- 1Centre for Environmental and Marine Studies, University of Aveiro, Aveiro, Portugal
- 2Center for Molecular Biodiversity, Zoological Research Museum Alexander Koenig, Bonn, Germany
- 3Insitute for Evolutionary Biology and Ecology, University of Bonn, Bonn, Germany
- 4Institute of Human Genetics, School of Medicine & University Hospital Bonn, University of Bonn, Bonn, Germany,
- 5Department of Genomics, Life&Brain Center, University of Bonn, Bonn, Germany
The enigmatic association of photosynthetically active chloroplasts from algae and some sacoglossan sea slugs, called functional kleptoplasty, is a functional unique system of photosymbioses observed in metazoans. Besides the specific adaptations of the slugs necessary to incorporate and maintain the plastids, the organelles need to ensure optimal photosynthesis. Photoprotective mechanisms in the plastids, namely the xanthophyll cycle (XC) and the high-energy dependent quenching (qE) part of the non-photochemical quenching (NPQ), and the repair of the D1 protein of Photosystem II (PSII) are considered crucial for kleptoplast longevity in the slugs. Here, we studied the sea slugs Elysia viridis fed with the naturally occurring XC and qE-deficient Bryopsis hypnoides, and E. timida fed on Acetabularia acetabulum, an alga that possesses both mechanisms. The aim of the study was to understand (i) whether qE remains active after ingestion of kleptoplasts by E. timida, (ii) how different light intensities affect the photosynthetic activity of kleptoplasts with and without photoprotection mechanisms, and (iii) if the kleptoplasts are able to repair photodamaged D1 protein. With regard to NPQ, freshly incorporated kleptoplasts responded to different light stress in the same manner as the chloroplasts in their native host algae. Even after 3 weeks of incorporation the qE part of NPQ was present in the kleptoplasts of E. timida. However, the presence of the qE component of NPQ did not prevent the kleptoplasts from significant PSII photoinactivation under high light intensities. This is probably due to the fact that the kleptoplasts have a reduced PSII repair capacity, despite plastid encoded repair mechanisms in every sacoglossan food source. Hence, photoprotective mechanisms are probably not a key factor explaining kleptoplast longevity in Sacoglossa sea slugs.
Introduction
Oxygenic photosynthesis is considered a key event in the evolution of organisms, yet it is restricted to bacteria, algae and higher plants (Fischer et al., 2016). Animals are heterotrophic and can only achieve the benefits of oxygenic photosynthesis by establishing a symbiosis with a phototrophic organism (Melo Clavijo et al., 2018). Some members of the gastropod taxon Sacoglossa go one step further and only retain the chloroplasts of their algae prey (Rumpho et al., 2007; Händeler et al., 2009) that are incorporated into cells of the digestive gland system (then called kleptoplasts). These kleptoplasts, either stemming from Ulvophyceae or the heterokontophyte Vaucheria littorea (Händeler et al., 2009; Christa et al., 2014c), may be kept photosynthetically active for weeks to months (Rumpho et al., 2000), a situation commonly known as “functional kleptoplasty.” Slugs are classified based on the activity of the kleptoplasts during starvation (Händeler et al., 2009) as non-retention (NR), short-term-retention (StR, up to 2 weeks) and long-term-retention (LtR, months). Recently, this categorization was extended to include non-incorporation (NI) (Rauch et al., 2018). Theory has it, that StR evolved at least twice independently in the Sacoglossa, followed by multiple evolutions of LtR in six different taxa (Christa et al., 2015).
Considering the unique occurrence of photosynthetically active plastids inside an animal it is no wonder that research has tried to uncover if the plastids might influence the slugs' gene expression profile (de Vries et al., 2015; Chan et al., 2018) or even their behavior (Schmitt and Waegele, 2011; Cartaxana et al., 2018) and what and how much the slugs gain from the alien organelle (see Wägele and Martin, 2014). However, while in corals and other photosymbiotic systems the nutritional support of the symbionts can make up more than 95% of the energy demands of the host, the contribution of the kleptoplasts to the physiology of the slugs is controversial, with estimates ranging from a minimum of 1% when starving (Rauch et al., 2017) up to 60% when feeding (Raven et al., 2001). Independent of the exact number, it is nevertheless likely that the alien organelles do provide some support, maybe rather as some kind of larder (Christa et al., 2014d; de Vries et al., 2014a,b), that might help the slugs in times of starvation.
Putting aside the potential relevance of kleptoplasts for the slugs' survival, the fact that kleptoplasts remain photosynthesizing in an animal host is nevertheless fascinating. About 2,000 proteins are needed to support photosynthesis in algae and plants, but only a fraction is encoded in the genome of plastids (Timmis et al., 2004) and none of the relevant genes were found in the slugs' genomes investigated so far (Wägele et al., 2011; Bhattacharya et al., 2013; de Vries et al., 2015; Han et al., 2015). This led to the search for alternative factors that enable kleptoplast longevity, and plastid photoprotection mechanisms came into focus (Jesus et al., 2010; Cruz et al., 2015). Photoprotection mechanisms are essential for balancing the absorption and use of light to fuel photosynthesis and the dissipation of absorbed light that is harmful (Chow and Aro, 2005). Excessive light intensities generate reactive oxygen species (ROS) that mainly target and cause damage to the central transmembrane D1 protein of Photosystem II (PSII) (Tyystjärvi and Aro, 1996), which is crucial for PSII function. Thus, photoprotection mechanisms operate to minimize D1 photodamage and ensure an optimal photosynthesis under the given light intensity.
Among the main photoprotection mechanisms are the xanthophyll cycle (XC) and the high-energy quenching (qE) component of the non-photochemical quenching (NPQ) (Jahns and Holzwarth, 2012; Goss and Lepetit, 2015). In green algae and land plants, the XC is based on the light-dependent conversion of the pigment violaxanthin to zeaxanthin, in red algae and stramenopiles on the light dependent conversion of diadinoxanthin to diatoxanthin (Demmig-Adams and Adams, 1996; Jahns and Holzwarth, 2012; Goss and Lepetit, 2015), and qE on the dissociation of antennae complexes from the PSII (Goss and Lepetit, 2015). Both mechanisms are generally widespread throughout algae and plants, yet some members of the Bryopsidales (Ulvophyceae) are XC and qE deficient (Christa et al., 2017; Handrich et al., 2017). This fact is particularly interesting in the context of kleptoplast longevity of sacoglossan sea slugs, because these mollusks feed primarily on ulvophycean algae to obtain their kleptoplasts. The relationship between the presence of photoprotection mechanisms and kleptoplast longevity is still puzzling. A variety of slugs that keep kleptoplasts active for more than 4 weeks feed on XC and qE-deficient species (Maeda et al., 2012; Christa et al., 2013), yet the same is true for species that feed on algae possessing these photoprotection mechanism (Greene, 1970; Händeler et al., 2009; Christa et al., 2014b; Schmitt et al., 2014). Nevertheless, under any given light intensity, damage to D1 occurs, independently of the presence or absence of photoprotection mechanisms. Once damaged, D1 needs to be pulled out from the reaction center and newly incorporated (Mulo et al., 2012). This so-called D1 turnover is crucial for photosynthetic organisms and largely nuclear controlled, although several tools, including psbA, tufA, and ftsH, are plastid encoded in the food sources of Sacoglossa (de Vries et al., 2013; Leliaert and Lopez-Bautista, 2015).
In particular FTSH, a quality control protease forming hetero- or homo-oligomers (Janska et al., 2013) that removes damaged D1 from the PSII reaction center (Mulo et al., 2012), came into focus as a factor enabling kleptoplast longevity in Sacoglossa (de Vries et al., 2013). However, it is still unknown whether FTSH in kleptoplasts can function, because the M41 domain, needed for proteolysis of damaged D1 (Zhang et al., 2010), is missing in plastid encoded FTSH of the food source of one LtR Sacoglossa (de Vries et al., 2013). But, for other food sources it was not yet analyzed if the M41 domain is also absent in plastid encoded FTSH. If and how the D1 repair and photoprotection mechanisms operate in kleptoplasts and if these mechanisms are essential for kleptoplast longevity is unknown. Yet, evidences by Vieira et al. (2009) let one speculate that the capacity to repair the D1 is highly reduced because high light intensities caused a decrease in kleptoplast PSII activity over time.
