- 1National Centre for Biological Sciences, Tata Institute of Fundamental Research, Bengaluru, India
- 2School of Biological Sciences, Illinois State University, Normal, IL, United States
- 3SASTRA University, Tirumalaisamudram, Thanjavur, India
- 4Centre for Ecological Sciences, Indian Institute of Science, Bengaluru, India
Non-lethal, trait-mediated effects of predation impact prey behavior and life-history traits. Studying how these effects in turn influence prey demography is crucial to understand prey life-history evolution. Mosquitoes are important vectors that claim several million lives every year worldwide by transmitting a range of pathogens. Several ecological factors affect life-history traits of both larval and adult mosquitoes, creating effects that cascade to population-level consequences. Few studies have comprehensively explored the non-lethal effects of predation and its interactions with resources and competition on larval, adult, and population traits of mosquitoes. Understanding these interactions is important because the effects of predation are hypothesized to rescue prey populations from the effects of density-dependence resulting from larval competition. Aedes aegypti larvae reared at two different larval densities and subjected to three non-lethal predator treatments were monitored for survival, development time, and adult size through the larval stages to adult eclosion, and adult females were monitored for survival and reproduction through their first gonotrophic cycle. Intraspecific competition increased larval development time, yielded small-bodied adults, and reduced fecundity in individuals exposed to predatory chemical cues as larvae. Exposure to cues from a living predator affected both body size and latency to blood feed in females. Analysis of life-table traits revealed significant effects of competition on net reproductive rate (R0) of mosquitoes. The interaction between competition and predator treatments significantly affected the cohort rate of increase (r) and the index of performance (r’). The index of performance, which estimates rate of population change based on the size-fecundity relationship, was significantly and positively correlated with r, but overestimated r slightly. Lack of significant effect of predator treatments and larval density on cohort generation time (Tc) further suggests that the observed effects of treatments on r and r’ were largely a consequence of the effects on R0. Also, the significant effects of treatment combinations on larval development time, adult body size and fecundity were ultimately manifested as effects on life-table traits estimated from adult survival and reproduction.
Introduction
Apart from being killed (lethal effect) prey individuals are influenced by predators in several other ways that are characterized as intimidation (McCauley et al., 2011). This “non-lethal” aspect of predation is best explained as a product of selection due to predation causing adaptive evolution of prey, favoring changes in life-history, morphology and behavior (Lima, 1998). Many of these adaptive changes can increase prey fitness when predation is likely, but also cost prey energy and opportunity to invest time in seeking resources such as food and mates (Abrams, 2000; Barbosa and Castellanos, 2005). The costs of these responses to the threat of predation become evident when they are induced in the absence of risk of prey death.
The consequences of lethal and non-lethal aspects of the predator-prey interaction, both direct and indirect, cascade from individuals to ecosystems (Lima, 1998). Both lethal and non-lethal predation influence prey, albeit through different mechanisms: density-mediated lethal effects result when predators reduce prey numbers by killing or consumption and trait-mediated non-lethal effects, on the other hand, result from costs associated with anti-predatory defenses that affect individual prey fitness (Peacor and Werner, 2001; Preisser et al., 2005; Bellamy and Alto, 2018). While predation effects are conventionally thought to depend most on lethality, a meta-analysis has shown that non-lethal effects of predation on prey can outweigh the consequences arising from prey lethality (Preisser et al., 2005; Cresswell, 2008). Non-lethal predation effects on prey behavior, morphology, ontogeny and physiology are quite well studied but only few studies have identified how indirect, trait-mediated non-lethal effects of predators impact prey demography (Zajac, 1995; Nelson et al., 2004; Preisser and Bolnick, 2008; Alto et al., 2012). Such demographic effects are important as they inform about how the threat of predation may impact population dynamics. From an applied perspective, such knowledge would enable us to foresee consequences of biological control strategies involving use of predators as enemies to control pests (Bellamy and Alto, 2018). However, these studies do not account for the interactive effects of non-lethal predation threat with other ecological factors that affect population dynamics, such as resource availability and intraspecific competition. Taking these interactions into consideration is particularly important because the effects of predation threat are hypothesized to rescue populations from the negative effects of density-dependence resulting from larval competition, and may even increase population size via “Hydra effect” (Abrams, 2009; McIntire and Juliano, 2018). Hydra effect manifests when predator-induced mortality or behavioral alterations in prey release over-exploited resources thereby increasing their resource productivity (Abrams, 2009).
Mosquitoes are collectively the most important vectors of human pathogens, posing serious health threats across the globe by transmitting pathogens causing diseases such as dengue, yellow fever, zika, chikungunya, several types of encephalitis, malaria, and filariasis (Gubler, 1998; Lounibos, 2002). As organisms with complex life cycles, mosquitoes inhabit aquatic and terrestrial environments as larvae and adults, respectively. Multiple ecological factors, both biotic and abiotic, affect the physiology (e.g., stored glycogen, protein and lipid reserves, starvation resistance), morphology (e.g., wing length, adult body mass), behavior (e.g., movement, space use preferences) and life-history traits (e.g., age at maturity, fecundity, longevity) of larvae and adults (Agnew et al., 2000; Costanzo et al., 2011; van Uitregt et al., 2012). These life-history traits strongly affect population rate of increase and individual fitness (Preisser and Bolnick, 2008; Noden et al., 2016). Such population-level consequences of ecological interactions are likely to affect transmission rates of mosquito-borne pathogens (Muturi et al., 2011; Moller-Jacobs et al., 2014; Bara et al., 2015).
Quantifying population-level consequences of mosquito cohorts from a full life-table (i.e., cohort rate of increase, r, and related life-table statistics) is particularly challenging because of the practical difficulties associated with following the reproductive phases of individual mosquitoes through their adult life (Juliano, 1998; Chmielewski et al., 2010). To circumvent the difficulties of quantifying r, Livdahl and Sugihara (1984) proposed the composite index of performance (r’) that estimates per capita growth rates of experimental cohorts. The composite index of performance (r’) takes measures of life-history traits that are expected to be correlates of r, such as adult female body size and days of emergence (from egg hatch to eclosion) and a fecundity-body size regression, to calculate r’ which is an approximation of r (Livdahl and Sugihara, 1984). This index eliminates the need for quantifying fecundity and survival through the lifespan of individual females and therefore is widely preferred by experimental ecologists to summarize demographics of insect populations (Dennis and Costantino, 1988; Livdahl and Willey, 1991; Paradise and Dunson, 1997; McPeek and Peckarsky, 1998; Armbruster and Hutchinson, 2002; Griswold and Lounibos, 2005; Alto et al., 2012; McIntire and Juliano, 2018).
The interactions between mosquito larvae and their predators and the ensuing changes in adult life-history traits have been extensively studied. Mosquito larvae sense predation threat using chemical cues originating from either the predator or the act of predation referred to as “kairomone” and “alarm cue,” respectively. While these cues have not been chemically characterized, studies have identified the relative importance of each of these in eliciting anti-predatory behavior in mosquito larvae (Ferrari et al., 2007, 2008; Kesavaraju and Juliano, 2010; Roberts, 2014). These predatory cues are either derived from dietary metabolites in predator feces post prey consumption or prey mechanically damaged in the process of predator attack (Chivers and Smith, 1998; Kesavaraju and Juliano, 2004), and in some cases the solid residues of predation (e.g., uneaten body parts, feces) have been implicated as the main active component of alarm cues (Kesavaraju and Juliano, 2010). Some species respond behaviorally to water borne cues from the act of predation but not to a non-feeding predator (Kesavaraju and Juliano, 2010). A recent study has, however, identified that physical cues when paired with chemical cues aid mosquito larvae in better assessing the risk of predation (Chandrasegaran et al., 2017). Some species show greater behavioral response to more concentrated cues from predation (Kesavaraju et al., 2007), and some can learn to associate novel predator kairomones with prey alarm cues (Ferrari et al., 2008). Multiple studies have shown that mechanically crushed prey larvae elicit prey behavioral and life-history responses that are similar to alarm cues produced by predators actively killing prey (Costanzo et al., 2011; Roberts, 2014; Chandrasegaran et al., 2018), and this observation has led to the use of crushed prey to simulate the alarm cues coming from predator-killed prey (McIntire and Juliano, 2018).
