- Division of Biological Sciences, The University of Montana, Missoula, MT, United States
I focus on the foraging efficiency (food intake per time) of a hypothetical forager that uses distinct levels of information about a set of discrete renewing resources. The information may be the distance to the resource (D), both distance and maximum productivity of the resource (DP), or distance, productivity, and the elapsed time since the forager last visited each resource (DPT). The expected foraging efficiencies are based on the results of a field experiment on wild capuchin monkeys (Janson, 2016a) that documented the use of integrative memories of resource location, productivity, and elapsed time since the previous visit. Computer simulations of the choice process are used to extend the resource parameter space beyond the field experiment. The goal is to explore to what extent the use of elapsed time in choosing discrete feeding sites is a benefit under various conditions of resource and consumer characteristics. Major findings include that the use of elapsed time generally increases foraging efficiency on renewing resources by 30–35%, without much effect of resource size or variability—indeed, DPT outperforms D or DP choice even when all resources have the same productivity. The relative benefit of DPT increases when the most productive resources are only moderately common (not so rare that they contribute little to food intake, nor so common that choosing the nearest resource is likely to arrive at a highly productive one). The relative benefit of DPT decreases when the forager's gut capacity is small or gut passage time is long because it cannot ingest all of the resource accumulated after long elapsed intervals. I suggest that memory for and use of elapsed intervals since prior visits in foraging choices will prove to be a common feature of diverse foraging animals whenever the presence of a forager depresses food availability in a local area.
Introduction
One of the persistent mysteries about primates is why, as an order, they have evolved relatively large brains for their body size compared to most other mammals (e.g., Martin, 1981; Allman, 1999). Two main approaches to answer this question approach it from opposite perspectives: the costs of having a large brain vs. the benefits of having it. Brain tissue is metabolically expensive (among the most expensive of all mammalian tissues: Allman, 1999), so having a large brain requires having enough energetic intake to support it. One approach, then, is to examine whether energetic constraints of diet, morphology, or habitat may explain variation in relative brain mass (Martin, 1981; Isler and Van Schaik, 2006). The premise of these studies is that larger brains are generally good for fitness, if the individual can afford them metabolically; why a large brain should be good for fitness is not necessarily specified.
The other approach focuses on why a larger brain might improve individual fitness. The major competing hypotheses look at the use of brains in social intelligence (review: Seyfarth and Cheney, 2012), foraging efficiency (e.g., Menzel, 1973; Milton, 1981; Janson, 2014), and tool use (e.g., Boesch and Boesch, 1984; Antinucci and Visalberghi, 1986). A common assumption of all these approaches is that relatively larger brains (or sometimes specific brain regions) are associated with more advanced cognitive abilities. While this assumption has some support (Reader and Laland, 2002), the correlation is far from perfect either between or within species (Holekamp et al., 2015). The social intelligence hypothesis may be a compelling general explanation of the benefit of advanced cognition (review: Wascher et al., 2018). However, the specific mechanism by which more advanced cognition benefits individuals socially is not always specified, although there is strong evidence that more socially-connected individuals often have higher fitness in primates (e.g., Silk et al., 2003). In any case, the social intelligence hypothesis does not directly address the costs of large brain size or provide an energetic mechanism to compensate for such costs. The foraging efficiency and tool use hypotheses have the potential to connect the costs and benefits of having larger brains, since both hypotheses postulate that more advanced cognitive skills can open up new feeding opportunities (e.g., Cognitive Buffer Hypothesis: Sol, 2009) or allow more efficient use of existing foraging options (review: Trapanese et al., 2018). It is this last possibility that I explore in this article. In particular, I ask how much more efficient is a foraging primate group that uses integrative “what-where-when” memory of food availability to plan patch-foraging decisions, compared to using only “where” or “where-what” information.
Increasing numbers of studies have shown that diverse primate species show strong evidence that they remember the locations of renewing food patches (“where” memory) and are able to organize these memories into a functional “mental map” that allows them to navigate in relatively direct routes from one feeding location to another (e.g., Menzel, 1978; Gallistel and Cramer, 1996; Janson, 1998; Bicca-Marques and Garber, 2004; Noser and Byrne, 2007). Quite a few studies have focused on whether primates choose optimal routes to travel among several goals, or use particular decision rules to plan multi-destination routes (e.g., Menzel, 1973; MacDonald and Wilkie, 1990; Cramer and Gallistel, 1997; Teichroeb, 2015; Janson, 2016a). Evidence for possible multi-goal planning is weak, contradictory, and at best seems to be limited to a few (2–3) future goals (review in Janson, 2014). Luckily, the details of route-planning may not matter much to foraging efficiency because even the simplest spatially-explicit foraging rule (“move the nearest food patch not recently used”) may provide long-term foraging efficiency quite close to that of optimal solutions (Anderson, 1983).
In addition to knowing where food sources are, primates may know how productive each resource is (“where-what” memory: e.g., Garber, 1989; Janson, 1998; Janmaat et al., 2006a; Cunningham and Janson, 2007). Evidence for knowledge of resource productivity typically requires showing that primates will prefer more distant resource patches only if these provide more food (Garber, 1988; Cunningham and Janson, 2007; Janson, 2007; Janmaat et al., 2014) or that they are more likely to visit more productive resources from a given distance (Janmaat et al., 2006a). Because most primate foods vary in productivity over time, both across years and seasons as well as within a season, knowing the long-term average productivity of a food patch is not much use unless coupled with more immediate knowledge of its fruiting state. Fruiting state has several components: (1) Does the patch contain ripe fruit at all; (2) How quickly are the fruit ripening? (3) How long has it been since the primate (or group) previously visited a given patch? The last of these components is important because primates may often exhaust the ripe fruit in a patch so that its energetic value drops to near zero by the end of a feeding bout (Janson, 1988).
