- Department of Biological Sciences and W. M. Keck Center for Behavioral Biology, North Carolina State University, Raleigh, NC, United States
Human activities cause many changes in wildlife populations, including phenotypic shifts that represent adaptations to new conditions and influence population dynamics, diversity, and persistence. Although many examples of phenotypic adjustment to anthropogenic disturbance exist, we rarely know the extent to which these changes result from genetic evolution or phenotypic plasticity. Furthermore, our understanding of how whole-organism performance changes as a result of habitat alteration is very limited. We tested how urbanization, an important type of global disturbance, influences fish swimming performance in urban streams. Because urban streams have higher water velocities during rain events than rural streams, we tested for increased steady-swimming performance in fish from urbanized watersheds. Across ten populations of wild-caught Creek Chub (Semotilus atromaculatus), we found that fish from streams in more urbanized areas exhibited a longer propulsive wavelength and a lower tailbeat frequency, expending lower levels of hydromechanical power during steady swimming. In a laboratory experiment, we reared individuals collected as fry from four populations with different urbanization histories, and found evidence for genetically based differences in swimming kinematics. Laboratory-reared fish derived from urbanized populations exhibited higher locomotor efficiency, matching predictions for adaptation to urban environments. We exposed laboratory-reared Creek Chub from urban and rural populations to artificially increased water velocity for 4 months and observed that only some populations exhibited plasticity. Urban populations may have lost maladaptive, velocity-induced plasticity in locomotor efficiency that is still present in rural populations. Evolutionary change in freshwater species may represent a widespread yet unrecognized consequence of anthropogenic activity.
Introduction
Human impacts on the environment present one of the greatest challenges for conservation worldwide (Young et al., 2005; Worm et al., 2009; Barbier, 2011). Urbanization in particular is a pervasive, rapidly expanding type of impact that causes dramatic changes in natural ecosystems (Marzluff, 2001; McKinney, 2008). These changes can lead to altered selection and phenotypic divergence in traits such as life history, endocrine traits, birdsong, and seed dispersal, all of which are known to evolve rapidly in urban settings (Partecke and Gwinner, 2007; Cheptou et al., 2008; Luther and Baptista, 2010; Bonier, 2012; Miranda et al., 2013; Alberti et al., 2017). Understanding and predicting trait changes can provide a better understanding of evolution and inform decisions for conservation and sustainable urban development (Carroll et al., 2014; Smith et al., 2014). However, there are substantial gaps in our knowledge: we rarely know the extent to which phenotypic responses are genetic or plastic, or how certain types of traits like whole-organism performance respond to anthropogenic disturbance. Also, although we know of some evolutionary responses to urbanization in terrestrial species, we know practically nothing about how stream species respond evolutionarily to the environmental changes caused by urbanization (Kern and Langerhans, 2018). This is surprising since much attention has been devoted to urban stream degradation (the “urban stream syndrome”) and other effects of human development on aquatic ecosystems (Paul and Meyer, 2001; Wenger et al., 2009; Knouft and Chu, 2015). It is becoming clear that our knowledge of human-induced ecological impacts in streams has far outpaced our needed understanding of evolutionary impacts.
Urbanization strongly affects freshwater streams because their topographic position makes them especially vulnerable to changes in land use (Walsh et al., 2005). One of the most salient changes to urban streams is their rapid rise in discharge following rain events. Impervious land cover like roads and buildings decreases infiltration and hastens the transport of water to streams such that urbanized streams experience faster water velocities during rainfall and more frequent high-discharge events (Poff et al., 2006; Jacobson, 2011). These greater peak flows and more frequent floods have been well documented in urban streams (Dunne, 1978; Shaw, 1994; McCuen, 1998).
This widespread urban hydrologic pattern provides the opportunity to test fundamental evolutionary questions. Studies of fish inhabiting undisturbed systems have uncovered adaptive differences in swimming performance among sites with different current velocities (Nelson et al., 2003; Langerhans, 2008), and it seems reasonable to predict that fish persisting in urban streams might similarly respond to human-altered hydrologic regimes (Nelson et al., 2008). In particular, fish in urban streams might exhibit greater “steady-swimming” performance (i.e., more energetically efficient cruising at constant speeds) than rural fish owing to the demands of human-caused hydrological changes. We tested this hypothesis by measuring differences in swimming performance among fish populations from streams that vary in degree of urbanization.
Swimming ability is an example of whole-organism performance (i.e., the performance of an ecologically relevant task such as running, biting, or jumping), and represents a key component of fitness (Arnold, 1983; Irschick et al., 2008; Langerhans, 2009a). Documented cases of evolution in complex phenotypes—like whole-organism performance—in response to human activity are exceedingly rare (Merilä and Hendry, 2014; Donihue and Lambert, 2015), and phenotypic responses to urbanization are often not tested for a genetic basis (Alberti et al., 2017). However, testing for a genetic basis can be valuable because the genetic vs. plastic basis of trait differentiation may help determine species persistence. Many responses to human impacts seem more plastic than genetic, which on the one hand may constrain species' ability to handle future conditions, since plasticity has a theoretical limit (Hendry et al., 2008). On the other hand, plasticity might allow species to persist long enough to evolve additional adaptations to modified environments (Pigliucci, 2001).
To test the effects of urbanization on steady-swimming abilities of stream fish, we conducted swim-tunnel experiments with a cyprinid minnow, Creek Chub (Semotilus atromaculatus), from streams across a range of urbanization levels. Creek Chub commonly inhabit rural and urban streams, and because of their short generation time (1–2 years to sexual maturity) they could have evolved measurable responses to urbanization over the past 20–120 years. We measured four kinematic swimming variables (tailbeat frequency, tailbeat amplitude, rostral amplitude, and propulsive wavelength) and an estimate of overall locomotor efficiency to assess differences among fish from historically urban, recently urbanized, and rural habitats. These kinematic variables are associated with swimming efficiency and allow us to test specific predictions about phenotypic divergence (McHenry et al., 1995). Based on biomechanical principles, urban fish that experience faster flowing water should benefit from stiffer bodies (longer propulsive wavelengths), reduced tailbeat frequency, and possibly reduced rostral and tailbeat amplitudes (McHenry et al., 1995; Langerhans, 2009b).
To test whether differences observed in the wild reflect plasticity, genetic differentiation, or both, we performed the same swimming measurements on laboratory-reared individuals from a subset of populations. Testing fish raised in a laboratory also reduces the likelihood that unmeasured environmental factors in urban streams (such as pollution) are responsible for any observed differences in swimming performance.
