/1352188
Mueller, P., Granse, D., Nolte, S., Do, H. T., Weingartner, M., Hoth, S., et al. (2017). Top-down control of carbon sequestration: grazing affects microbial structure and function in salt marsh soils. Ecol. Appl. 27:5. doi: 10.1002/eap.1534
Mueller, P., Ladiges, N., Jack, A., Schmiedl, G., Kutzbach, L., Jensen, K., et al. (2019). Assessing the long-term carbon-sequestraion potential of the semi-natural salt marshes in the European Wadden Sea. Ecosphere. 10:1. doi: 10.1002/ecs2.2556
Murray, P. J., Gill, E., Balsdon, S. L., and Jarvis, S. C. (2001). A comparison of methane emissions from sheep grazing pastures with different management intensities. Nutr. Cycl. Agroecosys. 60, 1–3. doi: 10.1023/A:1012654928177
Neuhaus, R., Stelter, T., and Kiehl, K. (1999). Sedimentation in salt marshes affected by grazing regime, topographical patterns and regional differences. Senck. Marit. 29:1. doi: 10.1007/BF03043134
Nolte, S., Mueller, F., Schuerch, M., Wanner, A., Esselink, P., Bakker, J. P., et al. (2013). Does livestock grazing affect sediment deposition and accretion rates in salt marshes? Estuar. Coast. Shelf Sci. 135, 296–305. doi: 10.1016/j.ecss.2013.10.026
Norris, K., Cook, T., O'Dowd, B., and Durdin, C. (1997). The density of redshank Tringa totanus breeding on the salt-marshes of the Wash in relation to habitat and its grazing management. J. Appl. Ecol. 34:4. doi: 10.2307/2405289
Olofsson, J., and Oksanen, L. (2002). Role in litter decomposition for the increased primary production in areas heavily grazed by reindeer: a litterbag experiment. Oikos. 96:3. doi: 10.1034/j.1600-0706.2002.960312.x
Olsen, Y. S., Dausse, A., Garbutt, A., Ford, H., Thomas, D. N., and Jones, D. L. (2011). Cattle grazing drives nitrogen and carbon cylingin a temperate salt marsh. Soil Biol. Biochem. 43:3. doi: 10.1016/j.soilbio.2010.11.018
Ostle, N. J., Levy, P. E., Evans, C. D., and Smith, P. (2009). UK land use and soil carbon sequestration. Land Use Policy. 26:1. doi: 10.1016/j.landusepol.2009.08.006
Pinares-Patino, C. S., D'Hpur, P., Jouany, J.-P., and Martin, C. (2007). Effects of stocking rate on methane and carbon dioxide emissions from grazing cattle. Agr. Ecosyst. Environ. 121, 1–2. doi: 10.1016/j.agee.2006.03.024
Proensky, L. M., Mueller, K. E., Augustine, D. J., and Derner, J. D. (2016). Thresholds and gradients in a semi-arid grassland: long-term grazing treatments induce slow, continuous and reversible vegetation change. J. Appl. Ecol. 53:4. doi: 10.1111/1365-2664.12630
R Core Team (2012). R: A Language and Environment for Statistical Computing. Vienna: R Foundation for Statistical Computing. Available online at: http://www.R-project.org/
Rauzi, F., and Hanson, C. L. (1966). Water intake and runoff affected by intensity of grazing. J. Range Manage. 19:6. doi: 10.2307/3895570
Reader, J., and Craft, C. (1999). Comparison of wetland structure and function on grazed and ungrazed salt marshes. J. Elisha Mitch. Sci. Soc. 115, 236–249.
Reeder, J. D., and Schuman, G. E. (2002). Influence of livestock grazing on C sequestration in semi-acid, mixed-grass and short-grass rangelands. Environ. Pollut. 116, 457–463. doi: 10.1016/S0269-7491(01)00223-8
Reid, R.S., Galvin, K.A., and Kruska, R.S. (2008). “Global significance of extensive grazing lands and pastoral societies: an introduction,” in Fragmentation in Semi-Arid and Arid Landscapes, eds K. A. Galvin, R. S. Reid, R. H. J. Behnke, and N. Thompson Hobbs (Heidleberg: Springer Netherlands), 1–24.