Predation threat, in the short term, impacts larval behavior (Ellis and Borden, 1970; Juliano and Gravel, 2002; Kesavaraju and Juliano, 2004) and, in the long term, affects larval-to-adult survivorship (Fox, 1975; Beketov and Liess, 2007), larval development time (Hechtel and Juliano, 1997; Agnew et al., 2000), larval growth and adult size (Hechtel and Juliano, 1997). Long-term consequences of predation risk of larvae on adult traits have frequently been studied by assessing changes in adult body size (Hechtel and Juliano, 1997; Costanzo et al., 2011; van Uitregt et al., 2012). Variation in adult body size is associated with variation in stored teneral reserves of carbohydrate, protein and lipid, which could directly impact adult resistance to starvation (Barrera, 1996), flight and swarming behavior (Van Handel, 1984; Yuval et al., 1994), and host-seeking behavior (Nasci, 1986). Altered reserves may also affect blood feeding behavior (Nasci, 1986; Scott and Takken, 2012), biting habits (Nasci, 1991), reproductive strategy and fecundity (Takken et al., 1998; Agnew et al., 2002; Mostowy and Foster, 2004; Ponlawat and Harrington, 2009; Maïga et al., 2014) and pathogen transmission potential (Scott et al., 2000). Adult longevity (Roux et al., 2015) and oviposition site selection preferences (Kiflawi et al., 2003; Bond et al., 2005) are other adult traits that can be affected by threat of predation during the larval stages. The effects of other ecological factors such as intra- and inter-specific competition (Noden et al., 2016), nutrition stress (Beketov and Liess, 2007), and desiccation risk (Sota and Mogi, 1992) on larval and adult life-history traits of mosquitoes have also been extensively studied.
This study of the yellow fever mosquito, Aedes aegypti, is a follow-up to experiments reported by Chandrasegaran et al. (2018). Specifically, we test for how the interactive effects of intraspecific competition and non-lethal predation threat experienced by developing larvae may affect demographic traits of adult mosquitoes that determine population dynamics. Mosquitoes were exposed to non-lethal predation during the larval stages using either chemical cues in isolation or in combination with physical cues from a live predator. Chandrasegaran et al. (2018) tested for interactive effects of nutrition availability, intraspecific competition for resources and non-lethal predation threat during larval stages on adult life-history traits. They identified subtle, context-dependent effects of non-lethal predation threat impacting larvae on individual traits such as adult male and female longevity and adult body composition of males. The observed effects on female longevity may be particularly important as longevity can be a strong determinant of both demography and vectorial capacity (Reiskind and Lounibos, 2009). To further our investigation, we comprehensively explored the interactive effects of non-lethal predation threat on individual and population-level traits of mosquito prey. We constructed a life-table using larval and adult traits quantified by following cohorts of mosquitoes through their larval stages to completion of the first gonotrophic cycle. We further assessed the effects of non-lethal threat of predation on rate of increase for cohorts of mosquitoes using: (1) A life-table approach quantifying fecundity measured in the first gonotrophic cycle and estimating cohort rate of increase (r) and (2) The composite index approach pioneered by Livdahl and Sugihara (1984) using first gonotrophic cycle fecundity predicted from average female wing length (r’). Using these results, we further analyzed if r’ is an adequate surrogate for r. Our overall goal is to assess how initial larval density and predation cues influenced life-table traits of adult mosquitoes, and ultimately affected population rate of increase. This study is among the few attempts to investigate the long-term consequences of non-lethal predation threat and its interactions by synthesizing many different life-history traits into an estimate of population dynamics.
Materials and Methods
Eggs of the yellow fever mosquito (Aedes aegypti) from a colony originating from New Orleans LA, USA, were hatched in deionized (DI) water. Freshly hatched larvae were reared in cylindrical white plastic containers in 30% dilution of nutrient medium prepared by adding 1 g of freshly powdered mixture of dog biscuit (Ol' Roy, Doane pet food, Brentwood TN, USA: 24% protein, 11% lipid, 57% carbohydrate by dry weight) and yeast (3:2) per 300 ml of DI water. Within each container was a predator enclosure consisting of a 3.5 cm diameter PVC pipe with the submerged end sealed with two layers of nylon mesh (0.6 and 0.3 mm openings) on the inside and outside, respectively. The mesh prevented mosquito larvae from entering or leaving the enclosure. The outer 0.3 mm mesh was removed after all larvae in the container reached the 3rd instar. The experiment included 2 levels of intraspecific competition among larvae (high and low, which were 78 and 26 newly hatched larvae, respectively) and 3 non-lethal predator threat treatments as described in detail by Chandrasegaran et al. (2018): live predation (chemical + physical cues), simulated predation (chemical cues only), and control. For live predation, a 3rd or 4th instar Toxorhynchites rutilus larva was added to the predator enclosure and offered ten 4th instar A. aegypti larvae daily. For simulated predation, we determined the mean number of A. aegypti larvae eaten by T. rutilus in the live predation enclosures on a given day and then added that number of 4th instar A. aegypti larvae, crushed with forceps, to each simulated predation enclosure daily. Costanzo et al. (2011) showed that crushed prey larvae were capable of eliciting responses similar to that produced by real predation. In controls, nothing was added into the predator enclosure. The experiment was setup with 6 replicate cohorts per treatment combination and development of mosquito larvae until eclosion was monitored as described by Chandrasegaran et al. (2018). In this study, however, we chose not to include the amount of larval nutrition as an experimental factor as fewer females survived to eclosion in two out of three nutrition treatments when faced with high larval competition in Chandrasegaran et al. (2018). At adult eclosion, female mosquitoes were grouped together in cages (0.7 L plastic containers 11.59 cm in diameter and 10.48 cm tall with a 0.6 mm nylon mesh on top) based on treatment combination and replicate identity and were provided with 20% sugar solution for 5 days. On days 6 and 7, only water was provided as sugar deprivation increases likelihood of female mosquitoes seeking a blood meal (Galal, 2017). Individual cages were placed inside a larger wooden cage lined with an electric heating pad to maintain ambient temperature (32°C) during the blood feeding procedure. An anesthetized mouse was placed on the nylon mesh top of each cage for 15–20 min for the mosquitoes to blood feed. Females that took blood meals were aspirated and confined individually in 15 ml glass vials, where they were provided with 20% sugar solution for 7 days and were then killed by freezing. Frozen females were then dissected to count Christophers Stage IV eggs in ovaries and to measure wing length. Ovary dissection was performed in Hanks' Balanced Salt Solution—HBSS 1X. Egg count data on mosquitoes that took a blood meal but died within 7 days were also included in this study. Females that died before blood feeding were used for wing measurement only.
Females that did not take a blood meal in a given attempt were provided with 20% sugar solution throughout the subsequent day and then provided with only DI water on the following day. They were then offered another blood meal on the third day. This procedure was repeated until the last mosquito alive in every cohort took a blood meal. The numbers of mosquitoes that took a blood meal during every blood feeding attempt was recorded and analyzed for treatment effects.
