- 1Department of Biology, McGill University, Montréal, QC, Canada
- 2Department of Zoology, Entomology and Fisheries Sciences, College of Natural Sciences, Makerere University, Kampala, Uganda
Aquatic ecosystems in tropical regions remain understudied and their long-term dynamics poorly understood. In East Africa, a better understanding of how natural communities of primary producers in small freshwater ecosystems respond to climatic variability is needed to improve management and conservation of aquatic resources. This study explored the response of algae and bacteria communities to marked hydrological variation over the past 1,500 years in a small western Ugandan crater lake, Lake Nkuruba. We analyzed sedimentary algal and bacterial pigments to evaluate the magnitude and direction of change in the autotrophic community in response to severe climatic perturbations in the region. The lithology of the Lake Nkuruba sediment core indicated that external forcing in the form of a major drought, associated with the Medieval Climate Anomaly, caused a heavy, short-lived detrital pulse to the basin that led to a brief but substantial disruption of the lake system in the second half of the Thirteenth century. The system appears to have recovered rapidly, and then transitioned to a more productive state than the one preceding the drought. The considerable variation observed in the sedimentary pigment biomarkers is likely linked with climatically-induced changes in the water column structure of this small crater lake. Our results highlight the challenge of defining appropriate baselines or reference conditions in climatically-sensitive East African aquatic ecosystems and disentangling long-term anthropogenic impacts from the strong regional hydrological flux at the decadal to centennial scale.
Introduction
Small lakes can be hotspots of biodiversity (Strayer and Dudgeon, 2010) and are critical to ecosystem service delivery; however, they remain some of the least investigated freshwater systems globally and are largely excluded from management planning (Biggs et al., 2017). This is particularly true in tropical regions, where small lakes are abundant on the landscape and are of critical importance to local human populations (Lewis, 2000), yet we still know very little about how they function and respond to global change, making it difficult to manage them responsibly. In western Uganda, one of the world's fastest growing and most vulnerable populations (Hartter et al., 2012) rely on small crater lakes to provide essential ecosystem services including water for domestic use and livestock and nourishment (source of protein) in the form of fish. Saulnier-Talbot et al. (2014) showed that these small tropical crater lakes can be very sensitive to climatic changes at the annual and decadal scale and provided a perspective of Twentieth century variability in crater Lake Nkuruba. For example, they found that temperature-driven increases in water column stability can have detrimental consequences for primary production, such as the expansion of the anoxic zone, which may limit the ability of lakes to support adequate fish populations and increase the proliferation and blooms of cyanobacteria (Saulnier-Talbot et al., 2014). To improve management, restoration, and conservation of these vital ecosystems, a more in-depth understanding of their dynamics on longer timescales is necessary, with a particular focus on identifying the timing and magnitude of the principal drivers of regional environmental change (Gelorini and Verschuren, 2013).
Ecosystem sustainability requires that evolutionary and historical ecological processes inherent to the given system are maintained. Therefore, it is vital to understand these processes and to define the most appropriate baselines for evaluating the magnitude and direction of current ecological changes. The study of lake sediment archives provides a vital perspective that contributes to making informed predictions on how ecosystems will respond to current and future environmental change based on extrapolations of how they reacted to disturbances in the past (e.g., Saulnier-Talbot, 2016). Previous studies conducted on sediment cores from western Ugandan crater lakes have shown that the paleolimnological approach is key to determining the magnitude and direction of ecological shifts in these lakes and their catchments relative to environmental change and human pressures (e.g., Ssemmanda et al., 2005, Ryves et al., 2011, Gelorini and Verschuren, 2013, Mills et al., 2014, Kiage et al., 2017). Here, we follow this approach and combine the analysis of lithological attributes with our study of sedimentary algal and bacterial pigments, to reconstruct long-term variation in the phototrophic community structure of a small crater lake located in the vicinity of Kibale National Park, western Uganda.
Sedimentary pigments, when well-preserved, are a particularly informative proxy of the response of lake production and primary producer composition to disturbances linked to climatic change, including water column stratification, and water depth and chemistry (e.g., McGowan et al., 2005). Several studies have shown that sedimentary pigments represent entirely plausible and reliable indicators of past environmental change at decadal to millennial scales in African lakes (e.g., Verschuren et al., 1999; Barker et al., 2000, Meyer et al., 2018). Because of its substantial depth, low transparency, strongly stratified water column, and thick anoxic hypolimnion (Chapman et al., 1998, Saulnier-Talbot et al., 2014), pigments should be well-preserved in the sediments of Lake Nkuruba. As such, the objective of this study was to use the long-term archive of algal and bacterial pigments to assess the response of the lacustrine autotrophic community to known, marked regional climatic perturbations over the past ~1,500 years.
Materials and Methods
Lake Nkuruba (0°31'00.85“N 30°18'11.06”E) is a small (3 ha), moderately deep (max z = 38 m, mean z = 16 m), slightly alkaline (pH = 8), freshwater lake (mean surface conductivity = 360 μS cm−1). It is sheltered from the wind by high forested walls that average 48 m above current water level. The lake has no known surface inflow or outflow and is likely fed solely by rain. It is therefore sensitive to hydrological variations. The presence of fissures within the lake's basin and exchange with groundwater cannot be excluded but is yet to be confirmed, although the region is known to be seismically active. Aquatic macrophytes are rare in the lake because the steep crater walls do not allow for much shallow littoral area. Extended monitoring of the lake (1992–2011) revealed a generally strongly stratified water column, albeit with occasional atelomixis, and even some rare complete turnover events allowing for the redistribution of nutrients into the system (Chapman et al., 1998, Saulnier-Talbot et al., 2014). Surface water temperature is relatively stable and averages around 23°C (Saulnier-Talbot et al., 2014), whereas surface dissolved oxygen concentrations are highly variable, ranging between 6 and 180% saturation (mean = 6.3 mg L−1; Efitre et al., 2016). The lake's small catchment is currently forested with mature trees thanks to conservation efforts deployed since the early 1990s. A nature reserve and community ecotourism campsite are established in the catchment. As such, this lake is of distinct ecological and human value for the region.
Nkuruba is located at an altitude of 1,520 m and is part of a group of around 90 crater lakes with highly variable conductivities that dot the landscape in southwestern Uganda. These volcanic explosion craters are thought to be of Late Pleistocene age and are associated with tectonic activity in the western branch of the East African rift system (Ssemmanda et al., 2005; Russell et al., 2007). The region sits on the Toro belt, which is a complex geological mosaic consisting of gneisses interlayered with micaeous schists, amphibolites, quartzites, and calcsilicates and partly covered with rift sediments (Link et al., 2010). The regional climate is tropical sub-humid and is under the influence of the Intertropical Convergence Zone (ITCZ) (Nicholson, 1996). It displays a seasonally bimodal rainfall pattern consisting of two rainy seasons (March-May and October-December) and two dry seasons (June-September and January-February) (Chapman et al., 2010). Air temperatures average around 20°C throughout the year.