Materials and Methods
Wild-Caught Fish
For trials of wild-caught fish, we collected 64 adult Creek Chub from ten streams in the Piedmont region of North Carolina, USA (Table 1). This particular region has well-documented differences in flow regime between urban and rural streams; previous work has demonstrated that urban streams in our study area are flashier than nearby rural streams (Brown et al., 2009). Using maps and aerial images, we specifically selected study sites to span variation in urbanization across this region. To avoid confounding factors such as habitat size, we further selected similarly sized streams (first and second order). To provide a quantitative measurement of urbanization at each site, we measured the percent of developed land cover within a one-mile radius of each collection site using the 2011 National Land Cover Database and QGIS 2.10. Developed land cover highly correlates with impervious surface cover (Jennings et al., 2004). Estimated developed land cover included all four subcategories in the National Land Cover Database, which comprises constructed materials (e.g., roads, parking lots, businesses, apartments, homes) and open spaces associated with urban development (e.g., lawns, golf courses, urban-associated vegetation). We also examined maps of each watershed, to ensure for example that major upstream development was absent above rural sites. Visual inspection of satellite and aerial images across time (Figure S1), combined with our quantitative measurements of land use, placed each of our sites into one of three “urbanization status” categories which were meant to capture each site's history of urban development (Figure 1). First, historically urban sites (n = 3) had high levels of urbanization (defined as here as > 70% developed land cover) and showed very little change in urban development across aerial photographs from the 1980s and satellite images from the 2010s. Second, recently urbanized sites (n = 3) had moderate to high levels of urbanization (30–80% developed land cover) and showed a substantial increase in developed land cover since the 1980s (Figure S1). Finally, rural sites (n = 4) had a continuously low proportion of developed land cover from the 1980s to the present (< 30% developed land cover). Since phenotypic change may lag behind environmental change (especially for genetic evolution rather than phenotypic plasticity), using these three categories—historically urban, recently urbanized, and rural—could prove more useful than a dichotomous urban/rural designation. The three categories capture the bulk of variation in urbanization in this geographic region, which includes an “old” New World city (Raleigh, North Carolina, founded in 1792), rapidly accelerating localized urban growth, and considerable rural area.
We collected Creek Chub during October 2013 to November 2014 using dip nets and a backpack electroshocker, and transported them in coolers to animal care facilities at North Carolina State University, where they were gradually introduced over several hours to 21°C water temperatures. We allowed each fish to acclimate to laboratory housing for at least three days prior to experimentation. This acclimation period may or may not completely remove stress effects, but previous work indicates that stress does not significantly impact fish swimming performance (Gregory and Wood, 1999; Lankford et al., 2005). Fish were housed in 76-liter aquaria (no flow-through) at 21°C and fed dry flakes daily ad libitum. For swimming trials, fish were moved with as brief air exposure as possible using a bucket and aquarium net into a clear Plexiglas tunnel measuring 15 × 15 × 50 cm. Once each fish recovered from the move (came to rest or began exploring the tunnel), water velocity in the tunnel was increased to 1.4 m/s. This velocity was designed to mimic challenging conditions during stormwater runoff in an urbanized area, yet still remain within the range of the steady-swimming gait (also known as cruising) for this species. Tunnel water velocity was more than twice the speed of average baseflow conditions (0.51 m/s) at our field sites.
Water flow inside the tunnel was powered by an electric sump pump (Leader Provort 540a; Ladson, SC, USA). Water was pumped into the tunnel through a honeycomb baffle of short drinking straws, which helped approximate laminar flow. To control for slight variations in water speed, we used a digital water velocity meter (Global Water Flow Probe) to measure the velocity at the specific location within the tunnel where the fish swam during each trial (mean ± standard error, 1.43 m/s ± 0.01). We also recorded water temperature after each trial for potential use as a covariate, in case small variations in temperature (e.g., from the running pump motor) might affect swimming performance (20.93°C ± 0.14). In rare cases where fish did not respond to the artificial current by swimming against it, they were returned to aquaria and retested on a different day. In our dataset these individuals (n = 3) represent <5% of the total number of individuals.
Swimming trials were recorded with a high-speed digital video camera (IDT N4-S1). A mirror propped underneath the tunnel at 45° allowed us to simultaneously capture ventral and lateral views of the fish as it swam at constant speed, maintaining position in the tunnel. From the video sequences (set to 400 fps with a few exceptions at 200 fps) we used tpsDig (Rohlf, 2013) to collect spatial coordinates of the fish's snout, body, and tail positions in order to measure four kinematic variables: tailbeat frequency, tailbeat amplitude, rostral amplitude, and propulsive wavelength (McHenry et al., 1995). Tailbeat frequency is measured in beats per second, with one beat representing a full cycle from one side of the body to the opposite side and back to the original location. Tailbeat amplitude is half the distance between the maximum left and right excursions of the most distal tip of the caudal fin during one complete tailbeat. Similarly, rostral amplitude is half the distance of the maximum left and right excursions of the tip of the snout. We calculated propulsive wavelength by doubling the propulsive half-wavelength, which is the distance between upper and lower crests of the sinusoidal wave created between the midbody and midtail region of a fish in motion (Webb et al., 1984). We measured each variable three times in each video and used the means for final analysis. Because kinematic variables may be correlated with one another, we performed principal components analysis (PCA) on the correlation matrix of the four kinematic variables to reduce data dimensionality. We retained PCs with eigenvalues ≥ 1 for analysis of kinematic variation, resulting in retention of the first two PCs, explaining 72.6% of the variance (Table 2).
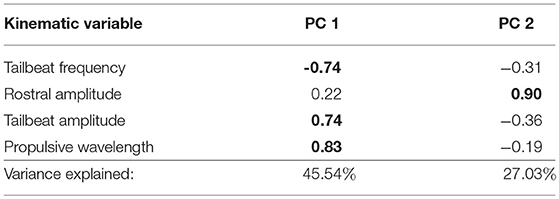
Table 2. Trait loadings and variance explained by the first two principal components for the PCA of swimming kinematic data in wild-caught Creek Chub (loadings ≥ |0.60| in bold text).
To provide a summary of the magnitude of thrust production, we estimated total hydromechanical power using Lighthill's elongated-body theory (Wu, 1971; Lighthill, 1975; Videler, 1993). We calculated power (P) as f 2H2B2(1–U/c), where f , tailbeat frequency; H, tailbeat amplitude; B, caudal fin depth; U, swimming speed, and c, wave speed (propulsive wavelength times tailbeat frequency). This parameter can be thought of as the total lateral force produced by the tail to overcome drag forces and maintain constant-velocity swimming. Holding swimming speed constant, a lower P indicates greater overall locomotor efficiency (i.e., less power consumed to swim at a given speed, analogous to miles per gallon).
We performed two sets of analyses to test for effects of urbanization on steady-swimming performance in wild-caught Creek Chub: one examining variation across categories of urbanization, and one utilizing estimates of continuous variation in degree of urbanization. First, we tested for kinematic and power differences between the three habitat types using a general linear mixed model (using restricted maximum likelihood) for each of the three response variables (PC 1, PC 2, and hydromechanical power) with body lengths per second as a covariate, habitat as a fixed effect, and population as a random effect. We included body lengths per second as a covariate to control for both variation in body size (Table S1) and any slight variation in water velocity—i.e., statistically adjust response variables to allow comparison among groups at a constant relative swimming speed. Body lengths per second is a standard metric for measuring and comparing fish swimming speed (Bainbridge, 1958). It was measured by recording fish swimming at a known speed in the tunnel (i.e., swimming “in place” against a constant current of known velocity) and dividing water velocity by body length (standard length). Thus, swimming speed was highly correlated with body length (r = −0.92, P < 0.0001), and all results are qualitatively no different than if we use body size as a covariate instead of swimming speed. We initially included water temperature as a covariate but excluded it due to non-significance. Second, we calculated population means (n = 10) for all three response variables (least-squares means, controlling for body lengths per second) and conducted Spearman rank correlations between swimming performance means and site-specific estimates of urban development land cover. The latter analyses took advantage of continuous variation in present-day urbanization levels to test whether populations surrounded by more urban land cover tend to exhibit greater steady-swimming performance. The amount of urban land cover was quantified by measuring the percentage of developed land cover in the 2.6 km (1 mi) radius around each site, using QGIS and the 2011 National Land Cover Database (Homer et al., 2015).