Estimation of Life-Table Traits and Statistical Analysis
We tested for effects of intraspecific competition, predator treatments, and their interaction on larval-adult transition traits such as median development time (hatching to eclosion) and proportion of females surviving to adulthood (assuming a 1:1 sex ratio at hatching), adult traits such as mean body size (mean of wing length measured from anal lobe to wing tip to the nearest 0.1 mm), proportion of females surviving to blood feed, mean number of feeding bouts required for all females to take a blood meal and mean fecundity in the first gonotrophic cycle (with wing length as covariate). We also tested for the same effects on life-table traits of mosquito cohorts i.e., net reproductive rate (R0), cohort generation time (Tc) and cohort rate of increase (r) as dependent variables.
Data on individual fecundity from first gonotrophic cycle and other adult traits were used to construct a life-table and to calculate the net reproductive rate (R0), cohort per capita rate of increase (r), and cohort generation time (Tc) (Noden et al., 2016), where
and
The cohort rate of increase (r) was estimated by solving the Euler-Lotka equation using the iterative solver add-in in MS Excel 2013.
In these equations, x denotes time from hatching to egg production in days, lx is age (x) specific survival, i.e., proportion of adult females surviving on day x, and mx is the fecundity of adult mosquitoes, i.e., mean number of mature eggs in dissected females on day x. For the initial estimate of r for the iterative solution, we used the approximation of r (Livdahl and Sugihara, 1984):
The index of performance (r’) developed by (Livdahl and Sugihara, 1984) was also estimated for the mosquito cohorts using female mean wing length to predict fecundity.
In the expression for r’, N0 denotes the initial density of females in the cohort (assumed as 50% of the cohort), Ax denotes the number of adult females eclosing on day x, wx is a measure of the mean size of females eclosing on day x, f (wx) is a function relating production of female eggs (multiplied by ½ assuming a 1:1 sex ratio in the dissected eggs) to female size (wing length in mm), and D is the time between adult emergence and reproduction. Axand wxwere estimated for each replicate container within each treatment. D was estimated to be 14 days based on the protocol (7 days post eclosion and prior to blood feeding + 7 days prior to ovary dissection procedure). The slopes for regression [f (wx)] relating egg count in individual females to their body size (wing length in mm) at eclosion did not differ significantly among the six treatment combinations (F5, 524 = 1.75, P = 0.121, Supplementary Material and Figure 1). Therefore, the regression [f (wx)] calculated for individual mosquitoes across all cohorts put together is:
All traits quantified on individual larval and adult mosquitoes, except for the egg count vs. body size regression, were averaged at the level of individual experiment containers which are also the units of replication. We used ANOVA to test for the effects of intraspecific competition, predator treatments, and their interaction on all dependent variables. Significant ANOVA effects were further analyzed using pairwise comparisons of means, with a Bonferroni adjustment for multiple tests. Pearson's correlation tests the strength of association of r’ and r. All assumptions of the tests were met. We used R version 3.4.2 (R Development Core Team, 2016) or SAS software, version 9.4 (SAS Institute Inc, 2012) to perform all analyses.
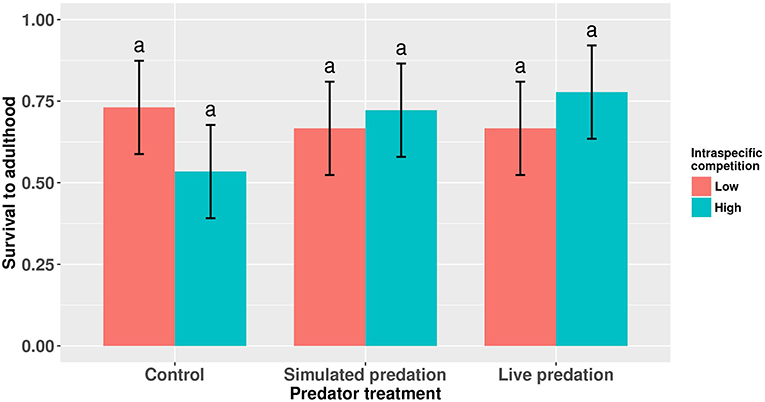
Figure 1. Proportion survivorship to eclosion of female Aedes aegypti larvae exposed to intraspecific competition and predator treatments. Values plotted are least squares means ±95% confidence intervals (CI). Data were statistically tested using ANOVA and significant effects are described in Table 1. Treatment means associated with the same letters are not significantly different in pairwise comparisons using Bonferroni adjustment for multiple tests.
Results
Larvae—Adult Transition
Survival of female Aedes aegypti larvae to adulthood was not affected by intraspecific competition and predation treatments (Table 1, Figure 1). Female A. aegypti larvae developed significantly more slowly when reared at elevated density of conspecifics, but neither the effect of predation treatment nor the interaction was significant (Table 2, Figure 2).
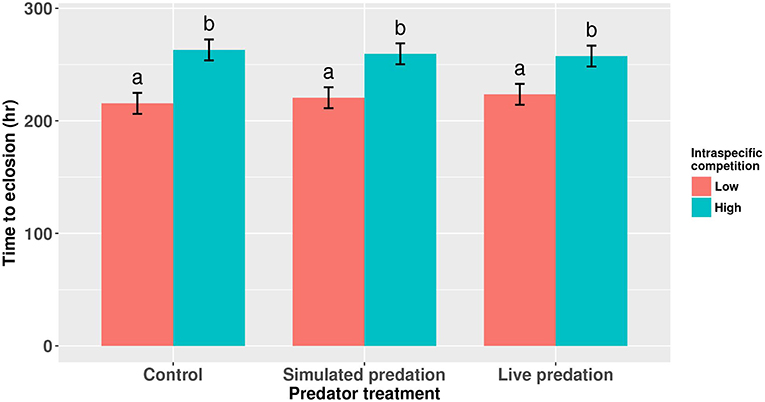
Figure 2. Development time of cohorts of female Aedes aegypti larvae exposed to intraspecific competition and predator treatments. Values plotted are least squares means ±95% CI. Data were statistically tested using ANOVA and significant effects are described in Table 2. Treatment means associated with the same letters are not significantly different in pairwise comparisons using Bonferroni adjustment for multiple tests.
Adult Traits
Elevated larval competition resulted in small-sized adult females (Table 3, Figure 3A). While simulated predation and live predator treatments had a significant positive effect on this measure of body size, the observed pairwise differences were not statistically significant (Table 3, Figure 3B). Predation and competition during the larval stages did not affect survival of adults between eclosion and first bout of blood feeding (Table 4, Figure 4). Adults from larvae exposed to live predator treatment required significantly fewer attempts before successful blood feeding compared to controls (Table 5, Figure 5). Fecundity in the first gonotrophic cycle was affected by predator treatments and its interaction with larval competition (Table 6, Figure 6). In “high” larval density, larvae exposed to simulated predation and live predation treatments produced significantly fewer eggs than controls as adult mosquitoes (Table 6; Figure 6). Whereas, in “low” larval density, only simulated predation treatment had a negative effect on adult fecundity (Table 6, Figure 6).