Evidence for knowledge of time-varying plant fruiting state by wild primates is sparse but continues to accumulate (review: Trapanese et al., 2018). Some primates apparently keep track of seasonal patterns of fruiting of at least a few of their food species (e.g., Menzel, 1991; Janmaat et al., 2011), and may even anticipate different rates of ripening according to weather conditions (Janmaat et al., 2006b). Both of these kinds of knowledge require updating the productivity status of resources, implying dynamic “where-what” memory, but they do not necessarily require keeping track of elapsed time since a previous feeding visit, which might imply “where-what-when” memory. While anecdotal evidence is abundant that primates rarely return to recently-used resources or foraging areas, only a few recent studies provide strong evidence that primates may remember and use location-specific memory of time elapsed since a prior visit (Janson, 2016a; Tujague and Janson, 2017).
While it may seem obvious that the use of “where-what-when” memory will provide better foraging decisions than use of “where-what” or only “where” memory, it is not clear how much of an energetic advantage more complex cognition may provide. Differences in energy gain between individuals with different cognitive strategies using renewing resources can be large (6.3-fold for hummingbirds: González-Gómez et al., 2011). However, just as simple spatial foraging rules may provide excellent approximations to complex route-choice problems (Anderson, 1983), it might also be true that “where-what-when” foraging decisions perform only marginally better than simple decision rules that ignore elapsed time but incorporate avoidance of any resources that were recently used (e.g., within a given day).
To assess the relative foraging benefits from using different kinds of cognition in foraging choices, I use two approaches. First, I use a new statistical analysis of previously-gathered data from a field experiment using feeding platforms in a wild population of brown capuchin monkeys (Sapajus nigritus) in Argentina (Janson, 2016a). That study revealed that their movement decisions were significantly better explained by the use of “where-what-when” memory than either “where” or “where-what” memory. The statistical model used for that analysis made predictions about each next choice of feeding site, given the assumed knowledge of different traits of the feeding sites. For the current study, I use a similar analysis that compares predicted site choice of the monkey group if the independent variable(s) were: (1) distance; (2) the ratio of average production per site to distance (H1 in Janson, 2016a), and (3) the ratio of time-dependent food production to distance (H2 in Janson, 2016a). I combine the predicted site choices under the three conditions with knowledge of the food reward regime at each site to calculate the expected food intake, the elapsed time and the travel distance for the feeding site choices made under the three levels of feeding site cognition. From these foraging parameters, I calculate a typical measure of expected foraging efficiency: food ingested per unit time. I compare foraging efficiency between the different levels of foraging cognition based on the observed choices in the field data.
Although the experiment provided strong evidence for the use of what-where-when memory in the monkeys' foraging decisions, it was constrained in using only one set of resource parameters: a total of 8 sites, 4 each with 2 levels of total food reward, and a single renewal rate of food as a function of time (Janson, 2016a). Thus, the relative feeding efficiencies derived from that study may hold only for the very particular conditions of that experiment. Therefore, I use computer simulations to mimic the foraging decisions of a hypothetical primate in a field of renewing food patches if it were to use only “where,” “where-what,” or “where-what-when” memory. This approach allows me to verify the results I found in the field experiment (Janson, 2016a) and also to expand the range of possible resource parameters to test how the relative foraging efficiencies of distinct cognitive foraging decisions depend on ecological context.
Methods
Field Experiments
The details of the field experiments are given in Janson (2016a). The essence of the setup was to provide a wild group of tufted capuchin monkeys in Argentina with eight feeding platform sites. Each site consisted of three platforms, on which the monkeys were provided with banana pieces. The platforms were deliberately spaced far enough apart (10 m) to minimize within-group contest competition (Janson, 1996), and the sites were set far enough apart (typically 200 m or more) to minimize the occurrence of group splitting between feeding sites (Janson, 2007). The quantity of banana pieces given at a site depended on the interval elapsed between the last feeding bout at the site and the current one. The number of banana pieces provided increased by 1 every 1 or 2 h, depending on the treatment. Half of the sites received the “low” treatment (1 added piece every 2 h) and the rest had the “high” treatment (1 piece added every hour); for both kinds of sites, the reward was held constant after elapsed time reached 48 h, at 24 and 48 pieces, respectively for the “low” and “high” treatments. The appropriate numbers of banana pieces were placed on the platforms of a site when the group had approached within 50 m of the platforms. Because no bananas were placed at a site until the group had nearly arrived, the monkeys could not use odor or visual cues to decide either where the platforms were or how much food would be there when it was provisioned. The experiments were run for a total of 68 days, during which the provisioning treatment was reversed at each site, to control for possible site-specific visitation preferences that had nothing to do with the provisioning regime. Each movement sequence from one site to another was considered to constitute a choice, conditionally independent of other choices in the movement sequence. The set of choices was fit to a sequence of increasing complex cognitive models with multinomial logistic regression using the “Choice” platform in JMP 11.0 (SAS Software Corp.). The resulting models provided expected probabilities of moving among feeding sites, given different assumed degrees of knowledge about the destinations (distance to the destination, D; distance plus the provisioning treatment at the destination, DP; distance, provisioning treatment and elapsed time, DPT). In the simplest model (H0 in Janson, 2016a), no values for distance, productivity, or elapsed time are used, so the modeled choices reflect any overall preference to visit a particular feeding site, ignoring other explanations. Given a cognitive model (D, DP, or DPT), the expected movement sequences can be used to calculate the expected food reward at each destination site, along with expected travel and feeding times. These foraging parameters, derived from the movements observed during the field experiment reported in Janson (2016a), are used here to calculate the relative feeding efficiency of hypothetical monkeys using different degrees of ecological knowledge of their feeding sites. The original choice data and associated metrics are available on the Dryad repository (Janson, 2016b).
