- 1Department of Entomology and Nematology, North Florida Research and Education Center, University of Florida, Quincy, FL, United States
- 2Department of Entomology and Nematology, Citrus Research and Education Center, University of Florida, Lake Alfred, FL, United States
The Asian citrus psyllid, Diaphorina citri Kuwayama, is the vector of the phytopathogenic bacterium, Candidatus Liberibacter asiaticus (CLas), that causes citrus greening. Diaphorina citri is attracted to methyl salicylate (MeSA) emitted by CLas-infected citrus. MeSA is catalyzed from salicylic acid (SA) by salicylic acid methyl transferase (SAMT). In addition, salicylate hydroxylase, an enzyme that degrades SA and suppresses host plant defense, is unregulated in CLas-infected as compared with uninfected citrus. Based on these findings, we hypothesized that CLas-induced plant volatile emissions and SAMT expression may decrease over time following CLas infection. We first identified the putative Citrus sinensis SAMT (CsSAMT) that methylates SA to MeSA. Thereafter we compared changes in SAMT expression and volatile expression between uninfected citrus, citrus recently (< 6 months) infected with CLas, and citrus infected with CLas for a long (>1 year) duration. Emission of MeSA increased in citrus recently infected with CLas, whereas both MeSA emissions and SAMT expression decreased in long-infected citrus as compared with uninfected controls. Also, there was a significant decrease in release of limonene from citrus infected >1 year, and a concomitant increase in release of β-caryophyllene in both recent and long-infected citrus as compared with controls. In behavioral assays, D. citri preferred settling on asymptomatic citrus recently infected with CLas over uninfected or long-infected citrus trees. The odor of citrus recently infected with CLas was more attractive to D. citri than the odor of uninfected or long-infected citrus. There was an association between the preference for citrus odor of recently infected plants and an increase of MeSA release following CLas infection. Overall, our study demonstrated pulsed changes in citrus odor release following CLas infection. We hypothesize that allocation of resources to plant defense in citrus decreases as a function of huanglongbing disease symptom progression. These changes affected the behavior of the CLas vector. Our study confirms previous reports demonstrating that plant defenses boosted following phytopatogen infection tend to decrease over time. Implications on the CLas epidemic are discussed.
Introduction
By definition, a plant pathogen modifies the physiology of its plant host. In recent years, several examples have been described where plant pathogens induce changes in volatile emissions from plants that affect the behavior of herbivores which also act as vectors of these pathogens (Eigenbrode et al., 2002; Mayer et al., 2008a,b; Mauck et al., 2010). In most cases, the infected plant is initially attractive to the vector of the pathogen, but decreases in quality as a host following onset of disease, inducing dispersal of the vector in search of better alternative hosts (Eigenbrode et al., 2002; Mauck et al., 2010; Mann et al., 2012). This succession of events: initial attraction to infected plant; followed by vector dispersal away from the sub-optimal host, coincident with pathogen acquisition, has been described as the “deceptive host hypothesis” (Mauck et al., 2010). Pathogen-induced changes in the host plant that increase recruitment of the vector to infected plants would serve as a selective advantage for the pathogen. A predicted consequence is the accelerated spread of the pathogen caused by an increase in the proportion of infected individuals in the population (Martini et al., 2016).
The Asian citrus psyllid, Diaphorina citri Kuwayama (Hempitera: Liividae), is the vector of the fastidious gram-negative, phloem-limited bacterium, Candidatus Liberibacter asiaticus (CLas). CLas is the agent responsible for the citrus-killing disease huanglongbing (HLB), or citrus greening. HLB is the most significant disease of cultivated citrus globally (Bové, 2006). The disease causes decline in root growth and primary leaf symptoms are characterized by yellowing along the veins and development of a blotchy-mottle pattern on leaves (Gottwald et al., 2007). Secondary symptoms are characterized by small leaves with chlorotic patterns similar to those induced by zinc and ion-deficiencies and increased fruit drop (Wang and Trivedi, 2013). Fruit yield and commercial value of harvested fruit decline; fruit become misshapen, lopsided, discolored, and lose their organoleptic properties, resulting in a sour taste (Bassanezi et al., 2009). Trees die 5–10 years after infection (Bové, 2006; Wang and Trivedi, 2013). Within 15 years, Florida citrus yield has been reduced 2-fold due to HLB.
Infection with CLas in citrus activates the salicylic acid (SA) pathway inducing release of the methylated SA derivate, methyl salicylate (MeSA) (Park et al., 2007; Fu and Dong, 2013; Nehela et al., 2018). MeSA is often associated with indirect plant defense in response to pathogens and phloem feeding insects, resulting in attraction of natural enemies (Park et al., 2007). In citrus, the D. citri vector is attracted to MeSA, which is induced by CLas infection (Mann et al., 2012; Aksenov et al., 2014; Martini et al., 2014b). Salicylic acid methyl transferase (SAMT), a member of the SABATH family, catalyzes the formation of MeSA from salicylic acid (SA) and S-adenosyl-L-methionine (Ross et al., 1999; Negre et al., 2002). However, salicylate hydroxylase (SAH), an enzyme that degrades SA and suppresses host plant defense, is also unregulated in CLas-infected as compared with uninfected citrus, which reduces SA in leaf tissue (Li et al., 2017). Based on these findings, we hypothesized that CLas-induced plant volatile emissions may occur as a discrete pulse following initial infection and decrease over time. Congruently, the effect on vector behavior following pathogen infection would also vary over time in association with changes in MeSA release.
The main objective of this investigation was to describe induced changes in D. citri behavior, MeSA emissions, and SAMT expression at various intervals following CLas infection of host plants. Initially, we identified the putative Citrus sinensis SAMT (CsSAMT) that methylates SA to MeSA. Concurrent volatile collection from headspace of citrus plants addressed possible association between MeSA emission and CsSAMT expression levels. Investigating both the change in MeSA release and CsSAMT expression addressed whether decreasing emission of MeSA over time following infection was associated with decreased CsSAMT expression vs. metabolic changes in infected plants. In addition, we confirmed that changes in vector behavioral response were mediated by volatiles emitted from plants.
Materials and Methods
Insect Culture
Diaphorina citri used in experiments were obtained from a culture established in 2000 from field populations in Polk County, FL, USA prior to the discovery of HLB in the state. This culture was maintained on “Hamlin” orange [Citrus sinensis (L.)] (Rutaceae) and Murraya paniculata seedlings with flush in a greenhouse at 27–28°C, 60–65% R.H., and 14L:10D photoperiod. Illumination in the greenhouse was supplemented with linear fluorescent 54 W lights (F54W/T5/865/ECO, GE lighting, Nela Park, OH). Bi-monthly quantitative PCR (qPCR) assays were conducted on randomly sampled nymphs and adults to confirm that psyllids and plants in this culture were not infected with CLas.
Plant Maintenance
Plants were purchased from a local nursery (Dundee, FL) and were certified free of CLas. CLas infection in host plants was induced by graft-inoculation of ~ 2-year old, non-infected “Valencia” C. sinensis with CLas-infected key lime (C. aurantifolia Swingle) budwood collected from citrus groves in Immokalee, FL, USA. Leaf samples from grafted plants were tested for CLas infection by qPCR 3 months after grafting, as described below (Pelz-Stelinski et al., 2010). Plants that tested positive for CLas were used in experiments. Uninfected plants used in experiments were cultivated from C. sinensis seed or obtained as potted seedlings from a certified HLB-free commercial nursery to minimize risk of undetectable latent infection with CLas in grafted plants. The nursery-obtained plants were confirmed negative for CLas infection by qPCR as described below. Non-infected and CLas-infected plants were maintained in separate secure rearing facilities with minimal risk of cross contamination under the environmental conditions described above for insects. Plants that were infected for 3 to < 6 months were referred to as newly infected, whereas plants infected for 15–24 months were referred to as long-infected. Control trees were also 2 years old at the beginning of the experiment. Therefore, all trees used in this study were between 2 and 4 years old. Typically, the newly infected plants were characterized by the absence of HLB-symptoms, whereas long-infected plants were characterized by apparent symptoms of HLB (blotchy-mottle pattern and crocking veins).