Because multiple comparisons (e.g., multiple tests on the same set of fish) can increase Type I error rates, we used QValue (Storey, 2003) to control the false discovery rate at 5% using the bootstrap procedure for estimating the probability of a true null hypothesis (Benjamini and Hochberg, 1995). The false discovery rate (FDR) is the proportion of significant tests that are in fact null. We accepted tests in this study as significant only when both the p-value of the test and its q-value (determined by the QValue program) were ≤ 0.05. Because all observed p-values ≤ 0.05 in the present study remained significant at the FDR of 5%, we straightforwardly interpret p-values ≤ 0.05 as being significant. Due to modest sample sizes in several populations, we avoid making strong conclusions regarding the absence of effects based on non-significant findings. Moreover, all results reported in this study are qualitatively unchanged using permutation tests in place of our parametric F tests (using the permuco R package; Kherad-Pajouh and Renaud, 2010, 2015).
Laboratory-Raised Fish
To test whether differences between populations persisted in fish reared in a common laboratory environment (which would suggest a genetic basis), we conducted swimming trials of laboratory-raised Creek Chub from four populations. We used dip nets and aquarium nets to catch late postlarval to very early stage juvenile Creek Chub (1.5–3.0 cm standard length; Buynak and Mohr, 1979) from four stream sites during the hatching and emergence period (June–July 2014; Washburn, 1948; Table 1). Sample sites were selected to cover the entire urbanization gradient. Fish were transported in 19-liter buckets to facilities at North Carolina State University and each population was randomly separated into two treatment groups—high velocity and low velocity (descriptions below). Fish were divided among eight ten-liter tanks according to population and treatment (2–15 Creek Chub per tank; Table S3). All tanks were part of a single recirculating system (Aquatic Habitats; Apopka, FL), which allowed populations and treatment groups to experience identical water conditions. Fish were fed brine shrimp and dry flakes twice daily and kept on a 12:12 h light-dark cycle. Water conditions were maintained at 0.5 ppt salinity and 25°C.
After 2 weeks of rearing all fish in common conditions to allow acclimation to the laboratory-rearing environment, we initiated water velocity treatments designed to roughly mimic flow conditions of urban and rural stream. High-velocity treatment groups received 60 min of intensified current once per week for 16 weeks to simulate increased velocity in urban streams during rainfall events. Current was generated by fully opening the two tank nozzles for each tank (two separate nozzles provide water to each tank within the recirculating system), which flushed tanks at a constant rate of 4.2 L/min (compared to 0.7 L/min at other times and throughout the low-velocity treatment). This created elevated, non-uniform water velocity within the tanks (~0.9–5.5 cm/s) and forced fish to swim against a moderate current to maintain position (~1–3.5 body lengths per second). Low-velocity treatment groups never received any water velocity increases, and experienced non-uniform water velocity ~0.1–0.9 cm/s within the tanks at all times throughout the experiment.
After 18 weeks of laboratory rearing (16 weeks of treatment and 2 weeks of initial acclimation time), fish were recorded swimming in a Plexiglas swim tunnel measuring 5 × 6 × 21 cm against a current of 0.27 m/s. Water temperature was held constant at 25°C. Dimensions and velocity differ from that used for the wild-caught fish above owing to the differences in body size between adult wild-caught fish and juvenile laboratory-raised fish (if we had tested juveniles at speeds of 1.4 m/s they would not have been able to swim against the current). As before, we used high-speed video and an angled mirror to capture a lateral and ventral view of the fish swimming, and we used the same methods as above to measure the same four kinematic variables and hydromechanical power.
We again performed a PCA on the four kinematic variables as described above, and retained the first two PCs for analysis, explaining 70% of the variance (Table 3). We fit a general linear model to examine kinematic and hydromechanical power variation across treatments, populations, and the interaction of treatment and population, with body lengths per second as a covariate to control for possible effects of body size. Our inclusion of body lengths per second in the model was in essence equivalent to including body size because all fish were tested at a common velocity, and thus this procedure appropriately adjusts for size effects. We further tested for non-linear effects of body size (squared term), but no non-linear effects were observed (p > 0.91). Moreover, we confirmed that body size broadly overlapped among populations within this smaller subset of populations (Table S2), permitting the proper statistical test and adjustment for body size. The treatment term tests for water velocity–induced phenotypic plasticity. The population term tests for environment-independent differences between populations, which would imply genetically based variation in swimming performance, though parental effects cannot be ruled out. The interaction between treatment and population tests whether velocity-induced plasticity differs between populations. For each term we calculated η2 (an estimate of effect size) to directly assess the relative importance of the terms (Langerhans and DeWitt, 2004). We used Tukey's honestly significant difference test to interpret significant categorical model terms (only groups with p ≤ 0.05 treated as significantly different).
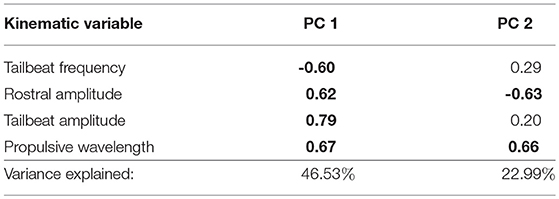
Table 3. Trait loadings and variance explained by the first two principal components for the PCA for swimming kinematic data in laboratory-raised Creek Chub (loadings ≥ |0.60| in bold text).
Animal Use Statement
This study was approved by the Institutional Animal Care and Use Committee at North Carolina State University.
Results
Wild-Caught Fish
In our tests of swimming-performance differences among the three categories of urbanization status, we found that only one of the three response variables showed significant differences. PC 1 scores of steady-swimming kinematics differed between habitats (F(2,59) = 6.24, p = 0.0035) and negatively co-varied with body lengths per second (F(1,59) = 135.36, p < 0.0001). Fish from historically urban populations exhibited significantly higher PC 1 scores than other populations, when statistically controlling for body lengths per second (Figure 2A). Higher PC 1 scores in historically urban fish indicated longer propulsive wavelengths (i.e., stiffer bodies), reduced tailbeat frequencies, and greater tailbeat amplitudes. For PC 2, the covariate was non-significant (F(1,59) = 0.14, p = 0.7126) and habitat type was marginally non-significant (F(2,59) = 2.79, p = 0.0694), suggesting a trend where rural fish had lower PC 2 scores than recently urbanized fish, with historically urban fish intermediate. Hydromechanical power negatively scaled with body lengths per second (F(1,59) = 14.22, p = 0.0004). Hydromechanical power did not differ significantly with habitat types (F(2, 59) = 1.80, p = 0.1746), even though the trends did match a priori predictions, with rural fish tending to require more hydromechanical power to swim at a given swimming speed compared to historically urban fish, and with recently urbanized fish intermediate between the two (Figure 2B).
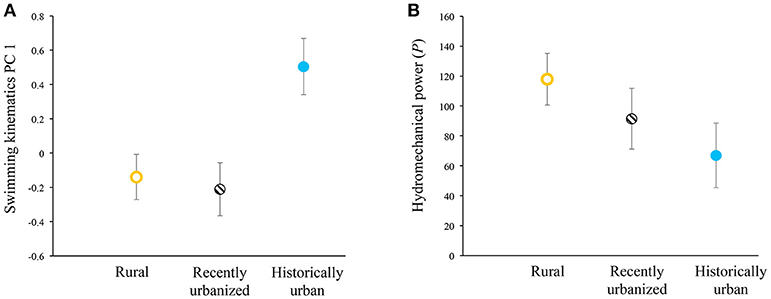
Figure 2. Variation in (A) swimming kinematics PC 1 scores and (B) hydromechanical power across the three habitat types for ten populations of wild-caught Creek Chub. Higher PC 1 scores reflect longer propulsive wavelengths (i.e., stiffer bodies), reduced tailbeat frequencies, and greater tailbeat amplitudes (Table 2), controlling for relative swimming speed (body lengths per second). Least-squares means ± 1 SE depicted.