Computer Simulations
All programming is performed in Pascal (Turbo Pascal 7.0; Borland Software Corp, implemented for Windows using Lazarus). Initially, the program mimics as closely as possible the constraints and general parameters of the wild capuchin study. This is done both to test the model—does it provide answers roughly similar to the observed experimental results—and to incorporate a set of realistic biological constraints that might affect the relative benefit of using more complex cognitive information in spatial foraging decisions. Specifically, I allow to vary:
1) The total number of food patches per home range (keeping the density of food patches the same by scaling the size of the home range in proportion to the number of food patches). This is set initially to eight, the number of platforms in the experiments, and the home range is set to 800 × 800 m, a reasonable approximation to the 900 × 700 m area in which the feeding sites were set up (Janson, 2016a).
2) The maximum and minimum productivity per patch. To maintain the setup similar to the experiment, I allow only two levels of productivity. The low level is half the high level. For convenience, I set the saturation level for the high treatment in the simulation equal to 2 and the low treatment equal to 1, as these units are arbitrary (all similar units, like gut capacity, are scaled in the same way). To convert results from the simulations into equivalent food intake in the experiment, each unit equals 24 banana pieces.
3) The fraction of all patches that are “low” vs. “high” productivity. Initially this value is set to 0.5, as in the experiment.
4) The renewal (ripening) period. As in the experiment, this is set initially to 48 h.
5) Group-level ingestion rate per hour is set initially to 12 (= 6 high-value patches/h), so that it takes the group 10 min to empty a full high-value patch; this value was close to that observed in the experiments (minutes to empty a feeding site = 1 + 0.19*(N banana pieces), P < 0.001). Time spent per visit to a site equaled the time spent feeding on the platforms (depending on the food available) plus a “clean up” time of 10 min, during which the group picked up scraps below the platforms and prepared to leave the site.
6) The gut capacity of the forager. Initially this is set to twice the value of the maximum patch productivity. This value is based on the observation that after a night of fasting, the monkeys in the field slowed their ingestion rate following the cumulative ingestion of about 100 banana pieces, which is close to the equivalent of 2 high-productivity feeding sites.
7) Gut passage time (average time for an ingested food item to pass through the digestive system) is initially set to 2 h, this being the value for capuchin monkeys in this population (Wehncke and Dominguez, 2007). Together with gut capacity, passage time dictates the rate of food digestive processing = (gut capacity)/(gut transit time), which in turn limits the long-term rate of food intake, even if ingestion rate in a patch could be much higher (see parameter 5).
8) Maximum ingestion of food (from patches) per day is set initially to 12 units, equating to 6 full high-productivity patches. In practice, the monkeys during the field experiments reported in Janson (2016a) could only ingest an average of 6 units per day, as this was the experimentally-determined productivity of the feeding sites. However, from other experiments, we know the monkeys can consume substantially more than 6 units per day.
9) Travel speed between sites was set to its average value of 15 m/min, although this value varied in a complex way with the expected reward at the destination site (higher rewards provoked faster travel), distance to the destination (the group traveled faster toward closer sites, probably because of increased scramble competition), and cumulative food ingested (the more banana pieces they had ingested on a given day, the slower their travel speed to the next site).
10) I initially set the active feeding period per day to 5 h (7 a.m. to noon) to mirror the fact that the group typically switched from feeding on banana pieces in the morning to foraging for insects in the afternoon.
The general rationale behind the choice of the variable parameters (1–8) is that each one constrains to some degree the ease of finding high-productivity patches (parameters 1–4) or the maximum benefit of feeding in a patch (parameters 5–8). I would expect that any parameter change that makes it easier to find high-value patches, or that reduces the relative value of feeding in a high-value patch, will reduce the relative benefit of using more complex cognitive information in patch choice. The code for the simulation is given in Supplementary Materials.