In this study, we investigated the NPQ and the repair capacity of kleptoplasts in Elysia viridis (Montagu, 1804) fed on the XC and qE-deficient Bryopsis hypnoides J. V. (Lamouroux, 1809), and in Elysia timida (Risso, 1818) fed on Acetabularia acetabulum P. C. (Silva, 1952), which possesses both mechanisms. Additionally, we set up a starvation experiment to understand if the qE component of NPQ is present and maintained during starvation in E. timida. Further we analyzed all available sequences of FTSH in food sources of Sacoglossa to screen for the M41 domain. Our results show that the net photoinhibition in kleptoplasts is similar irrespective of the presence or absence of photoprotection mechanisms, likely because the D1 repair cycle is not functional once ingested in the slugs' cytosol, which could be based on the absence of the M41 domain in plastid encoded FTSH of sacoglossan food sources.
Materials and Methods
Sea Slug Collection and Culturing
Specimens of E. viridis were collected in Aguda, Portugal (41°02′53.1″N, 8°39′12.0″W) during May and September 2017 from its native food source, Codium tomentosum. All individuals were transferred to the laboratory and fed with approximately 1 g (wet weight) of B. hypnoides (purchased at Experimental Phycology and Culture Collection of Algae at the University of Goettingen (EPSAG), strain nr. 7.86) for at least 1 month prior to the start of experiments. Elysia timida was collected in September 2017 in La Madrague, France (43°2′27,06″N, 6°6′3,463″E), and was fed with A. acetabulum, cultured in our laboratory for several years. For five individuals 15 pieces of A. acetabularia (5–6 cm) were provided. The food algae for both slugs were renewed every third day. All sea slugs and algae were cultured at 19°C under a 12 h day/12 h night cycle at 45 μmol photons m−2 s−1 provided by white LED (precision daylight sunrise 520 LED tubes, Sera, Heinsberg, Germany). A maximum of eight slugs were kept in glass jars with 800 mL Artificial Seawater (ASW) (AB Reef Salt, Aqua Medic, Bissendorf, Germany) with a salinity of 33 and the water was changed every other day. Fresh algae were provided twice a week. The algae were cultured in ASW enriched with Guillard's F/2 medium (Guillard, 1975) and in the case of B. hypnoides with constant aeration to ensure a movement of the alga.
Chlorophyll a Fluorescence Measurements
For chlorophyll a fluorescence measurements of kleptoplasts in E. viridis, E. timida and chloroplasts in B. hypnoides and A. acetabulum we used a Diving PAM (Walz, Effeltrich Germany). Samples of slugs and algae were placed on a microscopic slide, covered with a coverslipand mounted on the leaf clip to assure a constant, 6 mm distance from the optical fiber. All samples were incubated for 30 min in darkness before the measurements began. After dark acclimation, the maximum quantum yield (Fv/Fm; where Fv = Fm-Fo and Fo and Fm are the minimum and maximum fluorescence emitted by dark adapted samples, respectively) was determined by applying a saturation pulse (white light, >4,000 μmol photons m−2s−1). Samples were then exposed to 53, 239, or 879 μmol photons m−2 s−1 for 20 min of white actinic light and afterwards again transferred to darkness (recovery phase) for 30 min. During the experiment, saturation pulses were applied every 5 min to measure the effective PSII quantum yield ΔF/Fm' during exposure to actinic light (ΔF = Fm'-Fs; where Fs and Fm' are the minimum and maximum fluorescence emitted by light adapted samples, respectively), the Fv/Fm during the recovery and the NPQ (calculated as (Fm-Fm')/Fm'). Additionally, we starved E. timida for 1 and 3 weeks under low light (LL, 45 μmol photons m−2 s−1) conditions (see below) and repeated the measurements at 239 μmol photons m−2 s−1.
To test the repair capacity of chloroplasts and kleptoplasts of all species, samples were incubated for 2 h in darkness, either in the absence or the presence of 20 mM lincomycin hydrochloride monohydrate (pH adjusted to 8.1, Alfa Aaser, USA), an inhibitor of prokaryotic gene translation. We then determined the maximum quantum yield (Fv/Fm) and all samples were subsequently exposed to 1,300 μmol photons m−2 s−1 for 1 h of modulated red light (LED panel Openflourcam, Photosystem Instrument, Czech Republic). Afterwards, samples were again incubated in darkness (recovery) for 30 min and then Fv/Fm was determined. In this experiment, chlorophyll fluorescence was measured using a WATER-EDF Universal emitter-detector unit (Gademann Instruments GmbH, Würzburg, Germany) and a PAM-Control Unit (Walz, Effeltrich, Germany) with the optical fiber placed a 2–3 mm over the slugs and the application of a saturation pulse (>4,000 μmol photons m−2 s−1, modulated blue light). The rate constant of PSII inactivation, kPI, was calculated from the decrease in Fv/Fm (expressed as percentage of pre-illumination levels, relFv/Fm) considering the time of exposure (T) of the applied irradiance (E) and in the presence of lincomycin, chosen here to inhibit the repair of PSII (Campbell and Tyystjärvi, 2012), by kPI = ln(relFv/Fm)/T.
Starvation Experiment
The maximum starvation period of E. viridis from the Portuguese coast is around 28 days when feeding on Codium tomentosum or Bryopsis hypnoides. To analyze the effect of light stress conditions but meanwhile ensure to analyze healthy individuals, we starved E. viridis and E. timida for 21 days under (1) continuous dark (Dark), (2) Low Light conditions (LL, 45 μmol photons m−2 s−1) and (3) High Light conditions (HL, 250 μmol photons m−2 s−1) using the same conditions as for the culturing. Individuals were randomly selected and kept individually in a 100 mL plastic container with ASW and subsequently exposed to the different treatments. During the starvation experiment, the water was changed every other day.
Length and Fv/Fm Measurements
The length of all slug specimens was measured once a week by imaging each individual in a glass petri dish with 50 mL of ASW during crawling using a DP21 camera mounted on a SZX12 stereo microscope (Olympus, Tokyo, Japan). The average length of five images of the crawling individuals was used to determine the length. All images were analyzed using Fiji (version 2.0.0, Schindelin et al., 2012). We favored the measurements of length over weight, because measuring the length is non-invasive and less prone to artifacts based on external water content and egg mass deposition that might influence weight measurement. Fv/Fm was measured three times a week as previously described using a Diving PAM (Walz, Germany). The variation of Fv/Fm over time was described by fitting the models used by Vieira et al. (2009). All measurements were performed in biological triplicates.
Statistical Analyzes
Differences between Fv/Fm, NPQ and length among the different experiences were tested using an independent two-group t-test or a one-way Analysis of variance (ANOVA) as implemented in R (v. 3.4.4, R Core Team, 2018).
Analysis of Chloroplast Encoded FTSH Sequences of Food Source of Sacoglossa
We downloaded all available sequences of chloroplast encoded FTSH of Ulvophyceae algae from the National Center for Biotechnology Information (NCBI) web-page (see Supplementary Table 1). All sequences were subject to a hmmscan against the Pfam database (version 31.0) using DoMosaics (v. 0.95, Moore et al., 2013) and domain arrangements were visualized.
