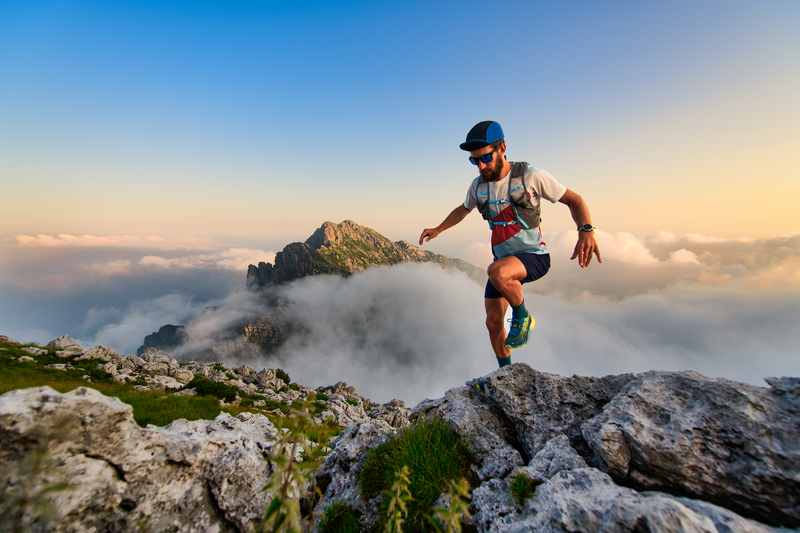
95% of researchers rate our articles as excellent or good
Learn more about the work of our research integrity team to safeguard the quality of each article we publish.
Find out more
REVIEW article
Front. Ecol. Evol. , 08 May 2019
Sec. Urban Ecology
Volume 7 - 2019 | https://doi.org/10.3389/fevo.2019.00136
This article is part of the Research Topic Trends in Urban Rodent Monitoring and Mitigation: Improving Our Understanding of Population and Disease Ecology, Surveillance and Control View all 15 articles
The strong innate fear response shown by laboratory rodents to predator cues could provide powerful and innovative tools for pest management. Predator cues are routinely used to induce fear and anxiety in laboratory rodents for pharmacological studies. However, research on the fear response induced by predator cues in different species of rodents in the wild has been inconclusive with results often contradictory to laboratory experiments. Potential explanations for this inconsistency include the prey's: (i) physiological state; (ii) parasite load; (iii) differential intensity of perceived threats; (iv) fear learning and habituation; and (v) information gathering. In this review, we first explore current knowledge on the sensory mechanisms and capabilities of rodents, followed by the discussion of each of these explanations within the context of their implications for the use of antipredator response as a pest rodent management tool. Finally, we make recommendations on potential solutions and strategies to resolve issues in rodent management related to these hypotheses.
Worldwide, rodents are prolific and pervasive pests, destroying crops, spreading disease, and causing enormous damage to infrastructure (Mills, 1999; Meerburg et al., 2009a,b). From a conservation perspective, introduced pest rodents have been linked to the demise of many native species around the world, particularly on islands (Atkinson, 1973, 1985; Capizzi et al., 2014). In several developing countries, rodents are considered the main cause of agricultural losses (Makundi et al., 1999). It is estimated that globally 77 million tons of food are lost annually due to rodent pests (John, 2014). In Asia alone, the annual consumption of food crops by rodents could feed 200 million people (Singleton, 2003). Due to rodents, rice harvest in Indonesia is reduced by 15% annually (Geddes, 1992). Tanzania loses US$45 million every year in reduced maize yield (Leirs, 2003) and in some areas of South America, rodent related damage to crops can amount to up to 90% of the total annual production (Rodriguez, 1993). A recent review on the impact of pest rodents in Africa, found that losses fall between 20 and 50% (Swanepoel et al., 2017). Overall, damage to pre and post-harvest crops affects approximately 280 million undernourished people worldwide (Stenseth et al., 2003; Meerburg et al., 2009b). Yet, the number of species of rodents that are consider pests, represents <10% of all the rodents species currently known (Singleton et al., 2007).
In most urban areas around the world, commensal rodents are common and live alongside humans in houses, buildings and other infrastructure such as sewers (Tobin and Fall, 2006). At high densities, rodents contaminate food, damage infrastructure, increase risk of fire by gnawing on electrical wiring and pose a risk to public health as diseases carriers (Tobin and Fall, 2006; Meerburg et al., 2009a; Almeida et al., 2013; Buckle and Smith, 2015). Wherever humans thrive, pest rodents do as well (Barnett, 2001; Lund, 2015).
The most common approach to rodent management follows the well-known framework of Integrated Pest Management (IPM; Singleton et al., 1999; Tobin and Fall, 2006). The IPM model is the combination of all available pest control methods with preventative measures to reduce subsequent pest population increases, while ensuring that these techniques are economically justified and do not pose a risk to human health and the environment (Food Agriculture Organization of the United Nations, 2018). IPM commonly includes monitoring, sanitation, physical interventions (e.g., exclusion, traps, deterrents), and ultimately rodenticides (Kaukeinen, 1994; Singleton et al., 1999; Bennett et al., 2003).
Physical interventions and the use of rodenticides can be particularly difficult to implement, due to difficulties intrinsic to rodent physiology and behavioral adaptations. Most pest rodent species show high levels of neophobia (Barnett, 1958; Barnett and Cowan, 1976; Meehan, 1984), both towards novel objects and tastes (Domjan, 1975; Rusiniak et al., 1979), which results in high levels of “trap-shyness” (Chitty, 1954; Taylor et al., 1974), and low bait acceptance (Brunton et al., 1993; Inglis et al., 1996). Rodents also learn from the experience of conspecifics (Lore et al., 1971); if conspecifics emit signals of distress—e.g., getting caught in a trap—they are less likely to approach the same area later on (Brudzynski and Chiu, 1995; Brechbühl et al., 2013; Haapakoski et al., 2018). Furthermore, the widespread use of rodenticides have induced the development of resistance in rodent populations to first and second-generation anticoagulant poisons (e.g., warfarin, bromadiolone, difenacoum, chlorophacinone; Thijssen, 1995; Pelz et al., 2005; Pelz, 2007; Rost et al., 2009; Buckle, 2013; Meerburg et al., 2014). Concurrently, the widespread use of these poisons can have considerable negative impacts on non-target wildlife (Howald et al., 1999; Eason et al., 2002; Lambert et al., 2007; Walker et al., 2008; Albert et al., 2010; Dowding et al., 2010; Lima and Salmon, 2010; Thomas et al., 2011; Gabriel et al., 2012; Elliott et al., 2014; Coeurdassier et al., 2018; Lohr and Davis, 2018; Rattner and Mastrota, 2018). The development of alternative and innovative ways of managing rodent pests is therefore of high importance.
History can provide inspiration for new and innovative ways to manage rodent pests. One of the oldest methods of controlling rodents is the use of cats. Cats started their domestication serendipitously as commensal relationships with humans, feeding upon the rodents that infested the stored grain of farmers (Clutton-Brock, 1999). Yet, the effect cats have on rodent populations may be more complex that just population reduction. The effect predators have on prey is not only defined by lethal interactions (Taylor, 1984), but also non-lethal effects. For example, in agricultural settings, the protection guard dogs provide to livestock is through deterrence instead of actual attacks and killing of predators (Hansen and Bakken, 1999; van Eeden et al., 2017). Hence, analogous rodent-deterrence strategies may be of value.
Risk of predation is ubiquitous to almost all taxa, and as such all species show some level of antipredator defense (Freeland, 1991; Caro, 2005). These defenses can be constitutive (e.g., spines in porcupines, thorns in plants; Fraenkel, 1959; Tollrian and Harvell, 1999) or inducible (e.g., morphology in tadpoles, coloration in some crustaceans, and behavioral modifications; Kerfoot and Sih, 1987; Harvell and Tollrian, 1999; Tollrian and Harvell, 1999; Creel et al., 2007; Schoeppner and Relyea, 2009). Constitutive defenses are favored when risk is constantly high and/or defenses are cheap, while inducible defenses are favored when risk is variable and defenses are costly (Tollrian and Harvell, 1999). Inducible defenses allow control on defense expression according to risk level, thus reducing the costs associated with it (Tollrian and Harvell, 1999). Inducible antipredator responses are expected to evolve only if the costs associated with them are offset by their effectiveness in reducing the rate of direct predation.
The antipredator responses and their associated costs drive an evolutionary arms race between predator and prey, and constitute what is known as the “ecology of fear” (Brown et al., 1999), “degree of fear” (Stankowich and Blumstein, 2005), or “cost of fear” (Martin, 2011). The costs of anti-predator responses can include reduced survival (Dudeck et al., 2018; MacLeod et al., 2018); growth (Pangle et al., 2007); fecundity (Ruxton and Lima, 1997; Naidenko et al., 2003; Voznessenskaya et al., 2003; Fuelling and Halle, 2004; Creel et al., 2007) and reproduction (Zanette et al., 2011; Bonnington et al., 2013; Dudeck et al., 2018). More recently, limited evidence have shown that predation risk can drive an increase in current reproductive investment with associated costs to future reproduction (Duffield et al., 2017; Haapakoski et al., 2018). In some extreme cases, fear can induce the development of chronic stress conditions similar to Post-Traumatic Stress Disorders (PTSD) not only in humans, but also in rodents, primates and rabbits (Clinchy et al., 2013). These costs are sometimes more important to the prey population than the lethal effects themselves (Brown and Alkon, 1990; Schmitz et al., 1997; Matassa and Trussell, 2011).
It is theoretically possible to use these non-consumptive costs as a way managing pest rodent populations (Singleton et al., 1999). Pest controllers could manipulate how commensal rodents perceive predation risk to deter them from areas of interest (e.g., crops, food storage facilities). The Landscape of Fear (LOF) framework is a theoretical tool that allows measurement of the way an animal perceives its environment, based on a trade-off between resources and safety, linked to specific areas of available habitat (Laundré et al., 2001), and thus is a spatial representation of the use of habitat by a prey species. This framework has been supported across a wide range of systems (Bleicher, 2017). The LOF is considered the basis in which the use of perceived predation risk as a management tool against pest rodents can be built upon (Krijger et al., 2017).
A major obstacle in the development of a fear-inducing rodent management technique is the variation in anti-predator responses displayed by wild rats and mice in comparison with their laboratory counterparts. Laboratory rodents respond to a myriad of different predator cues (Vernet-Maury et al., 1984; Blanchard R.J et al., 1991; Dielenberg and McGregor, 2001; Mongeau et al., 2003; Litvin et al., 2007; Kendig et al., 2011; Bowen et al., 2013; Wallace et al., 2013; Yilmaz and Meister, 2013; Ayral et al., 2015), and also demonstrate anxiety-like behaviors, often utilized to model the human condition (Apfelbach et al., 2005; Staples et al., 2008). However, evidence of wild rodents responding to predator cues is inconclusive. Studies with captive wild-type brown rats (Rattus norvegicus) (Berdoy and Macdonald, 1991; Macdonald et al., 1999) and wild caught black rats (Rattus rattus) (Burwash et al., 1998) showed antipredator responses consistent to those of their laboratory counterparts. Some field studies demonstrated that wild black rats show aversion to fox and cat feces (Banks, 1998), as well as changes in habitat use in the presence of dogs and cats (Mahlaba et al., 2017). In contrast, other studies have shown either no effect of ferret, cat or mongoose odors in black rat visitation (Garvey et al., 2017) and foraging (Bramley and Waas, 2001); and in more extreme cases, black rats were attracted and visitation increased in response to cat body odor (Carthey and Banks, 2016).
Several explanations for the variability in rodent responses to predator cues have been put forward, namely the prey's: (i) physiological state (Abrams, 1994); (ii) parasite loads (Macdonald et al., 1999); (iii) differential intensity of perceived threats (Kavaliers and Choleris, 2001); (iv) fear learning and habituation (Schulte, 2016); and (v) information gathering (Parsons et al., 2017). In this review, we first explore the current knowledge on the sensory mechanisms and capabilities of rodents, followed by the discussion of each of these explanations within the context of their implications for the use of antipredator response as a pest rodent management tool.
Before an animal responds to a predator, it needs to be able to detect its presence. Rodents can detect and respond to visual (Wallace et al., 2013; Yilmaz and Meister, 2013); auditory (Blanchard R.J et al., 1991; Mongeau et al., 2003; Litvin et al., 2007), and olfactory (Vernet-Maury et al., 1984; Dielenberg and McGregor, 2001; Kendig et al., 2011; Bowen et al., 2013; Ayral et al., 2015) predator cues, highlighting that their sensory capabilities are highly tuned to predator detection.
Laboratory rodents are commonly used as models to study the mammalian visual system (Hughes, 1979; Remtulla and Hallett, 1985; Legg and Lambert, 1990; Berardi and Maffei, 1999; Zoccolan et al., 2009). As rodents are predominantly prey species, they have laterally facing eyes that allow for a panoramic field of view that extends forwards and also covers the back of the animals head, enhancing potential threat detection (Hughes, 1979; Remtulla and Hallett, 1985). Through eye movement alone, rodents are capable of overlapping the fields of view of both eyes to obtain binocular vision, at the loss of a complete panoramic field of view (Wallace et al., 2013). Binocular vision is important for judging distance (Russell, 1932; Legg and Lambert, 1990) and visual acuity (Hughes, 1979; Remtulla and Hallett, 1985; Zoccolan et al., 2009), while a panoramic monocular vision allows for wide surveillance (Hughes, 1979; Remtulla and Hallett, 1985).
Due to the reliability of visual cues—i.e., seeing a predator is a perfect clue that there is a high risk of predation—most species, including rodents, respond to oversimplified representations of predators (Kavaliers and Choleris, 2001). Some of these representations can encompass only size (Hanson and Coss, 1997; Mathis and Vincent, 2000), shape (Coss and Ramakrishnan, 2000; Emile and Barros, 2009), coloration (Kelley and Magurran, 2003), movement (Yilmaz and Meister, 2013), or frontally positioned eyes (Topál and Csányi, 1994). Rodents in particular, are highly sensitive to movement (Wallace et al., 2013; Yilmaz and Meister, 2013). When exposed to an oversimplified looming stimulus simulating a raptor, laboratory rats maintain overhead binocular vision (Wallace et al., 2013), thus, enabling them to judge the raptor's elevation.
Mice and rats are particularly attuned to detect high frequency sounds, often higher than humans have the ability to detect (Heffner and Heffner, 2007, 2008). Accordingly, these rodents commonly produce high-frequency sounds that have been linked to conspecific communication (Portfors, 2007). In laboratory rodents, con-specific high-frequency alarm calls are used to communicate threats (Brudzynski and Chiu, 1995). However, rodents are also capable of detecting lower frequency sounds (Heffner and Heffner, 2007, 2008). Studies in voles and gray squirrels have shown that playback of raptor calls can incite antipredator responses (Bohls and Koehnle, 2017; Lyly et al., 2018).
In most mammals, olfaction is the most developed sensory system (Eisenberg and Kleiman, 1972), and rodents are no exception (Vernet-Maury et al., 1984; Dielenberg and McGregor, 2001; Kendig et al., 2011; Bowen et al., 2013; Ayral et al., 2015). In contrast with the singularity of visual (i.e., retina) and auditory (i.e., ear) sensing, smells are processed by multiple distinct olfactory systems, involving distinct receptor organs and central neuronal processes involved in detection (Chamero et al., 2012; Ma, 2012). The main olfactory system processes scents and flavors, while the accessory olfactory system processes con-specific and heterospecific chemical cues (pheromones and kairomones) (Ma, 2012).