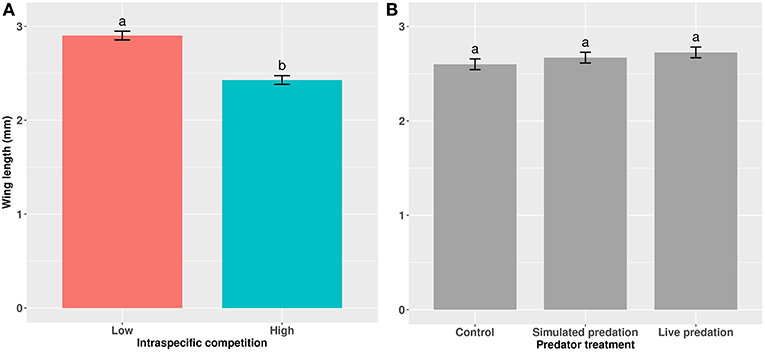
Figure 3. Wing length of cohorts of female Aedes aegypti exposed to (A) intraspecific competition and (B) predator treatments as larvae. Values plotted are least squares means ±95% CI. Data were statistically tested using ANOVA and significant effects are described in Table 3. Treatment means within (A) and within (B) associated with the same letters are not significantly different in pairwise comparisons using Bonferroni adjustment for multiple tests.
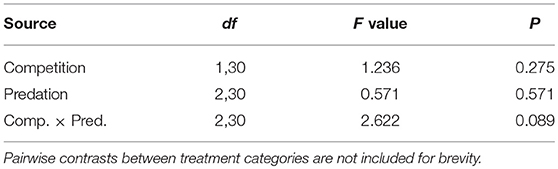
Table 4. ANOVA results for proportion of eclosed adult female Aedes aegypti surviving to take a blood meal.
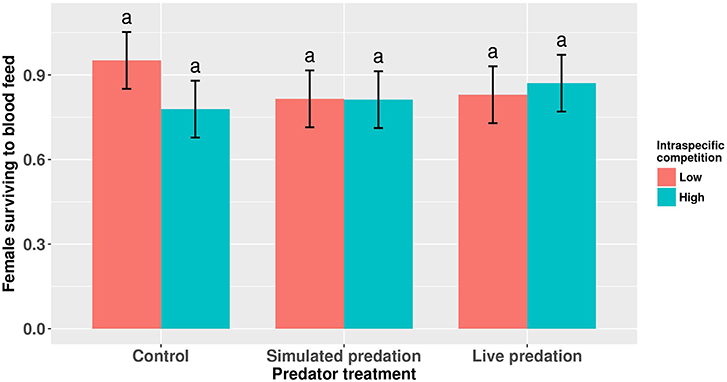
Figure 4. Proportion of eclosed adult female Aedes aegypti surviving to take a blood meal. Values plotted are least squares means ±95% CI. Data were statistically tested using ANOVA and significant effects are described in Table 4. Treatment means associated with the same letters are not significantly different in pairwise comparisons using Bonferroni adjustment for multiple tests.
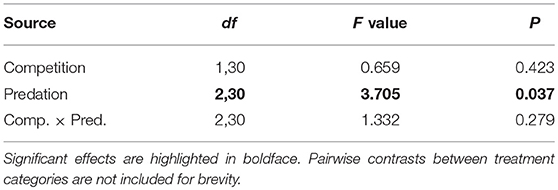
Table 5. ANOVA results for number of blood feeding bouts required for female Aedes aegypti to successfully take a blood meal.
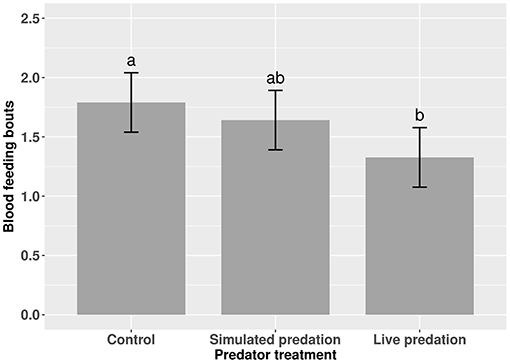
Figure 5. Number of blood feeding bouts required for adult female Aedes aegypti to successfully take a blood meal. Values plotted are least squares means ±95% CI. Data were statistically tested using ANOVA and significant effects are described in Table 5. Treatment means associated with the same letters are not significantly different in pairwise comparisons using Bonferroni adjustment for multiple tests.
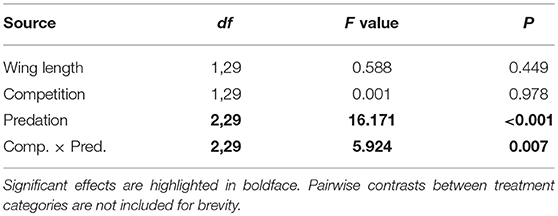
Table 6. ANOVA results for number of eggs produced by Aedes aegypti in first gonotrophic cycle with wing length as the covariate.
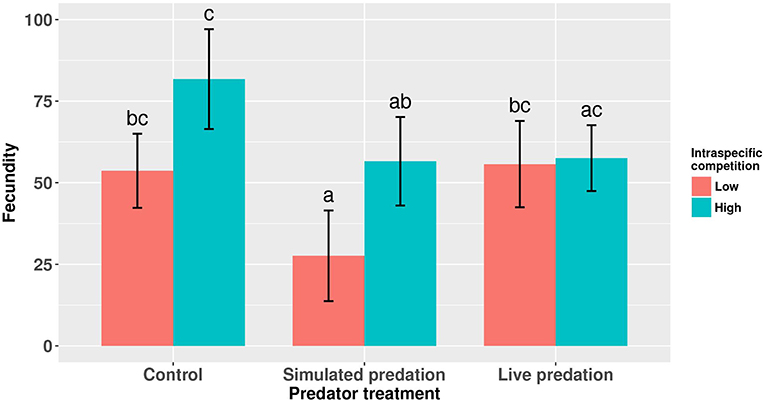
Figure 6. Number of eggs produced by adult female Aedes aegypti in first gonotrophic cycle. Values plotted are least squares means ±95% CI. Data were statistically tested using ANOVA and significant effects are described in Table 6. Treatment means associated with the same letters are not significantly different in pairwise comparisons using Bonferroni adjustment for multiple tests.
Life-Table Parameters
Elevated intraspecific larval competition lowered net reproductive value (Ro), cohort rate of increase (r) and index of performance (r’) (Table 7). The interaction of competition and predation treatments had significant effects on r and r’ (Table 7). Particularly, elevated competition reduced r in simulated predation treatment and r’ in control treatment respectively. No experiment variable affected cohort generation time (Tc) and importantly, predation treatment as a main effect did not affect any of the life-table traits (Table 7, Figure 7). The index of performance, r’ was significantly and positively correlated with per capita rate of increase estimated from the full life-table (Pearson correlation coefficient: 0.83, P < 0.001; Figure 8). The performance index tended to slightly overestimate r (Figure 8). For the same experiment, statistical conclusions about effects on r and r’ were qualitatively similar, yielding significant effects of intraspecific larval competition and of the interaction of competition and predation treatment (Table 7).

Table 7. ANOVA results for life table traits of Aedes aegypti such as cohort generation time (Tc), net reproductive rate (R0), cohort rate of increase (r) and index of population performance (r’).
