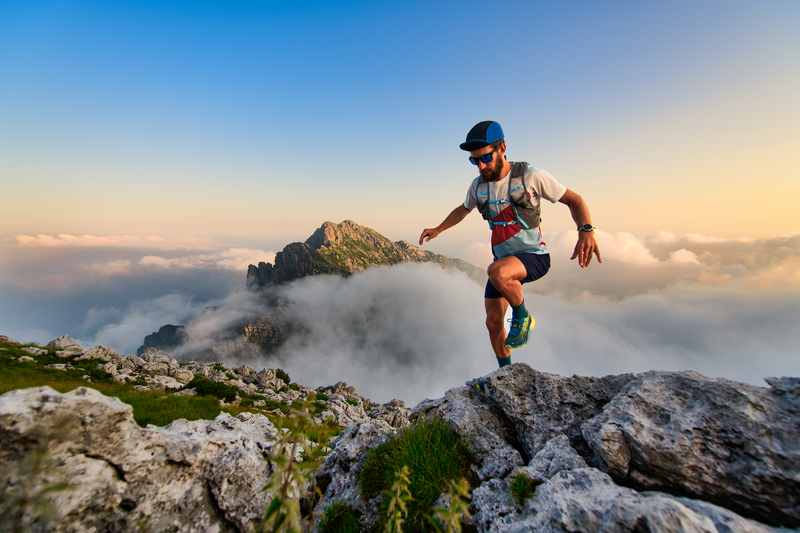
94% of researchers rate our articles as excellent or good
Learn more about the work of our research integrity team to safeguard the quality of each article we publish.
Find out more
ORIGINAL RESEARCH article
Front. Ecol. Evol. , 25 July 2018
Sec. Behavioral and Evolutionary Ecology
Volume 6 - 2018 | https://doi.org/10.3389/fevo.2018.00103
The social nature of termites has allowed them to become an ecologically dominant taxon. However, their nesting and foraging habits (decayed wood and/or soil), combined with frequent social interactions, enhances the risk of pathogen transmission. New dispersing kings and queens are especially vulnerable to pathogens due to the metabolic demands of nest construction, courtship, mating, oogenesis, and parental care, all while mounting an immune response to novel pathogens encountered upon leaving the natal nest. To quantify differential allocation of resources during colony establishment in response to disease exposure, Zootermopsis angusticollis kings and queens were paired after one or both individuals received an injection of saline, heat-killed Serratia marcescens (ecologically relevant, Gram-negative, soil bacterium), a sub-lethal dose of live S. marcescens, or were left untreated. We then quantified several indices of fitness, including the survival of the reproductive pair, onset and likelihood of oviposition, number of eggs produced, and egg quality as a function of parental immunological treatment. Our results uncovered complex and dynamic interactions between these fitness measures and pathogenic stress. Overall, pathogenic stress reduced the survival of kings and queens, the likelihood of oviposition and egg total, but not the onset of oviposition or egg quality, indicating that, in the face of disease, queens “opt” to maintain offspring quality over quantity. These impacts appear to be context-dependent—modulated by colony of origin, sex, mass, and the presence of a mate—rather than absolute. The acquisition of resources prior to colony foundation, combined with the effects of pathogenic exposure, can dramatically limit the success of termites. Based on these empirical data, we have developed a conceptual model of the first 30 days of colony life, involving two successive fitness checkpoints, survival and oviposition, followed by an initial growth phase in which the first egg cohort is produced. In summary, we identified not only the intrinsic and extrinsic factors that influence successful termite colony foundation, but also the maternal and paternal pathogen-induced effects. Such effects alter resource allocation decisions of parents toward their offspring, with cascading consequences on colony fitness.
Termites epitomize ecologically dominant, complex, animal societies (Wilson, 1971). This appears to be the case in spite of their nesting and foraging habitats, which, together with their densely populated colonies and frequent social interactions, potentially facilitate high rates of disease transmission (Rosengaus et al., 2011a; Schmid-Hempel, 2017). Termite species that nest in, and feed on, decayed wood and/or forage in soil are exposed to diverse microbial communities, resulting in high cuticular loads that are potentially pathogenic (Cruse, 1998; Rosengaus et al., 2003; Rosengaus and Reicheld, 2016). These conditions make termites an excellent system in which to test predictions about the role played by pathogens in the evolution of life-history traits in social insects (e.g., Hamilton and Zuk, 1982; Hamilton et al., 1990; Schwenke et al., 2016).