Predator recognition by olfactory cues does not require cortical information processing (Canteras et al., 2015). Discrimination of different odorants is achieved by the narrow sensitivity to chemical cues from each receptor type in the accessory olfactory system (AOS; Mucignat-Caretta, 2010; Ma, 2012; Canteras et al., 2015; Tromelin, 2016). In laboratory rodents, different predator odors activate receptors from distinct olfactory subsystems (Canteras et al., 2015). Carnivore urine activates TAAR4 neurons in the main olfactory epithelium (Ferrero et al., 2011; Dewan et al., 2013), cat fur activates vomeronasal organ receptors (McGregor et al., 2004), and stoat anal gland smells activate receptors in the Grueneberg ganglion (Pérez-Gómez et al., 2015). Therefore, activation of specific receptors allows for the recognition of specific predators. In the following section we will describe how after detection and recognition is achieved an appropriate response is induced. Interestingly, rodents are also capable of utilize olfactory signaling from conspecific in order to asses predation risk (Abel, 1991; Kikusui et al., 2001; Haapakoski et al., 2018). And in some cases these pheromones activate similar receptors as predator odors (Brechbühl et al., 2008, 2013).
On predator detection all sensory signals, regardless of their origin (i.e., visual, auditory or chemical), converge in the amygdala (Krettek and Price, 1977; Campeau and Davis, 1995; Chamero et al., 2012; Ma, 2012; Pérez-Gómez et al., 2015). Amygdala signaling initiates sensory and motor response coordination to the predatory threat (Miller and Vogt, 1984) provoking appropriate behavioral and physiological responses (Campeau and Davis, 1995), such as freezing or evasive behaviors. Thus, differences in rodents' antipredator responses are expected to arise because of differences in detection, recognition, or response to predator cues, mediated through complex but converging neural signaling. Modulation of this signaling pathway can occur at every stage based on the individual's internal state, prior experience, or the context, leading to observable variability in antipredator responses.
The risk of predation is ubiquitous to all animals (Freeland, 1991; Caro, 2005), however so is the need to forage and acquire resources (Charnov, 1979; Stephens and Krebs, 1986). Yet, laboratory rodents are commonly kept in standard captive husbandry conditions with ad libitum access to food and water, and controlled environmental variables such as temperature, humidity and photoperiod (Allmann-lselin, 2000; Hedrich and Bullock, 2004; National Research Council, 2010). In comparison, wild rodents must cope with a variety of environmental factors, while balancing the risk of predation and resource acquisition to optimize their fitness (Abrams, 1994).
Starvation alters the antipredator response in both laboratory (Shoham et al., 2000; Verma et al., 2016) and wild rodents. Starved captive wild-caught Anderson's gerbils (Gerbillus andersoni allenbyi) increase foraging despite predation risk (Berger-Tal and Kotler, 2010; Berger-Tal et al., 2010). Free-ranging deer mice (Peromyscus maniculatus) (Morris, 1997; Davidson and Morris, 2001) and house mice (Mus musculus domesticus) (Ylönen et al., 2002) reduce their foraging at the cost of increased predation risk, when the population density is high driving higher intraspecific competition and thus lower internal energetic state (Bedoya-Pérez et al., 2013).
Relative resource quality also alters the intensity of anti-predator responses (Thorson et al., 1998). Fox squirrels (Sciurus niger) (Brown and Morgan, 1995), Namaqua mouse (Micaelamys namaquensis) (Abu Baker and Brown, 2012), Anderson's gerbils (G. andersoni allenbyi), the greater Egyptian gerbil (G. pyramidurn) (Garb et al., 2000), Merriam's kangaroo rats (Dipodomys merriami), and pocket mice (Chaetodipus spp.) (Leaver and Daly, 2003) forage more intensely on highly nutritious food regardless of predation risk. Similarly, fox squirrels (S. niger), gray squirrels (S. carolinensis) (Schmidt et al., 1998), as well as the African unstriped ground squirrel (Xerus rutilus) (Fanson et al., 2010), reduce foraging on poor quality food and become more sensitive to predation risk (Bedoya-Pérez et al., 2013).
Other physiological, developmental, and reproductive factors can alter an animal's anti-predator response. For example, immunochallenged white-footed mice (Peromyscus leucopus) forage more and are less selective in their habitat use despite the risk of predation (Schwanz et al., 2011, 2012), and Anderson's gerbils (G. andersoni allenbyi) and the greater Egyptian gerbil (G. pyramidurn) increase foraging efforts during the reproductive season (Kotler et al., 2004). More relevant to rodent management, we know that pest rodents infected with Toxoplasma gondii showed a reduced aversion to predators (Berdoy et al., 1995a, 2000; Webster, 2007), we will this discuss this particular case in more detail in the following section.
To exploit anti-predator responses as a pest management tool, it is essential to recognize that wild rodents are not well-fed, homeostatic animals like their laboratory counterparts. If resources are low, and pest rodents are at risk of starvation, these animals are expected to show less-pronounced anti-predator behaviors than those shown by laboratory animals (Abrams, 1994).
Parasite loads can alter an animals perceived predation risk either by altering their internal physiological state (Schwanz et al., 2011, 2012), or through more complex mechanisms involving modulation of neuronal pathways (Berdoy et al., 1995a; Raveh et al., 2011). The complete disruption of anti-predator response in rodents infected by Toxoplasma gondii has received wide attention in recent years (Webster, 2007).
Toxoplasma gondii is a parasitic protozoan capable of infecting all mammals, including humans (Hutchison et al., 1969). Domestic cats and other felines are the final host of the parasite, and are the only animals known to shed the parasite's oocyst in their faces (Hutchison et al., 1969). Transmission of the parasite can occur from cat to cat, but more commonly involves an intermediate host, such as a rodent. Here the parasite multiplies and forms cysts in almost every organ, particularly in the brain (Hutchison et al., 1969; Berdoy et al., 1995b). When a cat predates upon an infected rodent, the parasite passes to the final host where sexual reproduction occurs (Berdoy et al., 1995b).
One of the first studies demonstrating a connection between T. gondii infections and changes in behavior showed that laboratory rats and mice were cognitively impaired by the parasite (Piekarski et al., 1978). Subsequent studies have showed that Toxoplasma-infected rats and mice display deficits in both learning capacities (Witting, 1979) including novel object recognition (Hutchison et al., 1980; Webster et al., 1994). It was suggested that the reduction in neophobia could cause rats to be more susceptible to predation (Webster et al., 1994). It was later demonstrated that Toxoplasma-infected rats are more likely to approach areas with signs of cats, although retaining certain level of innate aversion (Berdoy et al., 1995a). These findings were confirmed by the demonstration that, compared to non-infected rats, Toxoplasma-infected rats visited areas treated with cat urine more often that control areas or areas treated with rabbit urine (Berdoy et al., 2000; Webster, 2007).
Toxoplasma gondii likely does not alter the sensory detection of the threat (Vyas et al., 2007a), but instead modifies the learning processes specifically related to cat odor (Vyas et al., 2007a,b). Neurobiologically, T. gondii causes epigenetic changes in the DNA methylation in the medial amygdala causing greater expression of arginine vasopressin promoter (Hari Dass and Vyas, 2014). The infection also causes retraction of dendritic processes in basolateral amygdala neurons, reducing the amount of circulating corticosterone (Mitra et al., 2012). A reduction in corticosterone disrupts both the fight or flight response as well-fear memory consolidation (Stephens and Wand, 2012). Behaviorally, infected laboratory rats show reduced anxiety-like behaviors in exploration-based testing, but not during social interaction testing (Gonzalez et al., 2007). This may be because T. gondii favors vertical transmission (i.e., cat predation) over horizontal transmission (i.e., infected parents to offspring; Vyas and Sapolsky, 2010). However, research shows that the mechanisms involved in behavioral alterations in male rodents increases sexual arousal (House et al., 2011) and attractiveness (Dass et al., 2011), potentially allowing the parasite to be transmitted sexually and congenitally (Beverley, 1959, 1976; Dass et al., 2011).
In terms of rodent management, undoubtedly T. gondii can have profound consequences for the use of anti-predator responses as a tool. However, these consequences are dependent on the prevalence of the infection in the targeted pest rodent population. It is unlikely that this protozoan is the cause of the variation in response between laboratory and field studies, when the prevalence in the field is appreciatively low (Carthey and Banks, 2016). In cases where the prevalence may be high, the disruption of antipredator behavior by T. gondii could be used synergistically with predator cues. That is, other management tools (e.g., lethal and non-lethal traps) could be deployed at the same locations as predator cues, thus infected rodents could be removed, while uninfected may be repelled. This method may reduce the prevalence of T. gondii, not only in the rodent population, but at the community level. Reductions in the number of infected carriers would benefit non-specific host species that may be vulnerable to the infection, such as humans (Tenter et al., 2000; Dubey and Jones, 2008) and several endangered species that are more susceptible to develop negative symptoms from the infection [e.g., Eastern quoll, Dasyurus viverrinus (Fancourt and Jackson, 2014; Fancourt et al., 2014), Tamar wallabies, Macropus eugenii, and Bennett's wallabies, Macropus rufogriseus (Dubey and Crutchley, 2008)].
Due to the extensive and ever-growing body of knowledge on the mechanisms and potential consequences of T. gondii, we have focused on this particular parasite. However, little is known about the potential effects that other parasites may have in the anti-predator behavior of pest rodents. Some evidence suggests that some infections and diseases may have overarching population level consequences such as increase in reproduction (Duffield et al., 2017).
There are considerable differences between laboratory and field studies in the testing of rodent responses to fear stimuli. Laboratory rodents are usually tested in spatially constrained arenas, where they are presented with a single proximal stimulus. Field or semi-captive studies normally occur at much larger spatial scales, and animals are exposed to varying environmental conditions as well as the specific stimulus of interest. These differences can have important implications to the interpretation of the responses measured (Lima, 1998; Lima and Bednekoff, 1999). Allenbyi's gerbils (G. allenbyi) and the greater Egyptian gerbil (G. pyramidurn) exposed to Barn owls (Tyto alba) at close proximity show a greater reduction in activity than when presented with the same predator in a semi-captive setting (Abramsky et al., 1996). Similarly, house mice (M. musculus domesticus) (Dickman, 1992), bank voles (Clethrionomys glareolus), and meadow voles (Microtus pennsylvanicus) (Perrot-Sinal et al., 1996, 1999, 2000) show a strong aversion and reduce activity when exposed to mustelid odor in relatively small enclosures. Conversely, in large scale enclosures, hairy-footed gerbils (Gerbillurus tytonis) (Hughes and Ward, 1993), gray-tail voles (Microtus canicaudus) and bank voles (C. glareolus) show little to no anti-predator response. This pattern is also observed in other small non-rodent mammals (Ward et al., 1997) and fish (Fraser and Huntingford, 1986; Irving and Magurran, 1997).
Kavaliers and Choleris (2001) suggested that the differences between laboratory and field results may be due to differences in the intensity of the cue. They argue that laboratory animals commonly experience single intense cues while animals in field experiments, although sometimes exposed to the same type of cues, can also assess predation risk by integrating several other relevant cues (e.g., habitat structure, vision, odors, sound) simultaneously (Kavaliers and Choleris, 2001). Thus, the cue of interest (i.e., the cue manipulated by the experimenter) is “drowned out” by the information contained in the other signals. Additionally, it has been suggested that very high predation risk may, counterintuitively, reduce some antipredator responses and increase others (e.g., vigilance vs. time allocation; Lima, 1998). For example, at very high levels of risk, animals are expected to completely avoid an area either by moving away (i.e., habitat partitioning) or by hiding until the risk is reduced (i.e., time partitioning). While at low levels of predation risk, animals may choose to remain in the area but increase vigilance instead (Lima, 1998). This has been demonstrated in desert rodents (Abramsky et al., 1996) and consistently in rats (Blanchard D.C et al., 1991; Blanchard et al., 1993, 1998).
Hence, if a pest rodent management strategy is to be effective, consideration of the intensity and the distribution of the predator cues is essential. Here, we suggest that utilizing a combination of cues may prove more effective (e.g., predator odor paired with either predator call or conspecific alarm playback).
Before any animal can respond appropriately to reduce its risk of predation, it needs to be able to perceive and estimate such risk. Animals can use ultimate or proximate cues to estimate the risk of predation (Kavaliers and Choleris, 2001). Ultimate cues constitute the actual detection of a predator itself; these could be visual (Magurran and Girling, 1986; Atkins et al., 2017), auditory (Smith et al., 2017; Suraci et al., 2017b), or tactile (Kavaliers and Choleris, 2001). While proximate cues are commonly of a chemical nature, such as odors (Parsons et al., 2017). Ultimate cues convey immediate risk (i.e., the predator is present here right now), while proximate cues convey temporally dependent risk (Parsons and Blumstein, 2010; Parsons et al., 2017) (i.e., the predator was here sometime in the past; Parsons and Blumstein, 2010; Parsons et al., 2017). However, in most cases, these cues are encountered simultaneously—i.e., if an animal sees a predator, it can likely detect the predator by audition and olfaction. By separating the effects of different types of predator cues, each can convey different kinds of information—i.e., presence of a potential threat vs. identity of the threat (Blumstein et al., 2000; Mathis and Vincent, 2000). Ultimate cues of predation are potentially more reliable and convey a good estimate of the actual risk of predation. Proximate cues are more variable in nature, and can provide an underestimation of risk if predators are present, or an overestimation when they are absent (Lima and Dill, 1990; Abrams, 1994; Lima, 1998).
Once a prey animal detects a predator cue it must be able to recognize and assess the risk associated with it, leading to a fear response. In the brain, fear can be categorized in two distinct modes, innate and learnt (Canteras et al., 2015). Innate fear refers to the defensive response to aversive stimuli with no previous experience of such stimulus (Canteras et al., 2015; Parsons et al., 2017). Learned fear is the development of conditioned fear behaviors—i.e., defensive responses to a innocuous stimulus or context, shown after repeated pairings of the innocuous stimulus and an adverse one (Rescorla and Wagner, 1972).