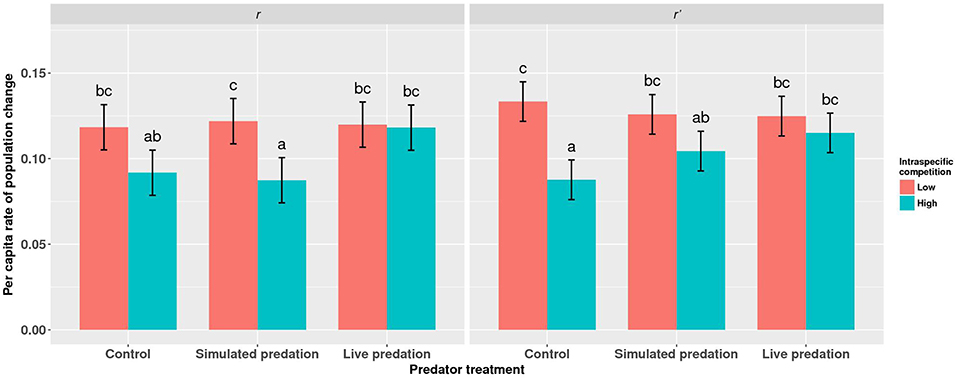
Figure 7. Least squares means (±95% CI) for per capita rate of population change (r) for cohorts of Aedes aegypti exposed to intraspecific competition and predation treatments as larvae. Data were statistically tested using a two-way ANOVA and results are summarized in Table 7. Treatment means associated with the same letters are not significantly different in pairwise comparisons using Bonferroni adjustment for multiple tests.
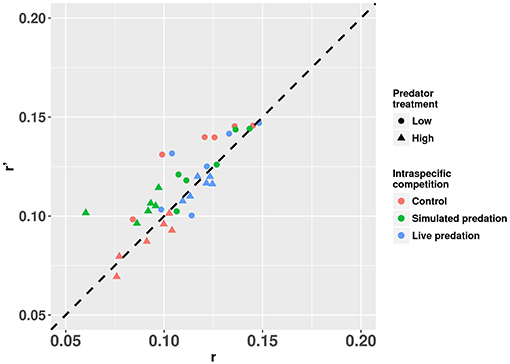
Figure 8. Estimates of r’ (projected per capita rate of population change) plotted against r (per capita rate of population change estimated from the full life table) for cohorts of Aedes aegypti exposed to intraspecific competition and predation treatments. Values plotted are least squares means. The dotted 45° line through the origin represents the perfect concurrence between r’ and r. Values below and above the line represent underestimation and overestimation, respectively.
Discussion
Our results suggest that intraspecific competition and non-lethal effects of predation experienced by larvae affect some larval and adult life-history traits but not others. We quantified these effects by following cohorts of mosquitoes through their larval and adult stages until the first gonotrophic cycle. The purpose of this study was to assess comprehensively the interactive effects of predation threat and population density on life-table traits. We further evaluated how well per capita population rate of change is estimated by the index of performance of Livdahl and Sugihara (1984) (r and r′, respectively). We use these results to discuss how individual life-history traits shaped by ecological influences translate into population-level consequences.
Life-table analysis revealed effects of larval competition on R0 (net reproductive rate), r (cohort rate of increase) and r’ (composite index of performance). Further analysis revealed that elevated competition lowered r’ and r in mosquito cohorts exposed to control and simulated predation treatment, respectively. Elevated intraspecific competition increased larval development time, resulted in relatively small-sized adults and specifically yielded less fecund individuals in simulated predation treatment. Live predator treatment affected both body size and propensity to blood feed in surviving individuals. Despite these predator effects on some adult traits, the influences on life-table traits, particularly r and r’, were products of the interaction of larval competition with predator treatment. The effects of predator treatment on adult traits were context-dependent on intraspecific competition. The interactive effect of predator treatments on fecundity was relatively more pronounced in larvae subjected to “high” intraspecific competition but had no effect on R0 and Tc. Cohort generation time Tc was insensitive to any of the independent variables in the analysis. For R0, predation and its interaction with larval competition had no significant effect, despite the effect on fecundity. It seems likely that inherent heterogeneity amongst individual mosquitoes within cohorts exposed to similar experiment treatments obscures the effects of larval rearing conditions on R0 and Tc (Noden et al., 2016). This heterogeneity is a ramification of the variability in larval and adult traits further accentuated by stringent larval environments (Edgerly and Livdahl, 1992; Lord, 1998; Juliano, 2009) (Figures 5–7). This suggests that the performance of a few females within a cohort dominate R0 and Tc and may compensate for low fecundity of other individuals severely enfeebled by larval competition and predation. Also, the lack of effect of competition on adult fecundity and r in the live predation treatment is an indication of predation rescuing mosquito cohorts from the negative effects of competition via “Hydra effect”. Further investigation is however needed to conclude if predatory cues are contributing to this effect via prudent resource exploitation (Abrams, 2009).
For r the cohort rate of increase, we detected a significant effect of the interaction between competition and predation treatments, despite the lack of such interactive effects on R0 and Tc. This result provides evidence that if the goal is to determine effects of environment on demography, surrogates such as individual fitness correlates and approaches that only examine lifetime fecundity (R0) or generation time (Tc) provide an incomplete picture of population dynamics, which is best described by the cohort's instantaneous rate of increase (r). In this experiment R0 appears to be a better indicator of r than Tc, but we have no reason to think this result will be general. The important conclusion, in our view, is that r is the best indicator of environmental effects on population dynamics because it incorporates effects of both lifetime reproductive output (R0), and generation time (Tc) (Livdahl and Sugihara, 1984; Siraj et al., 2017).
Behaviorally, mosquito larvae are known to exhibit stronger antipredatory responses to a combination of physical and chemical cues than to chemical cues in isolation (Chandrasegaran et al., 2017). On the contrary, this study identified chemical cues to negatively affect adult fecundity and the cohort rate of increase r in isolation (simulated predation treatment) but not in combination with physical cues from a live predator (live predation treatment). This is probably an indication of the effects of predation rescuing mosquito cohorts from the negative effects of intraspecific competition via “Hydra effect.” As live predator treatment did not affect larval survival to adulthood (Table 1, Figure 1) and predators in the treatment were restricted from culling prey, it is unlikely that predator-induced mortality would have produced the hypothesized effect. Therefore, further investigation is needed to conclude if chemical and physical cues together are contributing to the hydra effect via prudent resource exploitation resulting from altered larval behavior (Abrams, 2009). The effects of simulated and live predator treatments on mosquito biology and demography lead us to another conclusion that non-lethal effects of predators are strongly context-dependent on population density (intraspecific competition). Furthermore, these effects are not uniformly detectable across the many different fitness correlates that may be quantified in an experimental setting.
Environmental effects on larval and adult traits might also influence the reproductive strategy of adult females upon eclosion from challenging conditions. Plastic responses of individuals to physiological challenges could either result in delay of reproduction or terminal investment in reproduction (Stone et al., 2011; Contreras-Garduño et al., 2014). Along those lines, a recent study has discussed how individuals from high-density and competitive larval environments use sugar and blood meals to nourish themselves and make up for the effects of crowding that they suffered as larvae (Noden et al., 2016). Our experimental procedures limited us to considering only the effects of our manipulations on population dynamics of cohorts constrained to a single blood meal. In an experiment using a similar life-table analysis, Noden et al. (2016) followed females through as many blood meals and gonotrophic cycles as they could complete. We have exercised enough caution in drawing the above inferences as our results on population-level traits are entirely based on fecundity estimates for first gonotrophic cycle quantified by dissecting ovaries from females after one blood meal without mates. Though this is a frequently-used method to quantify fecundity in mosquitoes, we may not have captured the full effects of experiment variables on blood-feeding and life-table traits of mosquito cohorts as in a comprehensive full life-table approach (Armbruster and Hutchinson, 2002). Noden et al. (2016) suggested that a few very long lived females could exert a large influence on cohort rate of increase r, hence our approach only provides an approximation of the effects of the larval environment on population dynamics. Similarly, the lack of any significant effect of experiment variables on Tc may be a result of us limiting females to one gonotrophic cycle.