Results
Field Experiments
I use three multinomial logistic regressions (see Janson, 2016a) using observed movement choices between provisioned feeding sites to fit the effects on site choice of (1) food site distance alone (D), (2) distance plus provisioning treatment (DP), and (3) distance, provisioning treatment, and elapsed time (DPT). Each regression model is then used to predict the most likely feeding site as the next destination for each of the 212 site choices in the experiment. Because we control the amount of food provided at each feeding site according to provision treatment and elapsed time, I calculate the expected food reward at each predicted (most likely) feeding site in the three regressions. To convert expected reward and distance to more conventional measures of foraging efficiency (energy per time), I use the facts that feeding time increases predictably with the amount eaten at each site (Methods), and that travel speed between sites does not depend predictably on the particular pair of sites chosen but only on the distance between them (Janson, 2016a). Thus, for each regression I calculate measures of expected food intake per time, a measure of efficiency commonly assumed to align with fitness (Stephens and Krebs, 1986); patterns for food intake per distance were nearly identical to food intake per time. Relative to the models based on the use of increasing amounts of ecological cognition (D, DP, DPT), I provide measures of foraging efficiency from a “random” choice of destination sites based only the observed preferences to visit particular feeding sites overall (H0 in Janson, 2016a). While some sites were preferred over others “all else being equal,” these differences in baseline preference were not large enough to override the statistical effects of distance, productivity or time delay on site choice (Janson, 2016a). The comparison of foraging efficiencies under the distinct levels of assumed cognitive information show that the use of any information about destination site traits, even distance alone, provides much greater (at least twice) foraging efficiency relative to ignoring such information (Figure 1: “random” choice). Foraging efficiency is modestly higher (13%) when choices are based on all three types of cognitive information (DPT), relative to D or DP. Interestingly, the use of DP without elapsed time produces a slightly lower overall expected foraging efficiency than D alone. Inspection of the expected choices made under DP shows that sometimes more distant “high” treatment sites are preferred choices even when they had been used relatively recently, so that realized food intake is as low or lower than would have occurred if the choice were for one of the closer “low” treatment sites chosen using distance alone.
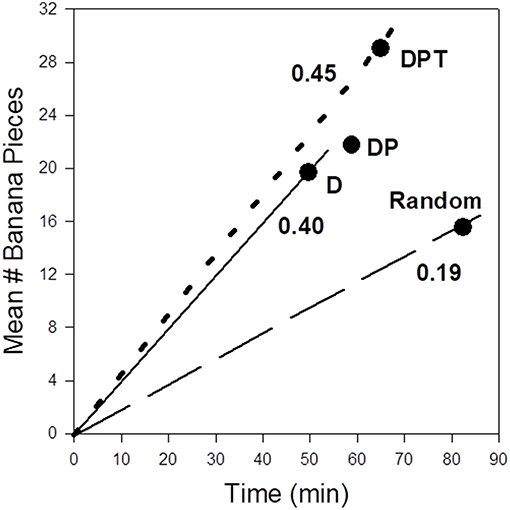
Figure 1. Expected foraging efficiencies (food intake vs. time spent in travel plus feeding per site visited) as a function of ecological cognition during field experiments in wild capuchin monkeys in Argentina (Janson, 2016a). The points correspond to foraging consequences of making the most profitable site choices based on different criteria: D, distance (nearest sites always preferred); DP, distance and provisioning (preferred sites have the highest ratio of the fixed provisioning treatment, divided by distance); DPT, distance plus provisioning and elapsed time since the previous visit to that site (preferred sties have the highest ratio of expected provisioning, based on provisioning treatment and elapsed time since the monkeys left a site, to distance). The lines for D and DP are virtually identical, so only one is drawn. The actual behavior of the monkeys conformed best to DPT. “Random” refers to foraging efficiency if all sites except the current visited site are chosen based on inferred site-specific preferences independent of productivity or distance (Methods).
Computer Simulations
The baseline simulation uses parameters very close to observed values in the experiment (Methods). The simulated E/T values are reasonably similar to those in the field experiment (Figure 1); the observed E/T values are 0.3–1.65 standard deviations lower than the simulated values (Table 1). The differences between simulated and experimental results are likely due to the spatial distribution of the simulated sites, which are randomly placed within the range (as opposed to rather evenly distributed as in the experiment). In the simulations, forager using D or DP choices sometimes become “trapped” in local clusters of feeding sites, using these repeatedly and inefficiently to the exclusion of other sites (see also Anderson, 1983). As in the results from the field experiment, the foraging efficiency using DP is slightly lower than using D alone. Indeed, with few exceptions, E/T for simulated foragers using DP is nearly identical to or less than for using only D (Table 1).
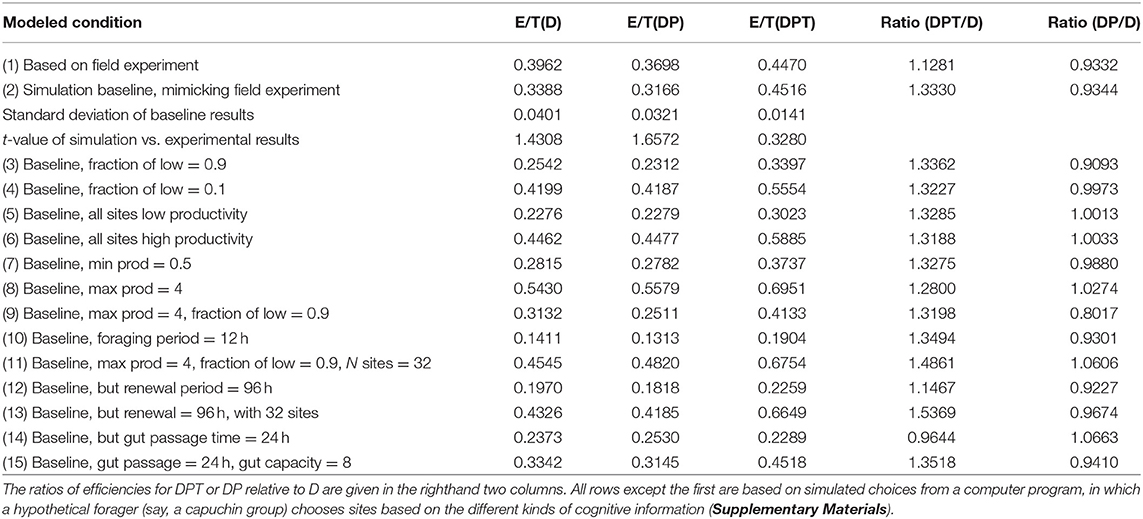
Table 1. Foraging efficiency (E/T, where E, food intake; T, time spent traveling and feeding per site visit) under different site choices based on distance alone (D), distance and site productivity (DP), or distance, productivity and elapsed time (DPT).