Spearman rank correlation tests revealed that Creek Chub from sites with greater urban development land cover had significantly higher swimming kinematic PC 1 scores (ρ = 0.69, p = 0.0289) and lower hydromechanical power (ρ = −0.67, p = 0.0330) (Figure 3); no relationship was observed for swimming kinematic PC 2 (ρ = 0.35, p = 0.3282). Thus, during steady swimming, Creek Chub populations in more urban areas tended to exhibit longer propulsive wavelengths, reduced tailbeat frequencies, greater tailbeat amplitudes, and employ lower overall hydromechanical power to swim at a given speed.
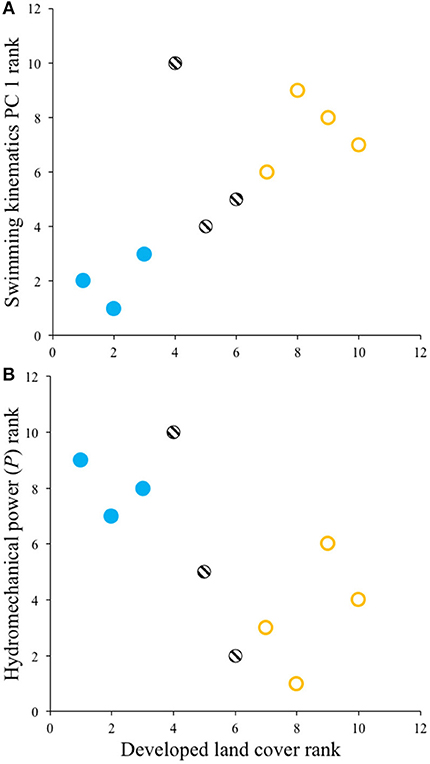
Figure 3. Spearman rank correlations showing that Creek Chub from streams with greater urban development had (A) higher swimming kinematics PC 1 scores and (B) lower hydromechanical power. Symbols follow Figure 2.
Laboratory-Raised Fish
We uncovered evidence suggesting genetically based differences between populations in swimming performance (all three variables) and for population-specific variation in water velocity–induced plasticity (two variables), but found no evidence of shared patterns of velocity-induced plasticity across populations (Table 4).
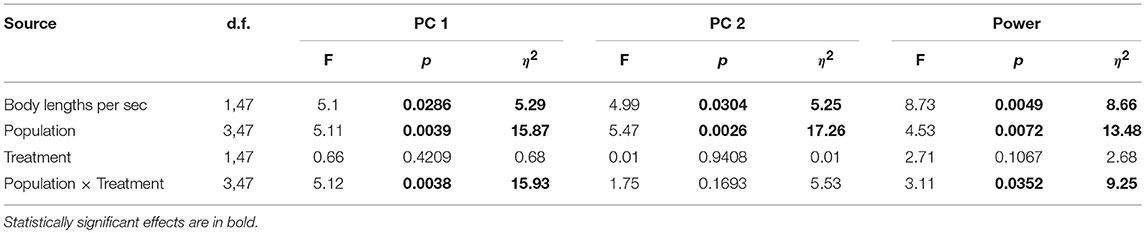
Table 4. Results of general linear models examining steady-swimming kinematic variation in laboratory-raised Creek Chub.
For the laboratory-raised Creek Chub, PC 1 scores were negatively correlated with body lengths per second and were significantly different between populations. For one population (Beaver Creek), PC 1 scores varied between velocity treatments (Figure 4A). A rural population, Rocky Branch, tended to exhibit higher PC 1 scores than other populations regardless of treatment. Plasticity was only evident for a recently urbanized population (Beaver Creek), with significantly lower PC 1 scores in the high-velocity treatment than in the low-velocity treatment (Figure 4A).
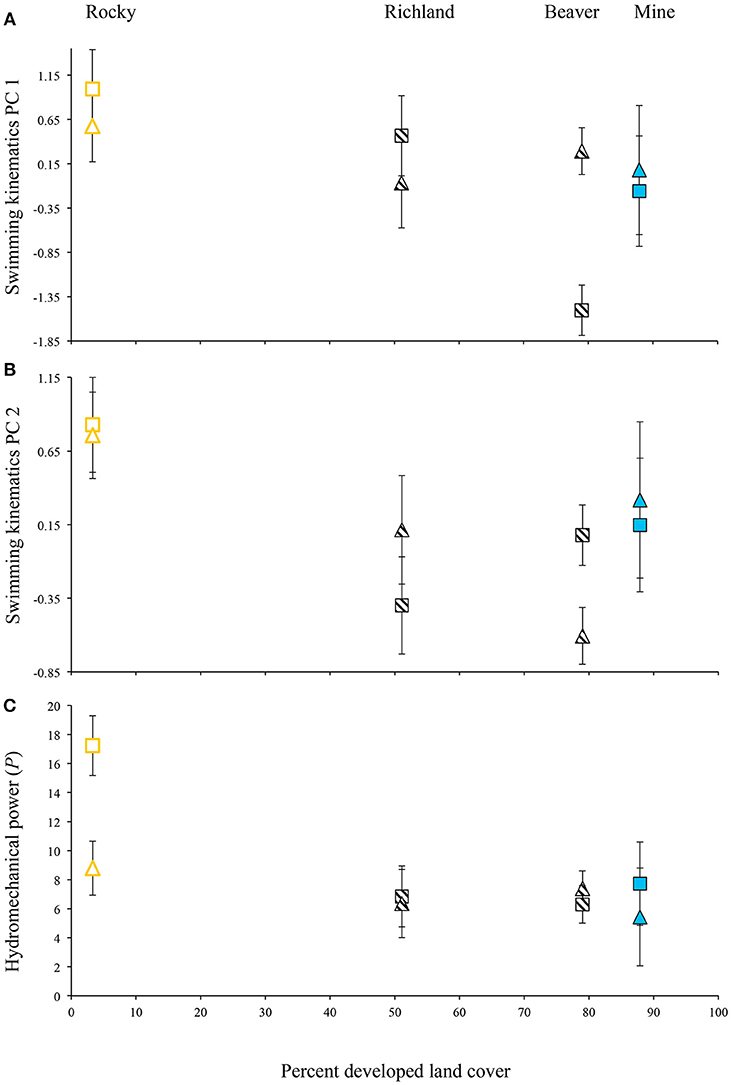
Figure 4. Variation in (A) swimming kinematics PC 1 scores, (B) swimming kinematics PC 2 scores, and (C) hydromechanical power for four Creek Chub populations raised in the laboratory under two velocity treatments, controlling for relative swimming speed (body lengths per second). Triangles, low-velocity treatment; squares, high-velocity treatment; open symbols, rural; hatched symbols, recently urbanized; solid symbols, historically urban. Least-squares means ± 1 SE depicted.
PC 2 also exhibited a negative relationship with body lengths per second and significantly differed among populations, but showed no significant velocity-induced plasticity (Figure 4B). Again, the rural site (Rocky Branch) exhibited the highest PC 2 scores.
Hydromechanical power was negatively associated with body lengths per second, significantly differed among populations, and showed velocity-induced plasticity within one population (Figure 4C). Rocky Branch exhibited the highest overall power, indicating that the rural population tended to use a greater amount of hydromechanical power to swim at the same speed as fish from urbanized areas. Rocky Branch was also the only population that exhibited significant velocity-induced plasticity in hydromechanical power: higher power was produced by fish raised in the high-velocity treatment.