Two sediment cores were retrieved from the middle area of the lake (Figure 1): in January 2008, a 27 cm short core was retrieved using a gravity corer (see results in Saulnier-Talbot et al., 2014); and in January 2009, a 150 cm-long core was retrieved using a percussion corer. The water-sediment interface was intact in both cores and even if slight degassing occurred, it did not affect the stratigraphical integrity of the sequences (as observed through the clear plastic tubes used for coring). The cores were examined visually, and their lithology was described before they were extracted vertically on site into 1 cm increments. At all times during sediment processing, care was taken not to expose the sediments to direct sunlight (which could risk degrading sedimentary pigments). Samples were kept in the dark and frozen until analyzed at McGill University's Department of Biology (Montréal, Canada) in the 2–3 months following fieldwork.
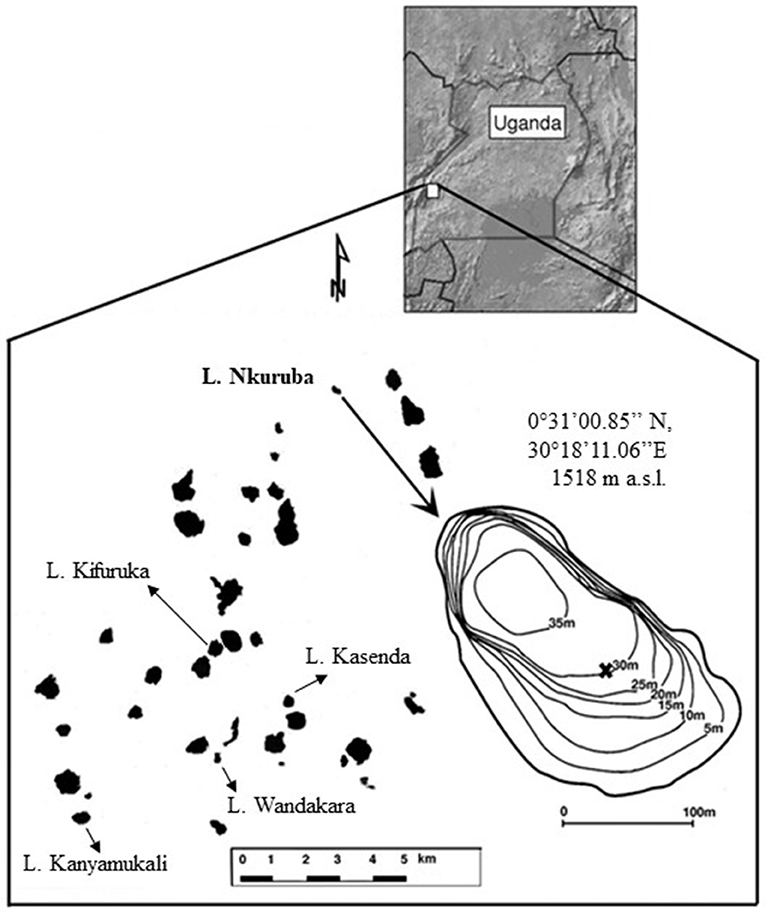
Figure 1. Location of the study lake within Uganda, position in relation to other nearby crater lakes (in black) and bathymetry of lake Nkuruba with location of the coring site (marked by X). Modified from (Saulnier-Talbot et al., 2014).
We combined the chronology of a previously-dated short core (Saulnier-Talbot et al., 2014) with the longer sediment record from Lake Nkuruba. The short core chronology was based on the measurement of 210Pb, 214Pb, and 214Bi in 16 core intervals using gamma spectrometry, and the constant rate of supply (CRS) model was applied to establish age-depth relationships (see Saulnier-Talbot et al., 2014 for results). Wiggle-matching of the LOI data from both cores suggested that 18 cm depth in the long core corresponded to 1910 CE, so we use this as an additional chronological marker. Three wood fragments retrieved from the long core were prepared at the Centre d'études nordiques' Radiochronology Lab (Université Laval, Canada) and dated using AMS 14C at the KECK Carbon Cycle (University of California, Irvine, USA). All 14C dates (Table 1) were calibrated using Calib Rev 7.0.4. (©Stuiver et al., 1986-2014) and are presented in the text as common era (CE) ages. We used the Bacon method in R (Blaauw and Christen, 2011) to construct an environmentally-realistic Bayesian age-depth model with a limited number of data points.
In the months following fieldwork, sediment samples were weighed, lyophilized, and weighed again to measure their water content. The organic and minerogenic content was determined using the loss on ignition technique (Heiri et al., 2003). Sedimentary pigment analysis was conducted at 2 cm intervals throughout the long core in April 2009 (for a total of 75 samples), following the method described in Saulnier-Talbot et al. (2014). Briefly, pigments were extracted in 100% acetone from pre-weighed samples of freeze-dried sediment, pre-processed by sonication in an ice bath for heat dissipation, purged of oxygen/air, and placed in an atmosphere of argon for 24 h at −20°C. Aliquots were analyzed using high-performance liquid chromatography (Zapata et al., 2000) (Waters HPLC, model no 600/626 with a Waters Photodiode Array 2996, a Waters 2475 Multi λ Fluorescence detector and a refrigerated Waters autosampler 717). Individual pigments were identified and quantified using external standards, and sedimentary pigment concentrations were calibrated relative to organic matter content (OM) of the sediment. Downcore sedimentary pigment preservation was conservatively evaluated by calculating the ratio Chl-a: total pheopigments (Leavitt and Hodgson, 2001). Most pigment data (except diatoxanthin, echinenone, and lutein) were square-root-transformed to normalize variance.
Stratigraphically constrained cluster analysis using unweighted pair-group average clustering (UPGMA; Legendre and Birks, 2012) and Chord similarity measure was implemented with the software Past (Hammer et al., 2001) on the pigment data to determine zonation through time. The core section corresponding to the high sedimentation event (114–74 cm depth) was lumped into one zone (P-3), which included the highest cophenetic distances (>0.3; see Supplementary Material). Other separations were also grouped (zones P-1, P-4, and P-5) at depths corresponding to concurrent changes in both pigment assemblages and lithological changes (see below). Stratigraphical diagrams were plotted using the software C2 (Juggins, 2011).