Quantification of CLas in Plant Samples
DNA from leaf samples was isolated using a modified CTAB method. Briefly, 200 mg of petiole tissue was ground with a Tissue Lyser (Fischer Sonic dismembrator 550, Fisher Scientific Waltham, MA) in 500 μl of CTAB buffer (100 ml/l 1M tris-HCl; 280 nl/l 5M NaCl; 40 ml/l 0.5 M EDTA; 20 g/l CTAB; 5 ml/l β-mercaptoethanol; 575 ml/l DNase free water, pH 5). The samples were incubated for 15 min at 55°C in a circulating water bath. The samples were centrifuged at 14 000 × g for 5 min and the supernatants were transferred to new tubes. Chloroform-isoamyl alcohol (250 μl) was added, and the samples were subsequently centrifuged at 16 000 × g for 1 min. The aqueous phase was transferred into new Eppendorf tubes and 50 μl of 7.5 M Na Acetate, followed by 500 μl of ice-cold absolute ethanol, was added. DNA was precipitated −20°C for 1 h, and the samples were centrifuged at 16 000 × g for 1 min. The supernatant was removed and the DNA was washed three times with 70% of ice-cold ethanol followed by centrifugation at 16 000 × g for 1 min. After the third wash, supernatant was aspirated, and the pellets were air dried for 15 min. The pellets were re-suspended in 250 μl DNase free water at 65°C for 20 min and then stored at −20°C. The concentration and purity of the extracted DNA was measured with a Nano Drop 2000 (Thermo Fisher Scientific, Waltham, MA).
The presence of CLas in citrus tissue was assessed using a multiplex TaqMan (Applied Biosystems, Foster City, CA) quantitative polymerase chain reaction (qPCR) assay targeting the 16S rRNA of CLas and the cytochrome oxidase gene of citrus (Pelz-Stelinski et al., 2010). The reactions were processed in a 7500 Fast Real-Time PCR System (Applied Biosystems), using Micro Amp Fast Optical 96-Well Reaction Plates (Applied Biosystems) and Micro Amp Optical Adhesive Film (Applied Biosystem). Reaction constituents, concentrations and protocol were as described in Coy et al. (2014). The qPCR routine was (1) 2 min at 50°C, (2) 10 min at 95°C, and (3) 40 cycles with 15 s at 95°C and 60 s at 60°C (data collection).
Host Preference Settling Assays
Psyllid settling experiments were conducted in a climate-controlled room (26°C and 45% RH) with overhead fluorescent lighting set to a 16:8 (L:D) light cycle. Two citrus plants were caged in 61 × 61 × 183 cm tall mesh cages (Bioquip, Rancho Dominguez, CA, USA). Pots and the sides of cages were covered with white benchtop pads to minimize external visual stimuli. One hundred, unsexed, unaged, and uninfected (CLas free culture) D. citri collected from the rearing cage were released directly into each mesh cage with a light source on the top of the cage (329.17 ± 25.94 lux), and the number of psyllids settling on each plant was counted every 24 h during 4 days. During the counting procedure, only D. citri that were immobile for more than 5 min were counted. D. citri adults were counted on: (1) asymptomatic CLas-infected citrus (infected for < 6 months) vs. uninfected citrus; (2) symptomatic CLas infected citrus (infected for > 12 months) vs. uninfected citrus; or (3) asymptomatic CLas-infected citrus (infected for < 6 months) vs. symptomatic CLas infected citrus (infected for > 12 months). Psyllids were counted on all leaves on each tree by progressing from lower to upper portions of plants and counting with aide of a hand clicker. Five replicates were conducted for each treatment combination.
Behavioral Response to Olfactory Cues
Response of D. citri to volatile cues alone in the absence of visual or tactile host cues was investigated using a two-port divided T-maze olfactometer (Analytical Research Systems, Gainesville, FL). This olfactometer was developed by Mann et al. (2011), to investigate response of D. citri to semiochemicals (Mann et al., 2012; Martini et al., 2014a; Martini and Stelinski, 2017). The olfactometer consists of a 30 cm long glass tube with 3.5 cm internal diameter bifurcated into two equal halves with a polytetrafluoroethylene (PTFE) strip forming a T-maze. Each half serves as an arm of the olfactometer, enabling the D. citri to make a choice between two potential odor fields. During the behavioral experiments, a constant airflow of 0.1 l. min−1 was maintained throughout the olfactometer. To obtain a light intensity of ~ 600 lux above the T-maze, the olfactometer was positioned vertically under a fluorescent 23 W light source (FLE23HT3/3/SW, GE Lighting, Cleveland, OH), mounted within a 1.0 × 0.6 × 0.6 m fiberboard box for uniform light diffusion. Only females were released individually at the base of the olfactometer (Martini et al., 2014a). Diaphorina citri females used in assays were 4–14 days old and kept with males; therefore, most were likely mated prior to initiation of the experiment. Females were allowed 300 s to exhibit a behavioral response. A positive response was recorded when a psyllid moved from the base and entered 1 cm into either arm of the olfactometer. Psyllids that did not enter 1 cm into either arm of the olfactometer were designated as non-responders (Martini et al., 2014a). The olfactometer was rotated every five psyllids to avoid positional bias, and the treatments were assigned randomly to each arm. In addition, the olfactometer apparatus was cleaned with Sparkleen (Fischerbrand, Fischer Scientific, Pittsburgh PA) and acetone after 20 replicates, and kept at 60°C until dry. The olfactometer arms were connected to citrus plants enclosed within two-port glass domes (38 cm height, 14.4 cm ID). The upper and lower portions of each plant were separated by a PTFE guillotine (2.5 cm width) as described in Martini et al. (2014a). Clean air was pushed into the bell jar to maintain airflow out of the glass dome and into the olfactometer at 0.1 l. min−1. The plants were positioned under a 150 W high-pressure sodium grow light (Hydrofarm, Petaluma, CA). All assays were conducted between 9:00 and 14:00 h. Psyllids were exposed to two treatments in the T-maze: (1) asymptomatic CLas-infected citrus (infected for < 6 months) vs. uninfected citrus, and (2) symptomatic CLas infected citrus (infected for > 12 months) vs. uninfected citrus. There were four different sets of plants used for the two treatments and 20 D. citri were tested per set of plants for each treatment (80 total psyllids per treatment).
Volatile Collection
A volatile collection system was used to describe the headspace profiles of citrus volatiles from uninfected citrus and plants infected with CLas (both asymptomatic and symptomatic) for various durations described above. Five replicate plants were analyzed for each treatment. The volatile collection system consisted of four parallel glass domes (38 cm height, 23 cm ID), each with two 3 cm ports. An inlet near the top was connected to incoming airflow and an outlet near the bottom of the dome was connected to a vacuum. Each glass dome was positioned onto a 5 cm PTFE guillotine so that each plant was separated into two parts for headspace collection (Martini et al., 2014a). A volatile collection trap (7.5 cm long) with 30 mg of HayeSep Q adsorbent (Volatile Assay Systems, Rensselaer, NY) was connected to the bottom outlet with a PTFE fitting. Volatiles emitted from the upper portion of each plant enclosed within each glass chamber were swept downward by incoming humidified and charcoal filter purified air at a rate of 1.0 l. min−1. The volatiles were forced to the bottom of the chamber by pulling air at 0.6 l. min−1 through the volatile collection traps with a controlled vacuum from the automated volatile collection system (Analytical Research Systems, Gainesville, FL). Leaf volatiles were collected during 24 h, starting between 14:00 and 16:00 h.