Results
Reaction to Light Intensities Is Identical in Kleptoplasts and Chloroplasts From The Same Alga
The Fv/Fm of plastids in A. acetabulum and of kleptoplasts in E. timida were identical (0.72 ± 0.02 and 0.72 ± 0.01, respectively) and also similar to the Fv/Fm of kleptoplasts in B. hypnoides and in E. viridis (0.70 ± 0.03 and 0.70 ± 0.05, respectively). Kleptoplasts in both slug species, generally showed a similar light intensity response of rel Fv/Fm (relative to pre-exposure status) and NPQ as the plastids in their native hosts (Figures 1, 2).
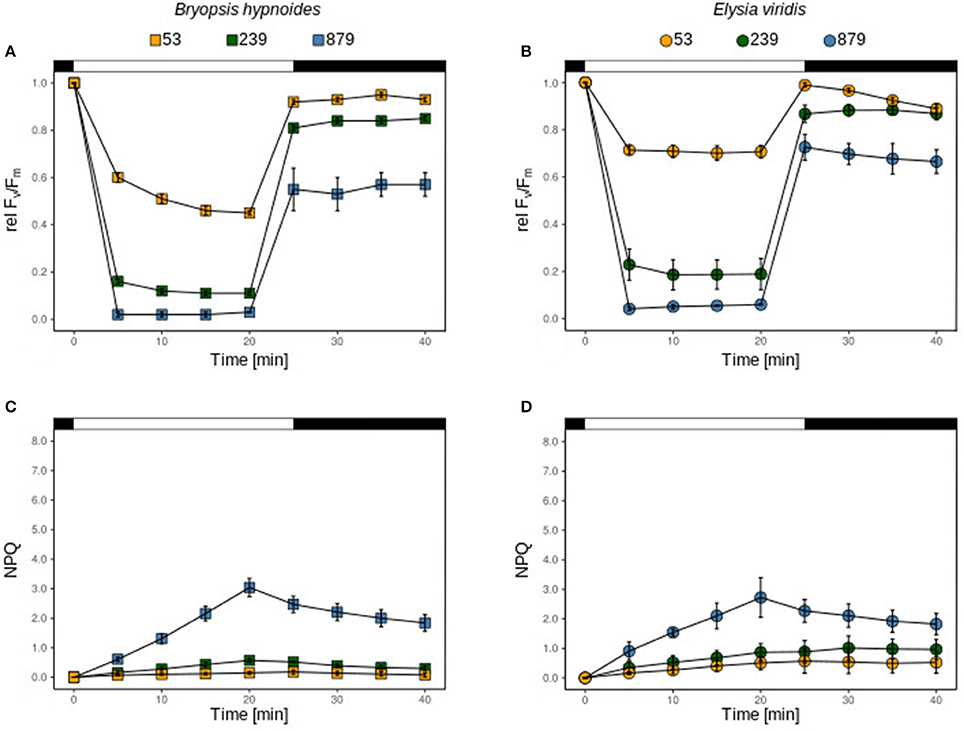
Figure 1. relFv/Fm and NPQ of chloroplasts of B. hypnoides (A,C) and kleptoplasts of freshly fed E. viridis (B,D) under three different light intensities (53, 239, and 879 μmol photons m−2 s−1). The bar represents the dark acclimation phase (first black part), the light exposure time (white part) and the recovery phase (second black part). All measurements were performed in biological triplicates and error bars show the standard deviation.
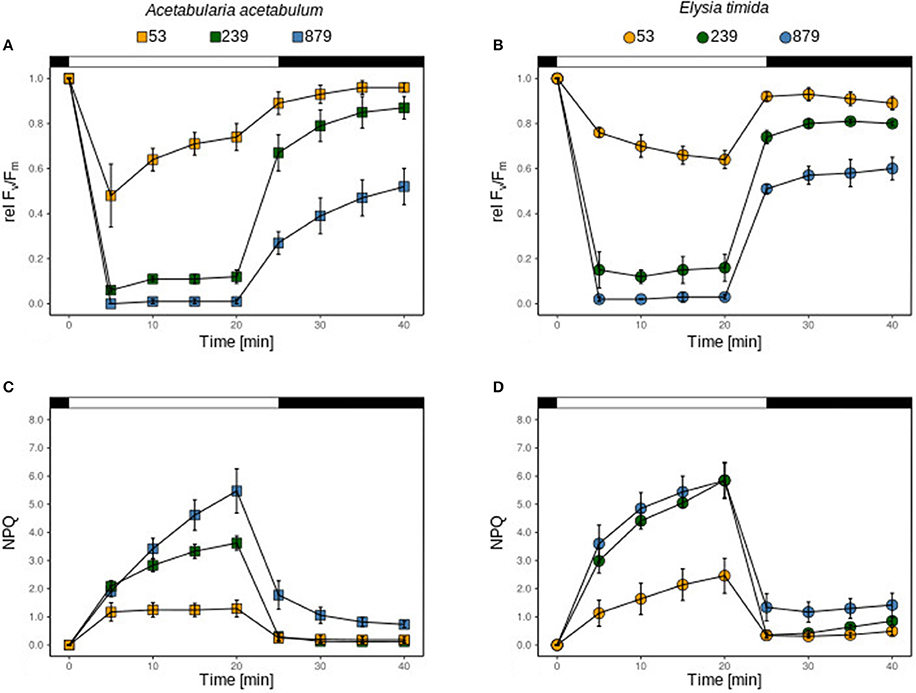
Figure 2. relFv/Fm and NPQ of chloroplasts of A. acetabulum (A,C) and kleptoplasts of freshly fed E. timida (B,D) under three different light intensities (53, 239, and 879 μmol photons m−2 s−1). The bar represents the dark acclimation phase (first black part), the light exposure time (white part) and the recovery phase (second black part). All measurements were performed in biological triplicates and error bars show the standard deviation.
Kleptoplasts in E. viridis showed a significantly higher relΔF/Fm' when exposed to 53 μmol photons m−2 s−1 than the plastids in the algae (t-test; p < 0.05; Figures 1A,B), however, the recovery after 20 min was significantly higher in the algae than in the slugs (t-test; p < 0.05, Figures 1A,B). Under 239 and 879 photons m−2 s−1 relΔFv/Fm', the recovery was similar (Figures 1A,B). The NPQ kinetics were almost identical under each light condition in both, the kleptoplasts in E. viridis and the kleptoplasts in B. hypnoides, (Figures 1C,D).
Regardless of the light intensity applied, NPQ increased slowly and linearly during the light exposure and was almost completely sustained after the light stress (Figures 1C,D).
Under 53 μmol photons m−2 s−1 the plastids in A. acetabulum showed a different kinetics of relFv/Fm in comparison with the kleptoplasts in E. timida; while relΔF/Fm' relaxed in the plastids of the algae, it continuously decreased in the kleptoplasts (Figures 2A,B). This, however, was not significantly different at the end of the light stress (t-test; p = 0.115), but at the end of the recovery phase relFv/Fm relaxed better in algae plastids (t-test; p = 0.085). Under 239 and 879 photons, m−2 s−1 relΔF/Fm' was similar between plastids and kleptoplasts (Figures 2A,B), which was also the case for relFv/Fm after 20 min of recovery (t-test; p = 0.189 and p = 0.300, respectively; Figures 2A,B). Under all light conditions, relFv/Fm in the algae plastids increased continuously during recovery, while in kleptoplasts it did not (Figures 2A,B). The NPQ kinetics in plastids and in kleptoplasts showed, irrespective of the light intensity applied, a fast increase upon illumination and a fast relaxation in the recovery phase (Figures 2C,D). The maximum NPQ measured after 20 min of light exposure was significantly higher in kleptoplasts exposed to 53 and 239 photons m−2 s−1 (t-test; p = 0.09 and p < 0.05, respectively), but identical after exposure to 879 photons m−2 s−1 (p = 0.63).