Results
The age-depth relationship determined for the Nkuruba long core was generated with the Bacon method (Blaauw and Christen, 2011) (Figure 2). The date obtained from the bottom of the long core indicates that it covers the last ~1,500 years, going back to the Sixth century CE. The two other 14C dates bracketed the beginning and the end of the dry sediment interval (Figure 3), placing it in the second half of the Thirteenth century. The oldest section of the core (L-1) had the slowest sedimentation rate (0.03 cm/yr), whereas the subsequent section (L 2-3) had an estimated high sedimentation rate of 1.5 cm/yr. Following this anomaly, sedimentation rates returned to a slower rate of 0.09 cm/yr (L 4-5). The topmost section of the core (L-6), representing the last ~100 years had an estimated accumulation rate of 0.18 cm/yr.
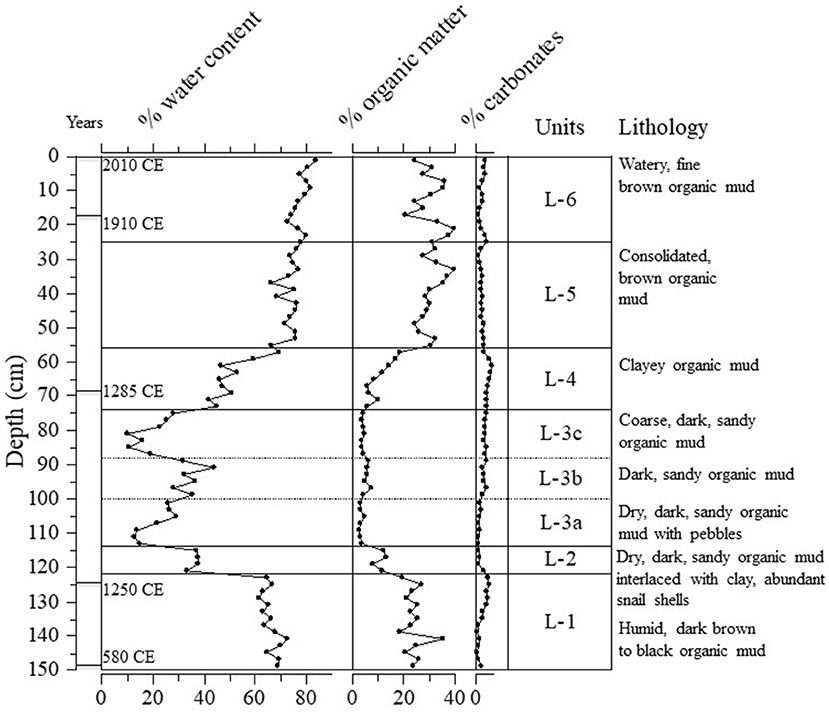
Figure 3. Sedimentary characteristics of the Nkuruba sediments used to determine the physical stratigraphical units of the core.
Sedimentary characteristics revealed a series of heterogeneous facies (distinctive units of material) differing substantially in water, organic, and carbonate content. Zonation of the long core based on observations of compositional changes in the sediment, resulted in 6 distinct lithological units (Figure 3). Units L-1, L-5, and L-6 contained the highest moisture and organic matter (OM) content, whereas units L-2, L-3, and L-4 were generally very dry and minerogenic. Unit L-3 was further divided into three sub-zones because of some notable variations in water content and lithology. The water content in the sediments varied between 10 and 80%, and OM varied between 3 and 40%. Comparatively, carbonate content varied less (between 1 and 6%) but was highest at the top of Unit L-1 and in L-4. Table 2 presents the stratigraphical position and approximate duration for each of the zones.
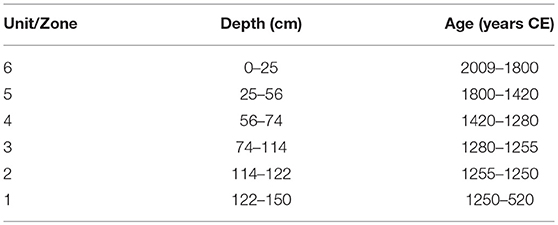
Table 2. Stratigraphical position (cm) and approximate duration (years CE) of lithological units (determined visually based on sedimentological characteristics) and pigment zones (determined using cluster analysis and matched with lithological zones) identified in the Nkuruba long core.
Results from the constrained cluster analysis on the pigment data showed that the greatest dissimilarities between downcore sediment pigment assemblages were located in the zone of rapid sediment accumulation, in the interval 1250–1285 CE (Figure 4 and Supplementary Materials), corresponding to Zones P-2 and P-3. Other transition periods identified through this analysis were located around 1420 and 1800 CE. The ratio of Chl-a: total pheopigments was highly stable throughout the sequence, except for a few punctuated deviations at the beginning of P-3 and in P-6 (Figure 4 and Supplementary Figure 2).
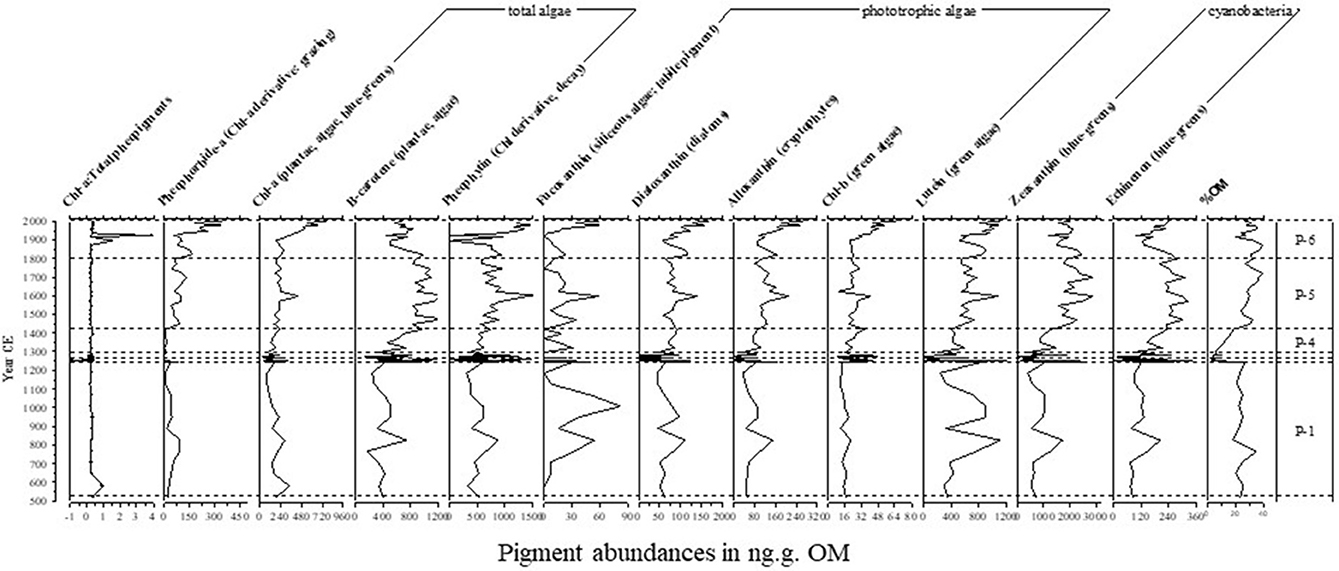
Figure 4. Temporal variation in the concentration of the most common sedimentary algal and bacterial pigments in Lake Nkuruba (all pigments expressed as ng of pigment per g organic weight of sediment). Zones identified using the UPGMA clustering approach (see Supplementary Figure 1) and matched with lithological zones (Figure 3).