Discussion
The ecological and evolutionary importance of whole-organism performance is undisputed, yet we know little about how human activities affect it (Irschick et al., 2008) or whether its response to human impacts more likely reflects genetic evolution or phenotypic plasticity (Hendry et al., 2008). Here we found that the steady-swimming performance of Creek Chub (wild and laboratory-raised) differs with stream urbanization, and that although some aspects of performance in some populations show velocity-induced plasticity, most of the differences between populations appear to reflect genetic differentiation, although controlled breeding experiments would be necessary to confirm this. Wild-caught Creek Chub from rural streams expended more hydromechanical power during steady swimming than urban fish swimming at the same speed, and patterns in laboratory-raised fish suggest a genetic basis to these differences as a rural population still showed lower steady-swimming efficiency than populations from urbanized streams. Our results demonstrate one way in which humans can impact the evolution of phenotypic traits and suggest that urbanization may be leaving a noticeable mark on whole-organism performance evolution in some stream species. The underlying biological causes of altered swimming performance were not measured here, but presumably, evolved differences in physiological and morphological attributes provide key sources: in fact, past work in Creek Chub has shown that body shape changes subsequent to urbanization (Kern and Langerhans, 2018), and the morphology and physiology of various stream fish also differ between impacted and unimpacted streams (Blevins et al., 2013; Gaulke et al., 2015; King et al., 2016; Pease et al., 2018).
For wild-caught Creek Chub, the results partially agreed with our predictions for how fish should adapt to higher peak water velocities in urbanized watersheds. First, Creek Chub from historically urban sites and from sites with greater overall developed land cover had longer propulsive wavelengths than fish from rural or recently urbanized sites. Longer propulsive wavelengths indicate greater body stiffness in fish (Blight, 1977; Long et al., 1996) and should increase steady-swimming efficiency (McHenry et al., 1995; Langerhans, 2009b); thus, the changes we observed could be adaptive. Second, wild-caught urban fish had lower tailbeat frequencies than rural fish, which should also indicate increased efficiency since tailbeat frequency often provides a useful surrogate for oxygen consumption (Herskin and Steffensen, 1998; Steinhausen et al., 2005). On the other hand, urban fish did not have significantly lower tailbeat amplitudes as might have been expected (since decreased amplitude is one way to reduce mechanical work). Altogether, the kinematics results indicate that Creek Chub swim differently in streams with different urbanization levels, and that in urbanized environments their kinematics indicate greater locomotor efficiency. Indeed, wild-caught Creek Chub from streams in more urbanized areas actually did exhibit greater swimming efficiency, as shown by their lower hydromechanical power during steady swimming. This suggests that Creek Chub populations from more urbanized areas incur lower metabolic costs while swimming steadily at the same speed as their more rural counterparts.
Do these differences in swimming performance arise via evolutionary change or phenotypic plasticity? By raising multiple populations in a common environment, we found evidence for a genetic basis to variation in swimming kinematics. We uncovered significant among-population variation in all three variables tested, mainly reflecting differentiation between the rural population and the three more urbanized populations. These patterns are consistent with our findings in wild-caught adults; fish derived from urbanized environments exhibited greater hydromechanical efficiency than fish derived from a rural environment, even after they had been raised in common conditions. This suggests adaptive evolution, in which fish responded to urbanization by evolving greater locomotor efficiency, which is probably an advantage when coping with the strong currents that frequently occur in urban streams after rain events.
While our results support genetic differentiation, parental effects on swimming performance cannot be ruled out because practical constraints (i.e., time to maturity in this species) precluded rearing multiple generations in the laboratory. However, in fish species the existence of maternal effects is commonly manifest in life-history traits such as offspring size (Reznick et al., 1996; Heath et al., 1999; Green, 2008), while our findings involve whole-organism performance independent of body size; and even maternal effects can facilitate contemporary evolution (Räsänen and Kruuk, 2007). Furthermore, prior work has often found that fish locomotor performance has a genetic basis (Nicoletto, 1995; Garenc et al., 1998; Ghalambor et al., 2004; Langerhans, 2009a; Johnson et al., 2010). Another possible alternative explanation is that natural selection or environmental effects on steady-swimming performance could have already occurred in the field prior to collection of laboratory-reared fish. To address this, as much as possible we minimized the amount of time for selection or environmental induction to have occurred by collecting very small fish soon after hatching within each population. We then raised all fish in the laboratory for a total of 18 weeks, which includes an especially formative period of development: their entire first summer. However, more prolonged laboratory rearing might produce more plasticity (Kelley et al., 2017).
Although laboratory-raised Creek Chub from urbanized populations showed some hallmarks of greater locomotor efficiency as predicted, such as lower tailbeat amplitudes and lower hydromechanical power, other among-population differences in laboratory-raised fish kinematics (as seen in PC 1) did not conform to predicted patterns even though kinematics in wild-caught fish did. It is unclear why kinematics in wild-caught and laboratory-raised Creek Chub were not more similar, but swimming efficiency in living animals is a complex activity influenced by many factors, including ontogeny, muscle fiber composition, physiological adaptations, body and fin morphology, and possibly genetic constraints on kinematics (Ghalambor et al., 2004; Lailvaux et al., 2010; Walker, 2010; Dalziel and Schulte, 2012), any one of which could be responsible for wild and laboratory-raised differences. Alternatively, the differences between wild and laboratory-raised fish could be due to countergradient evolution, in which genetic differences (along an environmental gradient) compensate for the impact of the environment on phenotype, thus minimizing phenotypic differences (Conover and Schultz, 1995).
The timescale of the evolutionary divergence we document here is within the range of previously documented, human-induced contemporary evolution (Hendry et al., 2008). Fish can evolve substantial divergence in fewer than 13 generations (Hendry et al., 2000), and given the 50-year or longer history of urbanization at many of our urban sites, and the 1–2 year generation time for Creek Chub, the divergence we observed is not unprecedented despite the common perception of evolution as a much slower process.
Our laboratory rearing experiment included a test for velocity-induced plasticity, which is not uncommon in fish (e.g., Broughton and Goldspink, 1978). In response, some Creek Chub populations showed plasticity in some performance variables. However, the overall effect of treatment for all populations taken together was not significant for any performance variable, and the effect size of the population term was as large as or larger than the effect of the interaction term in all cases. The modest sample sizes in the laboratory-reared populations lead us to interpret these non-significant results with caution; plasticity may be present in some populations at a magnitude too small for detection in this study. It is also conceivable that a stronger experimental treatment (faster or more frequent high-velocity exposure) would have elicited plastic responses from more populations or in more kinematic variables, though the high-velocity treatment should have been adequate since it produced a more than 6-fold increase in water velocity, caused elevated swimming activity, and approximated the frequency and duration of summer storms in the region for 4 months of postlarval rearing, which is a substantial growing period for these fish.