Photoprotection Mechanisms of Kleptoplasts Are Maintained During Starvation in E. timida
relΔF/Fm' was identical in kleptoplasts of E. timida in freshly fed, 1 week starved and 3 week starved individuals (Figure 3A). However, the recovery was significantly reduced in all starved specimens compared to the fed ones (t-test; p < 0.05 and p = 0.053 for 1 and 3 weeks starved individuals, respectively; Figure 3A), but there was no difference in the recovery between starved specimens (t-test; p = 0.73; Figure 3A). The kinetic of NPQ of kleptoplasts in E. timida was nearly identical to freshly fed conditions during 1 and 3 weeks of starvation (Figure 3B).
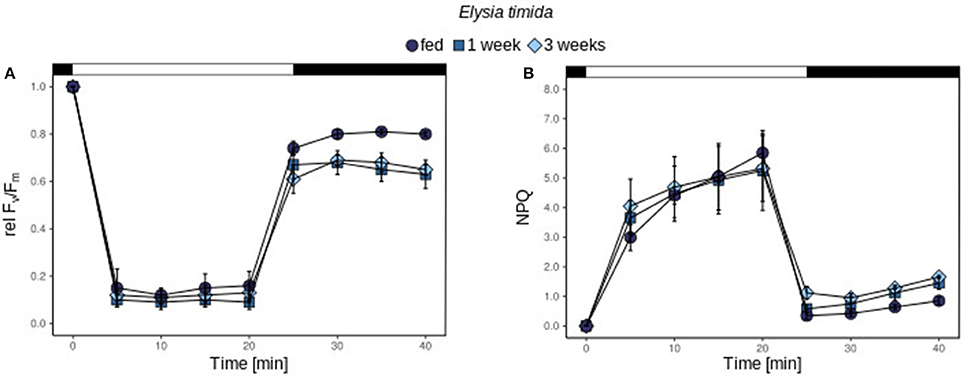
Figure 3. relFv/Fm and NPQ of kleptoplasts of freshly fed E. timida (A,B) under 239 μmol photons m−2 s−1 freshly fed (green circles), starved for 1 week (orange squares) and 3 weeks (blue diamonds). The bar represents the dark acclimation phase (first black part), the light exposure time (white part) and the recovery phase (second black part). All measurements were performed in biological triplicates and error bars show the standard deviation.
High Light Causes Net Photoinactivation of Kleptoplasts
During starvation, the Fv/Fm of kleptoplasts in Elysia viridis maintained in LL and under darkness decreased steadily over the first 2 weeks, which was followed by a rather abrupt decrease after 2 weeks of starvation in LL starved specimens (Figure 4A). In both treatments, the decrease correlated very well with values obtained by fitting the data point to the model proposed in Vieira et al. (2009) (r2 > 0.98; Figure 4A). In contrast, the Fv/Fm followed an exponential decrease (r2 = 0.996; Figure 4A), was already significantly reduced after 1 week of starvation under HL conditions (t-test; p < 0.01; Figure 4A) and was close to zero after 2 weeks (Figure 4A). After 3 weeks of starvation in HL conditions, Fv/Fm reached zero in all samples (Figure 4A). In contrast, the Fv/Fm of kleptoplasts in E. timida remained constant under LL and Dark conditions over the 3-week starvation period (Figure 4B). Under HL conditions the reduction of Fv/Fm during starvation followed a similar exponential decrease to kleptoplasts in E. viridis over the 3 weeks of starvation (r2 = 0.993; Figure 4B).
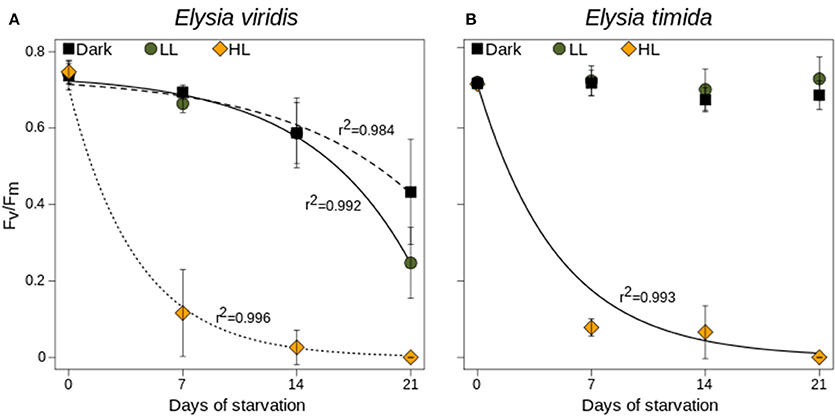
Figure 4. Fv/Fm measurements of E. viridis (A) and E. timida (B) during starvation and under darkness (black circles), 45 μmol photons m−2 s−1 (green circles) and 250 μmol photons m−2 s−1 (orange circles). All measurements were performed in biological triplicates and error bars show the standard deviation. Lines represent fitted values after the model from Vieira et al. (2009).
Length Reduction Is Independent of Light Conditions
All specimens of E. viridis and E. timida decreased in length, regardless of light exposure after 3 weeks' starvation (Figure 5A). In E. viridis the length reduction in Dark starved specimens was more pronounced than in LL and HL starved ones only after 14 days of starvation (p < 0.05; Figure 5A), which was negligible after 21 days of starvation (ANOVA, P = 0.413). In E. timida the length reduction was similar among light treatments, during the first 14 days of starvation (Figure 5B). After 21 days of starvation, the length reduction in LL was significantly lower than in HL and Dark starved specimens (t-test; p < 0.05; Figure 5B). Also, HL starved specimens had a significantly lower length reduction than specimens starved in darkness (p < 0.05; Figure 5B).
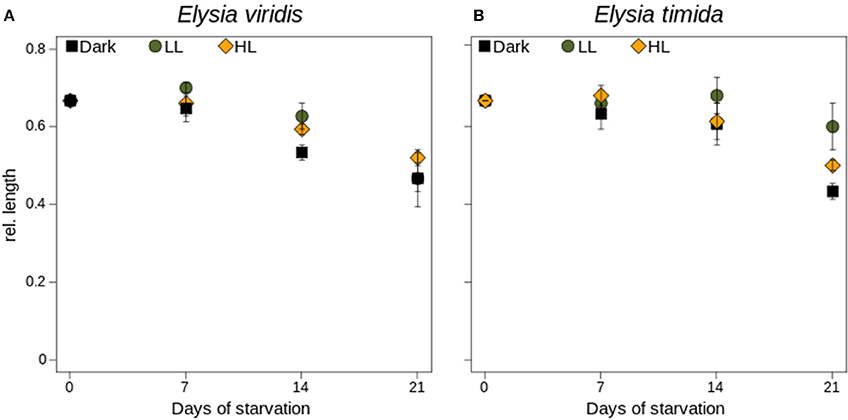
Figure 5. Length measurements of E. viridis (A) and E. timida (B) during starvation and under darkness (black circles), 45 μmol photons m−2 s−1 (green circles) and 250 μmol photons m−2 s−1 (orange circles). Please note that each specimen differed in absolute length, so we used the relative length as more comparable measure. All measurements were performed in biological triplicates and error bars show the standard deviation.
Kleptoplasts Have a Reduced Psii Repair Capacity
In both Sacoglossa species (Figure 6A), there were no significant differences between the Fv/Fm recovery of kleptoplasts under control conditions and when exposed to lincomycin (p > 0.5; Figure 6B). In the algae, however, the chloroplasts recovered significantly better under control conditions than under the lincomycin treatment (p < 0.05; Figure 6B). The rate constant of PSII photoinactivation, kPI, was lower for the kleptoplasts than for their natural host algae, reaching 1.3 × 10−4 and 1.6 × 10−4 s−1 for E. timida and E. viridis, and 3.9 × 10−4 and 2.9 × 10−4 s−1 for A. acetabulum and B. hypnoides, respectively (Figure 6C). Yet, only between kleptoplasts in E. timida and the plastids in A. acetabulum the difference was significant (p > 0.05).