Thus far, most studies on host/pathogen dynamics within termites have utilized mature colonies and, relative to the entire colony's population, a limited number of individuals, which may, therefore, result in unrealistic conclusions about host-pathogen interactions (reviewed by Chouvenc et al., 2011; Rosengaus et al., 2011a). Nonetheless, the early stages of colony life are a critical phase for social insects (Oster and Wilson, 1978). Incipient termite colonies are particularly vulnerable to infection for several reasons. First, upon dispersing from their natal nest, virgin alates (winged dispersers) have a high probability of encountering novel pathogens from their mates and at the new nesting site (Rosengaus and Traniello, 1993a; Rosengaus et al., 2000, 2003). Second, in the majority of termite species, a single king and queen establish new colonies monogamously (Vargo and Hussender, 2011), and, consequently, do so without the benefits of worker-derived social immunity (reveiwed by Rosengaus et al., 2011a; Cremer et al., 2018). Third, their larvae are altricial for the first two instars, relying solely on parental care (Rosengaus and Traniello, 1993b; Crosland et al., 1996), which further drains the parents' limited resources. These young larvae are also more susceptible to fungal pathogens than older nestmates (Rosengaus and Traniello, 2001). This combination of novel pathogens, absence of a worker force (and its concomitant social immunity) and susceptible young larvae, makes the incipient stage of colony growth termites extremely vulnerable to pathogens.
Pathogens create selective pressures that can force hosts into altered resource allocation “decisions”: invest limited resources into somatic maintenance (e.g., immune protection) or reproduction (e.g., terminal investment; Schwenke et al., 2016; Duffield et al., 2017; Figure 1). A commonly observed third alternative involves the concurrent, but diminished, investment in both immune function and reproduction (Morris, 1987; Schwenke et al., 2016; Duffield et al., 2017; Figure 1). These strategies are well documented across taxa, and have been attributed, in part, to resource limitations (Zera and Harshman, 2001; Schwenke et al., 2016; Duffield et al., 2017). Focusing on the impact of pathogenic stress during early stages of termite colony foundation and the resulting trade-offs, therefore, allow us to test these basic premises of parental investment, resource allocation and life-history theories.
Figure 1. Theoretical allocation strategies in response to pathogens, and their predicted outcomes. Arrows represent the direction in which metabolic energy is diverted to: I, immunity; R, reproduction. Hypothesized investment strategies fall within three theoretical allocation “decisions” in response to infection (Schwenke et al., 2016; Dani and Kondandaramaiah, 2017; Duffield et al., 2017). The second row describes predicted fitness outcomes resulting from each investment strategy.
During colony foundation, termite kings and queens invest energy in several competing demands: nest construction, courtship, gamete and offspring production, brood care, and provisioning their first progeny with essential nutrients and gut symbionts (Bignell et al., 2011). Ideally, rapid production of workers emancipates kings and queens from the energetic demands of nest maintenance and parental care, allowing them to focus solely on further offspring production (Matsuura and Kobayashi, 2010). This emancipation appears to be irreversible once the initial resources of the king and queen become depleted (Chouvenc and Su, 2017). We hypothesized, however, that when disease risk is high, termite reproductives will prioritize somatic maintenance at the expense of reproduction. These trade-offs should be measurable as delayed oviposition, reduced production of eggs and/or reduced egg quality (Figure 1). Only once the pathogen is neutralized should reproductives shift away from immunity and revert back to investing in colony growth (Schmid-Hempel, 2003; Contreras-Garduño et al., 2014).
The temporary re-direction of metabolic energy away from reproduction to cope with pathogens should have significant colony-wide fitness consequences. Unfortunately, little information exists on how kings and queens allocate resources to the many competing demands during the initial stage of colony foundation, particularly while facing pathogenic stress. Only a handful of studies have tackled the cascading fitness consequences of pathogen exposure during colony foundation (Calleri et al., 2005, 2006a,b, 2007). While these studies revealed trade-offs between immunity and reproduction, none addressed the multiple factors that can affect, singly and/or in combination, the successful establishment of colonies. To address this gap, we investigated the effects of exposure to a Gram-negative bacterial pathogen on several fitness parameters during early stages of colony foundation in the Pacific dampwood termite, Zootermopsis angusticollis. We examined the following fitness measures: survival of reproductives, onset and likelihood of oviposition, total number of eggs produced in the first 30 days post-pairing, and egg volume (a proxy for egg quality). By exposing both kings and/or queens to an ecologically relevant bacterium early during colony establishment, we identified various intrinsic and extrinsic factors that independently and/or in combination influence colony growth and development. Ultimately, this information yields a more comprehensive understanding of fitness costs, benefits and trade-offs against the backdrop of resource allocation, parental investment and life history theories in a non-model species with unique life-history traits among the social insects.