Volatiles were extracted from the collection traps by washing with 150 μl of dichloromethane. Nonyl acetate (1,080 ng) was added to the extracts as an internal standard. For each collection sample, 1 μl was manually injected into a Clarus 500 gas chromatograph-mass spectrometer (GC-MS) (PerkinElmer, Shelton, CT). The GC was equipped with a column capillary injector system. Data collection, storage, and subsequent analysis were performed on a Perkin Elmer chromatographic data system (TurboMass™). Helium was used as the carrier gas at a linear flow velocity of 2 ml min−1. All samples were analyzed on a fused silica RTX-5 capillary column (Restek Corporation, Bellefonte, PA), 60 cm × 0.25 mm ID. The temperature of the column oven was maintained at 40°C for 1 min and then increased at a rate of 7°C min−1 to a final temperature of 300°C, and maintained at 300°C for 6 min. The injector temperature was set at 270°C with the detector set at 200°C. Quantitation was based on GC-MS profiles and was accomplished by comparing peak areas of known amounts of nonyl acetate with the peak areas of compounds extracted from the leaves. Constituents of the plant volatile emissions were identified by comparison of mass spectra with spectra in the National Institute of Standards and Technology database and the spectra obtained from authentic reference compounds, when available. In addition, GC retention times of plant volatiles were compared with those authentic compounds, when available, on the RTX-5 column.
Identification of CsSAMT Amino Acid Sequences
A reiterative BLAST search strategy was employed to mine the NCBI and Phytozyome (phytozome.jgi.doe.gov) genomic and proteomic Citrus sinensis databases for putative SABATH enzyme sequences using the BSMT1, FAMT, GAMT1, GAMT2, IAMT, and JMT amino acid sequences from Arabidopsis thaliana, the SAMT sequence from Clarkia breweri, and the XMT (Cs1) sequence from Coffea arabica as queries (GenBank numbers, Supplementary Materials). Sequences from the multiple BLAST searches were aligned using Clustal X v. 2.0 (Larkin et al., 2007), and redundant sequences were identified and removed, resulting in a total of 35 putative C. sinensis SABATH amino acid sequences. After a preliminary phylogenetic analysis, four sequences were removed from further analysis: three partial sequences that did not group with representative SAMT sequences, and a putative transcription factor that contained the conserved domain of the Methyltransf_7 superfamily, resulting in a total of 31 putative C. sinensis SABATH sequences. These 31 sequences, named CsSABATH 1-31, were aligned with amino acid sequences representing all major clades of the SABATH family using Clustal X v. 2.0 (Larkin et al., 2007), including two with biochemically characterized SAMT activity, AlBSMT from Arabidopsis lyrata (Chen et al., 2003) and SlSAMT from Solanum lycopersicum (Tieman et al., 2010). Accession numbers for representatives are provided in Supplementary Materials. The alignment was visually inspected, and after minor adjustments, was subjected to three evolutionary models to infer the phylogenetic relationships of the putative C. sinensis and representative SABATH amino acid sequences. Two evolutionary models were used as implemented by PAUP* v. 4.0 b10 (Wilgenbusch and Swofford, 2003), neighbor-joining and maximum parsimony. Maximum parsimony was conducted using the heuristic search algorithm with 100 random sequence additions and tree-bisection-and-reconnection branch swapping. Bootstrapping with 5,000 replicates was conducted for both evolutionary models to determine the confidence of groupings. TreeView was used to visualize the resulting phylogenetic trees (Page, 1996). Both evolutionary models produced highly congruent trees (Figure 1), with maximum parsimony producing three trees, differing only in the internode arrangement of CsSABATH18, 19, and 20 (data not shown). For both evolutionary models, a single citrus amino acid sequence, CsSABATH1, grouped with other known SAMT representatives with 100% bootstrap support, and was named CsSAMT as the putative methyltransferase that methylates SA to MeSA.
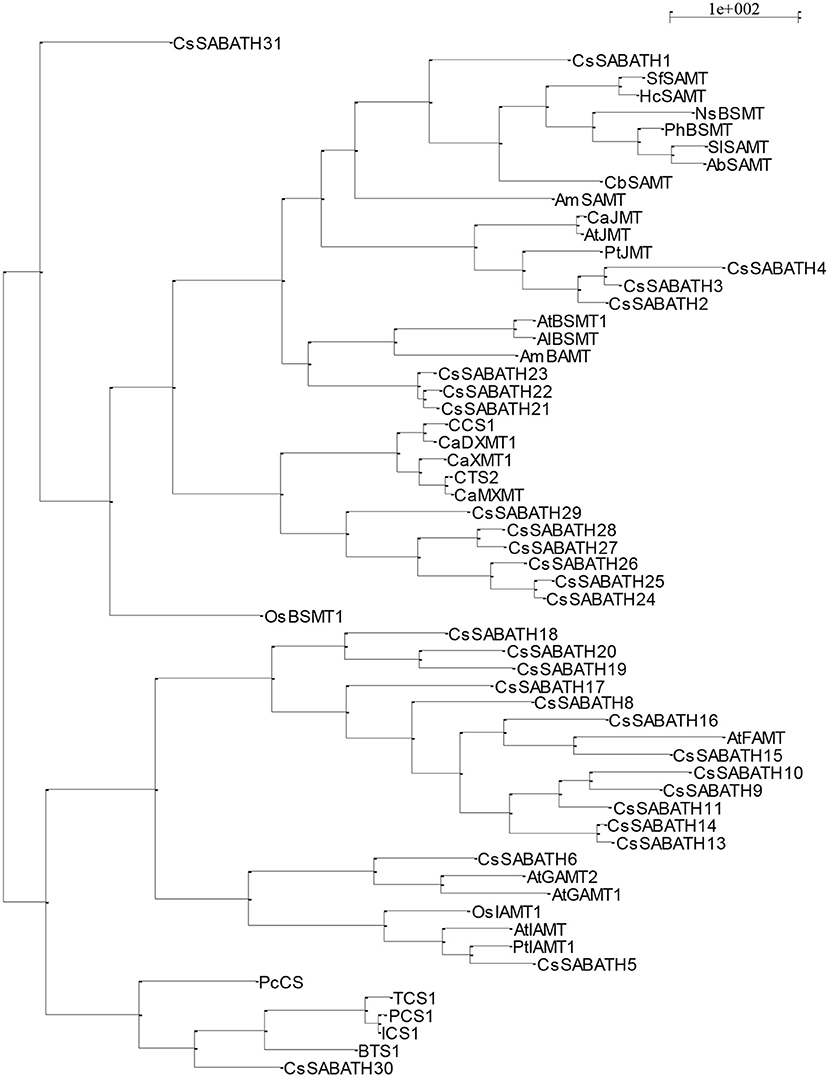
Figure 1. Neighbor-joining, phylogenetic tree depicting the inferred evolutionary relationships between the 31 SABATH amino acid sequences mined from the Citrus sinensis genome and representative SABATH sequences from other plant species. Amino acid sequences and accession numbers for CsSABATH and representative sequences provided in Supplementary Files 1, 2, respectively. Maximum parsimony produced three highly similar trees except for the internode placement of CsSABATH18, 19, and 20 (data not shown), one of which was identical to that produced by neighbor-joining. CsSABATH1 grouped with other known and characterized SAMTs and was named CsSAMT.