The fact that some laboratory-reared populations did respond in some kinematic variables to the velocity treatment could reflect adaptive plasticity. For instance, in the recently urbanized Beaver Creek population, fish in the high-velocity treatment displayed lower tailbeat amplitudes, which should indicate enhanced locomotor efficiency. However, other aspects of observed plasticity appear to counter adaptive hypotheses. For example, in the rural Rocky Branch population, fish in the high-velocity treatment showed higher hydromechanical power, which could indicate reduced locomotor efficiency under challenging flood conditions (rarer in rural streams). In light of this result, it is possible that urbanized populations have evolved means of diminishing the negative consequences of increased water velocities, thus maintaining similar locomotor efficiencies regardless of flow regime—that is, urban populations could have evolved changes in unmeasured traits (including adaptive plasticity in those traits) which eliminated the maladaptive velocity-induced plasticity historically present in locomotor efficiency. Fish might also be responding to changes in urban stream morphology (fewer riffles, less woody debris) that reduce spatial variation in water velocity. In general, to better understand how urbanization leads to changes in phenotypic plasticity, as may often occur in the wild (Lande, 2009, 2015; Crispo et al., 2010), future work will need to investigate velocity-induced plasticity in multiple traits that affect steady-swimming abilities.
In conclusion, we found that Creek Chub exhibit greater steady-swimming efficiency in urbanized streams, as predicted, suggesting urbanization could be an unappreciated yet major driver of evolution in aquatic organisms. Urbanization causes many changes in addition to water velocity: it can alter thermal regimes and structural composition of streams, introduce pollutants, and greatly reduce species diversity (Mcdonald et al., 2008; Brown et al., 2009), any of which could have also played a role in the present study, but we now know that alterations in flow regimes have major evolutionary consequences for fish (Langerhans, 2008; Haas et al., 2010; Franssen, 2011). This has at least two conservation ramifications: first, it suggests that altered flow regimes in urban streams are a strong driver of biological changes, implying that slowing down stormwater discharge may be an important part of mitigating the urban stream syndrome. Second, it indicates that for some species, urban populations may be genetically divergent from rural populations, which in turn has implications for how these populations are managed. However, whether adaptive responses to urban conditions could protect some species from population decline or extirpation is still unknown (Kark et al., 2007; Schiffers et al., 2012).
Data Availability Statement
Data is available upon request: the raw data supporting the conclusions of this manuscript will be made available by the authors, without undue reservation, to any qualified researcher.
Author Contributions
All authors listed have made a substantial, direct and intellectual contribution to the work, and approved it for publication.
Conflict of Interest Statement
The authors declare that the research was conducted in the absence of any commercial or financial relationships that could be construed as a potential conflict of interest.
Acknowledgments
We gratefully thank Jonathan Otten and Natalia Zbonack for their contributions to fish photography and video analysis; Benjamin Pluer, Chris Schalk, and Carmen Montaña for their aid in the field; and Erik Archer for assistance with live fish care.
Supplementary Material
The Supplementary Material for this article can be found online at: https://www.frontiersin.org/articles/10.3389/fevo.2018.00229/full#supplementary-material
References
Alberti, M., Marzluff, J., and Hunt, V. M. (2017). Urban driven phenotypic changes: empirical observations and theoretical implications for eco-evolutionary feedback. Phil. Trans. R. Soc. B. 372:20160029. doi: 10.1098/rstb.2016.0029
Arnold, S. J. (1983). Morphology, performance and fitness. Am. Zool. 23, 347–361. doi: 10.1093/icb/23.2.347
Bainbridge, R. (1958). The speed of swimming of fish as related to size and to the frequency and amplitude of the tail beat. J. Exp. Biol. 35, 109–133.
Barbier, E. B. (2011). Progress and challenges in valuing coastal and marine ecosystem services. Rev. Environ. Econ. Policy 6, 1–19. doi: 10.1093/reep/rer017
Benjamini, Y., and Hochberg, Y. (1995). Controlling the false discovery rate - a practical and powerful approach to multiple testing. J. R. Stat. Soc. Ser. B: Methodol. 57, 289–300. doi: 10.1111/j.2517-6161.1995.tb02031.x
Blevins, Z. W., Effert, E. L., Wahl, D. H., and Suski, C. D. (2013). Land use drives the physiological properties of a stream fish. Ecol. Indic. 24, 224–235. doi: 10.1016/j.ecolind.2012.06.016
Blight, A. R. (1977). The muscular control of vertebrate swimming movements. Biol. Rev. 52, 181–218. doi: 10.1111/j.1469-185X.1977.tb01349.x
Bonier, F. (2012). Hormones in the city: endocrine ecology of urban birds. Horm. Behav. 61, 763–772. doi: 10.1016/j.yhbeh.2012.03.016
Broughton, N. M., and Goldspink, G. (1978). Biochemical changes in the lateral muscles of roach, Rutilus rutilus from two habitats following exercise. J. Fish Biol. 13, 613–618. doi: 10.1111/j.1095-8649.1978.tb03474.x
Brown, L. R., Cuffney, T. F., Coles, J. F., Fitzpatrick, F., McMahon, G., Steuer, J., et al. (2009). Urban streams across the USA: lessons learned from studies in 9 metropolitan areas. J. North Am. Benthol. Soc. 28, 1051–1069. doi: 10.1899/08-153.1
Buynak, G. L., and Mohr, H. W. (1979). Larval development of creek chub and fallfish from two Susquehanna River tributaries. Prog. Fish-Cult. 41, 124–129. doi: 10.1577/1548-8659(1979)41[124:LDOCCA]2.0.CO;2
Carroll, S. P., Jorgensen, P. S., Kinnison, M. T., Bergstrom, C. T., Denison, R. F., Gluckman, P., et al. (2014). Applying evolutionary biology to address global challenges. Science 346, 1245993–1245993. doi: 10.1126/science.1245993
Cheptou, P.-O., Carrue, O., Rouifed, S., and Cantarel, A. (2008). Rapid evolution of seed dispersal in an urban environment in the weed Crepis sancta. Proc. Natl. Acad. Sci. U.S.A. 105, 3796–3799. doi: 10.1073/pnas.0708446105
Conover, D. O., and Schultz, E. T. (1995). Phenotypic similarity and the evolutionary significance of countergradient variation. Trends Ecol. Evol. 10, 248–252. doi: 10.1016/S0169-5347(00)89081-3
Crispo, E., Dibattista, J. D., Correa, C. C., Thibert-Plante, X., McKellar, A. E., Schwartz, A. K., et al. (2010). The evolution of phenotypic plasticity in response to anthropogenic disturbance. Evol. Ecol. Res. 12, 47–66.
Dalziel, A. C., and Schulte, P. M. (2012). Correlates of prolonged swimming performance in F2 hybrids of migratory and non-migratory threespine stickleback. J. Exp. Biol. 215, 3587–3596. doi: 10.1242/jeb.071951
Donihue, C. M., and Lambert, M. R. (2015). Adaptive evolution in urban ecosystems. Ambio. 44, 194–203. doi: 10.1007/s13280-014-0547-2
Franssen, N. R. (2011). Anthropogenic habitat alteration induces rapid morphological divergence in a native stream fish. Evol. Appl. 4, 791–804. doi: 10.1111/j.1752-4571.2011.00200.x
Garenc, C., Silversides, F. G., and Guderley, H. (1998). Burst swimming and its enzymatic correlates in the threespine stickleback (Gasterosteus aculeatus): full-sib heritabilities. Can. J. Zool. 76, 680–688. doi: 10.1139/z97-236
Gaulke, G. L., Wolfe, J. R., Bradley, D. L., Moskus, P. E., Wahl, D. H., and Suski, C. D. (2015). Behavioral and physiological responses of largemouth bass to rain-induced reductions in dissolved oxygen in an urban system. Trans. Am. Fish. Soc. 144, 927–941. doi: 10.1080/00028487.2015.1054517
Ghalambor, C. K., Reznick, D. N., and Walker, J. A. (2004). Constraints on adaptive evolution: the functional trade-off between reproduction and fast-start swimming performance in the Trinidadian guppy (Poecilia reticulata). Am. Nat. 164, 38–50. doi: 10.1086/421412
Green, B. S. (2008). “Maternal effects in fish populations,” in Advances in Marine Biology Vol 54, eds D. W. Sims (Cambridge: Academic Press), 1–105.