Zone P-1 (520–1250 CE) shows variable levels of pigments, with a general peak around 830 CE, which in not synchronous with a peak in OM values that occurred around 770 CE. In zone P-2 (1250–1255 CE), there is a rapid increase in all pigment concentrations, which could indicate higher overall production in the lake in this short period of time. Zones P-2 and P-3 correspond to the dry sediment interval (L2 and L-3) that occurred between ~1250–1280 CE. Zone P-3 is marked by the highest variability for all pigments, except for Pheophytin (see Table 3) and some strong declines in numerous pigments, which could be the result of the important increase in sediment accumulation during this period. In zone P-4 (1280–1420 CE), most pigments showed a recovery with an increasing trend. Zone P-5 (1420–1800 CE) contained higher than average concentrations of pigments, especially those associated with cyanobacteria (with respect to the 1,500 year record). Zone P-6 (1800 CE-present) showed stable values for most pigments followed by a general increase in the topmost section of the core. However, pigments in the core top, which were more recently deposited and had not undergone the same degree of diagenesis as in the rest of the sequence when it was extracted from the lake, are deemed less representative of relative change (Guilizzoni and Lami, 2002).

Table 3. Coefficient of variation estimates (standard deviation/mean*100) for each pigment in each zone.
Discussion
The lithology and biomarker components of the Nkuruba sediment archive indicated clear environmental variability over the past 1,500 years. In particular, there was a two-fold change in sediment water content accompanied by a temporary shift from organic mud to a matrix comprised of sand and pebbles during the second half of the Thirteenth century CE. Changes in the algal and bacterial community followed a similar trajectory, with substantial variations in pigment concentrations and the loss of labile indicators at that point in time. Our interpretation of this record, set in a regional context, is one of an ecosystem strongly driven by regional hydrological changes: the occurrence of an intense late medieval drought that disrupted the system considerably.
Analyzing Nkuruba's pigment record is complex because variations in pigment abundances are influenced by many factors, including pigment preservation, changes in sedimentation rate, and the compression of sediments with time; all of which must be interpreted carefully. Our coarse age-depth model for this core precludes the calculation of reliable pigment fluxes. However, our results suggest a three-fold increase in sedimentation rate (from 0.03 to 0.09 cm/year) between the pre- and post-Thirteenth century periods. Roughly, this would translate into even greater differences in pigment concentrations between pre-13th and post- Thirteenth century levels, with indications of the system being much more productive post- Thirteenth century. Anthropic disturbances could also potentially explain some of the variation in this lake's past dynamics (Mills et al., 2016), as human impacts in the region are perceptible as far back as 4,000 years ago (see Kiage et al., 2017). Although there is no available information on direct historical anthropogenic impacts at our study site beyond the early Twentieth century (Saulnier-Talbot et al., 2014), charcoal and pollen content of a sediment core from nearby (< 10 km away) Lake Kifuruka suggest that fires became more frequent, and vegetation changes reflected increased human disturbance in the catchment of that lake over the past 2,000 years (Kiage et al., 2017). Moreover, Russell et al. (2009) found that nearby Lake Wandakara (< 15 km away) was more sensitive to human disturbances in its catchment than to climatic change over the past 2,000 years, with considerable biogeochemical changes that even led to increased eutrophication. It is therefore likely that a similar scenario played-out in the vicinity of Nkuruba and in its basin, but the timing and magnitude of anthropic perturbations remain unknown, and we cannot currently link any human factors to the long-term past dynamics of Nkuruba. It is reasonable to suspect that deforestation in the lake's catchment could have exacerbated the effects of drought. We can use the Twentieth century record to support this argument as we know that during this time period the watershed was deforested, and pig farming was present, and yet we do not see changes in the lithology over the past 100 years that are nearly as dramatic as what was observed between 1250–1285 CE.
In its current state, Lake Nkuruba has low light penetration (Secchi depth ≈2 m) and a thick anoxic layer, that usually extends over about two thirds of the water column (Saulnier-Talbot et al., 2014); these factors contribute to the preservation of algal and bacterial pigments as they are deposited at the water-sediment interface and are eventually buried (see Leavitt, 1993, for review). Thus, despite high water temperatures (around 22°C), the relatively deep basin of Nkuruba renders it more resistant to drought than other, shallower crater lakes in the region and makes it a good candidate for the investigation of sedimentary pigment records. In fact, even pigments considered to be very labile, such as chlorophylls, fucoxanthin, and pheophorbide, appear to be well-preserved throughout this core, even into the oldest (>1,000 years) sediments. However, the major changes observed in the core sedimentology suggest that ideal conditions for the preservation of pigments were not always present throughout the sequence. The water and organic matter contents in zones L-2 and L-3 suggest some disturbance in the system that could potentially have affected pigment preservation temporarily in the corresponding zones P-2 and P-3 (1250–1280 CE).
The very base of the Nkuruba core likely corresponds to the period known in Europe and Asia as the Late Antique Little Ice Age (LALIA; Büntgen et al., 2016), which straddles the end of Antiquity and the beginning of the Middle Ages (500–650 CE). Understanding of climate change during this ~200 year period in Africa is still limited, and it is not currently known if temperatures cooled at that time, as in Europe and Asia. Except for an absence of the very labile pigment fucoxanthin, there is little indication in the Nkuruba sedimentary pigment record that could potentially reflect the effects of the LALIA, such as decreased lake levels reported from lakes Edward, Challa, and Tanganyika around that time (Nash et al., 2016).