Our comparison between estimates of r and r’ for mosquito cohorts suggests that r’ not only consistently correlated with r but also slightly overestimated r across treatment categories (Figure 8). The greater values of r’ compared to r are likely result of the assumption in calculating r’ that all females that reach adulthood will mate, blood feed, and lay eggs (Livdahl and Sugihara, 1984). In the real life-table calculation, some females may fail to blood feed, and some may die before developing eggs, which would lower r calculated from the life-table. Our approach to the life-table calculation of r is likely to result in an underestimate of r because we did not allow any females to lay >1 batch of eggs, and this contrasts with the approach used by Noden et al. (2016) and Chmielewski et al. (2010). Despite this difference in our studies, the over estimation of r by r’ and the strong positive correlation between the two is consistent with the conclusions of Chmielewski et al. (2010). Further, our results comparing r and r’ support the conclusion of Chmielewski et al. (2010) who suggested that r’ overestimates r primarily because the calculation of r’ assumes that females reproduce only once, whereas in real life-tables females may reproduce multiple times. In our calculation of r, we dissected females after one reproductive bout, in marked contrast to the approach taken by Chmielewski et al. (2010), and Noden et al. (2016), both of whom allowed females to reproduce multiple times. Chmielewski et al. (2010) found a greater magnitude of overestimation of r by r’ than that observed in our study [compare our Figure 8 to Chmielewski et al. (2010) Figure 1]. Thus, our r’ and r are based on similar assumptions and constraints on reproduction and the smaller difference between the two estimates suggests that r’ only slightly overestimates r when the latter is also constrained to allow only one reproductive bout. An encouraging observation for experimentalists is that despite r’ being a slight overestimate of r, the statistical conclusions about which factors significantly affected these measures of performance were very similar. Thus, as concluded by Chmielewski et al. (2010), the simplifying assumptions built into r’ still enable it to be an easily measured but consistent index of population performance and a suitable surrogate for r.
In conclusion, predation threat and competition interacted to affect both individual and predicted population-level performance of Aedes aegypti. This study, to the best of our knowledge, is the most thorough assessment of the population-level consequences of non-consumptive effects of predation and its interactions acting in the lives of larval mosquitoes. The results of this study highlight the importance of understanding the different pathways by which non-consumptive trait-mediated effects structure predator-prey interactions and population growth in mosquitoes (Preisser and Bolnick, 2008; Alto et al., 2012). Furthermore, it is intriguing to note how experiment variables affecting larval development time, adult body size and fecundity amongst other larval and adult traits end up influencing population-level traits. Exploring the mechanisms that trigger metamorphosis, govern larval development time and regulate body size at eclosion in response to biotic and abiotic factors is an important research direction for the future. As A. aegypti, and other mosquitoes are important vectors of disease, information on how varying larval environments impact vectorial capacity together with the findings of this study will be indispensable in furthering our knowledge on the dynamics of mosquito-borne diseases.
Data Availability Statement
All data files generated for this study are publicly available in figshare digital repository. URL: https://figshare.com/projects/Chandrasegaran_Juliano_-_Frontiers_in_Ecology_Evolution/59129.
Ethics Statement
This study was carried out in accordance with the principles of the Basel Declaration and recommendations of US National Institutes of Health Guide for the Care and Use of Laboratory Animals 8th edition. The protocol (IACUC #842043) was approved by the Illinois State University Institutional Animal Care and Use Committee.
Author Contributions
KC and SJ were equally involved in conception and design of this study, performing statistical analysis and writing the manuscript. KC conducted all experiments.
Funding
This research was supported by: Fulbright-Nehru Doctoral Research Fellowship 2014-15 from the US-India Educational Foundation, New Delhi (India) and the Institute of International Education, New York (USA); 2015 Patel Grant sponsored by the Institute of International Education; R.D.Wiegel Phi Sigma Research Grant from the School of Biological Sciences, Illinois State University all to KC; and by National Institute of Health grants 1R15AI094322-01A1 and 1R15AI124005-01 to SJ.
Conflict of Interest Statement
The authors declare that the research was conducted in the absence of any commercial or financial relationships that could be construed as a potential conflict of interest.
Acknowledgments
We thank S. Quader for inputs on conception of this study, standardization of experiment methods and comments on this manuscript; B.M. Sadd, S.K. Sakaluk and V.A. Borowicz for useful discussion, and access to reagents and laboratory equipment; K. Westby for assistance with ovary dissection procedure; G.D. Ower for help with maintenance of mosquito colonies; School of Biological Sciences, Illinois State University, Normal, Illinois (USA) for providing infrastructure.
Supplementary Material
The Supplementary Material for this article can be found online at: https://www.frontiersin.org/articles/10.3389/fevo.2019.00025/full#supplementary-material
References
Abrams, P. A. (2000). The evolution of predator-prey interactions: theory and evidence. Annu. Rev. Ecol. Syst. 31, 79–105. doi: 10.1146/annurev.ecolsys.31.1.79
Abrams, P. A. (2009). When does greater mortality increase population size? The long history and diverse mechanisms underlying the hydra effect. Ecol. Lett. 12, 462–474. doi: 10.1111/j.1461-0248.2009.01282.x
Agnew, P., Haussy, C., and Michalakis, Y. (2000). Effects of density and larval competition on selected life history traits of Culex pipiens quinquefasciatus (Diptera: Culicidae). J. Med. Entomol. 37, 732–735. doi: 10.1603/0022-2585-37.5.732
Agnew, P., Hide, M., Sidobre, C., and Michalakis, Y. (2002). A minimalist approach to the effects of density-dependent competition on insect life-history traits. Ecol. Entomol. 27, 396–402. doi: 10.1046/j.1365-2311.2002.00430.x
Alto, B. W., Malicoate, J., Elliott, S. M., and Taylor, J. (2012). Demographic consequences of predators on prey: Trait and density mediated effects on mosquito larvae in containers. PLoS ONE 7:e45785. doi: 10.1371/journal.pone.0045785
Armbruster, P., and Hutchinson, R. A. (2002). Pupal mass and wing length as indicators of fecundity in Aedes albopictus and Aedes geniculatus (Diptera: Culicidae). J. Med. Entomol. 39, 699–704. doi: 10.1603/0022-2585-39.4.699
Bara, J., Rapti, Z., Cáceres, C. E., and Muturi, E. J. (2015). Effect of larval competition on extrinsic incubation period and vectorial capacity of Aedes albopictus for dengue virus. PLOS ONE 10:e0126703. doi: 10.1371/journal.pone.0126703
Barbosa, P., and Castellanos, I. (2005). Ecology of Predator-Prey Interactions. New York, NY: Oxford University Press.