Gregory, T. R., and Wood, C. M. (1999). The effects of chronic plasma cortisol elevation on the feeding behaviour, growth, competitive ability, and swimming performance of juvenile rainbow trout. Physiol. Biochem. Zool. 72, 286–295. doi: 10.1086/316673
Haas, T. C., Blum, M. J., and Heins, D. C. (2010). Morphological responses of a stream fish to water impoundment. Biol. Lett. 6, 803–806. doi: 10.1098/rsbl.2010.0401
Heath, D. D., Fox, C. W., and Heath, J. W. (1999). Maternal effects on offspring size: variation through early development of chinook salmon. Evolution 53, 1605–1611. doi: 10.1111/j.1558-5646.1999.tb05424.x
Hendry, A. P., Farrugia, T. J., and Kinnison, M. T. (2008). Human influences on rates of phenotypic change in wild animal populations. Mol. Ecol. 17, 20–29. doi: 10.1111/j.1365-294X.2007.03428.x
Hendry, A. P., Wenburg, J. K., Bentzen, P., Volk, E. C., and Quinn, T. P. (2000). Rapid evolution of reproductive isolation in the wild: evidence from introduced salmon. Science 290, 516–519. doi: 10.1126/science.290.5491.516
Herskin, J., and Steffensen, J. F. (1998). Energy savings in sea bass swimming in a school: measurements of tail beat frequency and oxygen consumption at different swimming speeds. J. Fish Biol. 53, 366–376. doi: 10.1111/j.1095-8649.1998.tb00986.x
Homer, C. G., Dewitz, J. A., Yang, L., Jin, S., Danielson, P., Xian, G., et al. (2015). Completion of the 2011 National Land Cover Database for the conterminous United States-Representing a decade of land cover change information. Photogramm. Eng. Remote Sensing 81, 345–354. doi: 10.14358/PERS.81.5.345
Irschick, D. J., Meyers, J. J., Husak, J. F., and Le Galliard, J.-F. (2008). How does selection operate on whole-organism functional performance capacities? A review and synthesis. Evol. Ecol. Res. 10, 177–196.
Jacobson, C. R. (2011). Identification and quantification of the hydrological impacts of imperviousness in urban catchments: a review. J. Environ. Manage. 92, 1438–1448. doi: 10.1016/j.jenvman.2011.01.018
Jennings, D. B., Jarnagin, S. T., and Ebert, D. W. (2004). A modeling approach for estimating watershed impervious surface area from National Land Cover Data 92, Photogramm. Eng. Rem. Sens. 70, 1295–1307. doi: 10.14358/PERS.70.11.1295
Johnson, D. W., Christie, M. R., and Moye, J. (2010). Quantifying evolutionary potential of marine fish larvae: heritability, selection, and evolutionary constraints. Evolution 64, 2614–2628. doi: 10.1111/j.1558-5646.2010.01027.x
Kark, S., Iwaniuk, A., Schalimtzek, A., and Banker, E. (2007). Living in the city: can anyone become an “urban exploiter”? J. Biogeogr. 34, 638–651. doi: 10.1111/j.1365-2699.2006.01638.x
Kelley, J. L., Davies, P. M., Collin, S. P., and Grierson, P. F. (2017). Morphological plasticity in a native freshwater fish from semiarid Australia in response to variable water flows. Ecol. Evol. 7, 6595–6605. doi: 10.1002/ece3.3167
Kern, E. M. A., and Langerhans, R. B. (2018). Urbanization drives contemporary evolution in stream fish. Glob. Chang. Biol. 24, 3791–3803. doi: 10.1111/gcb.14115
Kherad-Pajouh, S., and Renaud, O. (2010). An exact permutation method for testing any effect in balanced and unbalanced fixed effect ANOVA. Comput. Stat. Data Anal. 54, 1881–1893. doi: 10.1016/j.csda.2010.02.015
Kherad-Pajouh, S., and Renaud, O. (2015). A general permutation approach for analyzing repeated measures ANOVA and mixed-model designs. Stat. Papers 56, 947–967. doi: 10.1007/s00362-014-0617-3
King, G. D., Chapman, J. M., Cooke, S. J., and Suski, C. D. (2016). Stress in the neighborhood: tissue glucocorticoid function relative to changes in stream quality for five species of stream fish. Sci. Total Environ. 547, 87–94. doi: 10.1016/j.scitotenv.2015.12.116
Knouft, J. H., and Chu, M. L. (2015). Using watershed-scale hydrological models to predict the impacts of increasing urbanization on freshwater fish assemblages. Ecohydrology 8, 273–285. doi: 10.1002/eco.1506
Lailvaux, S. P., Hall, M. D., and Brooks, R. C. (2010). Performance is no proxy for genetic quality: trade-offs between locomotion, attractiveness, and life history in crickets. Ecology 91, 1530–1537. doi: 10.1890/09-0963.1
Lande, R. (2009). Adaptation to an extraordinary environment by evolution of phenotypic plasticity and genetic assimilation. J. Evol. Biol. 22, 1435–1446. doi: 10.1111/j.1420-9101.2009.01754.x
Lande, R. (2015). Evolution of phenotypic plasticity in colonizing species. Mol. Ecol. 24, 2038–2045. doi: 10.1111/mec.13037
Langerhans, R. B. (2008). Predictability of phenotypic differentiation across flow regimes in fishes. Integr. Comp. Biol. 48, 750–768. doi: 10.1093/icb/icn092
Langerhans, R. B. (2009a). Morphology, performance, fitness: functional insight into a post-Pleistocene radiation of mosquitofish. Biol. Lett. 5, 488–491. doi: 10.1098/rsbl.2009.0179
Langerhans, R. B. (2009b). Trade-off between steady and unsteady swimming underlies predator-driven divergence in Gambusia affinis. J. Evol. Biol. 22, 1057–1075. doi: 10.1111/j.1420-9101.2009.01716.x
Langerhans, R. B., and DeWitt, T. J. (2004). Shared and unique features of evolutionary diversification. Am. Nat. 164, 335–349. doi: 10.1086/422857
Lankford, S. E., Adams, T. E., Miller, R. A., and Cech Jr, J. J. (2005). The cost of chronic stress: impacts of a nonhabituating stress response on metabolic variables and swimming performance in sturgeon. Physiol. Biochem. Zool. 78, 599–609. doi: 10.1086/430687
Lighthill, M. J. (1975). Mathematical Biofluiddynamics. Philadephia, PA: Society for Industrial and Applied Mathematics.
Long, J., Hale, M., McHenry, M., and Westneat, M. (1996). Functions of fish skin: flexural stiffness and steady swimming of longnose gar, Lepisosteus osseus. J. Exp. Biol. 199, 2139–2151.
Luther, D., and Baptista, L. (2010). Urban noise and the cultural evolution of bird songs. Proc. R. Soc. B Biol. Sci. 277, 469–473. doi: 10.1098/rspb.2009.1571
Marzluff, J. M. (2001). “Worldwide urbanization and its effects on birds,” in Avian Ecology and Conservation in an Urbanizing World, eds, J. M. Marzluff, R. Bowman, and R. Donnelly (Boston: Springer), 19–47.