RNA Isolation and cDNA Synthesis
Total RNA was isolated from leaf tissue using Trizol® Reagent (Life Technologies, Carlsbad, CA; 15596-026) according to manufacturer's protocols. Briefly, 100 mg of leaf tissue was finely chopped, placed in microcentrifuge tubes (Kontes, K749510-1590), and frozen with liquid nitrogen. Frozen tissue was pulverized to powder with a pestle (Kontes, K749521-1590) and 1 ml of Trizol® Reagent was added to each sample and vortexed. After incubating at room temperature for 5 min, 200 μl of chloroform was added to each sample and shaken vigorously. After incubating at room temperature for 3 min, samples were centrifuged at 16,000 × g for 15 min at 4°C. The aqueous layers were carefully transferred to clean microcentrifuge tubes, and RNA was precipitated from solution with 500 μl of molecular grade isopropanol and centrifugation at 16,000 × g for 10 min at 4°C. After washing the pellet with 75% molecular-grade ethanol, precipitated RNA was air dried and resuspended in 35 μl of 0.1 mM EDTA. Genomic DNA was digested using the DNA-free Kit (Life Technologies, AM1906), according to manufacturer's protocols. RNA integrity was determined by 1.5% agarose gel electrophoresis and quantified by NanoDrop 1000 (Thermo Fisher Scientific, Wilmington, DE). The Cloned AMV First-Strand cDNA Synthesis Kit (Invitrogen, Carlsbad, CA; 12328-032) was used to reverse-transcribe mRNA to cDNA using the Oligo (dT)20 primer and 150 ng of total RNA according to manufacturer's protocols. The resulting products were used directly in qPCR analysis. Reactions with all components minus the reverse transcriptase were conducted and used in qPCR to evaluate contribution of contaminating genomic DNA (described below).
CsSAMT Quantitative PCR
Intron/exon structure and putative transcript splice variants were determined using the Phytozyome entry for CsSAMT. Primers for qPCR were designed using Primer 3 and were selected to detect all predicted mRNA transcript splice variants for this gene (MTSal-qPCR-F5, 5′-TGGAACGCTTATCAGAATGG-3′; MTSal-qPCR-R5, 5′-ACAGCTCTCATGCAATTCG-3′). Primers for the Citrus sinensis cytochrome oxidase subunit 1 (Cox1; Genbank Accession number KF933043) were used as an endogenous control (CoxF1, 5′-GTATGCCACGTCGCATTCCAGA-3′, CoxR1 5′-GCCAAAACTGCTAAGGGCATTC-3′; REF). Amplicon sizes for MTSal and Cox1 are 94 and 68 bp, respectively. Quantitative PCR assays were conducted using Platinum SYBR Green qPCR SuperMix-UDG with ROX (Invitrogen, Carlsbad, CA) in reaction volumes of 20 μl with the following components (in μl): 7.16 DEPC water, 10 SYBR Green SuperMix-UDG, 0.4 forward and reverse primers (10 μM), 0.04 ROX, and 2 cDNA template. The qPCR scheme was: 50°C/2 min; 95°C/2 min; followed by 40 cycles of 95°C/15 s. Agarose gel electrophoresis and melt curve analyses were conducted to verify minimal impact on qPCR dynamics by the formation of primer-dimers.
Statistical Analysis
The numbers of psyllids that settled on each plant during the cage assay were compared with a Chi-square test on pooled data, for each observation day. The data were pooled after ensuring they were homogenous across the different replicates (Zar, 2009). As it consisted of the repletion of 12 successive tests, we applied a Bonferroni correction (α/12). The same procedure was applied to the data from the olfactometer experiment. Finally, the emission of volatiles including MeSA was compared across treatments with a generalized linear model with Poisson distribution and a log link function, while expression of the SABATH gene was assessed with a quasi-GLM model with Poisson distribution (function glm, family = quasipoisson) where the variance is given by φ × μ, where φ is the dispersion parameter and μ the mean, and standard errors multiplied by the square root of φ. This procedure was used because of the overdispersion of the data (Zuur et al., 2009). P-value is given by a subsequent Chi-square test in R.
Results
Host Preference Settling Assays
More D. citri settled on recently infected (< 6 months) than uninfected citrus plants (Figure 2A) after 1, (χ2 = 37.052, d.f. = 1, P < 0.001), 2 (χ2 = 70.591, d.f. = 1, P < 0.001), 3 (χ2 = 56.977, d.f. = 1, P < 0.001), and 4 days (χ2 = 60.548, d.f. = 1, P < 0.001, after Bonferonni correction). However, there was no difference in D. citri host preference plants that were infected > 12 months vs. uninfected control plants (Figure 2B) 1 (χ2 = 0.004, d.f. = 1, P > 0.05), 2 (χ2 = 0.584, d.f. = 1, P > 0.05), 3 (χ2 = 3.1.03, d.f. = 1, P > 0.05), and 4 days (χ2 = 4.661, d.f. = 1, P > 0.05, after Bonferonni correction) after release. More D. citri settled on recently infected plants (< 6 months) than plants infected > 12 months (Figure 2C) 1 (χ2 = 23.382, d.f. = 1, P < 0.001), 2 (χ2 = 15.727, d.f. = 1, P < 0.01), 3 (χ2 = 17.483, d.f. = 1, P < 0.01), and 4 days (χ2 = 32.443, d.f. = 1, P < 0.001, after Bonferonni correction) after release.
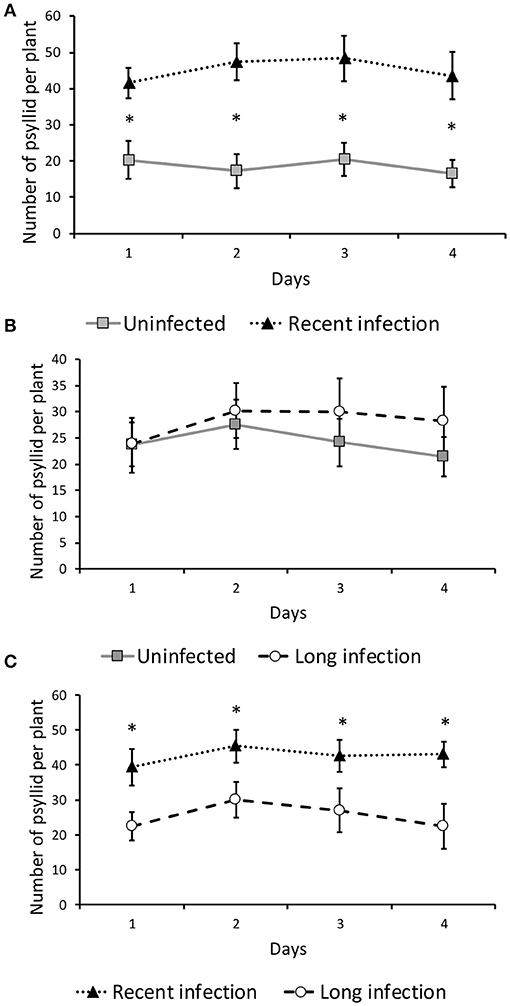
Figure 2. Mean number (± SE) of Diaphorina citri counted on citrus plants during 4 days of observation when given a choice between citrus plants infected by Candidatus liberibacter asiaticus < 6 months (newly infected) vs. uninfected plants (A), or infected > 12 months (long-infected) vs. uninfected plants (B), and recently infected vs. long infected plants (C) (*P < 0.01).
Behavioral Response to Olfactory Cues
When provided with a direct choice, significantly more D. citri exhibited attraction to the odors from recently infected (< 6 months) than from uninfected plants (χ2 = 6.39, d.f. = 1, P = 0.011, Figure 3). In this case, 26.6% of the psyllid did not respond in the olfactometer. However, D. citri exhibited no behavioral preference between the odors from plants infected > 12 months and uninfected plants (χ2 = 0.01, d.f. = 1, P = 0.908, Figure 3). For this comparison, 18.5% of the psyllids did not respond in the olfactometer.