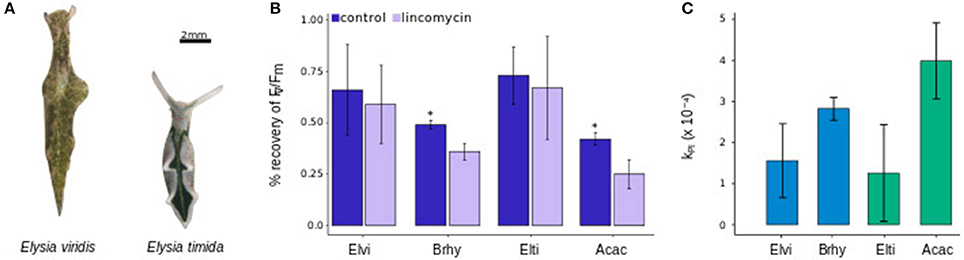
Figure 6. Images of E. viridis and E. timida (A) and the %-recovery of the Fv/Fm of chloroplasts in B. hypnoides (Brhy), A. acetabulum (Acac) and of kleptoplasts in E. viridis (Elvi) and E. timida (Elti) after exposure for 1 h at 1,300 μmol photons m−2 s−1 of red light (B) and the respective calculation of the constant rate of photoinhibition, kPI (C). Scale bar represents 2 mm. Stars indicate significant differences between control and lincomycin treated samples. All measurements were performed in biological triplicates and error bars show the standard deviation.
Ulvophyceaen Plastid Encoded FTSH Lack the M41 Domain
In the heterokontophyte Vaucheria littorea, FTSH consists of a AAA+ metalloprotease domain and a M41 domain (Figure 7). However, in all available chloroplasts genomes of Ulvophyceae, the AAA+ metalloprotease domain is split into two parts and the M41 domain is absent (Figure 7).
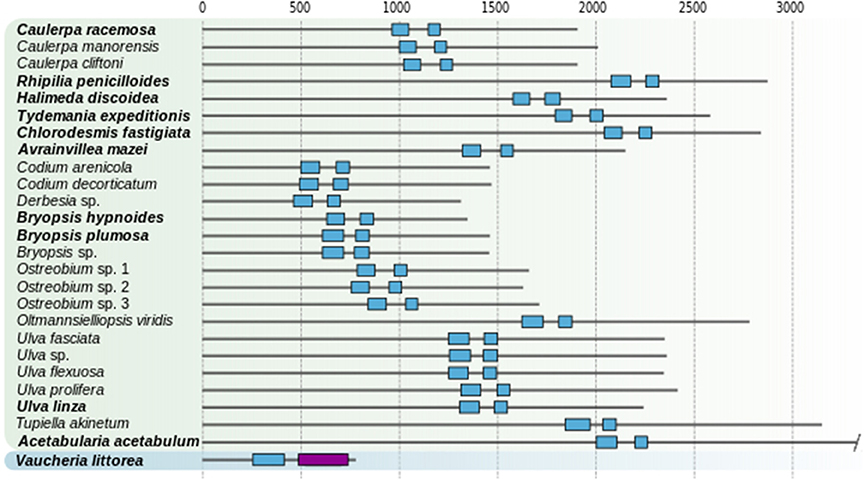
Figure 7. Domain architecture of FTSH proteins in Ulvophyceae and one heterokontophyte. Sacoglossan food sources are highlighted in bold. Ulvophyceae highlighted in green box, the heterokontophyte in blue.
Discussion
Our results revealed that photoprotection mechanisms, i.e., the qE component of NPQ, are still active during starvation in kleptoplasts of E. timida and may enhance kleptoplast longevity under light conditions similar to those of culturing. Despite this, they are not sufficient to prevent the kleptoplast from the same degree of net photoinactivation under excessive light intensities suffered by the kleptoplasts that lack the qE component, and are not sufficient to guarantee a high photosynthetic activity in starving conditions under excessive light intensities. Although every plastid genome of sacoglossan food sources possesses the postulated repair tools, including a copy of the ftsH gene, kleptoplasts stemming from Ulvophyceae alga seem to have only a very limited capacity for PSII repair.
After more than 30 years of intensive study of functional kleptoplasty in sacoglossan sea slugs, little is known about the fundamentals of how the kleptoplasts can stay active in an animal cytosol. Clearly, there is no algal genomic support (Pelletreau et al., 2011; Wägele et al., 2011; Bhattacharya et al., 2013), neither through remnants of an algae nucleus nor through a eukaryotic horizontal gene transfer (Rauch et al., 2015). It is still unknown whether the slugs are able to actively support the kleptoplasts to stay functional. Understanding the basics of kleptoplast photophysiology is thus necessary to find factors that might be beneficial for an autonomous functioning of the photosynthetic apparatus (Handrich et al., 2017). Therefore, photoprotection mechanisms might be important to reduce PSII photoinactivation (Serôdio et al., 2014; Christa et al., 2017) and an active repair of damaged D1 would ensure continuing PSII activity (de Vries et al., 2013).
The situation is more complicated, however. Many Sacoglossa feed on alga that lack important photoprotection mechanisms, such as the xanthophyll cycle (XC) and the qE part of NPQ (Cruz et al., 2015; Christa et al., 2017), and with regard to the kleptoplast longevity, there is no difference between the various retention forms since NR, StR and LtR forms feed on both types of algae and keep them for comparable starvation periods (Händeler et al., 2010; Christa et al., 2014b). The most prominent examples are Elysia chlorotica, which feeds on Vaucheria litorea, an alga that possess both mechanisms (Cruz et al., 2015), and Plakobranchus ocellatus, that consumes only XC and qE-deficient species (Maeda et al., 2012; Christa et al., 2013; Wade and Sherwood, 2018). In both species, the kleptoplasts stay active for more than 5 months (Greene, 1970; Händeler et al., 2009). Our results are similar to those that were obtained in studies in which species have been starved under light intensities that exceeded their culturing light conditions and resulted in a significant reduction of kleptoplast photosynthesis, independently of the presence or absence of the XC and qE (Vieira et al., 2009; Klochkova et al., 2010; Christa et al., 2013, 2015). During the HL treatment our data align with those from Vieira et al. (2009) in showing an exponential decrease of Fv/Fm, a pattern expected to occur in the absence of PSII repair (Campbell and Tyystjärvi, 2012). Here we show that, even in the presence of functional photoprotection mechanisms, that is the XC and the qE component of NPQ, which are active for at least 3 weeks of starvation, excessive light intensities lead to the same photoinactivation as in kleptoplasts of a species lacking these mechanisms. It appears that the activity of the XC and qE in kleptoplasts of A. acetabularia in E. timida might only be relevant for kleptoplast longevity under low light starving conditions.
A recent hypothesis suggests that the repair of damaged D1 might be key to kleptoplast longevity in sacoglossan sea slugs (de Vries et al., 2013), despite doubts based on photoinactivation under excessive light conditions that were previously raised (Vieira et al., 2009).
The presence of necessary chloroplast genome encoded components for the D1 protein, including the psbA, tufA, and ftsH genes, led to the hypothesis that kleptoplast longevity might be correlated to the presence of these repair components in certain plastids genomes of their food algae (de Vries et al., 2013). The hypothesis was based on the observation that kleptoplasts of Bryopsis hypnoides did not encode ftsH in the chloroplast (cp) genome but are degrading at a faster rate than kleptoplasts of A. acetabulum, in which cp genome ftsH is encoded. Unfortunately, the original publication of the Bryopsis hypnoides genome (Lü et al., 2011) lacked the annotation of ftsH, which was re-annotated later (Leliaert and Lopez-Bautista, 2015). Here, we determined that the ftsH gene in all previously sequenced and annotated cp genomes of Ulvophyceae (see Supplementary Table 1) lack the M41 domain. The M41 domain is crucial for the functionality of FTSH, in which monomers are assembled into hetero-oligomers (Janska et al., 2013) and at least one monomer needs to have the M41 domain (Zhang et al., 2010). It is thus likely that, although cp encoded, FTSH is not functioning in kleptoplasts, because there is no evidence that algal, nuclear encoded counterparts containing a M41 domain are present in the slugs genome (Rauch et al., 2015). Hence, kleptoplasts would not be able to repair damaged D1. This might be different for the heterokontophyte Vaucheria littorea. The cp genome of this alga encodes for a FTSH containing the M41 domain. Thus, it might be that a D1 repair mechanism is functional in kleptoplasts of this alga in Elysia chlorotica. Despite this, based on data currently available, kleptoplast longevity in other sacoglossan species likely does not depend on the repair of the D1 protein.