Innate fear is also known as “species memory” or “phyletic memory” (Canteras et al., 2015), and it has been described in a myriad of different taxa: including invertebrates (Dalesman et al., 2007); fish (Berejikian et al., 2003; Vilhunen and Hirvonen, 2003); amphibians (Semlitsch and Reyer, 1992); birds (Veen et al., 2000; Göth, 2001); marsupials (Anson and Dickman, 2013), rabbits (Monclús et al., 2005), rodents (Dielenberg and McGregor, 2001; Bowen et al., 2012, 2013; Parsons et al., 2017); ungulates (Chamaillé-Jammes et al., 2014); and primates (Gould and Sauther, 2007). In mammals, the neurocircuitry that categorizes innate fear responses is initiated by an increase in Fos expression in the posteroventral part of the medial amygdalar (MEApv) and in the dorsomedial part of the ventromedial hypothalamic nucleus (VMHdm) (Pérez-Gómez et al., 2015). The activation of the dorsomedial and central divisions of the VMH (VMHdm/c) are linked with the initiation of a series of context-dependent somatomotor and autonomic defensive behaviors, including generalized passive hiding and freezing responses, as well as running and jumping (Wang et al., 2015). Innate fear response, and the initiation of autonomic defensive behaviors, could therefore be considered the “default” mechanistic mode of defense when exposed to a novel predator cue. However, the future fear response to the same cue is not always fixed and can be modulated by experience; this is the paradigm of “habituation” (Rankin et al., 2009; Blumstein, 2016). Habituation is the reduction of a natural response to a stimulus as a consequence of repetitive exposure (Davis, 1970; Staddon, 1993). This can represent a major obstacle in the application of anti-predator responses for wildlife management (Bomford and O'Brien, 1990; Koehler et al., 1990; Samia et al., 2015; Blumstein, 2016). Habituation occurs when short-term memory suppresses the natural response to a recent stimulus (Staddon, 1993). Yet, if an animal fails to respond to a stimulus that signals an increase in predation risk, that animal is bound to suffer predation. Thus, it raises questions as to why habituation is widespread among several different taxa and stimuli types (Davis, 1970; Williams et al., 1990; Talling et al., 1998; Nowak et al., 2014) and why animals are not fearful at all times. This is explained by the fact that antipredator responses can be expensive (Ylönen and Brown, 2007; Martin, 2011; LaManna and Martin, 2016), and that not all stimuli can be regarded as honest. There are fundamental differences between the “actual” risk of predation and the “perceived” risk of predation (Creel, 2018), and most species have the cognitive tools to reduce the chances of “false positives” by learning.
After estimating the level of risk, an animal must be able to respond to reduce that risk. There are several stages to the predation process, through which antipredator defenses can act to reduce risks. Prey can reduce the probability of: being detected by the predator; that detection will lead to an attack; that an attack will lead to death or serious injury; and being the individual that is killed (Hamilton, 1971; Turner and Pitcher, 1986; Uetz and Hieber, 1994). To achieve this, animals can: (i) avoid a high risk area (Schmitz et al., 1997; Ojeda and Muñoz, 1999; Wirsing et al., 2008; Mao et al., 2010); (ii) wait until a risk decreases to become active (Lima and Bednekoff, 1999; Kotler et al., 2002; Valeix et al., 2009); (iii) reduce foraging (Brown et al., 1988; Herman and Valone, 2000; Altendorf et al., 2001); (iv) increase vigilance (Childress and Lung, 2003; Cresswell et al., 2003; Fortin et al., 2004; Embar et al., 2011; Iribarren and Kotler, 2012); (v) discourage predation by direct signaling (FitzGibbon and Fanshawe, 1988), (vi) employ active defenses (Corcoran and Conner, 2012); and (vii) aggregate with con-specifics (Hamilton, 1971; Pulliam, 1973; Bowen et al., 2013). In natural systems, these strategies are usually effective in unison (Kotler et al., 2010). Thus, predator defenses can be behavioral (Lima, 1990; Altendorf et al., 2001; Abramsky et al., 2002), morphological (Agrawal and Fishbein, 2006), physiological (Lima, 1998; van Donk et al., 1999), or ecological (Ojeda and Muñoz, 1999; Wirsing et al., 2008; Mao et al., 2010). These defenses are associated with non-consumptive costs and, in order to remain in the population, these costs need to be offset by a reduction in the “actual” risk of predation (Creel, 2018); these costs to risks ratio is what drives habituation.
Laboratory rodents demonstrate defensive responses to predators without previous experience (Parsons et al., 2017). However, this response is not unchallengeable, as prolonged exposure to predator cues reduces anti-predator behavior in laboratory rats (Williams et al., 1990). Moreover, it is well-understood that varying levels of predation risk can shape wild rodent anti-predator behavior (Brown et al., 1999; Ylönen and Brown, 2007). Thus, rodent antipredator responses seem to be non-binary (i.e., not simply “on and off”), and are shaped by fear learning (Staples et al., 2005).
Fear learning or contextual conditioning involves a different, although related pathway to innate fear neurocircuitry. The current working model for fear learning involves sequential activation, signaling, and feedback, primarily between four brain regions well-characterized for their role in fear acquisition and consolidation (McNally et al., 2011). This model describes how fear learning occurs when the difference between the actual vs. the expected intensity of a aversive unconditioned stimulus is encoded by an error signal (Rescorla and Wagner, 1972). McNally et al. (2011) it mostly involves conditioning with shock rather than with predator-related cues. However other studies have looked at conditioning with cat odor (e.g., Staples et al., 2005). When rats were placed in a context where they previously encountered a predator odor they displayed brain activation in a subset of the regions activated by the predator odor itself: this included the dorsal premammillary nucleus, ventrolateral periaqueductal gray, cuneiform nucleus and locus ceruleus (Staples et al., 2005). Little activation was seen in the amygdala or hippocampus. These results show that stimuli associated with predatory threat come to activate similar brain regions to the threat stimulus itself. If an animal experiences an unexpected aversive stimulus (e.g., predator attack), then the actual intensity of the stimulus will be higher than the expected, thus the stimulus would drive fear learning. Conversely, if an aversive stimulus—e.g., predator attack—was expected—e.g., encounter with a predator cue –, then the expected and actual intensity of aversive stimulus will match, and fear learning does not occur. Finally, when the actual intensity of the aversive stimulus is lower than the expected intensity—e.g., perceived predator cue but predator does not attack– then fear learning extinction occurs—i.e., habituation (Schaller, 1972; McNally et al., 2011).
In mammals, fear learning involves complex neural circuitry within the amygdala (McNally et al., 2011). When an aversive stimulus is detected (e.g., predator attack), this activates spinal and trigeminal dorsal horn neurons that project to the midbrain periaqueductal gray (PAG; McNally et al., 2011). Signaling then travels from the PAG, through the midline and intralaminar thalamus, to the dorsomedial prefrontal cortex (dmPFC) and lateral amygdala (LA) depolarizing pyramidal neurons (McNally et al., 2011). Associative fear learning is achieved when the co-occurrence of the aversive stimulus (e.g., predator attack) with an associated stimulus (e.g., predator cue) strengthen the thalamic and cortical afferent inputs to LA through N-methyl-D-aspartate receptor (NMDA)-mediated long term potentiation (McNally et al., 2011). Consequently, future exposure to the associated stimulus (e.g., predator cue) activates LA projection neurons to the central amygdala (CeA), leading to inhibition of the ventrolateral PAG (vlPAG), and inciting an antipredator response (McNally et al., 2011). Repeated exposures to the associated stimulus alone causes weakening of the auditory thalamic and cortical afferent inputs to LA pyramidal neurons through long term depression at NMDA receptors (McNally et al., 2011).
Learning aids in the recognition of threats, but through conditioning, it is the mechanism by which animals also estimate predation risk (Bolles, 1970; Crawford and Masterson, 1982; Cook and Mineka, 1990; Curio, 1993). There are many examples of different taxa that show certain innate responses to predators: from finches (Curio, 1993), moose (Berger et al., 2001), black-tail deer (Chamaillé-Jammes et al., 2014), Hokkaido deer (Osada et al., 2014), rabbits (Monclús et al., 2005), deer mice (Coss, 1999), ground squirrels (Hirsch and Bolles, 1980), and laboratory mice (Pérez-Gómez et al., 2015). But, more importantly, these innate predator responses can be enhanced or modified through fear learning (Berger et al., 2001). However, individual fear learning requires an animal to experience an adverse predator encounter directly, which may not be conducent to future survival. Thus, to acquired “knowledge” putatively through conspecifics is more advantageous (Russon, 1997).
Social learning occurs when an animal acquires information (i.e., the observer) by witnessing the actions of another, more experienced individual (i.e., the demonstrator; Heyes, 1994; Choleris and Kavaliers, 1999). The first taxa where social learning was characterized was fish (Von Frisch, 1942). Nowadays, social learning has been reported not only in other fish species (Chivers et al., 1995; Mirza and Chivers, 2000; Brown and Laland, 2005), but also birds (Curio et al., 1978; Curio, 1988; Magurran, 1989; Martínez et al., 2017); marsupials (Griffin and Evans, 2003); ungulates (Berger et al., 2001); primates (Cook et al., 1985; Bartecki and Heymann, 1987; Mineka and Cook, 1988; Cook and Mineka, 1990; Srivastava, 1991); laboratory rats (Lore et al., 1971); and mice (Kavaliers et al., 2001a,b; Sanders et al., 2013).
Both individual and social fear learning are widely used in wildlife management programs with species conservation goals (Griffin et al., 2001), yet has been somewhat neglected in the application of fear as a management tool. Schulte (2016) argues that when using artificial predator cue to alter the perceived predation risk by pest animals, a Batesian mimicry type dilemma is difficult to avoid. Batesian mimicry occurs when a non-dangerous species mimics the appearance, smell and/or behavior of a co-occurring dangerous species, thereby protecting itself from the attack of predators that have learned to avoid the dangerous species (Bates, 1861). This type of mimicry is maintained only when the relative frequency of the dangerous species is higher than that of the mimic, thus the predator maintains a conditioned avoidance to the mimic (Duncan and Sheppard, 1965). This argument also brings forward the effect of tolerance as another factor affecting fear response. In a comprehensive review, Blumstein (2016) stablished a clear difference between habituation and tolerance. Habituation is a process that acts at the scale of the individual, with each animal modifying their response to different stimulus based on their associated consequences across repeated exposures (Rankin et al., 2009; Blumstein, 2016). Tolerance, also involving a reduced response to a stimulus, and can emerge through habituation-like processes but also through other ecological pressures (e.g., competition; Owens, 1977; Blumstein, 2016). Moreover, tolerance through habituation can be transferable to different stimulus—e.g., squirrels inhabiting urban areas reduced their anti-predator response to foxes (Mccleery, 2009).
In a pest management context, the widespread deployment of predator cues (i.e., mimics) in the environment has the potential to overcome the relative frequency of the real predator, thus fear conditioning is lost (Schulte, 2016). Moreover, we would expect pest rodent populations inhabiting urban areas, would be more tolerant to human disturbances, and this tolerance may translate to a reduction in their antipredator response (Mccleery, 2009; Blumstein, 2016). A way of overcoming this dilemma is to maintain conditioning by aversive reinforcement (Kloppers et al., 2005; Leigh and Chamberlain, 2008; Cromsigt et al., 2013). These aversive reinforcements can be administered repeatedly (Huang et al., 1992; Dunsmoor et al., 2007) or be of high intensity (Abrams, 1994; Siegmund and Wotjak, 2007). However, this can be logistically difficult and undesirable (Baruch-Mordo et al., 2011). The implementation of repeated exposures of an intense aversive stimulus can be expensive, has negative animal welfare implications, or be lethal to the target and non-target species (Schulte, 2016). The question then becomes, what aversive reinforcement is required to maintain fear conditioning? One possible solution is to use predator cues that not only incite fear, but can also cause long-term anxiety (Schakner and Blumstein, 2013). This type of response to repeated stimulus is called sensitization and has been reported in seals (Götz and Janik, 2011, 2015), ungulates (Cox et al., 2012), and marsupials (Parsons and Blumstein, 2010). However, to date there is no evidence that rodents would show sensitization to predator cues.
In a comprehensive review of the role of predator odor in predator-prey interactions, Parsons et al. (2017) suggests that the attraction of prey to some predator smells may be due prey species gathering information either on the identity or temporal characteristics of the scent (Parsons et al., 2017). When an animal approaches the scent of a predator—i.e., predator inspection—it does so in order to obtain information about the actual risk of predation (Fishman, 1999). The animal may gain certain benefits from doing so, namely (i) acquiring information about the nature of the potential threat; (ii) informing conspecifics of the potential threat; (iii) deterring predator attack; and (iv) possibly even advertising one's quality to mates (Dugatkin and Godin, 1992).
Parsons et al. (2017) argues that this phenomenon can confound interpretation of empirical studies testing the repellent potential of predator scents and, as exemplar, describe how hairy-nosed wombats (Lasiorhinus latifrons), when presented with dingo (Canis dingo) scents, remained within 200 m of the stimulus (Sparrow et al., 2016). However, while still present in the area, wombats stopped their normal digging behavior, thus there was a significant reduction of their impact to human activity (Sparrow et al., 2016).
With the exception of pheromones (i.e., single species communication molecules), most scents are “dose-dependent” (Glimcher, 2010; Vasudevan and Vyas, 2013). Higher concentrations of a specific predator scent may convey higher or lower predation risk (Schmeisser et al., 2013). However, composition is also important. Scherer and Smee (2016), suggested that most prey species are sensitive to their predators' diet. For example, dwarf hamsters (Phodopus campelli) show a stronger anti-predator response when predators fed upon conspecifics (Apfelbach et al., 2015).
Another aspect of information gathering in predator cue inspection is the temporal component. Predator scent is normally complex, and composed of a myriad of different molecules, with different characteristics (Parsons et al., 2017). Each component within this complex mixture react to environmental conditions (e.g., bacterial decomposition, UV light) at different rates and in different manners (Rasmussen, 1988; Muller-Schwarze, 2006), ultimately modifying the chemical profile of the cue over time. Even handling and storage of the chemical cues can alter the way animals perceive them (Hoffmann et al., 2009; Hegab et al., 2014). It is therefore possible that changes in chemical structure of the signal may also change its information over time (Parsons and Blumstein, 2010). Older cues may convey that the predator is not there, and left a long time ago, while fresher cues can convey immediate danger (Hurst and Beynon, 2004; Parsons et al., 2017). However, there is some evidence that these changes may not affect rat responses (Rattus spp.) (Bytheway et al., 2013). Alternatively, animals may approach older odor cues since obtaining information from degraded scent may be more difficult, since only the non-volatile components remain (Parsons et al., 2017).
This raises the question; how can predator cues be applied in a rodent management context? The use of predator scent as a rodent management tool requires a better understanding on how these types of cues work. Just as adverse reinforcement is used to prevent habituation, the repeated application of odor cues to maintain their freshness can be logistically expensive and difficult. Current evidence suggest that wild rodents are not affected by aging scent (Bytheway et al., 2013), however, it is important to note that this is based on 1-day old scent. A better understanding is required when, along the aging time-line, anti-predator response disappears. This can help determine the rate at which the scent needs to be re-applied. Note, this is an issue specific to the use of chemical cues. Visual and auditory stimuli (or their pairing with chemical cues), might prove to be more effective.
Pest rodent species adaptability is what has placed them in constant conflict with humans. For humans, rodent populations' cost money, damage buildings, eat crops and transmit diseases. In contrast, rodents are “fighting” for their lives. Thus, it is not surprising that this constant struggle for survival pushes mice and rats to risk safety for food, and has made them very capable of assessing risks across very fine scales.
To use anti-predator responses as a rodent management tool, we need to follow a holistic approach. First, we need to understand that these animal's motivations are strong, thus our strategy needs to be stronger. It is essential to reinforce our approaches. Using a combination of predator and conspecific cues (e.g., predator odor, paired with predator models, and playbacks of both the predator and conspecifics alarm calls) to simulate high predation risk, but also maintaining the actual risks as high (e.g., repeated simultaneous aversive stimuli), could prevent most of the issues discussed in this review. For example, in agricultural systems, a sentinel system can be applied, where a crop area can be heavily guarded with simulated predators, while another is not, however treated with rodenticide. This can increase rodenticide intake, while reducing widespread use.