Barrera, R. (1996). Competition and resistance to starvation in larvae of container-inhabiting Aedes mosquitoes. Ecol. Entomol. 21, 117–127. doi: 10.1111/j.1365-2311.1996.tb01178.x
Beketov, M. A., and Liess, M. (2007). Predation risk perception and food scarcity induce alterations of life-cycle traits of the mosquito Culex pipiens. Ecol. Entomol. 32, 405–410. doi: 10.1111/j.1365-2311.2007.00889.x
Bellamy, S. K., and Alto, B. W. (2018). Mosquito responses to trait- and density-mediated interactions of predation. Oecologia 187, 1–11. doi: 10.1007/s00442-018-4107-5
Bond, J. G., Arredondo-Jiménez, J. I., Rodriguez, M. H., Quiroz-Martínez, H., and Williams, T. (2005). Oviposition habitat selection for a predator refuge and food source in a mosquito. Ecol. Entomol. 30, 255–263. doi: 10.1111/j.0307-6946.2005.00704.x
Chandrasegaran, K., Kandregula, S. R., Quader, S., and Juliano, S. A. (2018). Context-dependent interactive effects of non-lethal predation on larvae impact adult longevity and body composition. PLOS ONE 13:e0192104. doi: 10.1371/journal.pone.0192104
Chandrasegaran, K., Singh, A., Laha, M., and Quader, S. (2017). Playing it safe? Behavioural responses of mosquito larvae encountering a fish predator. Ethol. Ecol. Evol. 30, 70–87. doi: 10.1080/03949370.2017.1313785
Chivers, D. P., and Smith, R. J. F. (1998). Chemical alarm signalling in aquatic predator-prey systems: a review and prospectus. Ecoscience 5, 338–352. doi: 10.1080/11956860.1998.11682471
Chmielewski, M. W., Khatchikian, C., and Livdahl, T. (2010). Estimating the per capita rate of population change: How well do life-history surrogates perform? Ann. Entomol. Soc. Am. 103, 734–741. doi: 10.1603/AN09162
Contreras-Garduño, J., Rodríguez, M. C., Rodríguez, M. H., Alvarado-Delgado, A., and Lanz-Mendoza, H. (2014). Cost of immune priming within generations: Trade-off between infection and reproduction. Microbes Infect. 16, 261–267. doi: 10.1016/j.micinf.2013.11.010
Costanzo, K. S., Muturi, E. J., and Alto, B. W. (2011). Trait-mediated effects of predation across life-history stages in container mosquitoes. Ecol. Entomol. 36, 605–615. doi: 10.1111/j.1365-2311.2011.01302.x
Cresswell, W. (2008). Non-lethal effects of predation in birds. Ibis 150, 3–17. doi: 10.1111/j.1474-919X.2007.00793.x
Dennis, B., and Costantino, R. F. (1988). Analysis of steady-state populations with the gamma abundance model: application to Tribolium. Ecology 69, 1200–1213. doi: 10.2307/1941275
Edgerly, J. S., and Livdahl, T. P. (1992). Density-dependent interactions within a complex life cycle: the roles of cohort structure and mode of recruitment. J. Anim. Ecol. 61, 139–150. doi: 10.2307/5517
Ellis, R., and Borden, J. (1970). Predation by Notonecta undulata (Heteroptera: Notonectidae) on larvae of the yellow-fever mosquito. Ann. Entomol. Soc. Am. 63, 963–973. doi: 10.1093/aesa/63.4.963
Ferrari, M. C., Messier, F., and Chivers, D. P. (2008). Threat-sensitive learning of predators by larval mosquitoes Culex restuans. Behav. Ecol. Sociobiol. 62, 1079–1083. doi: 10.1007/s00265-007-0535-7
Ferrari, M. C. O., Messier, F., and Chivers, D. P. (2007). Variable predation risk and the dynamic nature of mosquito antipredator responses to chemical alarm cues. Chemoecology 17, 223–229. doi: 10.1007/s00049-007-0380-1
Fox, L. R. (1975). Factors influencing cannibalism, a mechanism of population limitation in the predator Notonecta hoffmanni. Ecology 56, 933–941. doi: 10.2307/1936303
Galal, F. H. (2017). Effect of sugar deprivation and host species on the blood-feeding of Culex (Culex) univittatus mosquito. J. Asia-Pac. Entomol. 20, 1387–1395. doi: 10.1016/j.aspen.2017.08.013
Griswold, M. W., and Lounibos, L. P. (2005). Competitive outcomes of aquatic container diptera depend on predation and resource levels. Ann. Entomol. Soc. Am. 98, 673–681. doi: 10.1603/0013-8746(2005)098[0673:COOACD]2.0.CO;2
Gubler, D. (1998). Resurgent vector-borne diseases as a global health problem. Emerg. Infect. Dis. 4, 442–450. doi: 10.3201/eid0403.980326
Hechtel, L. J., and Juliano, S. A. (1997). Effects of a predator on prey metamorphosis: plastic responses by prey or selective mortality? Ecology 78, 838–851. doi: 10.1890/0012-9658(1997)078[0838:EOAPOP]2.0.CO;2
Juliano, S. A. (1998). Species introduction and replacement among mosquitoes: interspecific resource competition or apparent competition? Ecology 79, 255–268. doi: 10.1890/0012-9658(1998)079[0255:SIARAM]2.0.CO;2
Juliano, S. A. (2009). Species interactions among larval mosquitoes: context dependence across habitat gradients. Annu. Rev. Entomol. 54, 37–56. doi: 10.1146/annurev.ento.54.110807.090611
Juliano, S. A., and Gravel, M. E. (2002). Predation and the evolution of prey behavior: an experiment with tree hole mosquitoes. Behav. Ecol. 13, 301–311. doi: 10.1093/beheco/13.3.301
Kesavaraju, B., Damal, K., and Juliano, S. A. (2007). Threat-sensitive behavioral responses to concentrations of water-borne cues from predation. Ethology 113, 199–206. doi: 10.1111/j.1439-0310.2006.01317.x
Kesavaraju, B., and Juliano, S. A. (2004). Differential behavioral responses to water-borne cues to predation in two container-dwelling mosquitoes. Ann. Entomol. Soc. Am. 97, 194–201. doi: 10.1603/0013-8746(2004)097[0194:DBRTWC]2.0.CO;2
Kesavaraju, B., and Juliano, S. A. (2010). Nature of predation risk cues in container systems: mosquito responses to solid residues from predation. Ann. Entomol. Soc. Am. 103, 1038–1045. doi: 10.1603/AN10007
Kiflawi, M., Blaustein, L., and Mangel, M. (2003). Oviposition habitat selection by the mosquito Culiseta longiareolata in response to risk of predation and conspecific larval density. Ecol. Entomol. 28, 168–173. doi: 10.1046/j.1365-2311.2003.00505.x
Lima, S. L. (1998). Nonlethal effects in the ecology of predator-prey interactions. BioScience 48, 25–34. doi: 10.2307/1313225
Livdahl, T., and Willey, M. (1991). Prospects for an invasion: competition between Aedes albopictus and native Aedes triseriatus. Science 253, 189–191. doi: 10.1126/science.1853204
Livdahl, T. P., and Sugihara, G. (1984). Non-linear interactions of populations and the importance of estimating per capita rates of change. J. Anim. Ecol. 53, 573–580. doi: 10.2307/4535
Lord, C. C. (1998). Density dependence in larval Aedes albopictus (Diptera: Culicidae). J. Med. Entomol. 35, 825–829. doi: 10.1093/jmedent/35.5.825
Lounibos, L. P. (2002). Invasions by insect vectors of human disease. Annu. Rev. Entomol. 47, 233–266. doi: 10.1146/annurev.ento.47.091201.145206
Maïga, H., Niang, A., Sawadogo, S. P., Dabiré, R. K., Lees, R. S., Gilles, J. R. L., et al. (2014). Role of nutritional reserves and body size in Anopheles gambiae males mating success. Acta Trop. 132, S102–S107. doi: 10.1016/j.actatropica.2013.08.018
McCauley, S. J., Rowe, L., and Fortin, M.-J. (2011). The deadly effects of “nonlethal” predators. Ecology 92, 2043–2048. doi: 10.1890/11-0455.1
McIntire, K. M., and Juliano, S. A. (2018). How can mortality increase population size? A test of two mechanistic hypotheses. Ecology 99, 1660–1670. doi: 10.1002/ecy.2375
McPeek, M. A., and Peckarsky, B. L. (1998). Life histories and the strengths of species interactions: combining mortality, growth, and fecundity effects. Ecology 79, 867–879. doi: 10.1890/0012-9658(1998)079[0867:LHATSO]2.0.CO;2
Moller-Jacobs, L. L., Murdock, C. C., and Thomas, M. B. (2014). Capacity of mosquitoes to transmit malaria depends on larval environment. Parasit. Vectors 7, 593–605. doi: 10.1186/s13071-014-0593-4
Mostowy, W. M., and Foster, W. A. (2004). Antagonistic effects of energy status on meal size and egg-batch size of Aedes aegypti (Diptera: Culicidae). J. Vector Ecol. 29, 84–93.