Sedimentological markers indicated relatively stable water and OM content of the sediment throughout zone L-1. Chlorophylls and their derivatives also remained relatively stable in zone P-1, but many pigments showed a peak around 140 cm depth (corresponding to c. 830 CE), suggesting that primary production was higher during that interval. This time-period corresponds to a well-documented episode of higher than average rainfall in East Africa, inferred from diverse proxies in several lakes, including lakes Edward (c. 900–1000 CE), Tanganyika (c. 800–850 CE), and Naivasha (c. 900 CE) (reviewed in Nash et al., 2016). It is therefore conceivable that the thickness of the oxygenated epilimnion during the period preceding the Medieval Climate Anomaly (MCA) could have varied due to higher rainfall and that occasional turnover events allowing for the resuspension of nutrients were a likely occurrence that would have stimulated growth. Conditions prevalent in this oldest section of the record seem to have been especially profitable to the phototrophic algae, and particularly the siliceous and green algae.
A recent review of continent-wide Holocene climate in Africa (Nash et al., 2016) highlights that the Middle Ages were a period of increasing aridity in Eastern Africa, culminating in the climax drought of the end of the MCA. Lake Edward, to the southwest of Nkuruba, was at its lowest level around 1050–1200 CE (Russell and Johnson, 2005); whereas in Lake Victoria, to the southeast of Nkuruba, dryer-than-average (Holocene) conditions peaked during a 100 year episode centered on 1200 CE (Stager et al., 2005). In other, shallower Ugandan crater lakes, located south of Nkuruba, drought appears to have peaked during the second half of the Twentieth century CE (Russell et al., 2007, 2009, Ryves et al., 2011; Mills et al., 2014). Nkuruba, as a rain-fed lake, is sensitive to hydrological variations and would still be sensitive to an intense drought despite its relatively deep basin. Sedimentological indicators from our study core indicate that in the Nkuruba catchment, the drought appears to have culminated in the second half of the Thirteenth century, slightly later than in the previously-investigated lakes in the region (Figure 5). This peak coincides remarkably well with the 1257 AD eruption of the Samalas volcano (Indonesia), considered to be one of the strongest of the past 2500 years (Swingedouw et al., 2017) and linked to short, but global climatic disturbances (Guillet et al., 2017). Given the much greater depth of Nkuruba, compared with the other investigated crater lakes in the region (Figure 5), it is possible that it only recorded the end of the drought, perhaps exacerbated by the Samalas eruption. Better chronological control of sediment cores from the region, in general, would improve our understanding of the apparent regional differences in the timing of maximum drought intensity.
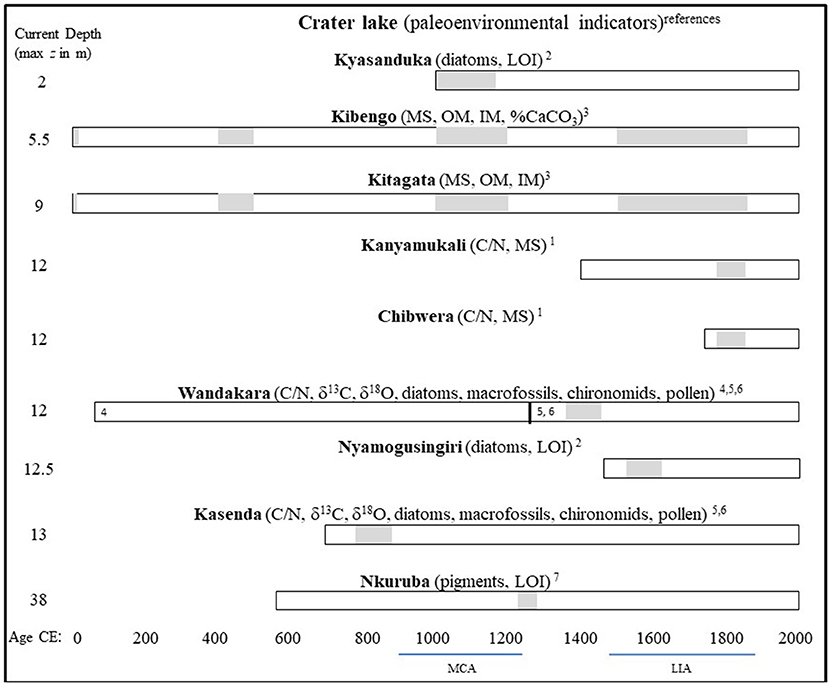
Figure 5. General timing of inferred drought events (plain gray shading) from various paleoenvironmental indicators in the sediments of western Ugandan crater lakes over the past 2000 years. Indicator abbreviations are as follows: LOI, loss on ignition; MS, magnetic susceptibility; OM, organic matter; IM, inorganic matter; C/N, carbon to nitrogen ratio. Exponents refer to the published literature: (1) (Bessems et al., 2008), (2) (Mills et al., 2014), (3) (Russell et al., 2007), (4) (Russell et al., 2009), (5) (Ryves et al., 2011), (6) (Ssemmanda et al., 2005), (7) this study. Lakes Kanyamukali, Wandakara, Kasenda, and Nkuruba are part of the Kasenda cluster and their locations are indicated in Figure 1. The other lakes are located in the Katwe-Kikorongo and Bunyaruguru clusters, about 60–70 km south-west of the Kasenda cluster, between lakes Edward and George. This southern region is drier, and those lakes have higher conductivities.
Around 1250 CE, there was a major perturbation in Nkuruba's environment shown by a drastic and sudden decrease in the core's water and OM content (unit L-2). This section of the sediment archive was unique in its composition as it contained abundant remains of snail shells, which were absent or rare from the other sections (although snails are currently present in the lake, especially in the shallow littoral area), indicating that peak favorable conditions for these organisms were only attained during this short interval. For snails to inhabit Nkuruba's system in such numbers, the water level would have presumably been low at the time, exposing a wider littoral zone in the shallower, southeastern region of the lake's basin (Figure 1) and providing adequate habitat for the snails. In turn, a shallow lake would have been less conducive to the preservation of pigments, possibly resulting in the generally lower and erratic pigment concentrations seen in zone P-3.
Following unit L-2, the massive dry facies of unit L-3 was deposited into the lake, likely originating from the parched soils and vegetation-depleted walls of the crater. Although the accumulation in this zone of the Nkuruba core was very rapid, a single slumping event is unlikely because of the presence of a well-delimited lithological sub-zonation in this section (a similar zonation occurs with the pigments, as can be seen in Supplementary Figure 2). A similar decrease in water and organic matter content values to extremely low levels was reported by Kiage et al. (2017) in a core extruded in 2014 from Lake Kifuruka (mean z = 5 m), located < 10 km away from Nkuruba. However, the dry period in the Lake Kifuruka core, based on 14C dating of leaf fragments, was about 1,200 years older than the dry period captured in the Lake Nkuruba core. This earlier timing of intense drought in Kifuruka would coincide with what is considered likely to be the driest period of the middle-late Holocene, at the start of the common era (Nash et al., 2016). The sediment archives of other nearby crater lakes should be investigated to see if these dry facies have been recorded throughout the region and if they correspond to one or more droughts.