However, these are not easy tasks, and economic, logistical, and ethical costs need to be addressed. Yet, some steps in the right direction have been made in other systems. For example, Cromsigt et al. (2013) proposed the use of more intense methods of hunting ungulates (e.g., using dogs, targeting females with calves) in order to induce fear and deter these animals from areas of interest. Regardless of the polemic animal welfare implications, this proposal aims at increasing the intensity of the perceived risk (Cromsigt et al., 2013). Suraci et al. (2017a) have developed a motion triggered systems to playback predator sounds only when an animal approaches the devices, the cue is only used at proximity, intensifying the fear response. The effectiveness of this device has been demonstrated with pumas (Puma concolor) (Smith et al., 2017), and raccoons (Procyon lotor) (Suraci et al., 2016). One point of caution is the long term consequences of simulated high predation risk deployment are not fully understood, and some have raised doubts about how high this perceived risk should be (Duffield et al., 2017; Haapakoski et al., 2018). Sustained perception of very high risks of predation can drive a cycle of dynamic terminal investment (Duffield et al., 2017), that can have the desired short term deterrent effects, but produce long term population increases.
There are also synergies that can be achieved, if we consider the biological nature of the fear response. For example, the modification of antipredator behavior by T. gondii infections could assist with the reduction on the prevalence of T. gondii, at the community level, as a tool in conservation programs (Dubey and Crutchley, 2008; Fancourt and Jackson, 2014; Fancourt et al., 2014).
The theoretical framework of using fear as a way of managing pest rodent populations is sound, but it is not in any way simple. Pest rodents, either native or introduced, are embedded within a dynamic ecological system. If anti-predator responses are to be used as a pest management tool, it is essential to recognize that rodent's anti-predator responses are non-binary and rely on complex contextual cues.
MB-P conceived and wrote the initial manuscript. KS assisted with writing of particular sections of the manuscript. RK, JL, MC, and IM offered editorial advice and help structure the manuscript. All authors discussed the ideas presented and contributed to the final manuscript.
This manuscript was written under the funding of the Australian Research Council Discovery Project Grant Project ID DP160105003.
The authors declare that the research was conducted in the absence of any commercial or financial relationships that could be construed as a potential conflict of interest.
We thank Jordyn Stuart and Samuel Banister for their insights in the chemistry of predator cues, Cilla Zhou and Neda Assareh for assistance in understanding laboratory fear conditioning and learning, and Dave Allsop for inspiring sections of the project.
Abel E. L. (1991). Alarm substance emitted by rats in the forced-swim test is a low volatile pheromone. Physiol. Behav. 50, 723–727.
Abramsky Z., Rosenzweig M. L., and Subach A. (2002). The cost of apprehensive foraging. Ecology 83, 1330–1340. doi: 10.2307/3071947
Abramsky Z., Strauss E. G., Subach A., Riechman A., and Kotler B. P. (1996). The effect of barn owls (Tyto alba) on the activity and microhabitat selection of Gerbillus allenby; and G. pyramidum. Oecologia 105, 313–319.
Abu Baker M. A., and Brown J. S. (2012). Patch use behaviour of Elephantulus myurus and Micaelamys namaquensis: the role of diet, foraging substrates and escape substrates. Afr. J. Ecol. 50, 167–175. doi: 10.1111/j.1365-2028.2011.01309.x
Agrawal A. A., and Fishbein M. (2006). Plant defense syndromes. Ecology 87, S132–S149. doi: 10.1890/0012-9658(2006)87[132:PDS]2.0.CO;2
Albert C. A., Wilson L. K., Mineau P., Trudeau S., and Elliott J. E. (2010). Anticoagulant rodenticides in three owl species from western Canada, 1988–2003. Arch. Environ. Contam. Toxicol. 58, 451–459. doi: 10.1007/s00244-009-9402-z
Allmann-lselin I. (2000). “4. Husbandry,” in The Laboratory rat Handbook of Experimental Animals, eds Krinke, G. J., Bullock, G. R., and Bunton, T. (London; San Diego, CA: Academic Press, Inc.), 45–55. doi: 10.1016/B978-0-12-426400-7.X5037-7
Almeida A., Corrigan R. M., and Sarno R. (2013). The economic impact of commensal rodents on small businesses in Manhattan's Chinatown: trends and possible causes. Suburb. Sustain. 1, 1–15. doi: 10.5038/2164-0866.1.1.2
Altendorf K. B., Laundr,é J. W., López González C. A., and Brown J. S. (2001). Assessing effects of predation risk on foraging behavior of mule deer. J. Mammal. 82, 430–439. doi: 10.1644/1545-1542(2001)082<0430:aeopro>2.0.co;2
Anson J. R., and Dickman C. R. (2013). Behavioral responses of native prey to disparate predators: naiveté and predator recognition. Oecologia 171, 367–77. doi: 10.1007/s00442-012-2424-7
Apfelbach R., Blanchard D. C., Blanchard R. J., Hayes R. A., and McGregor I. S. (2005). The effects of predator odors in mammalian prey species: a review of field and laboratory studies. Neurosci. Biobehav. Rev. 29, 1123–1144. doi: 10.1016/j.neubiorev.2005.05.005
Apfelbach R., Parsons M. H., Soini H. A., and Novotny M. V. (2015). Are single odorous components of a predator sufficient to elicit defensive behaviors in prey species? Front. Neurosci. 9:263. doi: 10.3389/fnins.2015.00263
Atkins A., Redpath S. M., Little R. M., and Amar A. (2017). Experimentally manipulating the landscape of fear to manage problem animals. J. Wildl. Manage. 81, 610–616. doi: 10.1002/jwmg.21227
Atkinson I. A. E. (1985). “The spread of commensal species of Rattus to oceanic islands and their effects on island avifaunas,” in Conservation of Island Birds: Case Studies for the Management of Threatened Island Species, ed Moors, P. (Cambridge: ICBP Technical Publication), 35–81.
Atkinson U. A. E. (1973). Spread of the ship rat (Rattus r. rattus L.) III New Zealand. J. R. Soc. New Zeal. 3, 457–472.
Ayral F., Artois J., Zilber A.-L., Widen F., Pounder K. C., Aubert D., et al. (2015). The relationship between socioeconomic indices and potentially zoonotic pathogens carried by wild Norway rats: a survey in Rhône, France (2010–2012). Epidemiol. Infect. 143, 586–599. doi: 10.1017/S0950268814001137
Banks P. B. (1998). Responses of Australian bush rats, Rattus fuscipes, to the odor of introduced Vulpes vulpes. J. Mammal. 79, 1260–1264.
Barnett S. A. (1958). Experiments on “neophobia” in wild and laboratory rats. Br. J. Psychol. 49, 195–201.
Barnett S. A. (2001). The Story of Rats: Their Impact on us, and Our Impact on Them. Crows Nest, NSW: Allen & Unwin.
Barnett S. A., and Cowan P. E. (1976). Activity, exploration, curiosity and fear: an ethological study. Interdiscip. Sci. Rev. 1, 43–62.
Bartecki U., and Heymann E. W. (1987). Field observation of snake-mobbing in a group of saddle-back tamarins, Saguinus fuscicollis nigrifrons. Folia Primatol. 48, 199–202.
Baruch-Mordo S., Breck S. W., Wilson K. R., and Broderick J. (2011). The Carrot or the stick? Evaluation of education and enforcement as management tools for human-wildlife conflicts. PLoS ONE 6:e15681. doi: 10.1371/journal.pone.0015681
Bates H. W. (1861). XXXII. Contributions to an insect fauna of the Amazon valley. Lepidoptera: Heliconidæ. Trans. Linn. Soc. London 23, 495–566.
Bedoya-Pérez M. A., Carthey A. J. R., Mella V. S. A., McArthur C., and Banks P. B. (2013). A practical guide to avoid giving up on giving-up densities. Behav. Ecol. Sociobiol. 67, 1–13. doi: 10.1007/s00265-013-1609-3
Bennett G. W., Owens J. M., and Corrigan R. M. (2003). Truman's Scientific Guide to Pest Management Operations. 6th Edn. Duluth, MN: Purdue University.
Berardi N., and Maffei L. (1999). From visual experience to visual function: Roles of neurotrophins. J. Neurobiol. 41, 119–126.
Berdoy M., and Macdonald D. W. (1991). Factors affecting feeding in wild rats. Acta Oecol. 12, 261–279.
Berdoy M., Webster J. P., and Macdonald D. W. (1995a). Parasite-altered behaviour: is the effect of Toxoplasma gondii on Rattus norvegicus specific? Parasitology 111(Pt 4), 403–409. doi: 10.1017/S0031182000065902
Berdoy M., Webster J. P., and Macdonald D. W. (1995b). The manipulation of rat behaviour by Toxoplasma gondii. Mammalia 59, 605–614. doi: 10.1515/mamm.1995.59.4.605
Berdoy M., Webster J. P., and Macdonald D. W. (2000). Fatal attraction in rats infected with Toxoplasma gondii. Proc. R. Soc. London. Ser. B Biol. Sci. 267, 1591–1594. doi: 10.1098/rspb.2000.1182
Berejikian B. A., Tezak E. P., and LaRae A. L. (2003). Innate and enhanced predator recognition in hatchery-reared chinook salmon. Environ. Biol. Fishes 67, 241–251. doi: 10.1023/A:1025887015436
Berger J., Swenson J. E., and Persson I.-L. (2001). Recolonizing carnivores and naïve prey: conservation lessons from Pleistocene extinctions. Science 291, 1036–1039. doi: 10.1126/science.1056466
Berger-Tal O., and Kotler B. P. (2010). State of emergency: Behavior of gerbils is affected by the hunger state of their predators. Ecology 91, 593–600. doi: 10.1890/09-0112.1
Berger-Tal O., Mukherjee S., Kotler B. P., and Brown J. S. (2010). Complex state-dependent games between owls and gerbils. Ecol. Lett. 13, 302–310. doi: 10.1111/j.1461-0248.2010.01447.x
Beverley J. K. A. (1959). Congenital transmission of Toxoplasmosis through successive generations of mice. Nature 183, 1348–1349. doi: 10.1038/1831348a0
Blanchard D. C., Blanchard R. J., and Rodgers R. J. (1991). “Risk assessment and animal models of anxiety,” in Animal Models in Psychopharmacology, eds Olivier, B., Mos, J., and Slangen, J. L. (Basel: Birkhäuser Basel), 117–134.
Blanchard R. J., Blanchard D. C., Agullana R., and Weiss S. M. (1991). Twenty-two kHz alarm cries to presentation of a predator, by laboratory rats living in visible burrow systems. Physiol. Behav. 50, 967–972.
Blanchard R. J., Nikulina J. N., Sakai R. R., McKittrick C., McEwen B., and Blanchard D. C. (1998). Behavioral and endocrine change following chronic predatory stress. Physiol. Behav. 63, 561–569.
Blanchard R. J., Yudko E. B., Rodgers R. J., and Blanchard D. C. (1993). Defense system psychopharmacology: an ethological approach to the pharmacology of fear and anxiety. Behav. Brain Res. 58, 155–165.
Bleicher S. S. (2017). The landscape of fear conceptual framework: definition and review of current applications and misuses. PeerJ. 5:e3772. doi: 10.7717/peerj.3772
Blumstein D. T. (2016). Habituation and sensitization: new thoughts about old ideas. Anim. Behav. 120, 255–262. doi: 10.1016/j.anbehav.2016.05.012
Blumstein D. T., Daniel J. C., Griffin A. S., and Evans C. S. (2000). Insular tammar wallabies (Macropus eugenii) respond to visual but not acoustic cues from predators. Behav. Ecol. 11, 528–535. doi: 10.1093/beheco/11.5.528
Bohls P., and Koehnle T. J. (2017). Responses of eastern gray squirrels (Sciurus carolinensis) to predator calls and their modulation by coat color. Am. Midl. Nat. 178, 226–236. doi: 10.1674/0003-0031-178.2.226
Bolles R. C. (1970). Species-specific defense reactions and avoidance learning. Psychol. Rev. 77, 32–48.
Bomford M., and O'Brien P. H. (1990). Sonic deterrents in animal damage control: a review of device tests and effectiveness. Wildl. Soc. Bull. 18, 411–422.
Bonnington C., Gaston K. J., and Evans K. L. (2013). Fearing the feline: domestic cats reduce avian fecundity through trait-mediated indirect effects that increase nest predation by other species. J. Appl. Ecol. 50, 15–24. doi: 10.1111/1365-2664.12025
Bowen M. T., Keats K., Kendig M. D., Cakic V., Callaghan P. D., and McGregor I. S. (2012). Aggregation in quads but not pairs of rats exposed to cat odor or bright light. Behav. Processes 90, 331–336. doi: 10.1016/j.beproc.2012.03.014
Bowen M. T., Kevin R. C., May M. D., Staples L. G., Hunt G. E., and McGregor I. S. (2013). Defensive aggregation (huddling) in Rattus norvegicus toward predator odor: individual differences, social buffering effects and neural correlates. PLoS ONE 8:e68483. doi: 10.1371/journal.pone.0068483
Bramley G. N., and Waas J. R. (2001). Laboratory and field evaluation of predator odors as repellents for kiore (Rattus exulans) and ship rats (R. rattus). J. Chem. Ecol. 27, 1029–1047. doi: 10.1023/a:1010399322861
Brechbühl J., Klaey M., and Broillet M.-C. (2008). Grueneberg ganglion cells mediate alarm pheromone detection in mice. Science 321, 1092–1095. doi: 10.1126/science.1160770
Brechbühl J., Moine F., Klaey M., Nenniger-Tosato M., Hurni N., Sporkert F., et al. (2013). Mouse alarm pheromone shares structural similarity with predator scents. Proc. Natl. Acad. Sci. U.S.A. 110, 4762–4767. doi: 10.1073/pnas.1214249110.
Brown C., and Laland K. (2005). Social learning and life skills training for hatchery reared fish. J. Fish Biol. 59, 471–493. doi: 10.1111/j.1095-8649.2001.tb02354.x
Brown J. S., and Alkon P. U. (1990). Testing values of crested porcupine habitats by experimental food patches. Oecologia 83, 512–518.
Brown J. S., Kotler B. P., Smith R. J., and Wirtz W. O. II. (1988). The effects of owl predation on the foraging behavior of heteromyid rodents. Oecologia 76, 408–415.
Brown J. S., Laundré J. W., and Gurung M. (1999). The ecology of fear: Optimal foraging, game theory, and trophic interactions. J. Mammal. 80, 385–399.
Brown J. S., and Morgan R. A. (1995). Effects of foraging behavior and spatial scale on diet selectivity: a test with fox squirrels. Oikos 74, 122–136.
Brudzynski S. M., and Chiu E. M. C. (1995). Behavioural responses of laboratory rats to playback of 22 kHz ultrasonic calls. Physiol. Behav. 57, 1039–1044.
Brunton C. F. A., Macdonald D. W., and Buckle A. P. (1993). Behavioural resistance towards poison baits in brown rats, Rattus norvegicus. Appl. Anim. Behav. Sci. 38, 159–174.