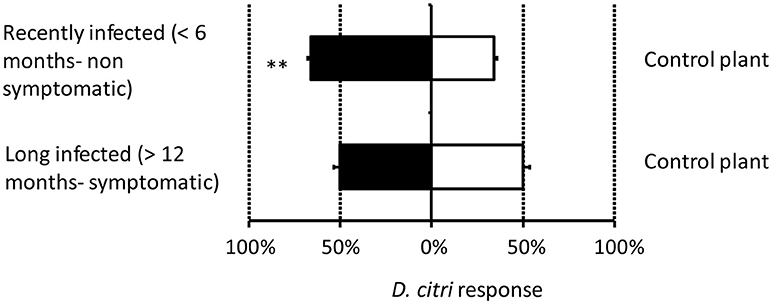
Figure 3. Response of Diaphorina citri females to odors from citrus plants recently infected by Candidatus Liberibacter asiaticus (< 6 months), or infected for a longer period (>12 months) vs. the odor from uninfected citrus within a T-maze olfactometer. Asterisks indicate significant differences between the two treatments (*P < 0.05).
Volatile Collection and CsSAMT Gene Expression
Volatile profiles from uninfected and CLas-infected plants differed significantly. Release of β-caryophyllene increased significantly in both recent and long infected plants, whereas sabinene, D-3-carene and limonene release decreased significantly in long infected plants as compared with uninfected controls (Table 1). Release of MeSA was significantly higher from recently infected (< 6 months) (GLM with Poisson distribution: χ2 = 39.816, d.f. = 11, P < 0.001) than from plants infected > 12 months or uninfected control plants (Table 1, Figure 4A). There was no significant difference in the amount of MeSA emitted between uninfected plants and those infected > 12 months (Figure 4A). In addition, expression of CsSAMT mRNA was significantly higher in recently infected (< 6 months) citrus than citrus infected > 12 months (quasi-GLM model with Poisson distribution: χ2 = 23.89, d.f. = 11, P = 0.006) (Figure 4B). Although there was a trend for greater expression of CsSAMT mRNA in recently infected plants (< 6 months) than uninfected controls, this difference was not statistically significant (Figure 4B).
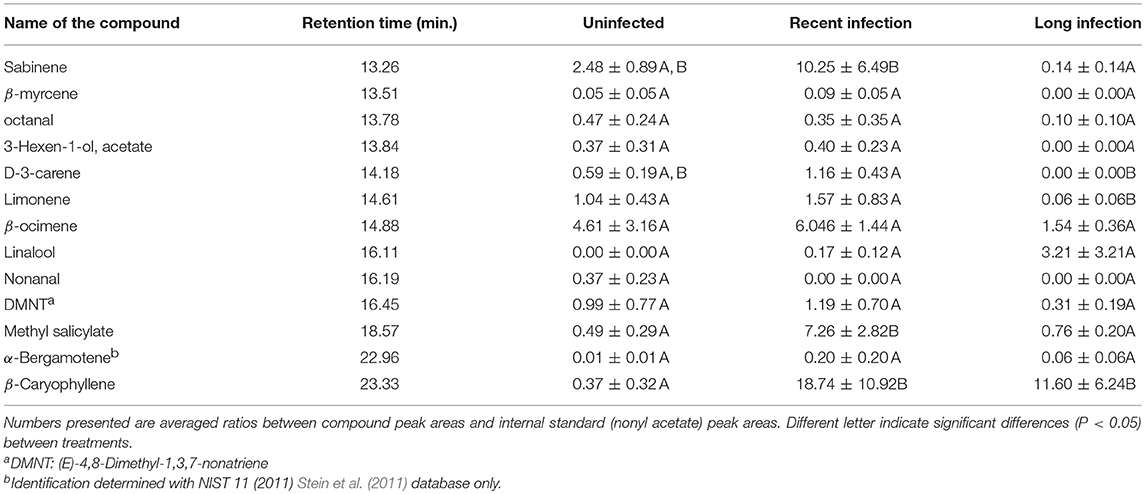
Table 1. Volatiles (Means ± SE; n = 4) released from uninfected citrus and citrus infected with Candidatus liberibacter asiaticus for various durations.
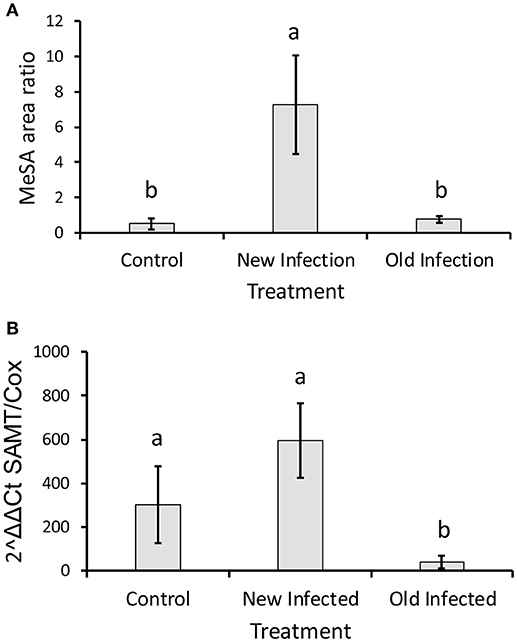
Figure 4. (A) Release of methyl salicylate by young infected, old infected, and uninfected citrus plants. The rates are measured as a ratio of the peak area of MeSA compared to the internal standard (nonyl acetate). (B) Expression of the gene SAMT compared to cox gene in newly infected, long-infected, and uninfected citrus plants. Different letters indicate significant difference (P < 0.05) among treatments.
Discussion
Plant pathogens transmitted by insects have evolved the capacity to affect host plant phenotype and physiology to attract vectors (Eigenbrode et al., 2002; Davis et al., 2012; Mann et al., 2012). This behavior can be mediated by manipulation of visual cues. For instance, the whitefly Bemisia tabaci (Gennadius) prefers to land on virus-infected over uninfected leaves based on visual cues as measured by assays that eliminate potential response to olfactory cues (Fereres et al., 2016). However, investigating behavioral preference of insect phytopathogen vectors as mediated by pathogen-induced olfactory cues has received considerably more attention. The capability of a pathogen to attract its vector by manipulating host phenotype accelerates the spread of disease by increasing the proportion of infected individuals within a population (Martini et al., 2016). Given the evolutionary advantage afforded to the pathogen, it is not surprising that this phenomenon is observed in a variety of insect orders. For instance, the striped cucumber beetle is attracted to volatiles emitted by wild gourds infected by the bacteria Erwinia tracheiphila, which is vectored by the beetle (Shapiro et al., 2012). The Cucumber mosaic virus induces volatiles that attract its two aphid vectors, Myzus persicae Sulzer and Aphis gossypii Glover (Mauck et al., 2010).
Herbivorous insects select their host by the perception of plant volatiles (kairomones) that often consist of ubiquitous compounds distributed generally among plant species. The selection of the host plant is based on the relative ratio of these constituent compounds (Bruce et al., 2005). MeSA is a common volatile associated with the activation of the SA defense pathway. In citrus, release of MeSA is extremely low or absent from trees that are not damaged by herbivore feeding or infected by pathogens (Mann et al., 2010, 2012; Martini and Stelinski, 2017; Patt et al., 2018). Given that MeSA is often associated with phloem-feeding insect damage, it attracts a large diversity of predators and parasitoids (Zhu and Park, 2005; Rodriguez-Saona et al., 2011; Martini et al., 2014b). It also repellent to some insects, presumably because it indicates activation of plant defenses (Hardie et al., 1994; Glinwood and Pettersson, 2000; Koschier et al., 2007; Martini et al., 2017).