Mature colonies of Z. angusticollis (n = 17) were collected from the Redwood East Bay Regional Park in Oakland, CA between 2013 and 2014. These colonies were used as a source of alates within the first two years of collection to minimize any effects of prolonged care under laboratory conditions (e.g., Cornelius and Osbrink, 2009; Hoffmann and Ross, 2018). Upon collection, colonies were maintained in covered tubs of up to 53 L (40 × 26 × 18 cm; Rosengaus et al., 1998, 2011b) and kept in the dark at 25°C and 60% relative humidity. White birch wood (Betulla) collected from eastern Massachusetts was used to supplement the original nesting and feeding material.
A total of 623 incipient colonies were established following the protocols described in Rosengaus and Traniello (1993a). Briefly, alates were removed from their respective parental colonies between February and October of 2014, sexed, de-winged, weighed, and housed individually in Petri dishes (60 × 15 mm) lined with a moistened Whatman #1 filter paper and 1–3 small pieces of birch wood (totaling ~2.5 mg) until the time of treatment (see below). Only heavily sclerotized alates with intact, but easily removable, wings were collected to ensure that virgin individuals who were physiologically and behaviorally motivated to mate were used in our experiments (Rosengaus et al., 2000; Hartke and Rosengaus, 2013). We assumed that these individuals were virgin as Z. angusticollis alates only complete their reproductive development when separated from the colony (Brent and Traniello, 2001, and references therein). Moreover, de-winged alates of this species mate only after secluding themselves in the copularium (Nutting, 1969). Kings and queens were experimentally treated (see below) and subsequently paired with a mate. Incipient colonies were stacked inside clear plastic boxes lined with damp paper towels to maintain a high humidity (~90% relative humidity). Water and birchwood chips were added to these incipient colonies as needed. Given that this experiment encompasses the developmental transition from winged, dispersing alates to actively reproducing pairs, we henceforth refer to these primary reproductives as kings and queens.
Serratia marcescens is a common, Gram-negative soil bacterium that naturally infects Z. angusticollis as well as other termite species (De Bach and Mcomie, 1939; Steinhous, 1959; Osbrink et al., 2001; Adams and Raj, 2005). S. marcescens has been isolated from the guts of termites and can co-exist within the termite. However, this bacterium can turn pathogenic if the insect is weakened, stressed, co-infected with other microorganisms (Chouvenc et al., 2011 and references therein), or if S. marcescens penetrates the hemocoel via translocation from the gut's lumen or via cuticular wounding (Mirabito and Rosengaus, 2016). We used injection of S. marcescens into the hemocoel to emulate infection via cuticular injury with the added benefit of known and consistent pathogenic loads across all similarly treated animals.
The strain used in our experiments was originally recovered from a naturally infected Z. angusticollis colony and was cultured as described by Rosengaus et al. (1999). It was grown at 37°C in sterile tryptic soy broth, pelleted, and re-suspended in sterile Burnes-Tracy saline (BTS). The BTS contained green food coloring (5 mL per L of BTS; McCormick) which allowed for visual confirmation of successful injection. The food coloring was metabolized within 24 h with no observable negative effects (Rosengaus et al., 1999). Live bacterial cultures (Live-Sm) suspended in green BTS contained 2 × 105 colony forming units (CFU)/mL. To decouple the effects of an active infection from immune-elicitation, a suspension of heat-killed bacteria (HK-Sm) was also prepared by boiling a live 107 CFU/mL stock suspension for 10 min. This resulted in a immunogenic solution containing Serratia-specific pathogen-associated molecular patterns (PAMPs; Siva-Jothy et al., 2005). The boiled suspension was plated onto tryptic soy agar to verify it was inert. None of the boiled suspensions used in this experiment yielded CFUs.
To test the separate and combined paternal and maternal effects from exposure to S. marcescens, we assigned colonies to four different treatments (Figure 2). Either the king, queen or both were injected with one of the following three suspensions: saline (1 μL sterile green BTS; i.e., control), heat-killed S. marcescens (1 μL of 107 CFU/mL in green BTS; HK-Sm), or live bacteria (1 μL of a 2 × 105 CFU/mL suspension of live S. marcescens suspended in green BTS, corresponding to a final sublethal dose of 200 CFUs per injection; Live-Sm). Because aseptic cuticular wounding in insects is known to elicit immune responses (Han et al., 1999; Wigby et al., 2008; Krautz et al., 2014) and potentially elicit trade-offs, an additional set of colonies were left untreated (naïve; Figure 2).