Buckle A. P. (2013). Anticoagulant resistance in the United Kingdom and a new guideline for the management of resistant infestations of Norway rats (Rattus norvegicus Berk.). Pest Manag. Sci. 69, 334–341. doi: 10.1002/ps.3309
Buckle A. P., and Smith R. H. (2015). Rodent Pests and Their Control. 2nd Edn. Oxfordshire; Boston, MA: CABI.
Burwash M. D., Tobin M. E., Woolhouse A. D., and Sullivan T. P. (1998). Field testing synthetic predator odors for roof rats (Rattus rattus) in Hawaiian macadamia nut orchards. J. Chem. Ecol. 24, 603–630.
Bytheway J. P., Carthey A. J. R., and Banks P. B. (2013). Risk vs. reward: how predators and prey respond to aging olfactory cues. Behav. Ecol. Sociobiol. 67, 715–725. doi: 10.1007/s00265-013-1494-9
Campeau S., and Davis M. (1995). Involvement of subcortical and cortical afferents to the lateral nucleus of the amygdala in fear conditioning measured with fear-potentiated startle in rats trained concurrently with auditory and visual conditioned stimuli. J. Neurosci. 15(3 Pt 2), 2312–2327.
Canteras N., Pavesi E., and Carobrez A. (2015). Olfactory instruction for fear: neural system analysis. Front. Neurosci. 9:276. doi: 10.3389/fnins.2015.00276
Capizzi D., Bertolino S., and Mortelliti A. (2014). Rating the rat: global patterns and research priorities in impacts and management of rodent pests. Mamm. Rev. 44, 148–168. doi: 10.1111/mam.12019
Caro T. (2005). Antipredator Defenses in Birds and Mammals. Chicago, IL: University of Chicago Press.
Carthey A. J. R., and Banks P. B. (2016). Naiveté is not forever: responses of a vulnerable native rodent to its long term alien predators. Oikos 125, 918–926. doi: 10.1111/oik.02723.
Chamaillé-Jammes S., Malcuit H., Le Saout S., and Martin J.-L. (2014). Innate threat-sensitive foraging: black-tailed deer remain more fearful of wolf than of the less dangerous black bear even after 100 years of wolf absence. Oecologia 174, 1151–1158. doi: 10.1007/s00442-013-2843-0
Chamero P., Leinders-Zufall T., and Zufall F. (2012). From genes to social communication: molecular sensing by the vomeronasal organ. Trends Neurosci. 35, 597–606. doi: 10.1016/j.tins.2012.04.011
Charnov E. L. (1979). Optimal Foraging: Some Thoretical Explorations. Salt Lake City, UT: Center of Quantitative Science, University of Washington, Seatle. Institute of Animal Resource Ecology, University of British Columbia Vancouver.
Childress M. J., and Lung M. A. (2003). Predation risk, gender and the group size effect: does elk vigilance depend upon the behaviour of conspecifics? Anim. Behav. 66, 389–398. doi: 10.1006/anbe.2003.2217
Chitty D. (1954). Control of Rats and Mice, eds Chitty, D. and Southern, H. N.. Oxford; Chicago, IL: Oxford: Clarendon Press.
Chivers D. P., Brown G. E., and Smith R. J. F. (1995). Acquired recognition of chemical stimuli from pike, Esox lucius, by Brook sticklebacks, Culaea inconstans (Osteichthyes, Gasterosteidae). Ethology 99, 234–242.
Choleris E., and Kavaliers M. (1999). Social learning in animals: sex differences and neurobiological analysis. Pharmacol. Biochem. Behav. 64, 767–776.
Clinchy M., Sheriff M. J., and Zanette L. Y. (2013). Predator-induced stress and the ecology of fear. Funct. Ecol. 27, 56–65. doi: 10.1111/1365-2435.12007
Clutton-Brock J. (1999). A Natural History of Domesticated Mammals. 2nd Edn. Cambridge: Cambridge University Press.
Coeurdassier M., Fritsch C., Jacquot M., van den Brink N. W., and Giraudoux P. (2018). “Spatial dimensions of the risks of rodenticide use to non-target small mammals and applications in spatially explicit risk modeling,” in Anticoagulant Rodenticides and Wildlife, eds van den Brink, N. W., Elliott, J. E., Shore, R. F., and Rattner, B. A. (Cham: Springer International Publishing), 195–227.
Cook M., and Mineka S. (1990). Selective associations in the observational conditioning of fear in rhesus monkeys. J. Exp. Psychol. Anim. Behav. Process. 16, 372. doi: 10.1037/0097-7403.16.4.372
Cook M., Mineka S., Wolkenstein B., and Laitsch K. (1985). Observational conditioning of snake fear in unrelated rhesus monkeys. J. Abnorm. Psychol. 94, 591–610. doi: 10.1037/0021-843X.94.4.591
Corcoran A. J., and Conner W. E. (2012). Sonar jamming in the field: effectiveness and behavior of a unique prey defense. J. Exp. Biol. 215, 4278–4287. doi: 10.1242/jeb.076943
Coss R. G. (1999). “Effects of relaxed natural selection on the evolution,” in Geographic Variation in Behavior: Perspectives on Evolutionary Mechanisms, eds Foster, S. A. and Endler, J. A. (New York, NY; Oxford: Oxford University Press on Demand), 180–208.
Coss R. G., and Ramakrishnan U. (2000). Perceptual aspects of leopard recognition by wild bonnet macaques (Macaca radiata). Behaviour 137, 315–335. doi: 10.1163/156853900502105
Cox T. E., Murray P. J., Hall G. P., and Li X. (2012). Manipulating resource use by goats with predator fecal odors. Wildl. Soc. Bull. 36, 802–806. doi: 10.1002/wsb.215
Crawford M., and Masterson F. A. (1982). Species-specific defense reactions and avoidance learning. Pavlov. J. Biol. Sci. Off. J. Pavlov. 17, 204–214.
Creel S. (2018). The control of risk hypothesis: reactive vs. proactive antipredator responses and stress-mediated vs. food-mediated costs of response. Ecol. Lett. 21, 947–956. doi: 10.1111/ele.12975
Creel S., Christianson D., Liley S., and Winnie J. A. J. (2007). Predation risk affects reproductive physiology and demography of elk. Science 315:960. doi: 10.1126/science.1135918
Cresswell W., Lind J., Kaby U., Quinn J. L., and Jakobsson S. (2003). Does an opportunistic predator preferentially attack nonvigilant prey? Anim. Behav. 66, 643–648. doi: 10.1006/anbe.2003.2233
Cromsigt J. P. G. M., Kuijper D. P. J., Adam M., Beschta R. L., Churski M., Eycott A., et al. (2013). Hunting for fear: innovating management of human-wildlife conflicts. J. Appl. Ecol. 50, 544–549. doi: 10.1111/1365-2664.12076
Curio E. (1988). “Cultural transmission of enemy recognition,” in Social Learning: Psychological and Biological Perspectives Comparative Cognition and Neuroscience Series, eds Zentall, T. R. and Galef, B. G. (Lawrence Erlbaum Associates, Inc.), 75–97. Available online at: https://books.google.com.au/books?id=mqFcAgAAQBAJ
Curio E. (1993). “Proximate and developmental aspects of antipredator behavior,” in Advances in the Study of Behavior, eds Slater, P. J. B., Rosenblatt, J. S., Snowdon, C. T., and Milinski, M. (San Diego, CA: Academic Press, Inc.), 135–238.
Curio E., Ernst U., and Vieth W. (1978). Cultural transmission of enemy recognition: one function of mobbing. Science 202, 899–901.
Dalesman S., Rundle S. D., and Cotton P. A. (2007). Predator regime influences innate anti-predator behaviour in the freshwater gastropod Lymnaea stagnalis. Freshw. Biol. 52, 2134–2140. doi: 10.1111/j.1365-2427.2007.01843.x
Dass S. A. H., Vasudevan A., Dutta D., Soh L. J. T., Sapolsky R. M., and Vyas A. (2011). Protozoan parasite Toxoplasma gondii manipulates mate choice in rats by enhancing attractiveness of males. PLoS ONE 6:e27229. doi: 10.1371/journal.pone.0027229
Davidson D. L., and Morris D. W. (2001). Density-dependent foraging effort of deer mice (Peromyscus maniculatus). Funct. Ecol. 15, 575–583. doi: 10.1046/j.0269-8463.2001.00569.x
Davis M. (1970). Effects of interstimulus interval length and variability on startle-response habituation in the rat. J. Comp. Physiol. Psychol. 72, 177–192.
Dewan A., Pacifico R., Zhan R., Rinberg D., and Bozza T. (2013). Non-redundant coding of aversive odours in the main olfactory pathway. Nature 497, 486–489. doi: 10.1038/nature12114
Dickman C. R. (1992). Predation and habitat shift in the House mouse, Mus domesticus. Ecology 73, 313–322.
Dielenberg R. A., and McGregor I. S. (2001). Defensive behavior in rats towards predatory odors: a review. Neurosci. Biobehav. Rev. 25, 597–609. doi: 10.1016/S0149-7634(01)00044-6
Domjan M. (1975). Poison-induced neophobia in rats: role of stimulus generalization of conditioned taste aversions. Anim. Learn. Behav. 3, 205–211.
Dowding C. V., Shore R. F., Worgan A., Baker P. J., and Harris S. (2010). Accumulation of anticoagulant rodenticides in a non-target insectivore, the European hedgehog (Erinaceus europaeus). Environ. Pollut. 158, 161–166. doi: 10.1016/j.envpol.2009.07.017
Dubey J. P., and Crutchley C. (2008). Toxoplasmosis in Wallabies (Macropus rufogriseus and Macropus eugenii): Blindness, Treatment with Atovaquone, and Isolation of Toxoplasma gondii. J. Parasitol. 94, 929–933. doi: 10.1645/GE-1448.1
Dubey J. P., and Jones J. L. (2008). Toxoplasma gondii infection in humans and animals in the United States. Int. J. Parasitol. 38, 1257–1278. doi: 10.1016/j.ijpara.2008.03.007
Dudeck B. P., Clinchy M., Allen M. C., and Zanette L. Y. (2018). Fear affects parental care, which predicts juvenile survival and exacerbates the total cost of fear on demography. Ecology 99, 127–135. doi: 10.1002/ecy.2050
Duffield K. R., Bowers E. K., Sakaluk S. K., and Sadd B. M. (2017). A dynamic threshold model for terminal investment. Behav. Ecol. Sociobiol. 71:185. doi: 10.1007/s00265-017-2416-z
Dugatkin L. A., and Godin J.-G. J. (1992). Prey approaching predators: a cost-benefit perspective. Ann. Zool. Fennici 29, 233–252.
Duncan C. J., and Sheppard P. M. (1965). Sensory discrimination and its role in the evolution of Batesian mimicry. Behaviour 24, 269–282.
Dunsmoor J. E., Bandettini P. A., and Knight D. C. (2007). Impact of continuous versus intermittent CS-UCS pairing on human brain activation during Pavlovian fear conditioning. Behav. Neurosci. 121, 635–642. doi: 10.1037/0735-7044.121.4.635
Eason C. T., Murphy E. C., Wright G. R. G., and Spurr E. B. (2002). Assessment of risks of brodifacoum to non-target birds and mammals in New Zealand. Ecotoxicology 11, 35–48. doi: 10.1023/A:1013793029831
Eisenberg J. F., and Kleiman D. G. (1972). Olfactory communication in mammals. Annu. Rev. Ecol. Syst. 3, 1–32.
Elliott J. E., Hindmarch S., Albert C. A., Emery J., Mineau P., and Maisonneuve F. (2014). Exposure pathways of anticoagulant rodenticides to nontarget wildlife. Environ. Monit. Assess. 186, 895–906. doi: 10.1007/s10661-013-3422-x
Embar K., Kotler B. P., and Mukherjee S. (2011). Risk management in optimal foragers: the effect of sightlines and predator type on patch use, time allocation, and vigilance in gerbils. Oikos 120, 1657–1666. doi: 10.1111/j.1600-0706.2011.19278.x
Emile N., and Barros M. (2009). Recognition of a 3D snake model and its 2D photographic image by captive black tufted-ear marmosets (Callithrix penicillata). Anim. Cogn. 12, 725–732. doi: 10.1007/s10071-009-0234-z
Fancourt B. A., and Jackson R. B. (2014). Regional seroprevalence of Toxoplasma gondii antibodies in feral and stray cats (Felis catus) from Tasmania. Aust. J. Zool. 62, 272–283. doi: 10.1071/ZO14015
Fancourt B. A., Nicol S. C., Hawkins C. E., Jones M. E., and Johnson C. N. (2014). Beyond the disease: Is Toxoplasma gondii infection causing population declines in the eastern quoll (Dasyurus viverrinus)? Int. J. Parasitol. Parasites Wildl. 3, 102–112. doi: 10.1016/j.ijppaw.2014.05.001
Fanson B. G., Fanson K. V., and Brown J. S. (2010). Ecological factors affecting the foraging behaviour of Xerus rutilus. African Zool. 45, 265–272. doi: 10.3377/004.045.0205.
Ferrero D. M., Lemon J. K., Fluegge D., Pashkovski S. L., Korzan W. J., Datta S. R., et al. (2011). Detection and avoidance of a carnivore odor by prey. Proc. Natl. Acad. Sci. U.S.A. 108, 11235–11240. doi: 10.1073/pnas.1103317108
Fishman M. A. (1999). Predator inspection: closer approach as a way to improve assessment of potential threats. J. Theor. Biol. 196, 225–235.
FitzGibbon C. D., and Fanshawe J. H. (1988). Stotting in Thomson's gazelles: an honest signal of condition. Behav. Ecol. Sociobiol. 23, 69–74.
Fortin D., Boyce M. S., Merrill E. H., and Fryxell J. M. (2004). Foraging costs of vigilance in large mammalian herbivores. Oikos 107, 172–180. doi: 10.1111/j.0030-1299.2004.12976.x
Fraenkel G. S. (1959). The raison d'etre of secondary plant substances: these odd chemicals arose as a means of protecting plants from insects and now guide insects to food. Science129, 1466–1470.
Fraser D. F., and Huntingford F. A. (1986). Feeding and avoiding predation hazard: the behavioral response of the prey. Ethology 73, 56–68.
Freeland W. J. (1991). “Plant secondary metabolites: biochemical coevolution with herbivores,” in Plant Defenses Against Mammalian Herbivory, eds Palo, R. T. and Robbins, C. T. (Boca Raton, FL: CRC Press), 61–81.
Fuelling O., and Halle S. (2004). Breeding suppression in free-ranging grey-sided voles under the influence of predator odour. Oecologia 138, 151–159. doi: 10.1007/s00442-003-1417-y
Gabriel M. W., Woods L. W., Poppenga R., Sweitzer R. A., Thompson C., Matthews S. M., et al. (2012). Anticoagulant rodenticides on our public and community lands: spatial distribution of exposure and poisoning of a rare forest carnivore. PLoS ONE 7:e40163. doi: 10.1371/journal.pone.0040163
Garb J., Kotler B. P., and Brown J. S. (2000). Foraging and community consequences of seed size for coexisting Negev desert granivores. Oikos 88, 291–300. doi: 10.1034/j.1600-0706.2000.880207.x
Garvey P. M., Glen A. S., Clout M. N., Wyse S. V., Nichols M., and Pech R. P. (2017). Exploiting interspecific olfactory communication to monitor predators. Ecol. Appl. 27, 389–402. doi: 10.1002/eap.1483
Glimcher P. W. (2010). Foundations of Neuroeconomic Analysis. New York, NY: Oxford University Press. doi: 10.1093/acprof:oso/9780199744251.001.0001
Gonzalez L. E., Rojnik B., Urrea F., Urdaneta H., Petrosino P., Colasante C., et al. (2007). Toxoplasma gondii infection lower anxiety as measured in the plus-maze and social interaction tests in rats: a behavioral analysis. Behav. Brain Res. 177, 70–79.