In our current investigation using the HLB pathosystem, we observed a decline in emission of MeSA from infected plants as the duration of disease progression increased from 3 to 6 months to more than 1 year. Trees that were sampled during a more recent stage of infection released nearly 8-fold more MeSA than uninfected counterparts. Similarly, β-caryophyllene increased in recently infected citrus confirming results from previous investigations (Mann et al., 2012; Patt et al., 2018). β-caryophyllene is a volatile commonly associated with citrus. This compound is present in high concentrations among headspace volatiles of known attractive hosts of D. citri, such as Murraya paniculata (Patt and Setamou, 2010; Robbins et al., 2012) and M. koenigii (Robbins et al., 2012; Beloti et al., 2017). Despite strong activation of D. citri antennal neurons by β-caryophyllene (Coutinho-Abreu et al., 2014), its effect on behavior of D. citri is unclear. β-caryophyllene is the main constituent, with β-Ocimene, of a volatile blend known to increase capture of D. citri on yellow sticky traps (Patt and Setamou, 2010). However, when tested as an isolated compound in olfactometers, conflicting results were reported; Mann et al. (2012) did not find any significant response associated to β-caryophyllene at 5 μg/μl, whereas Alquézar et al. (2017) found it to repel D. citri at 1 and 10 μg/μl. In other systems, plant pathogen infection induced increased release of β-caryophyllene. For instance, infection of apple trees with the phytoplasma, Candidatus Phytoplasma mali, induces release of ß-caryophyllene among leaf volatiles, and this increase attracts the phytoplasma vector, Cacopsylla picta Foerster (Hemiptera: Psyllidae) (Mayer et al., 2008a). Furthermore, β-caryophyllene has been successfully used in lures to increase trapping of C. picta in apple orchards (Mayer et al., 2008b; Gross et al., 2011).
It is possible that response of D. citri to citrus recently infected with CLas is mediated by both MeSA and β-caryophyllene. Further investigations should examine the possibility of an interaction between MeSA and β-caryophyllene. It is possible that β-caryophyllene is not attractive to D. citri alone, but that the combination of MeSA and β-caryophyllene may cause an additive or synergistic effect on behavioral response. We also suggest that additional experiments directly comparing the response of D. citri to trees of varying infection length should be completed to definitively conclude that recently infected CLas trees are more attractive than citrus trees infected for longer durations.
Complementary measurements of CsSAMT mRNA in leaf tissue revealed that expression levels decreased dramatically as the stage of disease progression increased. The concomitant rise and fall in CsSAMT mRNA levels and MeSA emissions, and the results of the phylogenetic analyses wherein CsSAMT groups in the clade with biochemically characterized SAMT enzymes, are consistent with the hypothesis that CsSAMT is the methyltransferase that catalyzes the formation of MeSA from SA in this pathosystem. Nevertheless, further biochemical and molecular investigation is required for confirmation. Recent data indicate that SA decreases in CLas infected plants due to the accumulation and activity of SAH (Li et al., 2017). The investigation by Li et al. measured SA levels on a much shorter time scale, and thus direct comparison of results between the two studies is impossible. However, both investigations suggest that modulation of SA and its methylated volatile, MeSA, by CLas changes host plant physiology to indirectly affect vector behavior.
Although host phenotype manipulation by plant pathogens has been described in several pathosystems, it is not yet clear how this system may have evolved. One hypothesis is that CLas benefits indirectly when D. citri are attracted to MeSA as an indicator of conspecifics for mate finding (Martini et al., 2014a). An alternative hypothesis is that CLas is actively inducing release of MeSA, because attracting D. citri would increase the proportion of infected vectors (Martini et al., 2016). However, MeSA release is significantly decreased by drought stress (Martini and Stelinski, 2017), as well as by activation of the JA pathway (Patt et al., 2018). Given the energetic cost of the SA pathway, there is a trade-off between allocation of resources to defense vs. other plant functions (Walters and Heil, 2007). In the case of an active pathogen manipulation, maintaining attraction of vectors and the emission of MeSA via CsSAMT expression would be expected despite plant decline and independent of abiotic factors. Therefore, a strong selection pressure on CLas to actively induce MeSA release seems unlikely as plants decline due to disease or abiotic stress. This suggests that attraction of D. citri to CLas infected plants is more likely incidental rather than an active manipulation of the plant by the pathogen.
More broadly, our results demonstrate that pathogen-induced changes in emission of volatile organic compounds (VOCs) are dynamic and vary as disease progresses. These results are congruent with Werner et al. (2009) who found that emission of volatiles from potato plants infected with potato leafroll virus increased 4–6 weeks after infection, but declined over time thereafter. Similarly, Martini et al. (2017) found that emission of MeSA from swamp bay, Persea palustris Sarg., increased directly following infection with the phytopathogenic fungus, Raffaelea lauricola, but declined rapidly as disease progressed, while emission of sesquiterpenes increased. Interestingly, MeSA was repellent to redbay ambrosia beetle, Xyleborus glabratus Eichhoff (Coleoptera: Scolytinae), that vector R. lauricola while sesquiterpenes were attractants (Martini et al., 2017). Consequently, beetle vectors were attracted to infected trees that showed symptoms of significant decline, but avoided newly infected, non-symptomatic counterparts (Martini et al., 2017).
The difference between CLas-D. citri pathosystem in citrus investigated here, and R. lauricola- Xyleborus glabratus pathosystem in Lauraceae (Martini et al., 2017), illustrates that pathogen-induced changes in VOC emission can have widely differing consequences on host selection behavior of vectors. These differences likely depend, in part, on differences in life history strategies that evolved among the hosts. For example, D. citri are attracted to newly emerging leaves as the only source of egg laying site (Tsai and Liu, 2000). In contrast, X. glabratus are attracted to declining and dying hosts, where development may be more optimal (Hulcr and Stelinski, 2017). The consequences of temporal changes in emission of pathogen-induced cues on vector behavior likely display considerable variation among the examples investigated thus far and discussed here depending on ecological differences of the vector species in question. Further comparative investigations of pathosystems in which pathogens appear to indirectly manipulate their own fate through behavior of the vector are necessary to find patterns among vectors with differing ecological strategies.
In the case of the CLas-D. citri pathosystem, pathogen mediated indirect effects on vector behavior appear to be mediated to a large degree by the regulation and release of a single volatile—MeSA. However, potential contribution of other volatiles in addition to MeSA was not definitively falsified. Our results indicate upregulated release of MeSA is induced during a period lasting ~6 months and when disease symptoms are not yet expressed visually. HLB is a disease characterized by a long latent period in the plant, which is one of the many characteristics hindering its management in agriculture (Bové, 2006; Wang and Trivedi, 2013). However, as plants begin to decline and fully express disease symptoms >12 months after infection, MeSA mediated effects on vector behavior diminish to a baseline characterizing uninfected plants. Our results suggest that this regulation is mediated by decreased expression of CsSAMT, thus decreasing methylation of SA to MeSA. Our experiments were conducted on seedling trees and under laboratory conditions. Such changes in volatile emission may occur at a slower rate in mature trees under field conditions. Complementary experiments conducted under field conditions on mature trees and with repeated measures are needed to fully elucidate the time course of MeSA and CsSAMT fluctuations.
Author Contributions
XM, MC, EK, and LS designed the study. XM, MC, and EK performed the study. XM and MC analyzed the data. XM and MC wrote the first version of the manuscript, which was edited and approved by all authors.
Conflict of Interest Statement
The authors declare that the research was conducted in the absence of any commercial or financial relationships that could be construed as a potential conflict of interest.
Acknowledgments
This research was supported by the Citrus Research and Development Foundation. We thank Angelique Hoyte, Laura Pescitelli King for technical assistance.