The contribution of kleptoplasts to the host metabolism is among the most controversially discussed topics in the research of functional kleptoplasty (Christa et al., 2014a; de Vries et al., 2014b; Wägele and Martin, 2014; Pierce et al., 2015). It has generally been assumed that the acquisition of functional kleptoplasts results in a substantial benefit for the slugs, e.g., helping to overcome starvation periods. However, some experimental evidence does not seem to support such claims. As in the present study, it was shown before that the tolerance to starvation is not necessarily linked to the photosynthetic activity of the kleptoplasts. For example, Elysia nicrocapitata was able to endure 5 months of starvation but kleptoplasts lost functionality after a couple of days (Klochkova et al., 2010). These 5 months even out-compete both Elysia species used here and others in which the kleptoplast are active for 2–3 months (Clark et al., 1981; Middlebrooks et al., 2012; Christa et al., 2014c, 2015).
Sacoglossan sea slugs tend to show a more pronounced reduction in body mass and body length under non-photosynthetic conditions, such as darkness or excessive light intensities (Giménez Casalduero and Muniain, 2008; Yamamoto et al., 2013; Cartaxana et al., 2017). These data were mostly interpreted as an indication that slugs starving under photosynthetic conditions gain substantial benefits from nutritional support of the kleptoplasts under starvation, but the situation is not that simple. It is still unknown how much the kleptoplasts might actually contribute to the host metabolism–data from CO2 fixation experiments range from 1% (Rauch et al., 2017) to 60% (Raven et al., 2001)–and thus if the kleptoplasts can compensate for the lack of nutrition. Further, how photosynthates are made accessible to the slugs is unknown, but at present it seems that they are only made accessible for the slugs upon digestion (Laetz et al., 2016, 2017). It appears that the transcriptional response from the slugs to starvation is less dependent on the photosynthetic activity of the kleptoplasts, but rather to the starvation itself (de Vries et al., 2015). Yet, this has only been tested in two species and more data, especially from NR forms, are needed.
Because we do not understand (i) how much nutritional support the slugs get from the kleptoplasts, (ii) how the photosynthates are made accessible to the slugs, (iii) what actually happens to the metabolism of the slugs when they are exposed to different starvation conditions, and (iv) how NR forms can endure similar starvation periods as StR and LtR forms, drawing conclusions about the nutritional support of kleptoplasts to the host metabolism from such data is complicated.
Author Contributions
GC and JS planned and set up the experiments. All authors performed the experiments, analyzed the data and wrote the manuscript.
Funding
We thank the Fundação para a Ciência e a Tecnologia (FCT, SFRH/BPD/109892/2015 to GC), the Alexander-Koenig-Gesellschaft (AKG), the PROMOS Program of the University of Bonn (CS), and the German Research Foundation (DFG, Wa 618/17) for funding of the research. For financial support, thanks are due to the Centre for Environmental and Marine Studies (UID/AMB/50017), FCT/Ministry of Science and Education through national funds, and co-funding by the European Fund For Regional Development, within the PT2020 Partnership Agreement and Compete 2020.
Conflict of Interest Statement
The authors declare that the research was conducted in the absence of any commercial or financial relationships that could be construed as a potential conflict of interest.
Acknowledgments
We want to thank Silja Frankenbach and William Schmitt for helping in algal and animal culturing, and Claudia Etzbauer for laboratory support.
Supplementary Material
The Supplementary Material for this article can be found online at: https://www.frontiersin.org/articles/10.3389/fevo.2018.00121/full#supplementary-material
References
Bhattacharya, D., Pelletreau, K. N., Price, D. C., Sarver, K. E., and Rumpho, M. E. (2013). Genome analysis of Elysia chlorotica egg DNA provides no evidence for horizontal gene transfer into the germ line of this kleptoplastic mollusc. Mol. Biol. Evol. 30, 1843–1852. doi: 10.1093/molbev/mst084
Campbell, D. A., and Tyystjärvi, E. (2012). Parameterization of photosystem II photoinactivation and repair. Biochimica Biophys. Acta Bioenerg. 1817, 258–265. doi: 10.1016/j.bbabio.2011.04.010
Cartaxana, P., Morelli, L., Quintaneiro, C., Calado, G., Calado, R., and Cruz, S. (2018). Kleptoplasts photoacclimation state modulates the photobehaviour of the solar-powered sea slug Elysia viridis. J. Exp. Biol. 221:jeb18046. doi: 10.1242/jeb.180463
Cartaxana, P., Trampe, E., Kühl, M., and Cruz, S. (2017). Kleptoplast photosynthesis is nutritionally relevant in the sea slug Elysia viridis. Sci. Rep. 7:7714. doi: 10.1038/s41598-017-08002-0
Chan, C. X., Vaysberg, P., Price, D. C., Pelletreau, K. N., Rumpho, M. E., and Bhattacharya, D. (2018). Active host response to algal symbionts in the sea slug Elysia chlorotica. Mol. Biol. Evol. 35, 1706–1711. doi: 10.1093/molbev/msy061
Chow, W. S., and Aro, E. M. (2005). “Photoinactivation and mechanisms of recovery,” in Photosystem II. Advances in Photosynthesis and Respiration, Vol. 22, eds T. J. Wydrzynski, K. Satoh, and J. A. Freeman (Dordrecht: Springer), 62–648.
Christa, G., Cruz, S., Jahns, P., Vries, J., Cartaxana, P., Esteves, A. C., et al. (2017). Photoprotection in a monophyletic branch of chlorophyte algae is independent of energy-dependent quenching (qE). New Phytol. 214, 1132–1144. doi: 10.1111/nph.14435
Christa, G., de Vries, J., Jahns, P., and Gould, S. B. (2014a). Switching off photosynthesis: the dark side of sacoglossan slugs. Commun. Integr. Biol. 7:e28029. doi: 10.4161/cib.28029
Christa, G., Gould, S. B., Franken, J., Vleugels, M., Karmeinski, D., Händeler, K., et al. (2014b). Functional kleptoplasty in a limapontioidean genus: phylogeny, food preferences and photosynthesis in Costasiella, with a focus on C. ocellifera (Gastropoda: Sacoglossa). J. Molluscan Stud. 80, 499–507. doi: 10.1093/mollus/eyu026
Christa, G., Händeler, K., Kück, P., Vleugels, M., Franken, J., Karmeinski, D., et al. (2015). Phylogenetic evidence for multiple independent origins of functional kleptoplasty in Sacoglossa (Heterobranchia, Gastropoda). Organ. Divers. Evol. 15, 23–36. doi: 10.1007/s13127-014-0189-z
Christa, G., Händeler, K., Schäberle, T. F., König, G. M., and Wägele, H. (2014c). Identification of sequestered chloroplasts in photosynthetic and non-photosynthetic sacoglossan sea slugs (Mollusca, Gastropoda). Front. Zool. 11:15. doi: 10.1186/1742-9994-11-15
Christa, G., Wescott, L., Schäberle, T. F., König, G. M., and Wägele, H. (2013). What remains after 2 months of starvation? Analysis of sequestered algae in a photosynthetic slug, Plakobranchus ocellatus (Sacoglossa, Opisthobranchia), by barcoding. Planta 237, 559–572. doi: 10.1007/s00425-012-1788-6
Christa, G., Zimorski, V., Woehle, C., Tielens, A. G., Wägele, H., Martin, W. F., et al. (2014d). Plastid-bearing sea slugs fix CO2 in the light but do not require photosynthesis to survive. Proc. R. Soc. London B: Biol. Sci. 281:20132493. doi: 10.1098/rspb.2013.2493
Clark, K. B., Jensen, K. R., Stirts, H. M., and Fermin, C. (1981). Chloroplast symbiosis in a non-Elysiid mollusc, Costasiella lilianae Marcus Hermaeidae: ascoglossa (= Sacoglossa): effects of temperature, light intensity, and starvation on carbon fixation rate. Biol. Bull. 160, 43–54.