Göth A. (2001). Innate predator-recognition in Australian brush-turkey (Alectura lathami, Megapodiidae) hatchlings. Behaviour 138, 117–136. doi: 10.1163/156853901750077826
Götz T., and Janik V. M. (2011). Repeated elicitation of the acoustic startle reflex leads to sensitisation in subsequent avoidance behaviour and induces fear conditioning. BMC Neurosci. 12:30. doi: 10.1186/1471-2202-12-30
Götz T., and Janik V. M. (2015). Target-specific acoustic predator deterrence in the marine environment. Anim. Conserv. 18, 102–111. doi: 10.1111/acv.12141
Gould L., and Sauther M. L. (2007). “Anti-predator strategies in a diurnal prosimian, the ring-tailed lemur (Lemur catta), at the Beza Mahafaly special reserve, Madagascar,” in Primate Anti-Predator Strategies, eds Gursky, S. L. and Nekaris, K. A. I. (Boston, MA: Springer), 275–288.
Griffin A. S., Blumstein D. T., and Evans C. S. (2001). Training captive-bred or translocated animals to avoid predators. Conserv. Biol. 14, 1317–1326. doi: 10.1046/j.1523-1739.2000.99326.x
Griffin A. S., and Evans C. S. (2003). Social learning of antipredator behaviour in a marsupial. Anim. Behav. 66, 485–492. doi: 10.1006/anbe.2003.2207
Haapakoski M., Hardenbol A. A., and Matson K. D. (2018). Exposure to chemical cues from predator-exposed conspecifics increases reproduction in a wild rodent. Sci. Rep. 8:17214. doi: 10.1038/s41598-018-35568-0
Hansen I., and Bakken M. (1999). Livestock-guarding dogs in Norway: Part I. Interactions. J. Range Manag. 52, 2–6.
Hanson M. T., and Coss R. G. (1997). Age differences in the response of California ground Squirrels (Spermophilus beecheyi) to avian and mammalian predators. J Comp Psychol. 111, 174–184. doi: 10.1037/0735-7036.111.2.174
Hari Dass S. A., and Vyas A. (2014). Toxoplasma gondii infection reduces predator aversion in rats through epigenetic modulation in the host medial amygdala. Mol. Ecol. 23, 6114–6122. doi: 10.1111/mec.12888
Harvell C. D., and Tollrian R. (1999). “Why inducible defenses,” in The Ecology and Evolution of Inducible Defenses (Princeton, NJ: Princeton University Press), 3–9.
Hedrich H. J., and Bullock G. R. (2004). The Laboratory Mouse. London; San Diego, CA: Academic Press, Inc.
Heffner H. E., and Heffner R. S. (2007). Hearing ranges of laboratory animals. J. Am. Assoc. Lab. Anim. Sci. 46, 20–22. Available online at: http://docserver.ingentaconnect.com/deliver/connect/aalas/15596109/v46n1/s3.pdf?expires=1555201600&id=0000&titleid=72010024&checksum=BC36FE70988307B511EB26D083F16400
Heffner H. E., and Heffner R. S. (2008). High-frequency hearing. Handb. Senses Audit. 55:60. doi: 10.1016/B978-012370880-9.00004-9
Hegab I. M., Shang G., Ye M., Jin Y., Wang A., Yin B., et al. (2014). Defensive responses of Brandt's voles (Lasiopodomys brandtii) to chronic predatory stress. Physiol. Behav. 126, 1–7. doi: 10.1016/j.physbeh.2013.12.001
Herman C. S., and Valone T. J. (2000). The effect of mammalian predator scent on the foraging behavior of Dipodomys merriami. Oikos 91, 139–145. doi: 10.1034/j.1600-0706.2000.910113.x
Hirsch S. M., and Bolles R. C. (1980). On the ability of prey to recognize predators. Z. Tierpsychol. 54, 71–84.
Hoffmann F., Musolf K., and Penn D. J. (2009). Freezing urine reduces its efficacy for eliciting ultrasonic vocalizations from male mice. Physiol. Behav. 96, 602–605. doi: 10.1016/j.physbeh.2008.12.014
House P. K., Vyas A., and Sapolsky R. (2011). Predator cat odors activate sexual arousal pathways in brains of Toxoplasma gondii infected rats. PLoS ONE 6:e23277. doi: 10.1371/journal.pone.0023277
Howald G. R., Mineau P., Elliott J. E., and Cheng K. M. (1999). Brodifacoum poisoning of avian scavengers during rat control on a seabird colony. Ecotoxicology 8, 431–447.
Huang I. N., Krukar J. D., and Miles S. P. (1992). Effects of reinforcement schedules on rats' choice behavior in extinction. J. Gen. Psychol. 119, 201–211.
Hughes J. J., and Ward D. (1993). Predation risk and distance to cover affect foraging behaviour in Namib desert gerbils. Anim. Behav. 46, 1243–1245. doi: 10.1006/anbe.1993.1320
Hurst J. L., and Beynon R. J. (2004). Scent wars: the chemobiology of competitive signalling in mice. Bioessays 26, 1288–1298. doi: 10.1002/bies.20147
Hutchison W. M., Aitken P. P., and Wells W. P. (1980). Chronic Toxoplasma infections and familiarity-novelty discrimination in the mouse. Ann. Trop. Med. Parasitol. 74, 145–150.
Hutchison W. M., Dunachie J. F., Siim J. C., and Work K. (1969). Life cycle of Toxoplasma gondii. Br. Med. J. 4, 806–806.
Inglis I. R. R., Shepherd D. S. S., Smith P., Haynes P. J. J., Bull D. S., Cowan D. P. D. P., et al. (1996). Foraging behaviour of wild rats (Rattus norvegicus) towards new foods and bait containers. Appl. Anim. Behav. Sci. 47, 175–190.
Iribarren C., and Kotler B. P. (2012). Patch use and vigilance behaviour by Nubian ibex: the role of the effectiveness of vigilance. Evol. Ecol. Res. 14, 223–234. Available online at: http://www.evolutionary-ecology.com/issues/v14/n02/hhar2705.pdf
Irving P. W., and Magurran A. E. (1997). Context-dependent fright reactions in captive european minnows: the importance of naturalness in laboratory experiments. Anim. Behav. 53, 1193–1201.
John A. (2014). Rodent outbreaks and rice pre-harvest losses in Southeast Asia. Food Secur. 6, 249–260. doi: 10.1007/s12571-014-0338-4
Kaukeinen D. E. (1994). “Rodent control in practice: householders, pest control operators and municipal authorities,” in Rodent Pests and Their Control, eds Buckle, A. P. and Smith, R. H. (Wallingford: CAB International), 249–271.
Kavaliers M., and Choleris E. (2001). Antipredator responses and defensive behavior: ecological and ethological approaches for the neurosciences. Neurosci. Biobehav. Rev. 25, 577–586. doi: 10.1016/S0149-7634(01)00042-2
Kavaliers M., Choleris E., and Colwell D. D. (2001a). Brief exposure to female odors “emboldens” male mice by reducing predator-induced behavioral and hormonal responses. Horm. Behav. 40, 497–509. doi: 10.1006/hbeh.2001.1714
Kavaliers M., Choleris E., and Colwell D. D. (2001b). Learning from others to cope with biting flies: social learning of fear-induced conditioned analgesia and active avoidance. Behav. Neurosci. 115, 661–674. doi: 10.1037/0735-7044.115.3.661
Kelley J. L., and Magurran A. E. (2003). Effects of relaxed predation pressure on visual predator recognition in the guppy. Behav. Ecol. Sociobiol. 54, 225–232. doi: 10.1007/s00265-003-0621-4
Kendig M. D., Bowen M. T., Kemp A. H., and McGregor I. S. (2011). Predatory threat induces huddling in adolescent rats and residual changes in early adulthood suggestive of increased resilience. Behav. Brain Res. 225, 405–414. doi: 10.1016/j.bbr.2011.07.058
Kerfoot W. C., and Sih A. (1987). Predation: Direct and Indirect Impacts on Aquatic Communities. Hanover, NH: University Press of New England.
Kikusui T., Takigami S., Takeuchi Y., and Mori Y. (2001). Alarm pheromone enhances stress-induced hyperthermia in rats. Physiol. Behav. 72, 45–50. doi: 10.1016/S0031-9384(00)00370-X
Kloppers E. L., St. Clair C. C., and Hurd T. E. (2005). Predator-resembling aversive conditioning for managing habituated wildlife. Ecol. Soc. 10:31. doi: 10.5751/ES-01293-100131
Koehler A. E., Marsh R. E., and Salmon T. P. (1990). “Frightening methods and devices/stimuli to prevent mammal damage-a review,” in Proceedings of the Fourteenth Vertebrate Pest Conference 1990 (Lincoln, OR).
Kotler B. P., Brown J. S., Bouskila A., Mukherjee S., and Goldberg T. (2004). Foraging games between gerbils and their predators: seasonal changes in schedules of activity and aprehension. Isr. J. Zool. 50, 256–271. doi: 10.1560/K8D7-8KCX-BLAW-Y2K5
Kotler B. P., Brown J. S., Dall S. R. X., Gresser S., Ganey D. T., and Bouskila A. (2002). Foraging games between gerbils and their predators: temporal dynamics of resource depletion and apprehension in gerbils. Evol. Ecol. Res. 4, 495–518. Available online at: http://www.evolutionary-ecology.com/issues/v04n04/ddar1310.pdf
Kotler B. P., Brown J. S., Mukherjee S., Berger-Tal O., and Bouskila A. (2010). Moonlight avoidance in gerbils reveals a sophisticated interplay among time allocation, vigilance and state-dependent foraging. Proc. R. Soc. B Biol. Sci. 277, 1469–1474. doi: 10.1098/rspb.2009.2036
Krettek J. E., and Price J. L. (1977). Projections from the amygdaloid complex to the cerebral cortex and thalamus in the rat and cat. J. Comp. Neurol. 172, 687–722.
Krijger I. M., Belmain S. R., Singleton G. R., Groot Koerkamp P. W., and Meerburg B. G. (2017). The need to implement the landscape of fear within rodent pest management strategies. Pest Manag. Sci. U.S.A. 73, 2397–2402. doi: 10.1002/ps.4626
LaManna J. A., and Martin T. E. (2016). Costs of fear: behavioural and life-history responses to risk and their demographic consequences vary across species. Ecol. Lett. 19, 403–413. doi: 10.1111/ele.12573
Lambert O., Pouliquen H., Larhantec M., Thorin C., and L'Hostis M. (2007). Exposure of raptors and waterbirds to anticoagulant rodenticides (Difenacoum, bromadiolone, coumatetralyl, coumafen, brodifacoum): epidemiological survey in Loire Atlantique (France). Bull. Environ. Contam. Toxicol. 79, 91–94. doi: 10.1007/s00128-007-9134-6
Laundré J. W., Hernández L., and Altendorf K. B. (2001). Wolves, elk, and bison: reestablishing the “landscape of fear” in Yellowstone National Park, U.S.A. Can. J. Zool. 79, 1401–1409. doi: 10.1139/z01-094
Leaver L. A., and Daly M. (2003). Effect of predation risk on selectivity in heteromyid rodents. Behav. Processes 64, 71–75. doi: 10.1016/s0376-6357(03)00108-6
Legg C. R., and Lambert S. (1990). Distance estimation in the hooded rat: experimental evidence for the role of motion cues. Behav. Brain Res. 41, 11–20.
Leigh J., and Chamberlain M. J. (2008). “Effects of aversive conditioning on behavior of nuisance Louisiana black bears,” in Human-Wildlife Conflicts, Vol. 2, 175–182. Available online at: http://www.jstor.org/stable/24875663
Leirs H. (2003). “Management of rodents in crops: the Pied Piper and his orchestra,” in Rats, Mice and People: Rodent Biology and Management (Canberra, ACT: Australian Centre for International Agricultural Research), 183–190.
Lima L. L., and Salmon T. P. (2010). “Assessing some potential environmental impacts from agricultural anticoagulant uses,” in Proceedings os the 24th Vertebrate Pest Conference, eds Timm, R. M. and Fagerstone, K. A. (Davis, CA: University of California), 199–203.
Lima S. L. (1990). Evolutionarily stable antipredator behavior among isolated foragers: on the consequences of successful escape. J. Theor. Biol. 143, 77–89.
Lima S. L. (1998). “Stress and decision making under the risk of predation: recent developments from behavioral, reproductive, and ecological perspectives,” in Advances in the Study of Behavior, eds Møller, A. P., Milinski, M., and Slater, P. J. (Academic Press), 215–290.
Lima S. L., and Bednekoff P. A. (1999). Temporal variation in danger drives antipredator behavior: the predation risk allocation hypothesis. Am. Nat. 153, 649–659.
Lima S. L., and Dill L. M. (1990). Behavioral decisions made under the risk of predation: a review and prospectus. Can. J. Zool. 68, 619–640.
Litvin Y., Blanchard D. C., and Blanchard R. J. (2007). Rat 22kHz ultrasonic vocalizations as alarm cries. Behav. Brain Res. 182, 166–172. doi: 10.1016/j.bbr.2006.11.038
Lohr M. T., and Davis R. A. (2018). Anticoagulant rodenticide use, non-target impacts and regulation: a case study from Australia. Sci. Total Environ. 634, 1372–1384. doi: 10.1016/j.scitotenv.2018.04.069
Lore R., Blanc A., and Suedfeld P. (1971). Empathic learning of a passive-avoidance response in domesticated Rattus norvegicus. Anim. Behav. 19, 112–114.
Lund M. (2015). “Commensal rodents,” in Rodent Pests and Their Control, eds Buckle, A. and Smith, R. H. (Wallingford: CAB International), 19–32.
Lyly M. S., Koivisto E., Huitu O., and Korpimäki E. (2018). Simulated owl predation risk to voles modifies browsing effects on tree seedling growth. Ann. Zool. Fennici 55, 93–101. doi: 10.5735/086.055.0109
Ma M. (2012). “Odor and pheromone sensing via chemoreceptors,” in Sensing in nature, ed López-Larrea, C. (New York, NY: Springer), 93–106.
Macdonald D. W., Mathews F., and Berdoy M. (1999). “The behaviour and ecology of Rattus norvegicus: from opportunism to kamikaze tendencies,” in Ecologically-Based Rodent Management, eds Singleton, G. R., Hinds, L. A., Leirs, H., and Zhang, Z. (Canberra, ACT: Australian Centre for International Agricultural Research), 49–80.
MacLeod K. J., Krebs C. J., Boonstra R., and Sheriff M. J. (2018). Fear and lethality in snowshoe hares: the deadly effects of non-consumptive predation risk. Oikos 127, 375–380. doi: 10.1111/oik.04890
Magurran A. E. (1989). Acquired recognition of predator odour in the European Minnow (Phoxinus phoxinus). Ethology 82, 216–223.