Supplementary Material
The Supplementary Material for this article can be found online at: https://www.frontiersin.org/articles/10.3389/fevo.2018.00185/full#supplementary-material
References
Aksenov, A. A., Martini, X., Zhao, W., Stelinski, L. L., and Davis, C. E. (2014). Synthetic blends of volatile, phytopathogen-induced odorants can be used to manipulate vector behavior. Front. Ecol. Evol. 2:78. doi: 10.3389/fevo.2014.00078
Alquézar, B., Volpe, H. X. L., Magnani, R. F., De Miranda, M. P., Santos, M. A., Wulff, N. A., et al. (2017). β-caryophyllene emitted from a transgenic Arabidopsis or chemical dispenser repels Diaphorina citri, vector of Candidatus Liberibacters. Sci. Rep. 7:5639. doi: 10.1038/s41598-017-06119-w
Bassanezi, R. B., Montesino, L. H., and Stuchi, E. S. (2009). Effects of huanglongbing on fruit quality of sweet orange cultivars in Brazil. Eur. J. Plant Pathol. 125, 565–572. doi: 10.1007/s10658-009-9506-3
Beloti, V. H., Santos, F., Alves, G. R., Bento, J. M. S., and Yamamoto, P. T. (2017). Curry leaf smells better than citrus to females of Diaphorina citri (Hemiptera: Liviidae). Arthropod. Plant. Interact. 11, 709–716. doi: 10.1007/s11829-017-9524-6
Bové, J. (2006). Huanglongbing: a destructive, newly-emerging, century-old disease of citrus. J. Plant Pathol. 88, 7–37. doi: 10.4454/jpp.v88i1.828
Bruce, T. J. A., Wadhams, L. J., and Woodcock, C. M. (2005). Insect host location: a volatile situation. Trends Plant Sci. 10, 269–274. doi: 10.1016/j.tplants.2005.04.003
Chen, F., D'Auria, J. C., Tholl, D., Ross, J. R., Gershenzon, J., Noel, J. P., et al. (2003). An Arabidopsis thaliana gene for methylsalicylate biosynthesis, identified by a biochemical genomics approach, has a role in defense. Plant J. 36, 577–588. doi: 10.1046/j.1365-313X.2003.01902.x
Coutinho-Abreu, I. V., McInnally, S., Forster, L., Luck, R., and Ray, A. (2014). Odor coding in a disease-transmitting herbivorous insect, the Asian citrus psyllid. Chem. Senses 39, 539–549. doi: 10.1093/chemse/bju023
Coy, M. R., Hoffmann, M., Kingdom Gibbard, H. N., Kuhns, E. H., Pelz-Stelinski, K. S., and Stelinski, L. L. (2014). Nested-quantitative PCR approach with improved sensitivity for the detection of low titer levels of Candidatus Liberibacter asiaticus in the Asian citrus psyllid, Diaphorina citri Kuwayama. J. Microbiol. Methods 102, 15–22. doi: 10.1016/j.mimet.2014.04.007
Davis, T. S., Horton, D. R., Munyaneza, J. E., and Landolt, P. J. (2012). Experimental infection of plants with an herbivore-associated bacterial endosymbiont influences herbivore host selection behavior. PLoS ONE 7:e49330. doi: 10.1371/journal.pone.0049330
Eigenbrode, S. D., Ding, H., Shiel, P., and Berger, P. H. (2002). Volatiles from potato plants infected with potato leafroll virus attract and arrest the virus vector, Myzus persicae (Homoptera: Aphididae). Proc. Biol. Sci. 269, 455–460. doi: 10.1098/rspb.2001.1909
Fereres, A., Peñaflor, M. F. G. V., Favaro, C. F., Azevedo, K. E. X., Landi, C. H., Maluta, N. K. P., et al. (2016). Tomato infection by whitefly-transmitted circulative and non-circulative viruses induce contrasting changes in plant volatiles and vector behaviour. Viruses 8:225. doi: 10.3390/v8080225
Fu, Z. Q., and Dong, X. (2013). Systemic acquired resistance: turning local infection into global defense. Annu. Rev. Plant Biol. 64, 839–863. doi: 10.1146/annurev-arplant-042811-105606
Glinwood, R. T., and Pettersson, J. (2000). Change in response of Rhopalosiphum padi spring migrants to the repellent winter host component methyl salicylate. Entomol. Exp. Appl. 94, 325–330. doi: 10.1046/j.1570-7458.2000.00634.x
Gottwald, T. R., Bassanezi, R. B., and Paulo, S. (2007). Citrus huanglongbing : the pathogen and its impact plant health progress. Plant Manag. Netw. 6, 1–18. doi: 10.1094/PHP-2007-0906- 01-RV
Gross, J., Mayer, C. J., and Eben, A. (2011). Development of innovative methods for trapping phytoplasma vectors by attractive infochemicals. Bull. Insectol. 64, 141–142.
Hardie, J., Isaacs, R., Pickett, J. A., Wadhams, L. J., and Woodcock, C. M. (1994). Methyl salicylate and (–)-(1R, 5S)-myrtenal are plant-derived repellents for black bean aphid, Aphis fabae Scop.(Homoptera: Aphididae). J. Chem. Ecol. 20, 2847–2855. doi: 10.1007/BF02098393
Hulcr, J., and Stelinski, L. L. (2017). The ambrosia symbiosis: from evolutionary ecology to practical management. Annu. Rev. Entomol. 62, 285–303. doi: 10.1146/annurev-ento-031616-035105
Koschier, E. H., Hoffmann, D., and Riefler, J. (2007). Influence of salicylaldehyde and methyl salicylate on post-landing behaviour of Frankliniella occidentalis Pergande. J. Appl. Entomol. 131, 362–367. doi: 10.1111/j.1439-0418.2007.01191.x
Larkin, M. A., Blackshields, G., Brown, N. P., Chenna, R., McGettigan, P. A., McWilliam, H., et al. (2007). Clustal W and Clustal X version 2.0. Bioinformatics 23, 2947–2948. doi: 10.1093/bioinformatics/btm404
Li, J., Pang, Z., Trivedi, P., Zhou, X., Ying, X., Jia, H., et al. (2017). “Candidatus Liberibacter asisaticus” encodes a functional salicylic acid (SA) hysdroxylase that degrades SA to suppress plant defenses. Mol. Plant Microbe Interact. 30, 620–630. doi: 10.1094/MPMI-12-16-0257-R
Mann, R., Rouseff, R., Smoot, J., Castle, W., and Stelinski, L. (2011). Sulfur volatiles from Allium spp. affect Asian citrus psyllid, Diaphorina citri Kuwayama (Hemiptera: Psyllidae), response to citrus volatiles. Bull. Entomol. Res. 101, 89–97. doi: 10.1017/S0007485310000222
Mann, R. S., Ali, J. G., Hermann, S. L., Tiwari, S., Pelz-Stelinski, K. S., Alborn, H. T., et al. (2012). Induced release of a plant-defense volatile “deceptively” attracts insect vectors to plants infected with a bacterial pathogen. PLoS Pathog. 8:e1002610. doi: 10.1371/journal.ppat.1002610
Mann, R. S., Qureshi, J. A., Stansly, P. A., and Stelinski, L. L. (2010). Behavioral response of Tamarixia radiata (Waterston) (Hymenoptera: Eulophidae) to volatiles emanating from Diaphorina citri Kuwayama (Hemiptera: Psyllidae) and citrus. J. Insect Behav. 23, 447–458. doi: 10.1007/s10905-010-9228-6
Martini, X., Hughes, M. A., Killiny, N., George, J., Lapointe, S. L., Smith, J. A., et al. (2017). The fungus Raffaelea lauricola modifies behavior of its symbiont and vector, the redbay ambrosia beetle (Xyleborus Glabratus), by altering host plant volatile production. J. Chem. Ecol. 43, 519–531. doi: 10.1007/s10886-017-0843-y
Martini, X., Kuhns, E. H., Hoyte, A., and Stelinski, L. L. (2014a). Plant volatiles and density-dependent conspecific female odors are used by Asian citrus psyllid to evaluate host suitability on a spatial scale. Arthropod. Plant. Interact. 8, 453–460. doi: 10.1007/s11829-014-9326-z
Martini, X., Pelz-Stelinski, K. S., and Stelinski, L. L. (2014b). Plant pathogen-induced volatiles attract parasitoids to increase parasitism of an insect vector. Front. Ecol. Evol. 2:8. doi: 10.3389/fevo.2014.00008
Martini, X., and Stelinski, L. L. (2017). Drought stress affects response of phytopathogen vectors and their parasitoids to infection- and damage-induced plant volatile cues. Ecol. Entomol. 42, 721–730. doi: 10.1111/een.12439
Martini, X., Willett, D. S., Kuhns, E. H., and Stelinski, L. L. (2016). Disruption of vector host preference with plant volatiles may reduce spread of insect-transmitted plant pathogens. J. Chem. Ecol. 42, 357–367. doi: 10.1007/s10886-016-0695-x
Mauck, K. E., De Moraes, C. M., and Mescher, M. C. (2010). Deceptive chemical signals induced by a plant virus attract insect vectors to inferior hosts. Proc. Natl. Acad. Sci. U.S.A. 107, 3600–3605. doi: 10.1073/pnas.0907191107
Mayer, C. J., Vilcinskas, A., and Gross, J. (2008a). Pathogen-induced release of plant allomone manipulates vector insect behavior. J. Chem. Ecol. 34, 1518–1522. doi: 10.1007/s10886-008-9564-6
Mayer, C. J., Vilcinskas, A., and Gross, J. (2008b). Phytopathogen lures its insect vector by altering host plant odor. J. Chem. Ecol. 34, 1045–1049. doi: 10.1007/s10886-008-9516-1
Negre, F., Kolosova, N., Mann, C., and Dudareva, N. (2002). Novel-S-adenosyl-L-methionine:salicylic methyltransferase, an enzyme responsible for biosynthesis of methyl salicylate and methyl benzoate, is not involved in floral scent production in snapdragon flowers. Arch. Biochem. Biophys. 406, 261–270. doi: 10.1016/S0003-9861(02)00458-7
Nehela, Y., Hijaz, F., Elzaawely, A. A., El-Zahaby, H. M., and Killiny, N. (2018). Citrus phytohormonal response to Candidatus Liberibacter asiaticus and its vector Diaphorina citri. Physiol. Mol. Plant Pathol. 102, 24–35. doi: 10.1016/j.pmpp.2017.11.004
Page, R. D. M. (1996). Treeview : an application to display phylogenetic trees on personal computers. Comput. Appl. Biosci. 12, 357–358.