Figure 2. Experimental design. The numbers in each box represent the number of incipient colonies initially set up for each treatment: kings (K) and/or queens (Q) were treated with one of the treatments (saline, HK-Sm, Live-Sm). QK indicates both reproductives were treated while Q-- and --K designate the member of the pair who received the treatment while its mate remained naïve (--). This scheme resulted in 9 treatment combinations. A tenth treatment had both kings and queens untreated (naïve) to provide a baseline of normal colony fitness parameters during the first 30 days.
All injections were performed using a picospritzer III (Parker Hannifin) and pulled borosilicate capillary tubes (~2 μm in diameter). Isolated kings and queens were cold immobilized inside Petri dishes for a maximum of 20 min. Only individuals receiving an injection (saline controls, HK-Sm, and Live-Sm) were cold immobilized. By including completely untreated individuals (naïve), we could determine the full extent of treatment effects compared to minimally handled individuals. The saline treatment allowed us to separate the effects of immune-elicitation from the unavoidable consequences of handling and wounding. Both the capillary tip and the termite's abdomen were swabbed with 70% ethanol. The capillary was then inserted between the third and fifth ventral intersegmental membranes and the assigned treatment suspension was injected directly into the hemocoel. Following injection, individuals were alcohol-swabbed again and given 30–40 min to recover before pairing with their randomly assigned mate. Survival and all other fitness-related measurements were quantified as a function of the number of days elapsed post-treatment (i.e., post-pairing).
Incipient colonies were examined every other day for 30 days post-treatment. During each census, we recorded king and queen survival, time to first oviposition, and total number of eggs observed. Dead individuals were removed immediately and were never replaced. Because mated queens can continue to oviposit fertilized eggs despite the death of the king (personal observation), colonies in which kings died but queens were alive were censused for the entire 30-day period.
In insects, egg size is a reliable indicator of egg quality (Fox and Czesak, 2000). Egg size is positively correlated with various fitness-related traits including hatchling size and resilience to various environmental stressors (reviewed by Fox and Czesak, 2000; Parker and Johnston, 2006; Dani and Kondandaramaiah, 2017). Egg size is also negatively correlated with developmental time (reviewed by Fox and Czesak, 2000; Parker and Johnston, 2006). When present, newly laid eggs were carefully removed with ethanol-sterilized feather-weight forceps (BioQuip) and imaged (Qspot camera) with a dissecting microscope at 40X magnification. Length and width were measured using the SPOT imaging software. Since most termite eggs approach the shape of a cylinder, volume was estimated using the formula:
where W = width and L = length (Brent and Traniello, 2002). Although this formula may slightly overestimate actual egg volume, this bias was consistent across treatments. A total of 1,138 eggs across all treatments were imaged (Table 1).
All statistical analyses were carried out using IBM SPSS, version 24. Because genetic relatedness between kings and queens (i.e., nestmate or non-nestmate) is inherently encompassed within the variable “colony of origin” (COO), only the latter was used in the analyses. Time of alate collection was not considered in any of the statistical models because alate emergence appears to be characteristic of each source colony and is thus, an inherent variable encompassed within COO (personal observations; Supplemental Table 6). Given that saline-injected reproductives tended to have a lower survival than naïve reproductives (Figure 3), and the fact that cuticular injury is known to elicit immunity (Han et al., 1999; Wigby et al., 2008; Krautz et al., 2014), both the naïve and saline treatments were included in all statistical analyses, with the latter always considered the control. Due to limitations imposed by the number of available reproductives, our experimental design was unbalanced (Supplemental Figure 1). Therefore, we were unable to generate Cox proportional regression models that included both the main effects and the interactions of king and queen treatment. Due to the flexibility and robustness of mixed effect models, this unbalanced design did not influence analyses of egg number and egg volume. Sample sizes for all analyses are given in Figure 2 and Table 1. Individuals missing any of the measured variables were excluded from the models.
Figure 3. Survival curves generated from the Cox proportional regression analysis. Dotted gray arrows denote the time interval in which there was the steepest decline in survival. This time preceded the period in which reproductives were likely forced to make physiological “decisions” between immunity and reproduction. The mean (inverted triangle) and interquartile range of the onset of oviposition (bold black line) for surviving colonies are overlaid on the X-axis. Letters to the right of each survival curve represent significant differences following a Bonferonni correction.