Magurran A. E., and Girling S. L. (1986). Predator model recognition and response habituation in shoaling minnows. Anim. Behav. 34, 510–518.
Mahlaba T. A., Monadjem A., McCleery R., and Belmain S. R. (2017). Domestic cats and dogs create a landscape of fear for pest rodents around rural homesteads. PLoS ONE 12:e0171593. doi: 10.1371/journal.pone.0171593
Makundi R. H., Oguge N. O., and Mwanjabe P. S. (1999). “Rodent pest management in East Africa - an ecological approach,” in Ecologically-Based Rodent Management, eds Singleton, G. R., Hinds, L. A., Leirs, H., and Zhang, Z. (Canberra, ACT: Australian Centre for International Agricultural Research), 460–476.
Mao J. S., Boyce M. S., Smith D. W., Singer F. J., Vales D. J., Vore J. M., et al. (2010). Habitat selection by elk before and after wolf reintroduction in Yellowstone National Park. J. Wildl. Manage. 69, 1691–1707. doi: 10.2193/0022-541X(2005)69[1691:HSBEBA]2.0.CO;2
Martínez A. E., Parra E., Collado L. F., and Vredenburg V. T. (2017). Deconstructing the landscape of fear in stable multi-species societies. Ecology 98, 2447–2455. doi: 10.1002/ecy.1935
Matassa C. M., and Trussell G. C. (2011). Landscape of fear influences the relative importance of consumptive and nonconsumptive predator effects. Ecology 92, 2258–2266. doi: 10.1890/11-0424.1
Mathis A., and Vincent F. (2000). Differential use of visual and chemical cues in predator recognition and threat-sensitive predator-avoidance responses by larval newts (Notophthalmus viridescens). Can. J. Zool. 78, 1646–1652. doi: 10.1139/z00-090
Mccleery R. A. (2009). Changes in fox squirrel anti-predator behaviors across the urban–rural gradient. Landsc. Ecol. 24:483. doi: 10.1007/s10980-009-9323-2
McGregor I. S., Hargreaves G. A., Apfelbach R., and Hunt G. E. (2004). Neural correlates of cat odor-induced anxiety in rats: region-specific effects of the benzodiazepine midazolam. J. Neurosci. 24, 4134–4144. doi: 10.1523/jneurosci.0187-04.2004
McNally G. P., Johansen J. P., and Blair H. T. (2011). Placing prediction into the fear circuit. Trends Neurosci. 34, 283–292. doi: 10.1016/j.tins.2011.03.005
Meerburg B. G., Singleton G. R., and Kijlstra A. (2009a). Rodent-borne diseases and their risks for public health. Crit. Rev. Microbiol. 35, 221–270. doi: 10.1080/10408410902989837
Meerburg B. G., Singleton G. R., and Leirs H. (2009b). The year of the rat ends—time to fight hunger! Pest Manag. Sci. 65, 351–352. doi: 10.1002/ps.1718
Meerburg B. G., van Gent-Pelzer M. P., Schoelitsz B., and van der Lee T. A. (2014). Distribution of anticoagulant rodenticide resistance in Rattus norvegicus in the Netherlands according to Vkorc1 mutations. Pest Manag. Sci. 70, 1761–1766. doi: 10.1002/ps.3809
Miller M. W., and Vogt B. A. (1984). Direct connections of rat visual cortex with sensory, motor, and association cortices. J. Comp. Neurol. 226, 184–202.
Mills J. N. (1999). “The role of rodents in emerging human disease: examples from the hantaviruses and arenaviruses,” in Ecologically-Based Rodent Management, eds Singleton, G. R., Hinds, L. A., Leirs, H., and Zhang, Z. (Canberra, ACT: Australian Centre for International Agricultural Research), 134–160.
Mineka S., and Cook M. (1988). “Social learning and the acquisition of snake fear in monkeys,” in Social Learning: Psychological and Biological Perspectives, eds Zentall, T. R., Galef, J. B. G., and Zentall, T. R. (Mahwah, NJ: Lawrence Erlbaum Associates, Inc.), 24.
Mirza R. S., and Chivers D. P. (2000). Predator-recognition training enhances survival of brook trout: evidence from laboratory and field-enclosure studies. Can. J. Zool. 78, 2198–2208. doi: 10.1139/z00-164
Mitra R., Sapolsky R. M., and Vyas A. (2012). Toxoplasma gondii infection induces dendritic retraction in basolateral amygdala accompanied by reduced corticosterone secretion. Dis. Model. Mech. 6, 516–520. doi: 10.1242/dmm.009928
Monclús R., Rödel H. G., Von Holst D., and De Miguel J. (2005). Behavioural and physiological responses of naïve European rabbits to predator odour. Anim. Behav. 70, 753–761. doi: 10.1016/j.anbehav.2004.12.019
Mongeau R., Miller G. A., Chiang E., and Anderson D. J. (2003). Neural correlates of competing fear behaviors evoked by an innately aversive stimulus. J. Neurosci. 23, 3855–3868. doi: 10.1523/JNEUROSCI.23-09-03855.2003
Morris D. W. (1997). Optimally foraging deer mice in prairie mosaics: a test of habitat theory and absence of landscape effects. Oikos 80, 31–42.
Mucignat-Caretta C. (2010). The rodent accessory olfactory system. J. Comp. Physiol. A Neuroethol. Sensory, Neural, Behav. Physiol. 196, 767–777. doi: 10.1007/s00359-010-0555-z
Muller-Schwarze D. (2006). Chemical Ecology of Vertebrates. Cambridge: Cambridge University Press. doi: 10.1017/CBO9780511607233
Naidenko S. V., Naidenko S. V., Clark L., and Voznessenskaya V. V. (2003). Predator Presence Affects the Reproductive Success of Prey in Outdoor Conditions. Vol. 203, 1–6. Available online at: https://digitalcommons.unl.edu/icwdm_usdanwrc/203
National Research Council (2010). Guide for the Care and Use of Laboratory Animals. Washington, DC: National Academies Press.
Nowak K., Le Roux A., Richards S. A., Scheijen C. P. J., and Hill R. A. (2014). Human observers impact habituated samango monkeys' perceived landscape of fear. Behav. Ecol. 25, 1199–1204. doi: 10.1093/beheco/aru110
Ojeda F. P., and Muñoz A. A. (1999). Feeding selectivity of the herbivorous fish Scartichthys viridis: effects on macroalgal community structure in a temperate a rocky intertidal coastal zone. Mar. Ecol. Prog. Ser. 184, 219–229.
Osada K., Miyazono S., and Kashiwayanagi M. (2014). Pyrazine analogs are active components of wolf urine that induce avoidance and fear-related behaviors in deer. Front. Behav. Neurosci. 8:276. doi: 10.3389/fnbeh.2014.00276
Pangle K. L., Peacor S. D., and Johannsson O. E. (2007). Large nonlethal effects of an invasive invertebrate predator on zooplankton population growth rate. Ecology 88, 402–412. doi: 10.1890/06-0768
Parsons M. H., Apfelbach R., Banks P. B., Cameron E. Z., Dickman C. R., Frank A. S. K., et al. (2017). Biologically meaningful scents: a framework for understanding predator-prey research across disciplines. Biol. Rev. 93, 98–114. doi: 10.1111/brv.12334
Parsons M. H., and Blumstein D. T. (2010). Familiarity breeds contempt: kangaroos persistently avoid areas with experimentally deployed dingo scents. PLoS ONE 5:e10403. doi: 10.1371/journal.pone.0010403
Pelz H.-J. (2007). Spread of resistance to anticoagulant rodenticides in Germany. Int. J. Pest Manag. 53, 299–302. doi: 10.1080/09670870701245223
Pelz H. J., Rost S., Hünerberg M., Fregin A., Heiberg A. C., Baert K., et al. (2005). The genetic basis of resistance to anticoagulants in rodents. Genetics 170, 1839–1847. doi: 10.1534/genetics.104.040360
Pérez-Gómez A., Bleymehl K., Stein B., Pyrski M., Birnbaumer L., Munger S. D., et al. (2015). Innate predator odor aversion driven by parallel olfactory subsystems that converge in the ventromedial hypothalamus. Curr. Biol. 25, 1340–1346. doi: 10.1016/j.cub.2015.03.026
Perrot-Sinal T., Ossenkopp K. P., and Kavaliers M. (2000). Influence of a natural stressor (predator odor) on locomotor activity in the meadow vole (Microtus pennsylvanicus): modulation by sex, reproductive condition and gonadal hormones. Psychoneuroendocrinology 25, 259–276. doi: 10.1016/S0306-4530(99)00054-2
Perrot-Sinal T. S., Heale V. R., Ossenkopp K. -P., and Kavaliers M. (1996). Sexually dimorphic aspects of spontaneous activity in meadow voles (Microtus pennsylvanicus): effects of exposure to fox odor. Behav. Neurosci. 110, 1126–1132. doi: 10.1037/0735-7044.110.5.1126
Perrot-Sinal T. S., Ossenkopp K.-P. P., and Kavaliers M. (1999). Effects of repeated exposure to fox odor on locomotor activity levels and spatial movement patterns in breeding male and female meadow voles (Microtus pennsylvanicus). J. Chem. Ecol. 25, 1567–1584.
Piekarski G., Zippelius H. M., and Witting P. A. (1978). Auswirkungen einer latenten Toxoplasma-infektion auf das lernvermogen von weiben laboratoriumsratten und -mausen. Z. Parasitenkd. 57, 1–15.
Portfors C. V. (2007). Types and functions of ultrasonic vocalizations in laboratory rats and mice. J. Am. Assoc. Lab. Anim. Sci. 46, 28–34. Available online at: http://docserver.ingentaconnect.com/deliver/connect/aalas/15596109/v46n1/s5.pdf?expires=1555202270&id=0000&titleid=72010024&checksum=5B489695FC2D58757E685F6847D2A840
Rankin C. H., Abrams T., Barry R. J., Bhatnagar S., Clayton D. F., Colombo J., et al. (2009). Habituation revisited: an updated and revised description of the behavioral characteristics of habituation. Neurobiol. Learn. Mem. 92, 135–138. doi: 10.1016/j.nlm.2008.09.012
Rasmussen L. E. (1988). Chemosensory responses in two species of elephants to constituents of temporal gland secretion and musth urine. J. Chem. Ecol. 14, 1687–1711. doi: 10.1007/BF01014552
Rattner B. A., and Mastrota F. N. (2018). “Anticoagulant rodenticide toxicity to non-target wildlife under controlled exposure conditions,” in Anticoagulant Rodenticides and Wildlife, eds van den Brink, N. W., Elliott, J. E., Shore, R. F., and Rattner, B. A. (Cham: Springer International Publishing), 45–86.
Raveh A., Kotler B. P., Abramsky Z., and Krasnov B. R. (2011). Driven to distraction: detecting the hidden costs of flea parasitism through foraging behaviour in gerbils. Ecol. Lett. 14, 47–51. doi: 10.1111/j.1461-0248.2010.01549.x
Remtulla S., and Hallett P. E. (1985). A schematic eye for the mouse, and comparisons with the rat. Vision Res. 25, 21–31.
Rescorla R. A., and Wagner A. R. (1972). “A theory of Pavlovian conditioning : variations in the effectiveness of reinforcement and nonreinforcement,” in Classical Conditioning II: Current Research and Theory, eds Black, A. H. and Prokasy, W. F. (New York, NY: Appleton-Century-Crofts), 64–99.
Rodriguez M. J. A. (1993). Roedores Plagas: un Problema Permanente en America Latina y el Caribe. Santiago: Oficina Regional de la FAO para America Latina y el Caribe.
Rost S., Pelz H. -J., Menzel S., MacNicoll A. D., León V., Song K. -J., et al. (2009). Novel mutations in the VKORC1 gene of wild rats and mice – a response to 50 years of selection pressure by warfarin? BMC Genet. 10:4. doi: 10.1186/1471-2156-10-4
Rusiniak K. W., Hankins W. G., Garcia J., and Brett L. P. (1979). Flavor-illness aversions: potentiation of odor by taste in rats. Behav. Neural Biol. 25, 1–17.
Russell J. T. (1932). Depth discrimination in the rat. Pedagog. Semin. J. Genet. Psychol. 40, 136–161.
Russon A. E. (1997). “Exploiting the expertise of others,” in Machiavellian Intelligence II: Extensions and Evaluations, eds Whiten, A. and Byrne, R. W. (Cambridge: Cambridge University Press), 174–206.
Ruxton G. D., and Lima S. L. (1997). Predator–induced breeding suppression and its consequences for predator–prey population dynamics. Proc. R. Soc. London. Ser. B Biol. Sci. 264, 409–415.
Samia D. S., Nakagawa S., Nomura F., Rangel T. F., and Blumstein D. T. (2015). Increased tolerance to humans among disturbed wildlife. Nat. Commun. 6:8877. doi: 10.1038/ncomms9877
Sanders J., Mayford M., and Jeste D. (2013). Empathic fear responses in mice are triggered by recognition of a shared experience. PLoS ONE 8:e74609. doi: 10.1371/journal.pone.0074609
Schakner Z. A., and Blumstein D. T. (2013). Behavioral biology of marine mammal deterrents: a review and prospectus. Biol. Conserv. 167, 380–389. doi: 10.1016/j.biocon.2013.08.024
Schaller G. B. (1972). The Serengeti Lion: A Study of Predator-Prey Relations. Chicago, IL: University of Chicago Press.
Scherer A. E., and Smee D. L. (2016). A review of predator diet effects on prey defensive responses. Chemoecology 26, 83–100. doi: 10.1007/s00049-016-0208-y
Schmeisser E., Pollard K. A., and Letowski T. (2013). Olfaction Warfare: Odor as Sword and Shield. Aberdeen: Army Research Laboratory. doi: 10.21236/ADA577342
Schmidt K. A., Brown J. S., and Morgan R. A. (1998). Plant defenses as complementary resources: a test with squirrels. Oikos 81, 130–142.
Schmitz O. J., Beckerman A. P., and O'Brien K. M. (1997). Behaviorally mediated trophic cascades: effects of predation risk on food web interactions. Ecology 78, 1388–1399.
Schoeppner N. M., and Relyea R. A. (2009). Interpreting the smells of predation: how alarm cues and kairomones induce different prey defences. Funct. Ecol. 23, 1114–1121. doi: 10.1111/j.1365-2435.2009.01578.x
Schulte B. A. (2016). “Learning and applications of chemical signals in vertebrates for human–wildlife conflict mitigation,” in Chemical Signals in Vertebrates 13, eds Schulte, B. A., Goodwin, T. E., and Ferkin, M. H. (Cham: Springer International Publishing), 499–510.
Schwanz L. E., Brisson D., Gomes-Solecki M., and Ostfeld R. S. (2011). Linking disease and community ecology through behavioural indicators: immunochallenge of white-footed mice and its ecological impacts. J. Anim. Ecol. 80, 204–214. doi: 10.1111/j.1365-2656.2010.01745.x
Schwanz L. E., Previtali M. A., Gomes-Solecki M., Brisson D., and Ostfeld R. S. (2012). Immunochallenge reduces risk sensitivity during foraging in white-footed mice. Anim. Behav. 83, 155–161. doi: 10.1016/j.anbehav.2011.10.020
Semlitsch R. D., and Reyer H.-U. (1992). Modification of anti-predator behaviour in tadpoles by environmental conditioning. J. Anim. Ecol. 61, 353–360.