The ratio of E/T when simulating the use of DPT vs. D is remarkably stable near 1.3–1.35 under broadly varying conditions of minimum or maximum feeding site productivity, the fraction of sites that are high vs. low productivity sites, and the duration of foraging, as long as the number of feeding sites is kept at the baseline number of 8 (Table 1: conditions 2–10). Indeed, the substantial advantage of using DPT vs. D remains even when P does not vary among the sites (all sites are low or high: conditions 5, 6). This last result points out that the benefit of incorporating elapsed time is primarily due to the fact that resources renew, not to variance in productivity (P) among resources.
The relative benefit of DPT vs. D varies from the baseline value under only some conditions. First, DPT performs even better than D when the number of feeding sites increases to 12 (all other parameters being equal), although the benefit wanes at even higher numbers of resources (Figure 2). Inspection of the simulation progress showed that DPT foragers take advantage of the greater number of high-productivity sites to mostly avoid use of low-productivity sites altogether. Second, the relative benefit of DPT vs. D drops slightly to 1.15 when each site renews over 96 h instead of the baseline 48 h (Table 1: 12). This result may appear paradoxical, as the longer renewal period would seem to favor paying more attention to time (waiting even longer produces larger rewards). However, the lower rate of renewal (only 3 new units of food per day) means that feeding success of all foragers is strongly constrained by total production, regardless of cognitive strategy. This interpretation is supported by the marked increase in the relative performance of DPT over D when the same 96-h renewal is coupled with a 4-fold increase in site numbers to 32 (Table 1: 13).
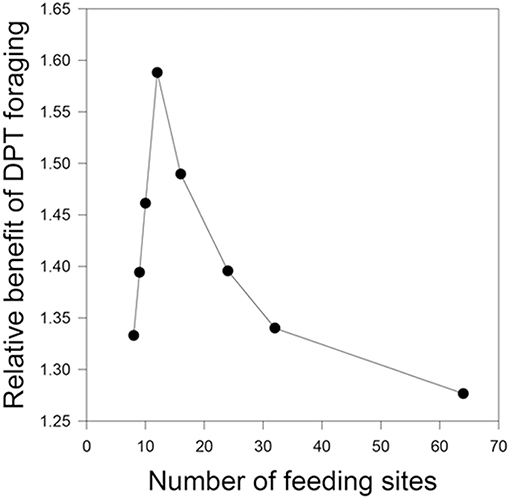
Figure 2. Relative benefit of time-sensitive foraging decisions as a function of the number of resources per home range. The baseline condition (N = 8, as in the field experiments) does not provide enough food to satiate the group during the day, so both time-insensitive and time-sensitive foragers are limited by the rate of production of the food patches. As the number of patches increases, time-sensitive foragers increase their relative food intake by focusing choices on the sites not recently used. When patches are very common, most patches are not recently used and are at maximum accumulated production; in this case, the relative benefits of paying attention to elapsed time diminish.
Second, DPT actually performed worse than D (Table 1: 14) when gut passage time is increased from 2 h (typical of capuchin monkeys) to 24 h (typical of many folivores and some cercopithecine monkeys: Lambert, 2002). This decrease occurs because slow gut passage means that a forager would typically arrive at a patch already partly full and thus cannot consume all of the greater amount of food in a patch accumulated by delaying the visit. This gut passage constraint can be offset by having a larger gut capacity; increasing gut capacity from the baseline of 4 to 8 restores the relative benefit of DPT over D (Table 1: 15).
Discussion
The results provided here suggest that integrating elapsed time since a prior visit to each food patch can often markedly increase the foraging efficiency of a primate (or any patch-foraging animal) that uses renewing food sites (Figure 1). Conditions that favor using elapsed time to choose among renewing food sites are that food should be plentiful enough (either a high enough number of sites, or sufficiently productive sites, or both: Table 1), but not so abundant that even simple strategies that use only distance can perform reasonably well (Figure 2). The relative benefit of using elapsed time in spatial choice of feeding sites is notable even when all sites have the same productivity (Table 1: 5 & 6)—as long as the forager leaves a site depleted of food and it recovers over time, it is worth tracking elapsed time at each site. This result suggests that perception and memory of elapsed time since the last visit to a given site may prove to be far more common than we currently understand—in principle, animals foraging on any renewing resource (e.g., grass) could benefit by tracking where they have been and avoiding short-term revisits to depleted areas (cf. Cody, 1971).