Muturi, E. J., Kim, C.-H., Alto, B. W., Berenbaum, M. R., and Schuler, M. A. (2011). Larval environmental stress alters Aedes aegypti competence for Sindbis virus: environmental stress increases vector competence. Trop. Med. Int. Health 16, 955–964. doi: 10.1111/j.1365-3156.2011.02796.x
Nasci, R. S. (1986). The size of emerging and host-seeking Aedes aegypti and the relation of size to blood-feeding success in the field. J. Am. Mosq. Control. Assoc. 2, 61–62.
Nasci, R. S. (1991). Influence of larval and adult nutrition on biting persistence in Aedes aegypti (Diptera: Culicidae). J. Med. Entomol. 28, 522–526. doi: 10.1093/jmedent/28.4.522
Nelson, E. H., Matthews, C. E., and Rosenheim, J. A. (2004). Predators reduce prey population growth by inducing changes in prey behavior. Ecology 85, 1853–1858. doi: 10.1890/03-3109
Noden, B. H., O'Neal, P. A., Fader, J. E., and Juliano, S. A. (2016). Impact of inter- and intra-specific competition among larvae on larval, adult, and life-table traits of Aedes aegypti and Aedes albopictus females. Ecol. Entomol. 41, 192–200. doi: 10.1111/een.12290
Paradise, C. J., and Dunson, W. A. (1997). Insect species interactions and resource effects in treeholes: are helodid beetles bottom-up facilitators of midge populations? Oecologia 109, 303–312. doi: 10.1007/s004420050088
Peacor, S. D., and Werner, E. E. (2001). The contribution of trait-mediated indirect effects to the net effects of a predator. Proc. Natl. Acad. Sci. U. S. A. 98, 3904–3908. doi: 10.1073/pnas.071061998
Ponlawat, A., and Harrington, L. C. (2009). Factors associated with male mating success of the dengue vector mosquito, Aedes aegypti. Am. J. Trop. Med. Hyg. 80, 395–400. doi: 10.4269/ajtmh.2009.80.395
Preisser, E. L., and Bolnick, D. I. (2008). The many faces of fear: comparing the pathways and impacts of nonconsumptive predator effects on prey populations. PLoS ONE 3:e2465. doi: 10.1371/journal.pone.0002465
Preisser, E. L., Bolnick, D. I., and Benard, M. F. (2005). Scared to death? The effects of intimidation and consumption in predator–prey interactions. Ecology 86, 501–509. doi: 10.1890/04-0719
R Development Core Team (2016). R: A Language and Environment for Statistical Computing. Vienna: R Foundation for Statistical Computing. Available online at: http://www.R-project.org.
Reiskind, M. H., and Lounibos, L. P. (2009). Effects of intraspecific larval competition on adult longevity in the mosquitoes Aedes aegypti and Aedes albopictus. Med. Vet. Entomol. 23, 62–68. doi: 10.1111/j.1365-2915.2008.00782.x
Roberts, D. (2014). Mosquito larvae change their feeding behavior in response to kairomones from some predators. J. Med. Entomol. 51, 368–374. doi: 10.1603/ME13129
Roux, O., Vantaux, A., Roche, B., Yameogo, K. B., Dabiré, K. R., Diabaté, A., et al. (2015). Evidence for carry-over effects of predator exposure on pathogen transmission potential. Proc. R. Soc. B Biol. Sci. 282:20152430. doi: 10.1098/rspb.2015.2430
Scott, T. W., Amerasinghe, P. H., Morrison, A. C., Lorenz, L. H., Clark, G. G., Strickman, D., et al. (2000). Longitudinal Studies of Aedes aegypti (Diptera: Culicidae) in Thailand and Puerto Rico: Blood feeding frequency. J. Med. Entomol. 37, 89–101. doi: 10.1603/0022-2585-37.1.89
Scott, T. W., and Takken, W. (2012). Feeding strategies of anthropophilic mosquitoes result in increased risk of pathogen transmission. Trends Parasitol. 28, 114–121. doi: 10.1016/j.pt.2012.01.001
Siraj, A. S., Oidtman, R. J., Huber, J. H., Kraemer, M. U. G., Brady, O. J., Johansson, M. A., et al. (2017). Temperature modulates dengue virus epidemic growth rates through its effects on reproduction numbers and generation intervals. PLoS Negl. Trop. Dis. 11, e0005797. doi: 10.1371/journal.pntd.0005797
Sota, T., and Mogi, M. (1992). Interspecific variation in desiccation survival time of Aedes (Stegomyia) mosquito eggs is correlated with habitat and egg size. Oecologia 90, 353–358. doi: 10.1007/BF00317691
Stone, C. M., Hamilton, I. M., and Foster, W. A. (2011). A survival and reproduction trade-off is resolved in accordance with resource availability by virgin female mosquitoes. Anim. Behav. 81, 765–774. doi: 10.1016/j.anbehav.2011.01.008
Takken, W., Klowden, M. J., and Chambers, G. M. (1998). Effect of body size on host seeking and blood meal utilization in Anopheles gambiae sensu stricto (Diptera: Culicidae): The disadvantage of being small. J. Med. Entomol. 35, 639–645. doi: 10.1093/jmedent/35.5.639
van Uitregt, V. O., Hurst, T. P., and Wilson, R. S. (2012). Reduced size and starvation resistance in adult mosquitoes, Aedes notoscriptus, exposed to predation cues as larvae: costs and benefits of inducible defences in mosquito larvae. J. Anim. Ecol. 81, 108–115. doi: 10.1111/j.1365-2656.2011.01880.x
Yuval, B., Holliday-Hanson, M. L., and Washing, R. K. (1994). Energy budget of swarming male mosquitoes. Ecol. Entomol. 19, 74–78. doi: 10.1111/j.1365-2311.1994.tb00392.x
Keywords: Aedes aegypti, non-lethal predation, larval competition, life-table, larval development time, body size, fecundity, population-level traits
Citation: Chandrasegaran K and Juliano SA (2019) How Do Trait-Mediated Non-lethal Effects of Predation Affect Population-Level Performance of Mosquitoes? Front. Ecol. Evol. 7:25. doi: 10.3389/fevo.2019.00025
Received: 04 October 2018; Accepted: 23 January 2019;
Published: 12 February 2019.
Edited by:
Alex Perkins, University of Notre Dame, United StatesReviewed by:
Marta Strecker Shocket, Stanford University, United StatesMatteo Marcantonio, University of California, Davis, United States
John Drake, University of Georgia, United States
Michelle V. Evans, University of Georgia, United States, in collaboration with John Drake
Copyright © 2019 Chandrasegaran and Juliano. This is an open-access article distributed under the terms of the Creative Commons Attribution License (CC BY). The use, distribution or reproduction in other forums is permitted, provided the original author(s) and the copyright owner(s) are credited and that the original publication in this journal is cited, in accordance with accepted academic practice. No use, distribution or reproduction is permitted which does not comply with these terms.
*Correspondence: Karthikeyan Chandrasegaran, a2FydGhpa2V5YW5jQG5jYnMucmVzLmlu; c2l2YXNha3RoaS5rYXJ0aGlrZXlhbkBnbWFpbC5jb20=