Richards, K. R. (2004). A brief overview of carbon sequestration economics and policy. Environ. Manage. 33, 545–558. doi: 10.1007/s00267-003-9111-8
Richardson, J. T. E. (2011). Eta squared and partial eta squared as measures of effect size in educational research. Edu. Res. Rev. 6, 135–147. doi: 10.1016/j.edurev.2010.12.001
Robinson, W.O. (1927). The determination of organic matter in soils by means of hydrogen peroxide. J. Agric. Res. 34, 339–356.
Rodwell, J.S., Pigott, C.D., Ratcliffe, D.A., Malloch, A.J.C., Birks, H.J.B., Proctor, M.C.F., et al. (2000). British Plant Communities. Volume 5: Maritime Communities and Vegetation of Open Habitats. Cambridge: Cambridge University Press.
Sammul, M., Kauer, K., and Köster, T. (2012). Biomass accumulation during reed encroachment reduces efficiency of restoration of Baltic coastal grasslands. Appl. Veg. Sci. 15:2. doi: 10.1111/j.1654-109X.2011.01167.x
Saunders, C. J., Megonigal, J. P., and Reynolds, J. F. (2006). Comparison of belowground biomass in C3- and C4-dominated mixed communities in a Chesapeake Bay brackish marsh. Plant Soil. 280, 1–2. doi: 10.1007/s11104-005-3275-3
Scanlon, D., and Moore, T. R. (2000). Carbon dioxide production from peatland soil profiles: the influence of temperature, oxic/anoxic conditions and substrate. Soil Sci. 165:2. doi: 10.1097/00010694-200002000-00006
Schipper, L. A., Mudge, P. L., Kirschnaum, M. U. F., Hedley, C. B., Golubiewski, N. E., Smaill, S. J., et al. (2017). A review of soil carbon change in New Zealand's grazed grasslands. New Zeal. J. Agr. Res. 60:2. doi: 10.1080/00288233.2017.1284134
Schumacher, B.A. (2002). United States Environmental Protection Agency. Methods for the Determination of Total Organic Carbon (TOC) in Soils and Sediments. Las Vegas, NV: Ecological Risk Assessment Support Center, Office of Research and Development.
Schuman, G. E., Reeder, D. J., Manley, J. T., Hart, R. H., and Manley, W. A. (1999). Impact of grazing management on the carbon and nitrogen balance of a mixed-grass rangeland. Ecol. Appl. 9:1. doi: 10.1890/1051-0761(1999)009[0065:IOGMOT]2.0.CO;2
Schuster, J. L. (1964). Root development of native plants under three grazing intensities. Ecology. 45:1. doi: 10.2307/1937107
Sharps, E., Smart, J., Mason, L. R., Jones, K., Skov, M. W., Garbutt, A., et al. (2017). Nest trampling and ground nesting birds: quantifying temporal and spatial overlap between cattle activity and breeding redshank. Ecol Evol. 7:16. doi: 10.1002/ece3.3271
Shepherd, D., Burgess, D., Jickells, T., Andrews, J., Cave, R., Turner, R. K., et al. (2007). Modelling the effects and economics of managed realignment on the cycling and storage of nutrients, carbon and sediments in the Blackwater estuary UK. Estuar. Coast. Shelf Sci. 73, 3–4. doi: 10.1016/j.ecss.2007.01.019
Shi, Z. (1992). Recent saltmarsh accretion and sea level fluctuations in the Dyfi Estuary, central Cardigan Bay, Wales, UK. Geo-Mar. Lett. 13:3. doi: 10.1007/BF01593192
Sollins, P., Homann, P., and Caldwell, B. A. (1996). Stabilization and destabilization of soil organic matter: mechanisms and controls. Geoderma. 74, 1–2. doi: 10.1016/S0016-7061(96)00036-5
Sousa, A. I., Lillebo, A. I., Pardal, M. A., and Cacador, I. (2010). The influence of Spartina maritima on carbon retention capacity in salt marshes from warm-temperate estuaries. Mar. Pollut. Bull. 61, 4–6. doi: 10.1016/j.marpolbul.2010.02.018