After the end of the MCA, there is evidence that there was a return to moister hydroclimatic conditions in the region, with humidity reaching its maximum between 1200 and 1500 CE (Nash et al., 2016). In unit L-4 and zone P-4 (1280–1420 CE) the water and OM content of the sediment and the algal and bacterial assemblage reverted to pre-thirteenth-century conditions within about 100–150 years. These results illustrate the high resilience of the primary trophic level in Nkuruba's ecosystem following an extreme climatic event.
Drier conditions returned to parts of East Africa after 1500 CE, with evidence from the Lake Edward record suggesting that the climate reverted to conditions comparable to those of the peak MCA drought (Russell and Johnson, 2007). A trend toward declining rainfall during the LIA was also observed in other Ugandan crater lakes (Bessems et al., 2008). However, lithological characteristics in the Nkuruba record do not point to low humidity in unit L-5 (1420–1800 CE). Organic matter content even reached some of its highest values of the last 1,500 years in this zone, reflecting high autochthonous (and also potentially allochthonous) production. The highly variable pigment record in zone P-5 (1420–1800 CE) does not point unambiguously toward either drier or wetter conditions. The dominant cyanobacterial component of the assemblage could suggest higher temperatures, lower lake levels and especially increased stratification of the water column. In fact, many cyanobacteria taxa achieve maximum growth rates at elevated temperatures (e.g., Paerl et al., 2011), which explains why they are a major component of the biota in tropical lakes. They also out-perform other phototrophs in highly stable water columns because many taxa can effectively regulate buoyancy. However, our previous findings about the recent dynamics of the study lake (Saulnier-Talbot et al., 2014) established that the algal and bacterial community in Lake Nkuruba, including cyanobacteria, is currently very sensitive to increasing temperature and the associated stability metrics of the water column (and also to extreme rainfall events, changes in land-use in the catchment, and possibly to fish population fluctuations). Currently, in Lake Nkuruba, primary production (with a signal strongly linked to concentrations of pigments such as β-carotene, lutein, alloxanthin, and echinenone) is highly positively correlated with depth to anoxia. This translates into higher sedimentary pigment concentrations when the thickness of the mixed surface layer is greater (i.e., deeper mixing). Therefore, the more productive zones P-5 and P-6 of the long core could correspond to periods of generally greater depth to anoxia in the past. A thicker oxygenated epilimnion in a crater lake that is protected from the wind by high walls can be the result of various environmental factors, including cooler temperatures and cloudier conditions, which favor turbulent mixing. The inferred enhanced primary production could also be the result of cultural eutrophication, as was reported by Russell et al. (2009) for nearby Lake Wandakara. We consider it likely that zone P-5 corresponds to the Little Ice Age, which has been dated to around 1500–1800 CE in westernmost East Africa (Russell et al., 2007).
Zone P-6 is a period of high primary production of phototrophic algae, along with decreased concentrations of cyanobacterial pigments relative to the main-phase LIA. The more highly resolved record of the sedimentary pigments of this period, analyzed from the short core taken in Nkuruba (Saulnier-Talbot et al., 2014) indicated that primary production over the last century had been highly variable within the context of that timeframe (about 100 years). It was found that the Twentieth century changes were linked to human-induced disturbances in the lake and its catchment, including the introduction of fish, along with deforestation, and pig farming in the catchment. The hindsight provided by the 1,500 year record from the long core now enables the contextualization of the recent variability in the lake's dynamics. Placed in a longer historical context, the recent decadal-scale changes in the Nkuruba pigment concentrations are dwarfed by the higher-amplitude changes during the past 1,500 years. However, the most recent accumulation in the Nkuruba core does show some of the highest pigment concentrations of the past 1,500 years, even without considering values from the surface sediment (which could be misleading due to differential degradation).
Conclusion
By providing insight into the past magnitude and direction of changes in lake primary producers, sediment archives improve our capacity to evaluate community response and resilience to severe climatic perturbations. Based on a ~1,500 year sediment archive of Lake Nkuruba, we have demonstrated that this Ugandan crater lake underwent high-amplitude hydrological changes but that primary producers appear to have recovered rapidly. However, it remains unclear how ecosystem resilience will be affected by the combined pressures of climatic variability and increasing human pressures in the future. A further point illustrated by this study is the complexity of determining baseline conditions for lakes in East Africa, where climate over the past ~1,500 years has been highly variable and clearly had strong repercussions on climate-sensitive aquatic ecosystem dynamics.
Author Contributions
ES-T, LC, and IG-E conceived the ideas and designed the methodology; ES-T, JE, and KS collected the data; ES-T analyzed the data; ES-T and IG-E led the writing of the manuscript. All authors contributed critically to the drafts and gave final approval for publication.
Funding
This research was supported by grants from the National Science Foundation (NSF; http://www.nsf.gov/), the Wildlife Conservation Society (http://www.wcs.org/), the National Science and Engineering Research Council of Canada (NSERC; http://www.nserc-crsng.gc.ca/) and the Canada Research Chair in Respiratory Ecology to LC, and by NSERC and the Canadian Foundation for Innovation (CFI) grants to IG-E. ES-T benefitted from a postdoctoral fellowship from the Fonds québécois pour la Recherche sur la Nature et les Technologies (FQRNT; http://www.frqnt.gouv.qc.ca/) and from a grant for field work from the Percy Sladen Memorial Fund (http://www.linnean.org/The-Society/awards_and__grants/Awards_and_Grants). No individuals employed or contracted by the funders (other than the named authors) played any role in: study design, data collection, and analysis, decision to publish, or preparation of the manuscript. Permission and all necessary permits were obtained for the described study, which complied with all relevant regulations, from the appropriate Uganda government authorities (Uganda National Council for Science and Technology).
Conflict of Interest Statement
The authors declare that the research was conducted in the absence of any commercial or financial relationships that could be construed as a potential conflict of interest.
Acknowledgments
We thank Jovan Abwooli for his indispensable help with fieldwork and Keely Mills for fruitful discussions about the Ugandan crater lakes.