For foragers that rely on discrete feeding sites, using information on patch productivity without including elapsed time may not be very worthwhile. Simulated foragers using DP information rarely do markedly better than those using only D (only 2 of 14 simulated conditions in Table 1). The relatively poor performance of DP foragers might seem paradoxical, since they are using more information in making foraging choices. However, the use of DP criteria in site choice necessarily implies choosing sites with higher expected average productivity that are farther away than the closest feeding site (otherwise the choice is identical to using D alone). If these chosen high-productivity sites have recently been visited, however, their actual accumulated food may be less than closer low-productivity sites, so that the forager gains nothing for the cost of extra travel to the site. Thus, we should not generally expect foragers to use information on site productivity without also tracking elapsed time since they visited each site. Simulated foragers using DP did notably better than those using D in only two situations. First is when there are several high-quality patches and many low-quality patches (Table 1: 11). In this case, when animals can forage efficiently on only high-quality patches, they should largely ignore low-quality ones—DP modestly outperformed D, although DPT outperformed both. It may be that such situations are common, so that DP might be a logical evolutionary stepping stone between D and DPT. Second is when gut passage is very slow (Table 1: 14), when DP outperforms both D and DPT. This result depends rather sensitively on limited gut capacity, as the outcome reverts to the “usual” pattern when gut capacity is increased only modestly (Table 1: 15). Additional simulations across a broader range of parameters may reveal other cases in which DPT performs poorly relative to simpler strategies.
One strong caveat to these results is that the calculation based both on field experiments and on computer simulations are done without the presence of competitors that might visit the same food patches as the focal foragers. Competitors may reduce a feeding site to zero food at some time during that patch's “recovery” interval, as perceived by a resident forager. Such interruptions of recovery, especially if not predictable, can seriously degrade the value of using the DPT strategy relative to D, as the resident forager may choose to travel further to reach what should be a more valuable patch (Figure 1), only to find that it is far less valuable than expected. The author's preliminary results from such simulated intrusions suggests that DPT remains a better strategy than D at low frequencies of competition, but intrusions at high rates can reduce the value of DPT to make it perform no better or even more poorly than using only D information. Thus, we may expect that foragers using renewing patches should incorporate elapsed time especially for resources to which they have “private” access (few competitors exist), resources for which intrusions are not likely to make much difference in food accumulation (“super-productive” sites: Janson, 1988), or they may expend considerable effort to monitor and expel intruders from “their” array of renewing feeding sites (e.g., hummingbirds: Gill, 1988).
An additional deep assumption of the current simulations is patch permanence. If patches have short productive periods or change either production levels or renewal rates quickly over time, foragers would have difficulty tracking these values. In such a case, memory for past values might be a poor guide for future foraging decisions. As in the case of intruders entering a home range, resident foragers might expend extra effort to visit more distant patches that are expected to be more productive, but find that these had less reward than anticipated. Such “mistakes” might be mitigated by strategies in which foragers learn aspects of species-level ripening synchrony (Janmaat et al., 2011), predictable effects of weather on ripening rate (Janmaat et al., 2006a) or other predictable clues to varying patch quality. If patches disappear quickly or vary unpredictably through time, any cognitive foraging strategy (D, DP, or DPT) would have limited use.
Notwithstanding the foregoing limitations, the results of this study suggest that under many conditions, tracking elapsed time since a food patch was last used will benefit foragers, if the patch renews predictably with time. Factors that limit a forager's ability to harvest accumulated productivity (slow renewal rate, low numbers of feeding sites, limited gut capacity, slow gut emptying) will also limit the amount of benefit from time-sensitive foraging. Perhaps surprisingly, the benefit does not depend on variation in productivity between patches—even when all patches have identical productivities and renewal rates, the forager's history of feeding at distinct patches generates enough standing variation in accumulated food among patches to favor using elapsed time to choose the next feeding patch. In light of these results, it is not surprising that evidence for time-sensitive foraging decisions has been found in well-controlled studies of diverse vertebrates (Zinkivskay et al., 2009; review: Crystal, 2010) including a spectrum of ecological specializations including cache-making granivores (e.g., Clayton and Dickinson, 1999), nectarivores (González-Gómez et al., 2011), frugivores (Janmaat et al., 2014; Janson, 2016a), and generalists (Crystal, 2010). Given that the benefit of time-sensitive foraging seems to be robust to considerable variation in patch and forager parameters, it is worth extending the search for time-sensitive foraging to species that are less obvious candidates—folivores (where leaf patches may be depleted: Snaith and Chapman, 2005), grazers (where the patches may be particular fields or openings of renewing vegetation), fish (especially those that live in stable home ranges with limited foraging locations), and large mobile predators (whose presence may behaviorally depress prey availability in a local area even if few prey are actually killed). The practical and conceptual challenges of documenting time sensitive foraging (review: Crystal, 2010) should not prevent us from discovering how widespread this capacity is among animals.
Ethics Statement
The field experiment cited in this study was performed under IACUC approval from the University of Montana: 040-07CJDBS-120507.
Author Contributions
The author confirms being the sole contributor of this work and has approved it for publication.
Conflict of Interest Statement
The author declares that the research was conducted in the absence of any commercial or financial relationships that could be construed as a potential conflict of interest.
Acknowledgments
The field experiment thatmotivated this analysis was done by the author with the expert collaboration of Maria Celia Baldovino and Maria Paula Tujague, as well as the stalwart assistance in the field of Ilan Kolkowitz, Annie Green, Julia Monk and Flor Espinosa.