Cruz, S., Cartaxana, P., Newcomer, R., Dionisio, G., Calado, R., Serôdio, J., et al. (2015). Photoprotection in sequestered plastids of sea slugs and respective algal sources. Sci. Rep. 5:7904. doi: 10.1038/srep07904
Demmig-Adams, B., and Adams, W. W. (1996). The role of xanthophyll cycle carotenoids in the protection of photosynthesis. Trends Plant Sci. 1, 21–26.
de Vries, J., Christa, G., and Gould, S. B. (2014a). Plastid survival in the cytosol of animal cells. Trends Plant Sci. 19, 347–350. doi: 10.1016/j.tplants.2014.03.010
de Vries, J., Habicht, J., Woehle, C., Huang, C., Christa, G., Wägele, H., et al. (2013). Is ftsH the key to plastid longevity in sacoglossan slugs? Genome Biol. Evol. 5, 2540–2548. doi: 10.1093/gbe/evt205
de Vries, J., Rauch, C., Christa, G., and Gould, S. B. (2014b). A sea slug's guide to plastid symbiosis. Acta Soc. Botanicorum Pol. 83, 415–421. doi: 10.5586/asbp.2014.042
de Vries, J., Woehle, C., Christa, G., Wägele, H., Tielens, A. G., Jahns, P., et al. (2015). Comparison of sister species identifies factors underpinning plastid compatibility in green sea slugs. Proc. R. Soc. Lon. B: Biol. Sci. 282:20142519. doi: 10.1098/rspb.2014.2519
Fischer, W. W., Hemp, J., and Johnson, J. E. (2016). Evolution of oxygenic photosynthesis. Annu. Rev. Earth Planet. Sci. 44, 647–683. doi: 10.1146/annurev-earth-060313-054810
Giménez Casalduero, F., and Muniain, C. (2008). The role of kleptoplasts in the survival rates of Elysia timida (Risso, 1818):(Sacoglossa: Opisthobranchia) during periods of food shortage. J. Exp. Mar. Biol. Ecol. 357, 181–187. doi: 10.1016/j.jembe.2008.01.020
Goss, R., and Lepetit, B. (2015). Biodiversity of NPQ. J. Plant Physiol. 172, 13–32. doi: 10.1016/j.jplph.2014.03.004
Greene, R. (1970). Symbiosis in sacoglossan opisthobranchs: functional capacity of symbiotic chloroplasts. Mar. Biol. 7, 138–142.
Guillard, R. R. L. (1975). “Culture of phytoplankton for feeding marine invertebrates,” in Culture of Marine Invertebrate Animals (Boston, MA: Springer), 29–60.
Händeler, K., Grzymbowski, Y. P., Krug, P. J., and Wägele, H. (2009). Functional chloroplasts in metazoan cells-a unique evolutionary strategy in animal life. Front. Zool. 6:28. doi: 10.1186/1742-9994-6-28
Händeler, K., Waegele, H., Wahrmund, U., Ruedinger, M., and Knoop, V. (2010). Slugs' last meals: molecular identification of sequestered chloroplasts from different algal origins in Sacoglossa (Opisthobranchia, Gastropoda). Mol. Ecol. Resour. 10 968–978. doi: 10.1111/j.1755-0998.2010.02853.x
Han, J. H., Klochkova, T. A., Han, J. W., Shim, J., and Kim, G. H. (2015). Transcriptome analysis of the short-term photosynthetic sea slug Placida dendritica. Algae 30:303–312. doi: 10.4490/algae.2015.30.4.303
Handrich, M., de Vries, J., Gould, S. B., Serôdio, J., and Christa, G. (2017). Ulvophyceaen photophysiology and research opportunities. Perspect. Phycol. 4, 83–92. doi: 10.1127/pip/2017/0074
Jahns, P., and Holzwarth, A. R. (2012). The role of the xanthophyll cycle and of lutein in photoprotection of photosystem II. Biochimica Biophy. Acta Bioenerg. 1817, 182–193. doi: 10.1016/j.bbabio.2011.04.012
Janska, H., Kwasniak, M., and Szczepanowska, J. (2013). Protein quality control in organelles—AAA/FtsH story. Biochimica Biophy. Acta Mol. Cell Res. 1833, 381–387. doi: 10.1016/j.bbamcr.2012.03.016
Jesus, B., Ventura, P., and Calado, G. (2010). Behaviour and a functional xanthophyll cycle enhance photo-regulation mechanisms in the solar-powered sea slug Elysia timida (Risso, 1818). J. Exp. Mar. Biol. Ecol. 395, 98–105. doi: 10.1016/j.jembe.2010.08.021
Klochkova, T. A., Han, J. W., Kim, J. H., Kim, K. Y., and Kim, G. H. (2010). Feeding specificity and photosynthetic activity of Korean sacoglossan mollusks. Algae 25, 217–227. doi: 10.4490/algae.2010.25.4.217
Laetz, E. M., Moris, V. C., Moritz, L., Haubrich, A. N., and Wägele, H. (2017). Photosynthate accumulation in solar-powered sea slugs-starving slugs survive due to accumulated starch reserves. Front. Zool. 14:4. doi: 10.1186/s12983-016-0186-5
Laetz, E. M., Rühr, P. T., Bartolomaeus, T., Preisfeld, A., and Wägele, H. (2016). Examining the retention of functional kleptoplasts and digestive activity in sacoglossan sea slugs. Organ. Divers. Evol. 17, 87–99. doi: 10.1007/s13127-016-0308-0
Lamouroux, J. V. F. (1809). Observations sur la physiologie des algues marines, et description de cinq nouveaux genres de cette famille. N. Bull. Sci. Soc. Philomathique Paris 1, 330–333.
Leliaert, F., and Lopez-Bautista, J. M. (2015). The chloroplast genomes of Bryopsis plumosa and Tydemania expeditiones (Bryopsidales, Chlorophyta): compact genomes and genes of bacterial origin. BMC Genomics 16:204. doi: 10.1186/s12864-015-1418-3
Lü, F., Xü, W., Tian, C., Wang, G., Niu, J., Pan, G., et al. (2011). The Bryopsis hypnoides plastid genome: multimeric forms and complete nucleotide sequence. PLoS ONE 6:e14663. doi: 10.1371/journal.pone.0014663
Maeda, T., Hirose, E., Chikaraishi, Y., Kawato, M., Takishita, K., Yoshida, T., et al. (2012). Algivore or phototroph? Plakobranchus ocellatus (Gastropoda) continuously acquires kleptoplasts and nutrition from multiple algal species in nature. PLoS ONE 7:e42024. doi: 10.1371/journal.pone.0042024
Melo Clavijo, J., Serôdio, J., Donat, A., and Christa, G. (2018). Polymorphic adaptations in metazoans to establish and maintain photosymbioses. Biol. Rev. doi: 10.1111/brv.12430. [Epub ahead of print].
Middlebrooks, M. L., Bell, S. S., and Pierce, S. K. (2012). The kleptoplastic sea slug Elysia clarki prolongs photosynthesis by synthesizing chlorophyll a and b. Symbiosis 57, 127–132. doi: 10.1007/s13199-012-0187-x
Montagu, G. (1804). Description of several marine animals found on the south coast of Devonshire. Trans. Linn. Soc. Lond. 7, 61–85.