Mcdonald, R. I., Kareiva, P., and Forman, R. T. T. (2008). The implications of current and future urbanization for global protected areas and biodiversity conservation. Biol. Conserv. 141, 1695–1703. doi: 10.1016/j.biocon.2008.04.025
McHenry, M. J., Pell, C. A., and Long, J. H. J (1995). Mechanical control of swimming speed: stiffness and axial wave form in undulating fish models. J. Exp. Biol. 198, 2293–2305.
McKinney, M. L. (2008). Effects of urbanization on species richness: a review of plants and animals. Urban Ecosyst. 11, 161–176. doi: 10.1007/s11252-007-0045-4
Merilä, J., and Hendry, A. P. (2014). Climate change, adaptation, and phenotypic plasticity: the problem and the evidence. Evol. Appl. 7, 1–14. doi: 10.1111/eva.12137
Miranda, A. C., Schielzeth, H., Sonntag, T., and Partecke, J. (2013). Urbanization and its effects on personality traits: a result of microevolution or phenotypic plasticity? Glob. Chang. Biol. 19, 2634–2644. doi: 10.1111/gcb.12258
Nelson, J. A., Gotwalt, P. S., Simonetti, C. A., and Snodgrass, J. W. (2008). nvironmental correlates, plasticity, and repeatability of differences in performance among blacknose dace (Rhinichthys atratulus) populations across a gradient of urbanization. Physiol. Biochem. Zool. 81, 25–42. doi: 10.1086/523304
Nelson, J. A., Gotwalt, P. S., and Snodgrass, J. W. (2003). Swimming performance of blacknose dace (Rhinichthys atratulus) mirrors home-stream current velocity. Can. J. Fish. Aqua. Sci. 60, 301–308. doi: 10.1139/f03-023
Nicoletto, P. F. (1995). Offspring quality and female choice in the guppy, Poecilia reticulata. Anim. Behav. 49, 377–387. doi: 10.1006/anbe.1995.0050
Partecke, J., and Gwinner, E. (2007). Increased sedentariness in European Blackbirds following urbanization: a consequence of local adaptation? Ecology 88, 882–890. doi: 10.1890/06-1105
Paul, M. J., and Meyer, J. L. (2001). Streams in the urban landscape. Annu. Rev. Ecol. Syst. 32, 333–365. doi: 10.1146/annurev.ecolsys.32.081501.114040
Pease, J. E., Grabowski, T. B., Pease, A. A., and Bean, P. T. (2018). Changing environmental gradients over forty years alter ecomorphological variation in Guadalupe Bass Micropterus treculii throughout a river basin. Ecol. Evol. 8, 8508–8522. doi: 10.1002/ece3.4349
Pigliucci, M. (2001). Phenotypic Plasticity: Beyond Nature and Nurture. Baltimore, MD: Johns Hopkins University Press.
Poff, N. L., Bledsoe, B. P., and Cuhaciyan, C. O. (2006). Hydrologic variation with land use across the contiguous United States: geomorphic and ecological consequences for stream ecosystems. Geomorphology 79, 264–285. doi: 10.1016/j.geomorph.2006.06.032
Räsänen, K., and Kruuk, L. E. B. (2007). Maternal effects and evolution at ecological time-scales. Funct. Ecol. 21, 408–421. doi: 10.1111/j.1365-2435.2007.01246.x
Reznick, D., Callahan, H., and Llauredo, R. (1996). Maternal effects on offspring quality in poeciliid fishes. Am. Zool. 36, 147–156. doi: 10.1093/icb/36.2.147
Schiffers, K., Bourne, E. C., Lavergne, S., Thuiller, W., and Travis, J. M. J. (2012). Limited evolutionary rescue of locally adapted populations facing climate change. Philos. Trans. R. Soc. B Biol. Sci. 368, 20120083–20120083. doi: 10.1098/rstb.2012.0083
Smith, T. B., Kinnison, M. T., Strauss, S. Y., Fuller, T. L., and Carroll, S. P. (2014). Prescriptive evolution to conserve and manage biodiversity. Annu. Rev. Ecol. Syst. 45, 1–22. doi: 10.1146/annurev-ecolsys-120213-091747
Steinhausen, M. F., Steffensen, J. F., and Andersen, N. G. (2005). Tail beat frequency as a predictor of swimming speed and oxygen consumption of saithe (Pollachius virens) and whiting (Merlangius merlangus) during forced swimming. Marine Biol. 148, 197–204. doi: 10.1007/s00227-005-0055-9
Storey, J. D. (2003). The positive false discovery rate: a Bayesian interpretation and the q-value. Ann. Stat. 31, 2013–2035. doi: 10.1214/aos/1074290335
Walker, J. A. (2010). An integrative model of evolutionary covariance: a symposium on body shape in fishes. Integr. Comp. Biol. 50, 1051–1056. doi: 10.1093/icb/icq014
Walsh, C. J., Roy, A. H., Feminella, J. W., Cottingham, P. D., Groffman, P. M., and Morgan, R. P. (2005). The urban stream syndrome: current knowledge and the search for a cure. J. North Am. Benthol. Soc. 24, 706–723. doi: 10.1899/04-028.1
Washburn, G. N. (1948). Propagation of the creek chub in ponds with artificial raceways. Trans. Am. Fish. Soc. 75, 336–350. doi: 10.1577/1548-8659(1945)75[336:POTCCI]2.0.CO;2
Webb, P. W., Kostecki, P. T., and Stevens, E. D. (1984). The effect of size and swimming speed on locomotor kinematics of rainbow trout. J. Exp. Biol. 109, 77–95.
Wenger, S. J., Roy, A. H., Jackson, C. R., Bernhardt, E. S., Carter, T. L., Filoso, S., et al. (2009). Twenty-six key research questions in urban stream ecology: an assessment of the state of the science. J. North Am. Benthol. Soc. 28, 1080–1098. doi: 10.1899/08-186.1
Worm, B., Hilborn, R., Baum, J. K., Branch, T. A., Collie, J. S., Costello, C., et al. (2009). Rebuilding global fisheries. Science 325, 578–585. doi: 10.1126/science.1173146
Wu, T. Y.-T. (1971). Hydromechanics of swimming propulsion. Part 1. Swimming of a two-dimensional flexible plate at variable forward speeds in an inviscid fluid. J. Fluid Mech. 46, 337–355. doi: 10.1017/S0022112071000570
Keywords: biomechanics, contemporary evolution, Creek Chub, land use, phenotypic plasticity, Semotilus atromaculatus, urbanization, whole-organism performance
Citation: Kern EMA and Langerhans RB (2019) Urbanization Alters Swimming Performance of a Stream Fish. Front. Ecol. Evol. 6:229. doi: 10.3389/fevo.2018.00229
Received: 23 May 2018; Accepted: 07 December 2018;
Published: 07 January 2019.
Edited by:
Susan C. Walls, United States Geological Survey, United StatesReviewed by:
Sean Lema, California Polytechnic State University, United StatesMichael J. Pauers, Milwaukee Public Museum, United States
Jay A. Nelson, Towson University, United States
Copyright © 2019 Kern and Langerhans. This is an open-access article distributed under the terms of the Creative Commons Attribution License (CC BY). The use, distribution or reproduction in other forums is permitted, provided the original author(s) and the copyright owner(s) are credited and that the original publication in this journal is cited, in accordance with accepted academic practice. No use, distribution or reproduction is permitted which does not comply with these terms.
*Correspondence: Elizabeth M. A. Kern, ZWtlcm5AZXdoYS5hYy5rcg==
†Present Address: Elizabeth M. A. Kern, Department of Life Science, Ewha Womans University, Seoul, South Korea