Supplementary Material
The Supplementary Material for this article can be found online at: https://www.frontiersin.org/articles/10.3389/fevo.2019.00125/full#supplementary-material
References
Anderson, J. D. (1983). Optimal foraging and the traveling salesman. Theo. Pop. Biol. 24, 145–159. doi: 10.1016/0040-5809(83)90038-2
Antinucci, F., and Visalberghi, E. (1986). Tool use in Cebus apella: a case study. Int. J. Primatol. 7, 351–363. doi: 10.1007/BF02693700
Bicca-Marques, J. C., and Garber, P. A. (2004). Use of spatial, visual, and olfactory information during foraging in wild nocturnal and diurnal anthropoids: a field experiment comparing Aotus, Callicebus, and Saguinus. Am. J. Primatol. 62, 171–187. doi: 10.1002/ajp.20014
Boesch, C., and Boesch, H. (1984). Mental map in wild chimpanzees: an analysis of hammer transports for nut cracking. Primates 25, 160–170. doi: 10.1007/BF02382388
Clayton, N. S., and Dickinson, A. (1999). Scrub jays (Aphelocoma coerulescens) remember the relative time of caching as well as the location and content of their caches. J. Comp. Psychol. 113, 403–416. doi: 10.1037/0735-7036.113.4.403
Cody, M. L. (1971). Finch flocks in the Mohave Desert. Theor. Pop. Biol. 2, 142–148. doi: 10.1016/0040-5809(71)90012-8
Cramer, A. E., and Gallistel, C. R. (1997). Vervet monkeys as travelling salesmen. Nature 387, 464–464. doi: 10.1038/387464a0
Crystal, J. D. (2010). Episodic-like memory in animals. Behav. Brain Res. 215, 235–243. doi: 10.1016/j.bbr.2010.03.005
Cunningham, E. P., and Janson, C. H. (2007). Integrating information about location and value of resources by white faced-saki monkeys (Pithecia pithecia). Anim. Cogn. 10, 293–304. doi: 10.1007/s10071-007-0077-4
Gallistel, C. R., and Cramer, A. E. (1996). Computations on metric maps in mammals: getting oriented and choosing a multi-destination route. J. Exper. Biol. 199, 211–217.
Garber, P. A. (1988). Foraging decisions during nectar-feeding by tamarin monkeys (Saguinus mystax and Saguinus fuscicollis). Biotropica 20, 100–106. doi: 10.2307/2388181
Garber, P. A. (1989). Role of spatial memory in primate foraging patterns: Saguinus mystax and Saguinus fuscicollis. Am. J. Primatol. 19, 203–216. doi: 10.1002/ajp.1350190403
Gill, F. B. (1988). Trapline foraging in hermit hummingbirds: competition for an undefended renewing resource. Ecology 69, 1933–1942. doi: 10.2307/1941170
González-Gómez, P. L., Bozinovic, F., and Vásquez, R. A. (2011). Elements of episodic-like memory in free-living hummingbirds, energetic consequences. Anim. Behav. 81, 1257–1262. doi: 10.1016/j.anbehav.2011.03.014
Holekamp, K. E., Dantzer, B., Stricker, G., Shaw Yoshida, K. C., and Benson-Amram, S. (2015). Brains, brawn and sociality: a hyaena's tale. Anim. Behav. 103:237e248. doi: 10.1016/j.anbehav.2015.01.023
Isler, K., and Van Schaik, C. P. (2006). Metabolic costs of brain size evolution. Biol. Lett. 22, 557–560. doi: 10.1098/rsbl.2006.0538
Janmaat, K. R. L., Byrne, R. W., and Zuberbühler, K. (2006a). Evidence for a spatial memory of fruiting states of rainforest trees in wild mangabeys. Anim. Behav. 72, 797–807. doi: 10.1016/j.anbehav.2005.12.009
Janmaat, K. R. L., Byrne, R. W., and Zuberbühler, K. (2006b). Primates take weather into account when searching for fruit. Curr. Biol. 16, 1232–1237. doi: 10.1016/j.cub.2006.04.031
Janmaat, K. R. L., Chapman, C. A., Meijer, R., and Zuberbühler, K. (2011). The use of fruiting synchrony by foraging mangabey monkeys: a ‘simple tool’ to find fruit. Anim. Cogn. 15, 83–96. doi: 10.1007/s10071-011-0435-0
Janmaat, K. R. L., Polansky, L., Ban, S. D., and Boesch, C. (2014). Wild chimpanzees plan their breakfast time, type, and location. Proc. Natl. Acad. Sci. U.S.A. 111, 16343–16348. doi: 10.1073/pnas.1407524111
Janson, C. H. (1988). Food competition in brown capuchin monkeys (Cebus apella): quantitative effects of group size and tree productivity. Behaviour 105, 53–76. doi: 10.1163/156853988X00449
Janson, C. H. (1996). “Toward an experimental socioecology of primates: examples from Argentine brown capuchin monkeys (Cebus apella nigritus),” in Adaptive Radiations of Neotropical Primates, eds M. Norconk, P. Garber, and A. Rosenberger (New York, NY: Plenum Press), 309–325.