Supplementary Material
The Supplementary Material for this article can be found online at: https://www.frontiersin.org/articles/10.3389/fevo.2018.00223/full#supplementary-material
Supplementary Figure 1. Stratigraphically constrained cluster analysis of pigment data using unweighted pair-group average clustering (UPGMA; Legendre and Birks, 2012) with Chord similarity measure. The dark rectangle indicates the zones corresponding to the highest sedimentation rate in the core.
Supplementary Figure 2. Downcore pigment data presented on the depth (cm) scale showing splits corresponding to UPGMA clustering (>0.16 chord distance in Supplementary Figure 1).
References
Barker, P., Telford, R., Merdaci, O., Williamson, D., Taieb, M., Vincens, A., et al. (2000). The sensitivity of a Tanzanian crater lake to catastrophic tephra input and four millennia of climate change. Holocene 10, 303–310. doi: 10.1191/095968300672848582
Bessems, I., Verschuren, D., Russell, J. M., Hus, J., Mees, F., and Cumming, B. F. (2008). Paleolimnological evidence for widespread late 18th century drought across equatorial East Africa. Palaeogeogr. Palaeoclimatol. Palaeoecol. 259, 107–120. doi: 10.1016/j.palaeo.2007.10.002
Biggs, J., von Fumetti, S., and Kelly-Quinn, M. (2017). The importance of small water bodies for biodiversity and ecosystem services: implications for policy makers. Hydrobiologia 793, 3–39. doi: 10.1007/s10750-016-3007-0
Blaauw, M., and Christen, J. A. (2011). Flexible paleoclimate age-depth models using an autoregressive gamma process. Bayesian Anal. 6, 457–474. doi: 10.1214/11-BA618
Büntgen, U., Myglan, V. S., Charpentier Ljungqvist, F., McCormick, M., Di Cosmo, N., Sigl, M., et al. (2016). Cooling and societal change during the late antique little ice age from 536 to around 660 AD. Nat. Geosci. 9, 231–236. doi: 10.1038/ngeo2652
Chapman, C. A., Chapman, L. J., Jacob, A. L., Rothman, J. M., Omeja, P., Reyna Hurtado, R., et al. (2010). Tropical tree community shifts: implications for wildlife conservation. Biol. Conserv. 143, 366–374. doi: 10.1016/j.biocon.2009.10.023
Chapman, L. J., Chapman, C. A., Crisman, T. L., and Nordlie, F. G. (1998). Dissolved oxygen and thermal regimes of a Ugandan crater lake. Hydrobiologia 385, 201–211. doi: 10.1023/A:1003527016384
Efitre, J., Murie, D. J., and Chapman, L. J. (2016). Age validation, growth and mortality of introduced Tilapia zillii in Crater Lake Nkuruba, Uganda. Fish. Manage. Ecol. 23, 66–75. doi: 10.1111/fme.12163
Gelorini, V., and Verschuren, D. (2013). Historical climate-human-ecosystem interaction in East-Africa: a review. Afr. J. Ecol. 51, 409–421. doi: 10.1111/aje.12045
Guilizzoni, P., and Lami, A. (2002). “Paleolimnology: use of algal pigments as indicators,” in The Encyclopedia of Environmental Microbiology, ed G. Bitton (Chinchester: John Wiley and Sons), 2306–2317.
Guillet, S., Corona, C., Stoffel, M., Khodri, M., Lavigne, F., Ortega, P., et al. (2017). Climate response to the Samalas volcanic eruption in 1257 revealed by proxy records. Nat. Geosci. 10, 123–128. doi: 10.1038/ngeo2875
Hammer, Ø., Harper, D. A. T., and Ryan, P. D. (2001). PAST: paleontological statistics software package for education and data analysis. Palaeontol. Electr. 4:9pp. Available online at: http://palaeo-electronica.org/2001_1/past/issue1_01.htm
Hartter, J., Stampone, M. D., Ryan, S. J., Kirner, K., and Chapman, C. A. (2012). Patterns and perceptions of climate change in a biodiversity conservation hotspot. PLoS ONE 7:e32408. doi: 10.1371/journal.pone.0032408
Heiri, O., Lotter, A. F., and Lemcke, G. (2003). Loss on ignition as a method for estimating organic and carbonate content in sediments: reproducibility and comparability of results. J. Paleolimnol. 25, 101–110. doi: 10.1023/A:1008119611481
Juggins, S. (2011). C2 Version 1.7.7 Software for Ecological and Palaeoecological Data Analysis and Visualisation. Newcastle upon Tyne: Newcastle University.
Kiage, L. M., Howey, M., Hartter, J., and Palace, M. (2017). Paleoenvironmental change in tropical Africa during the Holocene based on a record from Lake Kifuruka, western Uganda. J. Quat. Sci. 32, 1099–1111. doi: 10.1002/jqs.2986
Leavitt, P. R. (1993). A review of factors that regulate carotenoid and chlorophyll deposition and fossil pigment abundance. J. Paleolimnol. 9, 109–127. doi: 10.1007/BF00677513
Leavitt, P. R., and Hodgson, D. A. (2001). “Sedimentary pigments,” in Developments in Paleoenvironmental Research, Vol. 3. Tracking Environmental Change Using Lake Sediments: terrestrial and siliceous indicators, eds J. P Smol, H. J. B Birks, W. M Last (Dordrecht: Kluwer Academic Publishers), 295–325.
Legendre, P., and Birks, H. J. B. (2012). “Clustering and partitioning,” in Tracking Environmental Change Using Lake Sediments Vol. 5 Data Handling and Numerical Techniques. eds H. J. B. Birks, A. F. Lotter, J. Juggins, and J.P. Smol, (Springer, NY) 167–200.