Shoham S., Marcus E. -L., Avraham Y., and Berry E. M. (2000). Diet restriction increases enkephalin- and dynorphin-like immunoreactivity in rat brain and attenuates long-term retention of passive avoidance. Nutr. Neurosci. 3, 41–55. doi: 10.1080/1028415X.2000.11747302
Siegmund A., and Wotjak C. T. (2007). A mouse model of posttraumatic stress disorder that distinguishes between conditioned and sensitised fear. J. Psychiatr. Res. 41, 848–860. doi: 10.1016/j.jpsychires.2006.07.017
Singleton G. R. (2003). Impacts of Rodents on Rice Production in Asia. Los Baños: International Rice Research Institute. Available online at: http://books.irri.org/DPS45_content.pdf
Singleton G. R., Brown P. R., Jacob J., and Aplin K. P. (2007). Unwanted and unintended effects of culling: a case for ecologically-based rodent management. Integr. Zool. 2, 247–259. doi: 10.1111/j.1749-4877.2007.00067.x
Singleton G. R., Hinds L. A., Leirs H., and Zhang Z. (1999). Ecologically-Based Rodent Management, eds Singleton, G. R., Hinds, L. A., Leirs, H., and Zhang, Z. (Canberra, ACT: Australian Centre for International Agricultural Research).
Smith J. A., Suraci J. P., Clinchy M., Crawford A., Roberts D., Zanette L. Y., et al. (2017). Fear of the human ‘super predator' reduces feeding time in large carnivores. Proc. R. Soc. B Biol. Sci. 284:20170433. doi: 10.1098/rspb.2017.0433
Sparrow E. E., Parsons M. H., and Blumstein D. T. (2016). Novel use for a predator scent: preliminary data suggest that wombats avoid recolonising collapsed burrows following application of dingo scent. Aust. J. Zool. 64, 192–197 doi: 10.1071/ZO15068
Srivastava A. (1991). Cultural transmission of snake-mobbing in free-ranging Hanuman langurs. Folia Primatol. 56, 117–120.
Stankowich T., and Blumstein D. T. (2005). Fear in animals: a meta-analysis and review of risk assessment. Proc. R. Soc. B Biol. Sci. 272, 2627–2634. doi: 10.1098/rspb.2005.3251
Staples L. G., Hunt G. E., Cornish J. L., and McGregor I. S. (2005). Neural activation during cat odor-induced conditioned fear and ‘trial 2' fear in rats. Neurosci. Biobehav. Rev. 29, 1265–1277. doi: 10.1016/j.neubiorev.2005.04.009
Staples L. G., McGregor I. S., Apfelbach R., and Hunt G. E. (2008). Cat odor, but not trimethylthiazoline (fox odor), activates accessory olfactory and defense-related brain regions in rats. Neuroscience 151, 937–947. doi: 10.1016/j.neuroscience.2007.11.039
Stenseth N. C., Leirs H., Skonhoft A., Davis S. A., Pech R. P., Andreassen H. P., et al. (2003). Mice, rats, and people: the bio-economics of agricultural rodent pests. Front. Ecol. Environ. 1, 367–375. doi: 10.1890/1540-9295(2003)001[0367:MRAPTB]2.0.CO;2
Stephens D. W., and Krebs J. (1986). Foraging Theory, 1st Edn, eds Krebs, J. R. and Clutto-Brock, T. (Princeton, NJ: Princenton University Press).
Stephens M. A., and Wand G. (2012). Stress and the HPA axi: role of glucocorticoids in alcohol dependence. Alcohol Res. Curr. Rev. 34, 468–483. Available online at: https://pubs.niaaa.nih.gov/publications/arcr344/468-483.pdf
Suraci J. P., Clinchy M., Dill L. M., Roberts D., and Zanette L. Y. (2016). Fear of large carnivores causes a trophic cascade. Nat. Commun. 7:10698. doi: 10.1038/ncomms10698
Suraci J. P., Clinchy M., Mugerwa B., Delsey M., Macdonald D. W., Smith J. A., et al. (2017a). A new automated behavioural response system to integrate playback experiments into camera trap studies. Methods Ecol. Evol. 8, 957–964. doi: 10.1111/2041-210X.12711
Suraci J. P., Roberts D. J., Clinchy M., and Zanette L. Y. (2017b). Fearlessness towards extirpated large carnivores may exacerbate the impacts of naïve mesocarnivores. Behav. Ecol. 28. doi: 10.1093/beheco/arw178
Swanepoel L. H., Swanepoel C. M., Brown P. R., Eiseb S. J., Goodman S. M., Keith M., et al. (2017). A systematic review of rodent pest research in Afro-Malagasy small-holder farming systems: are we asking the right questions? PLoS ONE 12:e0174554. doi: 10.1371/journal.pone.0174554
Talling J. C., Waran N. K., Wathes C. M., and Lines J. A. (1998). Sound avoidance by domestic pigs depends upon characteristics of the signal. Appl. Anim. Behav. Sci. 58, 255–266.
Taylor K. D., Hammond L. E., and Quy R. J. (1974). The reactions of common rats to four types of live-capture trap. J. Appl. Ecol. 11, 453–459.
Tenter A. M., Heckeroth A. R., and Weiss L. M. (2000). Toxoplasma gondii: from animals to humans. Int. J. Parasitol. 30, 1217–1258. doi: 10.1016/S0020-7519(00)00124-7
Thijssen H. H. W. (1995). Warfarin based rodenticides: mode of action and mechanism of resistance. Pestic. Sci. 43, 73–78.
Thomas P. J., Mineau P., Shore R. F., Champoux L., Martin P. A., Wilson L. K., et al. (2011). Second generation anticoagulant rodenticides in predatory birds: probabilistic characterisation of toxic liver concentrations and implications for predatory bird populations in Canada. Environ. Int. 37, 914–920. doi: 10.1016/j.envint.2011.03.010
Thorson J. M., Morgan R. A., Brown J. S., and Norman J. E. (1998). Direct and indirect cues of predatory risk and patch use by fox squirrels and thirteen-lined ground squirrels. Behav. Ecol. 9, 151–157.
Tobin M. E., and Fall M. W. (2006). “Pest control: rodents,” in U.S. Department of Agriculture's National Wildlife Research Center (Staff Publication), 67, 1–21. Available online at: https://digitalcommons.unl.edu/cgi/viewcontent.cgi?article=1065&context=icwdm_usdanwrc
Tollrian R., and Harvell C. D. (1999). “The evolution of inducible defenses: current ideas,” in The Ecology and Evolution of Inducible Defenses, eds Tollrian, R. and Harvell, C. D. (Princeton, NJ: Princeton University Press), 306–321.
Topál J., and Csányi V. (1994). The effect of eye-like schema on shuttling activity of wild house mice (Mus musculus domesticus): context-dependent threatening aspects of the eyespot patterns. Anim. Learn. Behav. 22, 96–102.
Tromelin A. (2016). Odour perception: a review of an intricate signalling pathway. Flavour Fragr. J. 31, 107–119. doi: 10.1002/ffj.3295
Turner G. F., and Pitcher T. J. (1986). Attack abatement: a model for group protection by combined avoidance and dilution. Am. Nat. 128, 228–240.
Uetz G. W., and Hieber C. S. (1994). Group size and predation risk in colonial web-building spiders: analysis of attack abatement mechanisms. Behav. Ecol. 5, 326–333.
Valeix M., Loveridge A. J., Chamaillé-Jammes S., Davidson Z., Murindagomo F., Fritz H., et al. (2009). Behavioral adjustments of African herbivores to predation risk by lions: spatiotemporal variations influence habitat use. Ecology 90, 23–30. doi: 10.1890/08-0606.1
van Donk E., Lürling M., and Lampert W. (1999). “Consumer-induced changes in phytoplankton: inducibility, costs, benefits, and the impact on grazers,” in The Ecology and Evolution of Inducible Defenses, eds Tollrian, R. and Harvell, C. D. (Princeton, NJ: Princeton University Press), 89–103.
van Eeden L. M., Crowther M. S., Dickman C. R., Macdonald D. W., Ripple W. J., Ritchie E. G., et al. (2017). Managing conflict between large carnivores and livestock. Conserv. Biol. 32, 26–34. doi: 10.1111/cobi.12959
Vasudevan A., and Vyas A. (2013). Kairomonal communication in mice is concentration-dependent with a proportional discrimination threshold. F1000Research 2, 195. doi: 10.12688/f1000research.2-195.v1
Veen T., Richardson D. S., Blaakmeer K., and Komdeur J. (2000). Experimental evidence for innate predator recognition in the Seychelles warbler. Proc. R. Soc. London. Ser. B Biol. Sci. 267, 2253–2258. doi: 10.1098/rspb.2000.1276
Verma D., Wood J., Lach G., Herzog H., Sperk G., and Tasan R. (2016). Hunger promotes fear extinction by activation of an amygdala microcircuit. Neuropsychopharmacology 41, 431–439. doi: 10.1038/npp.2015.163
Vernet-Maury E., Polak E. H., and Demael A. (1984). Structure-activity relationship of stress-inducing odorants in the rat. J. Chem. Ecol. 10, 1007–1018.
Vilhunen S., and Hirvonen H. (2003). Innate antipredator responses of Arctic charr (Salvelinus alpinus) depend on predator species and their diet. Behav. Ecol. Sociobiol. 55, 1–10. doi: 10.1007/s00265-003-0670-8
Von Frisch K. (1942). Über einen schreckstoff der fischhaut und seine biologische bedeutung. Z. Vgl. Physiol. 29, 46–145.
Voznessenskaya V. V., Naidenko S. V., Feoktistova N. Y., Krivomazov G. J., Miller L. A., and Clark L. (2003). Predator Odours as Reproductive Inhibitors for Norway Rats. USDA National Wildlife Research Center – Staff Publications, 251.
Vyas A., Kim S. K., Giacomini N., Boothroyd J. C., and Sapolsky R. M. (2007b). Behavioral changes induced by Toxoplasma infection of rodents are highly specific to aversion of cat odors. Proc. Natl. Acad. Sci. U.S.A. 104, 6442–6447. doi: 10.1073/pnas.0608310104
Vyas A., Kim S. K., and Sapolsky R. M. (2007a). The effects of Toxoplasma infection on rodent behavior are dependent on dose of the stimulus. Neuroscience 148, 342–348. doi: 10.1016/j.neuroscience.2007.06.021
Vyas A., and Sapolsky R. (2010). Manipulation of host behaviour by Toxoplasma gondii: what is the minimum a proposed proximate mechanism should explain? Folia Parasitol. 57, 88–94. doi: 10.14411/fp.2010.011
Walker L. A., Turk A., Long S. M., Wienburg C. L., Best J., and Shore R. F. (2008). Second generation anticoagulant rodenticides in tawny owls (Strix aluco) from Great Britain. Sci. Total Environ. 392, 93–98. doi: 10.1016/j.scitotenv.2007.10.061
Wallace D. J., Greenberg D. S., Sawinski J., Rulla S., Notaro G., and Kerr J. N. (2013). Rats maintain an overhead binocular field at the expense of constant fusion. Nature 498, 65–69. doi: 10.1038/nature12153
Wang L., Chen I. Z., and Lin D. (2015). Collateral pathways from the ventromedial hypothalamus mediate defensive behaviors. Neuron 85, 1344–1358. doi: 10.1016/j.neuron.2014.12.025
Ward J. F., Macdonald D. W., and Doncaster C. P. (1997). Responses of foraging hedgehogs to badger odour. Anim. Behav. 53, 709–720.
Webster J. P. (2007). The effect of Toxoplasma gondii on animal behavior: playing cat and mouse. Schizophr. Bull. 33, 752–756. doi: 10.1093/schbul/sbl073
Webster J. P., Brunton C. F., and Macdonald D. W. (1994). Effect of Toxoplasma gondii upon neophobic behaviour in wild brown rats, Rattus norvegicus. Parasitology 109, 37–43. doi: 10.1017/S003118200007774X
Williams J. L., Rogers A. G., and Adler A. P. (1990). Prolonged exposure to conspecific and predator odors reduces fear reactions to these odors during subsequent prod-shock tests. Anim. Learn. Behav. 18:453. doi: 10.3758/BF03205327
Wirsing A. J., Heithaus M. R., Frid A., and Dill L. M. (2008). Seascapes of fear: evaluating sublethal predator effects experienced and generated by marine mammals. Mar. Mammal Sci. 24, 1–15. doi: 10.1111/j.1748-7692.2007.00167.x
Witting P. A. (1979). Learning capacity and memory of normal and Toxoplasma-infected laboratory rats and mice. Zeitschrift für Parasitenkd. 61, 29–51.
Yilmaz M., and Meister M. (2013). Rapid innate defensive responses of mice to looming visual stimuli. Curr. Biol. 23, 2011–2015. doi: 10.1016/j.cub.2013.08.015
Ylönen H., and Brown J. S. (2007). “Fear and the foraging, breeding, and sociality of rodents,” in Rodent Societies. An Ecological & Evolutionary Perspective, eds Wolff, J. O. and Sherman, P. W. (Chicago, IL: The University of Chicago Press), 328–341.
Ylönen H., Jacob J., Davies M. J., and Singleton G. R. (2002). Predation risk and habitat selection of Australian house mice, Mus domesticus, during an incipient plague: desperate behaviour due to food depletion. Oikos 99, 284–289. doi: 10.1034/j.1600-0706.2002.990208.x
Zanette L. Y., White A. F., Allen M. C., and Clinchy M. (2011). Perceived predation risk reduces the number of offspring songbirds produce per year. Science 334, 1398–1401. doi: 10.1126/science.1210908
Keywords: non-consumptive effects, anti-predator response, fear, predator cues, learning, fear conditioning, pest rodents
Citation: Bedoya-Pérez MA, Smith KL, Kevin RC, Luo JL, Crowther MS and McGregor IS (2019) Parameters That Affect Fear Responses in Rodents and How to Use Them for Management. Front. Ecol. Evol. 7:136. doi: 10.3389/fevo.2019.00136
Received: 23 October 2018; Accepted: 05 April 2019;
Published: 08 May 2019.
Edited by:
Bastiaan G. Meerburg, Wageningen University and Research, NetherlandsReviewed by:
Inge Milou Krijger, Wageningen University and Research, NetherlandsCopyright © 2019 Bedoya-Pérez, Smith, Kevin, Luo, Crowther and McGregor. This is an open-access article distributed under the terms of the Creative Commons Attribution License (CC BY). The use, distribution or reproduction in other forums is permitted, provided the original author(s) and the copyright owner(s) are credited and that the original publication in this journal is cited, in accordance with accepted academic practice. No use, distribution or reproduction is permitted which does not comply with these terms.
*Correspondence: Miguel A. Bedoya-Pérez, bWlndWVsLmJlZG95YXBlcmV6QHN5ZG5leS5lZHUuYXU=
Disclaimer: All claims expressed in this article are solely those of the authors and do not necessarily represent those of their affiliated organizations, or those of the publisher, the editors and the reviewers. Any product that may be evaluated in this article or claim that may be made by its manufacturer is not guaranteed or endorsed by the publisher.
Research integrity at Frontiers
Learn more about the work of our research integrity team to safeguard the quality of each article we publish.