Janson, C. H. (1998). Experimental evidence for spatial memory in foraging wild capuchin monkeys, Cebus apella. Anim. Behav. 55, 1229–1243. doi: 10.1006/anbe.1997.0688
Janson, C. H. (2007). Experimental evidence for route integration and strategic planning in wild capuchin monkeys. Anim. Cogn. 10, 341–356. doi: 10.1007/s10071-007-0079-2
Janson, C. H. (2014). Death of the (traveling) salesman: primates do not show clear evidence of multi-step route planning. Am. J. Primatol. 76, 410–420. doi: 10.1002/ajp.22186
Janson, C. H. (2016a). Capuchins, space, time and memory: an experimental test of what-where-when memory in wild monkeys. Proc. R. Soc. B 283:20161432 doi: 10.1098/rspb.2016.1432
Janson, C. H. (2016b). Data from: Capuchins, space, time and memory: an experimental test of what-where-when memory in wild monkeys. Dryad. doi: 10.5061/dryad.sm47b
Lambert, J. E. (2002). Digestive retention times in forest guenons (Cercopithecus spp.) with reference to chimpanzees (Pan troglodytes). Int. J. Primatol. 23, 1169–1185. doi: 10.1023/A:1021166502098
MacDonald, S. E., and Wilkie, D. M. (1990). Yellow-nosed monkeys' (Ceropithecus ascanius whitesidei) spatial memory in a simulated foraging environment. J. Comp. Psych. 104, 382–387. doi: 10.1037/0735-7036.104.4.382
Martin, R. D. (1981). Relative brain size and basal metabolic rate in terrestrial vertebrates. Nature 293, 57–60. doi: 10.1038/293057a0
Menzel, C. R. (1991). Cognitive aspects of foraging in Japanese monkeys. Anim. Behav. 41, 397–402. doi: 10.1016/S0003-3472(05)80840-1
Menzel, E. W. (1978). “Cognitive mapping in chimps,” in Cognitive Processes in Animal Behavior, eds S. Hulse, H. Fowler, and W. K. Honigs (Hillsdale, NJ: L. Erlbaum Associates), 375–422.
Menzel, E. W. Jr. (1973). Chimpanzee spatial memory organization. Science 182, 943–945. doi: 10.1126/science.182.4115.943
Milton, K. (1981). Distribution patterns of tropical food plants as an evolutionary stimulus to primate mental development. Am. Anthropol. 83, 534–548. doi: 10.1525/aa.1981.83.3.02a00020
Noser, R., and Byrne, R. W. (2007). Mental maps in chacma baboons (Papio ursinus) using intergroup encounters as a natural experiment. Anim. Cogn. 10, 331–340. doi: 10.1007/s10071-006-0068-x
Reader, S. K., and Laland, K. N. (2002). Social intelligence, innovation, and enhanced brain size in primates. PNAS 99, 4436–4441. doi: 10.1073/pnas.062041299
Seyfarth, R. M., and Cheney, D. L. (2012). “Knowledge of social relations,” in Evolution of Primate Societies, eds J. M. Mitani, J. Call, P. M. Kappeler, R. A. Palombit, and J. B. Silk (Chicago, IL: University of Chicago Press), 628–642
Silk, J. B., Alberts, S. C., and Altmann, J. (2003). Social bonds of female baboons enhance infant survival. Science 302, 1231–1234. doi: 10.1126/science.1088580
Snaith, T. V., and Chapman, C. A. (2005). Towards an ecological solution to the folivore paradox: patch depletion as an indicator of within-group scramble competition in red colobus monkeys (Piliocolobus tephrosceles). Behav. Ecol. Sociobiol. 59, 185–190. doi: 10.1007/s00265-005-0023-x
Sol, D. (2009). Revisiting the cognitive buffer hypothesis for the evolution of large brains. Biol. Lett. 5, 130–133. doi: 10.1098/rsbl.2008.0621
Stephens, D. W., and Krebs, J. R. (1986). Foraging Theory. Princeton, NJ: Princeton University Press.
Teichroeb, J. A. (2015). Vervet monkeys use paths consistent with context-specific spatial movement heuristics. Ecol. Evol. 5, 4706–4716. doi: 10.1002/ece3.1755
Trapanese, C., Meunier, H., and Masi, S. (2018). What, where and when: spatial foraging decisions in primates. Biol. Rev. 94, 483–502. doi: 10.1111/brv.12462
Tujague, M. P., and Janson, C. H. (2017). Wild capuchin monkeys anticipate the amount of ripe fruit in natural trees. Anim. Cogn. 20, 841–853. doi: 10.1007/s10071-017-1105-7
Wascher, C. A. F., Kulahci, I. G., Langley, E. J. G., and Shaw, R. (2018). How does cognition shape social relationships? Phil. Trans. R. Soc. B 373:20170293. doi: 10.1098/rstb.2017.0293
Wehncke, E. V., and Dominguez, C. A. (2007). Seed disperal ecology of non-restricted frugivores, capuchin monkeys in three neotropical forests. J. Trop. Ecol. 23, 519–528. doi: 10.1017/S0266467407004257
Keywords: foraging efficiency, ecological cognition, frugivory, primates, field experiments, computer models
Citation: Janson CH (2019) Foraging Benefits of Ecological Cognition in Fruit-Eating Primates: Results From Field Experiments and Computer Simulations. Front. Ecol. Evol. 7:125. doi: 10.3389/fevo.2019.00125
Received: 30 September 2018; Accepted: 28 March 2019;
Published: 18 April 2019.
Edited by:
Deborah M. Gordon, Stanford University, United StatesReviewed by:
Amanda Dawn Melin, University of Calgary, CanadaFernando Campos, University of Texas at San Antonio, United States
Copyright © 2019 Janson. This is an open-access article distributed under the terms of the Creative Commons Attribution License (CC BY). The use, distribution or reproduction in other forums is permitted, provided the original author(s) and the copyright owner(s) are credited and that the original publication in this journal is cited, in accordance with accepted academic practice. No use, distribution or reproduction is permitted which does not comply with these terms.
*Correspondence: Charles H. Janson, Y2hhcmxlcy5qYW5zb25AbXNvLnVtdC5lZHU=