Stewart, K. E. J, Bourn, N. A. D., and Thomas, J. A. (2001). An evaluation of three quick methods commonly used to assess sward height in ecology. J. Appl. Ecol. 38:5. doi: 10.1046/j.1365-2664.2001.00658.x
Stumpf, R. P. (1983). The process of sedimentation on the surface of a saltmarsh. Esutar. Coast. Shelf Sci. 17:5. doi. 10.1016/0272-7714(83)90002-1
Tanentzap, A. J., and Coomes, D. A. (2012). Carbon storage in terrestrial ecosystems: do browsing and grazing herbivores matter? Biol. Rev. 87:1. doi: 10.1111/j.1469-185X.2011.00185.x
Tanner, C. B., and Mamaril, C. P. (1958). Pasture soil and compaction by animal traffic. Crop Sci. Soc. Am. 51:6. doi: 10.2134/agronj1959.00021962005100060007x
Valiela, I., Teal, J. M., Allen, S. D., Van Etten, R., Goehringer, D., and Volkmann, S. (1985). Decomposition in salt marsh ecosystems: the phases and major factors affecting disappearance of above-ground organic matter. J. Exp.l Mar. Biol. Ecol. 88:1. doi: 10.1016/0022-0981(85)90080-2
Van de Broek, M., Vandendriessche, C., Poppelmonde, D., Merckx, R., Temmerman, S., and Govers, G. (2018). Long-term organic carbon sequestration in tidal marsh sediments is dominated by old-aged allochthonous inputs in a macrtidal estuary. Glob. Change Biol. 24:6. doi: 10.1111/gcb.14089
Van der Wal, D., Pye, K., and Neal, A. (2002). Long-term morphological change in the Ribble Estuary, northwest England. Mar. Geol. 189, 3–4. doi: 10.1016/S0025-3227(02)00476-0
Verhoeven, K. J. F., Simonsen, K. L., and McIntyre, L. M. (2004). Implementing false discovery rate control: increasing your power. Oikos. 108:3. doi: 10.1111/j.0030-1299.2005.13727.x
Wallage, Z. E., Holden, J., and McDonald, A. T. (2006). Drain blocking: an effective treatment for reducing dissolved organic carbon loss and water discolouration in a drained peatland. Sci. Total Environ. 367, 2–3. doi: 10.1016/j.scitotenv.2006.02.010
Wang, Z., Zeng, D., and Partrick, W. H. J. (1996). Methane emissions from natural wetlands. Environ. Monit. Assess. 42:1–2. doi: 10.1007/BF00394047
Ward, S. E., Smart, S. M., Quirk, H., Tallowin, J. R. B., Mortimer, S. R., Shiel, R. S., et al. (2016). Legacy effects of grassland management on soil carbon depth. Glob. Change Biol. 22:8. doi: 10.1111/gcb.13246
Winfrey, M. R., and Ward, D. M. (1983). Substrates for sulfate reduction and methane production in intertidal sediments. Appl. Environ. Mircob. 45, 193–199.
Keywords: blue carbon, grazing, saltmarsh, broad-scale, environmental context
Citation: Harvey RJ, Garbutt A, Hawkins SJ and Skov MW (2019) No Detectable Broad-Scale Effect of Livestock Grazing on Soil Blue-Carbon Stock in Salt Marshes. Front. Ecol. Evol. 7:151. doi: 10.3389/fevo.2019.00151
Received: 31 October 2018; Accepted: 17 April 2019;
Published: 10 May 2019.
Edited by:
Divya Karnad, Ashoka University, IndiaReviewed by:
Peter Mueller, Universität Hamburg, GermanyReza Erfanzadeh, Tarbiat Modares University, Iran
Copyright © 2019 Harvey, Garbutt, Hawkins and Skov. This is an open-access article distributed under the terms of the Creative Commons Attribution License (CC BY). The use, distribution or reproduction in other forums is permitted, provided the original author(s) and the copyright owner(s) are credited and that the original publication in this journal is cited, in accordance with accepted academic practice. No use, distribution or reproduction is permitted which does not comply with these terms.
*Correspondence: Rachel J. Harvey, cmFjaGVsaGFydmV5MTRAZ21haWwuY29t