Moore, A. D., Held, A., Terrapon, N., Weiner, J. III., and Bornberg-Bauer, E. (2013). DoMosaics: software for domain arrangement visualization and domain-centric analysis of proteins. Bioinformatics 30, 282–283. doi: 10.1093/bioinformatics/btt640
Mulo, P., Sakurai, I., and Aro, E. M. (2012). Strategies for psbA gene expression in cyanobacteria, green algae and higher plants: from transcription to PSII repair. Biochimica Biophy. Acta Bioenerg. 1817, 247–257. doi: 10.1016/j.bbabio.2011.04.011
Pelletreau, K. N., Bhattacharya, D., Price, D. C., Worful, J. M., Moustafa, A., and Rumpho, M. E. (2011). Sea slug kleptoplasty and plastid maintenance in a metazoan. Plant Physiol. 155, 1561–1565. doi: 10.1104/pp.111.174078
Pierce, S. K., Curtis, N. E., and Middlebrooks, M. L. (2015). Sacoglossan sea slugs make routine use of photosynthesis by a variety of species-specific adaptations. Invertebr. Biol. 134, 103–115. doi: 10.1111/ivb.12082
Rauch, C., Jahns, P., Tielens, A. G., Gould, S. B., and Martin, W. F. (2017). On being the right size as an animal with plastids. Front. Plant Sci. 8:1402. doi: 10.3389/fpls.2017.01402
Rauch, C., Tielens, A. G., Serôdio, J., Gould, S. B., and Christa, G. (2018). The ability to incorporate functional plastids by the sea slug Elysia viridis is governed by its food source. Mar. Biol. 165:82. doi: 10.1007/s00227-018-3329-8
Rauch, C., de Vries, J., Rommel, S., Rose, L. E., Woehle, C., Christa, G., et al. (2015). Why it is time to look beyond algal genes in photosynthetic slugs. Genome Biol. Evol. 7, 2602–2607. doi: 10.1093/gbe/evv173
Raven, J., Walker, D., Jensen, K., Handley, L., Scrimgeour, C., and McInroy, S. (2001). What fraction of the organic carbon in sacoglossans is obtained from photosynthesis by kleptoplastids? An investigation using the natural abundance of stable carbon isotopes. Mar. Biol. 138, 537–545. doi: 10.1007/s002270000488
R Core Team (2018). R: A Language and Environment for Statistical Computing. Vienna: R Foundation for Statistical Computing
Risso, A. (1818). Mémoire sur quelques Gastropodes nouveaux, Nudibranches et Tectibranches observés dans la mer de Nice. J. Phys. Chim. Nat. Arts 87, 368–377.
Rumpho, M. E., Dastoor, F. P., Manhart, J. R., and Lee, J. (2007). “The kleptoplast,” in The Structure and Function of Plastids, eds R. R. Wise and J. K. Hoober (Dodrecht: Springer), 451–473.
Rumpho, M. E., Summer, E. J., and Manhart, J. R. (2000). Solar-powered sea slugs. mollusc/algal chloroplast symbiosis. Plant Physiol. 123, 29–38. doi: 10.1104/pp.123.1.29
Schindelin, J., Arganda-Carreras, I., Frise, E., Kaynig, V., Longair, M., Pietzsch, T., et al. (2012). Fiji: an open-source platform for biological-image analysis. Nat. Methods 9, 676–682. doi: 10.1038/nmeth.2019
Schmitt, V., Händeler, K., Gunkel, S., Escande, M. L., Menzel, D., Gould, S. B., et al. (2014). Chloroplast incorporation and long-term photosynthetic performance through the life cycle in laboratory cultures of Elysia timida (Sacoglossa, Heterobranchia). Front. Zool. 11:5. doi: 10.1186/1742-9994-11-5
Schmitt, V., and Waegele, H. (2011). Behavioral adaptations in relation to long-term retention of endosymbiotic chloroplasts in the sea slug Elysia timida (Opisthobranchia, Sacoglossa). Thalassas 27, 226–238.
Serôdio, J., Cruz, S., Cartaxana, P., and Calado, R. (2014). Photophysiology of kleptoplasts: photosynthetic use of light by chloroplasts living in animal cells. Philos. Trans. R. Soc. B Biol. Sci. 369:20130242. doi: 10.1098/rstb.2013.0242
Silva, P. C. (1952). A Review of Nomenclatural Conservation in the Algae From the Point of View of the Type Method, Vol 25. University of California Press.
Timmis, J. N., Ayliffe, M. A., Huang, C. Y., and Martin, W. (2004). Endosymbiotic gene transfer: organelle genomes forge eukaryotic chromosomes. Nat. Rev. Genet. 5, 123–135. doi: 10.1038/nrg1271
Tyystjärvi, E., and Aro, E. M. (1996). The rate constant of photoinhibition, measured in lincomycin-treated leaves, is directly proportional to light intensity. Proc. Nat. Acad. Sci. U.S.A. 93, 2213–2218.
Vieira, S., Calado, R., Coelho, H., and Serôdio, J. (2009). Effects of light exposure on the retention of kleptoplastic photosynthetic activity in the sacoglossan mollusc Elysia viridis. Mar. Biol. 156:1007. doi: 10.1007/s00227-009-1144-y
Wade, R. M., and Sherwood, A. R. (2018). Updating Plakobranchus cf. ianthobapsus (Gastropoda, Sacoglossa) host use: diverse algal-animal interactions revealed by NGS with implications for invasive species management. Mol. Phylogenet. Evol. doi: 10.1016/j.ympev.2018.07.010. [Epub ahead of print].
Wägele, H., Deusch, O., Händeler, K., Martin, R., Schmitt, V., Christa, G., et al. (2011). Transcriptomic evidence that longevity of acquired plastids in the photosynthetic slugs Elysia timida and Plakobranchus ocellatus does not entail lateral transfer of algal nuclear genes. Mol. Biol. Evol. 28, 699–706. doi: 10.1093/molbev/msq239
Wägele, H., and Martin, W. F. (2014). “Endosymbioses in sacoglossan seaslugs: plastid-bearing animals that keep photosynthetic organelles without borrowing genes,” in Endosymbiosis, ed W. Löffelhardt (Vienna: Springer), 291–324.
Yamamoto, S., Hirano, Y. M., Hirano, Y. J., Trowbridge, C. D., Akimoto, A., Sakai, A., et al. (2013). Effects of photosynthesis on the survival and weight retention of two kleptoplastic sacoglossan opisthobranchs. J. Mar. Biol. Assoc. U.K. 93, 209–215. doi: 10.1017/S0025315412000628
Keywords: kleptoplasty, light stress, photosymbiosis, photoinactivation, Sacoglossa
Citation: Christa G, Pütz L, Sickinger C, Melo Clavijo J, Laetz EMJ, Greve C and Serôdio J (2018) Photoprotective Non-photochemical Quenching Does Not Prevent Kleptoplasts From Net Photoinactivation. Front. Ecol. Evol. 6:121. doi: 10.3389/fevo.2018.00121
Received: 05 June 2018; Accepted: 25 July 2018;
Published: 22 August 2018.
Edited by:
Gordon M. Bennett, University of California, Merced, United StatesReviewed by:
Johann Lavaud, Centre National de la Recherche Scientifique (CNRS), FranceGillian H. Gile, Arizona State University, United States
Copyright © 2018 Christa, Pütz, Sickinger, Melo Clavijo, Laetz, Greve and Serôdio. This is an open-access article distributed under the terms of the Creative Commons Attribution License (CC BY). The use, distribution or reproduction in other forums is permitted, provided the original author(s) and the copyright owner(s) are credited and that the original publication in this journal is cited, in accordance with accepted academic practice. No use, distribution or reproduction is permitted which does not comply with these terms.
*Correspondence: Gregor Christa, gregor.christa@ua.pt