Lewis, W. M. Jr. (2000). Basis for the protection and management of tropical lakes. Lakes Reserv. Res. Manage. 5, 35–48. doi: 10.1046/j.1440-1770.2000.00091.x
Link, K., Koehn, D., Barth, M. G., Tiberindwa, J. V., Barifaijo, E., Aanyu, K., et al. (2010). Continuous cratonic crust between the Congo and Tanzania blocs in western Uganda. Int. J. Earth Sci. 99, 1559–1573. doi: 10.1007/s00531-010-0548-8
McGowan, S., Leavitt, P. R., Hall, R. I., Anderson, N. J., Jeppesen, E., and Odgaard, B. V. (2005). Controls of algal abundance and community composition during ecosystem state change. Ecology 86, 2200–2211. doi: 10.1890/04-1029
Meyer, I., Van Daele, M., Fiers, G., Verleyen, E., De Batist, M., and Verschuren, D. (2018). Sediment reflectance spectroscopy as a paleo-hydrological proxy in East Africa. Limnol. Oceanogr. Methods 16, 92–105. doi: 10.1002/lom3.10230
Mills, K., Ryves, D. B., Anderson, N. J., Bryant, C. L., and Tyler, J. J. (2014). Expressions of climate perturbations in western Ugandan crater lake sediment records during the last 1000 years. Climate Past 10, 1581–1601. doi: 10.5194/cp-10-1581-2014
Mills, K., Schillereff, D., Saulnier-Talbot, É., Gell, P., Anderson, N. J., Arnaud, F., et al. (2016). Deciphering long-term records of natural variability and human impact as recorded in lake sediments: the palaeolimnological puzzle. WIREs Water 4:e1404. doi: 10.1002/wat2.1195
Nash, D. J., De Cort, G., Chase, B. M., Verschuren, D., Nicholson, S. E., Shanahan, T. M., et al. (2016). African hydroclimatic variability during the last 2000 years. Quat. Sci. Rev. 154, 1–22. doi: 10.1016/j.quascirev.2016.10.012
Nicholson, S. E. (1996). “A review of climate dynamics and climate variability in Eastern Africa,” in The Limnology, Climatology and Paleoclimatology of the East African Lakes, eds T. C. Johnson and E. O. Odada (Gordon and Breack Publishers), 25–56.
Paerl, H. W., Hall, N. S., and Calandrino, E. S. (2011). Controlling harmful cyanobacterial blooms in a world experiencing anthropogenic and climatic-induced change. Sci. Total Environ. 409, 1739–1745. doi: 10.1016/j.scitotenv.2011.02.001
Russell, J., and Johnson, T. C. (2005). A high-resolution geochemical record from Lake Edward, Uganda-Congo, and the timing and causes of tropical African drought during the late Holocene. Quat. Sci. Rev. 24, 1375–1389. doi: 10.1016/j.quascirev.2004.10.003
Russell, J., and Johnson, T. C. (2007). Little ice age drought in equatorial Africa: intertropical convergence zone migrations and El-Nino-southern oscillation variability. Geology 35, 21–24. doi: 10.1130/G23125A.1
Russell, J. M., McCoy, S. J., Verschuren, D., Bessems, I., and Huang, Y. (2009). Human impacts, climate change, and aquatic ecosystem response during the past 2000 yr at Lake Wandakara, Uganda. Quat. Res. 27, 315–324. doi: 10.1016/j.yqres.2009.06.008
Russell, J. M., Verschuren, D., and Eggermont, H. (2007). Spatial complexity of ‘Little Ice Age' climate in East Africa: sedimentary records from two crater lake basins in western Uganda. Holocene 17, 183–193. doi: 10.1177/0959683607075832
Ryves, D. B., Mills, K., Bennicke, O., Brodersen, K. P., Lamb, A. L., Leng, M. J., et al. (2011). Environmental change over the last millennium recorded in two contrasting crater lakes in western Uganda, eastern Africa (Lakes Kasenda and Wandakara). Quat. Sci. Rev. 30, 555–569. doi: 10.1016/j.quascirev.2010.11.011
Saulnier-Talbot, É. (2016). Paleolimnology as a tool to achieve environmental sustainability in the Anthropocene: an Overview. Geosciences 6:26. doi: 10.3390/geosciences6020026
Saulnier-Talbot, É., Gregory-Eaves, I., Simpson, K. G., Efitre, J., Nowlan, T. E., Taranu, Z. E., et al. (2014). Small changes in climate can profoundly alter the dynamics and ecosystem services of tropical crater lakes. PLoS ONE 9:e86561. doi: 10.1371/journal.pone.0086561
Ssemmanda, I., Ryves, D. B., Bennicke, O., and Appleby, P. G. (2005). Vegetation history in western Uganda during the last 1200 years: a sediment-based reconstruction from two crater lakes. Holocene 15, 119–132. doi: 10.1191/0959683605hl774rp
Stager, J. C., Ryves, D., Cumming, B. F., Meeker, L. D., and Beer, J. (2005). Solar variability and the levels of Lake Victoria, East Africa, during the last millennium. J. Paleolimnol. 33, 243–251. doi: 10.1007/s10933-004-4227-2
Strayer, D. L., and Dudgeon, D. (2010). Freshwater biodiversity conservation: recent progress and future challenges. J. North Am. Benthol. Soc. 29, 344–358. doi: 10.1899/08-171.1
Stuiver, M., Reimer, P. J., and Reimer, R. W (1986–2014). CALIB Radiocarbon Calibration Program Rev. 7.0.2. Available online at: http://calib.qub.ac.uk/calib/
Swingedouw, D., Mignot, J., Ortega, P., Khodri, M., Menegoz, M., Cassou, C., et al. (2017). Impact of explosive volcanic eruptions on the main climate variability modes. Glob. Planet. Change 150, 24–45. doi: 10.1016/j.gloplacha.2017.01.006
Verschuren, D., Cocquvt, C., Tibby, J., Neil Roberts, C., and Leavitt, P. R. (1999). Long-term dynamics of algal and invertebrate communities in a small, fluctuating tropical soda lake. Limnol. Oceanogr. 44, 1216–1231. doi: 10.4319/lo.1999.44.5.1216
Keywords: Africa, drought, lake levels, paleolimnology, sedimentary pigments
Citation: Saulnier-Talbot É, Chapman LJ, Efitre J, Simpson KG and Gregory-Eaves I (2018) Long-Term Hydrologic Fluctuations and Dynamics of Primary Producers in a Tropical Crater Lake. Front. Ecol. Evol. 6:223. doi: 10.3389/fevo.2018.00223
Received: 24 January 2018; Accepted: 05 December 2018;
Published: 18 December 2018.
Edited by:
Gavin L. Simpson, University of Regina, CanadaReviewed by:
Teresa Vegas-Vilarrúbia, University of Barcelona, SpainAndrea Lami, Istituto di Ricerca Sulle Acque (IRSA), Italy
Copyright © 2018 Saulnier-Talbot, Chapman, Efitre, Simpson and Gregory-Eaves. This is an open-access article distributed under the terms of the Creative Commons Attribution License (CC BY). The use, distribution or reproduction in other forums is permitted, provided the original author(s) and the copyright owner(s) are credited and that the original publication in this journal is cited, in accordance with accepted academic practice. No use, distribution or reproduction is permitted which does not comply with these terms.
*Correspondence: Émilie Saulnier-Talbot, emilie.saulnier-talbot@cen.ulaval.ca
†Present Address: Kyle G. Simpson, Centre for Ocean Climate Chemistry, Institute of Ocean Sciences, Fisheries & Oceans Canada, Sidney, BC, Canada