Park, S. W., Kaimoyo, E., Kumar, D., Mosher, S., and Klessig, D. F. (2007). Methyl salicylate is a critical mobile signal for plant systemic acquired resistance. Science 318, 113–116. doi: 10.1126/science.1147113
Patt, J. M., Robbins, P. S., Niedz, R., McCollum, G., and Alessandro, R. (2018). Exogenous application of the plant signalers methyl jasmonate and salicylic acid induces changes in volatile emissions from citrus foliage and influences the aggregation behavior of Asian citrus psyllid (Diaphorina citri), vector of Huanglongbing. PLoS ONE 13:e0193724. doi: 10.1371/journal.pone.0193724
Patt, J. M., and Setamou, M. (2010). Responses of the Asian citrus psyllid to volatiles emitted by the flushing shoots of its rutaceous host plants. Environ. Entomol. 39, 618–624. doi: 10.1603/EN09216
Pelz-Stelinski, K., Brlansky, R., and Rogers, M. (2010). Transmission parameters for Candidatus Liberibacter asiaticus by Asian citrus psyllid (Hemiptera: Psyllidae). J. Econ. Entomol. 103, 1531–1541. doi: 10.1603/EC10123
Robbins, P. S., Alessandro, R. T., Stelinski, L. L., and Lapointe, S. L. (2012). Volatile profiles of young leaves of Rutaceae spp. varying in susceptibility to the Asian citrus psyllid (Hemiptera: Psyllidae). Florida Entomol. 95, 774–776. doi: 10.1653/024.095.0331
Rodriguez-Saona, C., Kaplan, I., Braasch, J., Chinnasamy, D., and Williams, L. (2011). Field responses of predaceous arthropods to methyl salicylate: a meta-analysis and case study in cranberries. Biol. Control 59, 294–303. doi: 10.1016/j.biocontrol.2011.06.017
Ross, J., Nam, K., D'Auria, J., and Pichersky, E. (1999). S-adenosyl-L-methionine:salicylic acid carboxyl methyltransferase, an enzyme involved in floral scent production and plant defense, represents a new class of plant methyltransferases. Arch. Biochem. Biophys. 376, 9–16. doi: 10.1006/abbi.1999.1255
Shapiro, L., De Moraes, C. M., Stephenson, A. G., Mescher, M. C., and van der Putten, W. (2012). Pathogen effects on vegetative and floral odours mediate vector attraction and host exposure in a complex pathosystem. Ecol. Lett. 15, 1430–1438. doi: 10.1111/ele.12001
Stein, S. E., Anzor, M., White, E., Zaikin, V., Zhu, D., Sparkman, O. D., et al. (2011). NIST Standard Reference Database 1A. 1–65.
Tieman, D., Zeigler, M., Schmelz, E., Taylor, M., Rushing, S., Jones, J., et al. (2010). Functional analysis of a tomato salicylic acid methyl transferase and its role in synthesis of the flavor volatile methyl salicylate. Plant J. 62, 113–123. doi: 10.1111/j.1365-313X.2010.04128.x
Tsai, J. H., and Liu, Y. H. (2000). Biology of Diaphorina citri (Homoptera: Psyllidae) on four host plants. J. Econ. Entomol. 93, 1721–1725. doi: 10.1603/0022-0493-93.6.1721
Walters, D., and Heil, M. (2007). Costs and trade-offs associated with induced resistance. Physiol. Mol. Plant Pathol. 71, 3–17. doi: 10.1016/j.pmpp.2007.09.008
Wang, N., and Trivedi, P. (2013). Citrus huanglongbing: a newly relevant disease presents unprecedented challenges. Phytopathology 103, 652–665. doi: 10.1094/PHYTO-12-12-0331-RVW
Werner, B. J., Mowry, T. M., Bosque-Pérez, N. A., Hongjian, D., and Eigenbrode, S. D. (2009). Changes in green peach aphid responses to potato leafroll virus—induced volatiles emitted during disease progression. Environ. Entomol. 38, 1429–1438. doi: 10.1603/022.038.0511
Wilgenbusch, J. C., and Swofford, D. (2003). Inferring evolutionary trees with PAUP*. Curr. Protoc. Bioinformatics 1, Unit 6.4 doi: 10.1002/0471250953.bi0604s00
Zhu, J., and Park, K. C. (2005). Methyl salicylate, a soybean aphid-induced plant volatile attractive to the predator Coccinella septempunctata. J. Chem. Ecol. 31, 1733–1746. doi: 10.1007/s10886-005-5923-8
Keywords: huanglongbing, pathogen induced volatiles, phytopathogenic bacteria, psylloidea, methyl salicylate, salicylic acid methyl transferase
Citation: Martini X, Coy M, Kuhns E and Stelinski LL (2018) Temporal Decline in Pathogen-Mediated Release of Methyl Salicylate Associated With Decreasing Vector Preference for Infected Over Uninfected Plants. Front. Ecol. Evol. 6:185. doi: 10.3389/fevo.2018.00185
Received: 19 July 2018; Accepted: 26 October 2018;
Published: 16 November 2018.
Edited by:
Juergen Gross, Julius Kühn-Institut, GermanyReviewed by:
Martin James Steinbauer, La Trobe University, AustraliaIslam S. Sobhy, KU Leuven, Belgium
Copyright © 2018 Martini, Coy, Kuhns and Stelinski. This is an open-access article distributed under the terms of the Creative Commons Attribution License (CC BY). The use, distribution or reproduction in other forums is permitted, provided the original author(s) and the copyright owner(s) are credited and that the original publication in this journal is cited, in accordance with accepted academic practice. No use, distribution or reproduction is permitted which does not comply with these terms.
*Correspondence: Xavier Martini, eG1hcnRpbmlAdWZsLmVkdQ==
†Present Address: Monique Coy, Corteva Agriscience, Johnston, IA, United States