To estimate the relative risk of death as a function of immunological treatment while controlling for the effects of confounding variables, a Cox proportional regression model was generated in which each individual king and queen was considered as a single datum (overall n = 1,205; naïve = 462, saline = 197, HK-Sm = 187, and Live-Sm = 359). Since the survival of kings and queens during the critical stages of colony establishment depend on both the focal individual's traits (intrinsic variables) and traits of their mate (extrinsic variables), the following variables were included: treatment, sex, COO and mass of the focal individual; and the treatment, COO and mass of the mate. Post-hoc pairwise comparisons between the four immunological treatments of the focal individual were conducted using Bonferroni correction (setting a more conservative p-value of 0.008). Visual assessment of the plotted hazard function and the log-minus-log plots confirmed that the assumption of proportional hazards was met for all Cox proportional regression models.
To test the impact of parental immunological treatment on the onset and likelihood of oviposition, two Cox proportional regression models were generated using each mated pair (i.e., incipient colony) as the unit of measurement. The first model, testing whether parental treatment influenced onset of oviposition, only included colonies in which the female had both survived and oviposited (n = 263; Table 1). The second model included all colonies with surviving females, regardless of oviposition status (n = 338; Table 1). This model allowed us to examine overall differences in the likelihood of oviposition as a function of treatment. King and queen treatment, COO, mass, as well as the interaction queen mass × queen treatment were included in the models. Post-hoc pairwise comparisons were conducted between the four immunological treatments (for both kings and queens, separately) using a Bonferroni correction (p ≤ 0.008).
Two mixed effects models were generated to determine if pathogenic exposure impacted (1) total number of eggs laid during the first 30-day census period, and (2) egg volume; using log-transformed egg totals and the average egg volume for each colony, respectively. By averaging egg volume per incipient colony, we accounted for possible repeated measure effects (i.e., measuring numerous eggs from the same queens) and indirectly for possible effects of laying order (see Matsuura and Kobayashi, 2010). Only colonies in which the queen both lived and produced at least one egg were included in these analyses (n = 263 colonies; Table 1). Both models included king treatment, queen treatment, and king survival as fixed factors; king mass, queen mass, and the days elapsed to first oviposition as continuous covariates; and queen and king COO as categorical random factors. All main effects and the following two-way interactions were included in each of the models: queen treatment × queen mass, queen treatment × king death, queen treatment × king treatment, queen treatment × king mass, king treatment × queen mass, king treatment × king mass. Where applicable, post-hoc comparisons were conducted using the Dunn-Sidak method. The residuals generated by the linear mixed effects models were normally distributed. Two additional fixed effects were included in the analysis of average egg volume: egg total and egg total × queen treatment.
Results indicated that both treatment of the focal individual and mate treatment were highly significant and independent predictors of survival (Wald (W) = 35.2, df = 3, p < 0.001, W = 13.3, df = 1, p = 0.004; respectively; Figure 3). Individuals who received the live S. marcescens treatment were twice as likely to die as naïve individuals (W = 32.2, df = 1, p < 0.001; Figure 3) and 1.5 times more likely to die than saline-injected individuals (W = 5.8, df = 1, p = 0.02; Figure 3). HK-Sm were 1.7 times more likely to die than their naïve counterparts (W = 10.8, df = 1, p = 0.001; Figure 3), but were not significantly different from the either the saline (W = 1.6, df = 1, p = 0.2; Figure 3) or Live-Sm treated individuals (W = 0.6, df = 1, p = 0.4; Figure 3). Saline-injected controls were 1.4 times as likely to die as naïve individuals, although this was not significantly different after Bonferroni correction (W = 4, df = 1, p = 0.04, Figure 3). The greatest decline in survival occurred between days 1–10 across all four treatments.
The death of a mate was the strongest predictor of the focal reproductive's survival (W = 231.7, df = 1, p < 0.001). Individuals whose mates died were 4.8 times more likely to die than those with surviving mates. Sex was also a significant and independent predictor of survival (W = 12.5, df = 1, p < 0.001), with males being 1.5 times more likely to die than females. Although the focal individual's own mass was not a significant predictor of its survival (W = 0.5, df = 1, p = 0.5), individuals who had heavier mates had a tendency to survive longer (mate mass: W = 3.7, df = 1, p = 0.06). Neither COO nor the mate's COO were significant contributing factors to the model (W = 23.9, df = 17, p = 0.1; W = 18.6, df = 16, p = 0.3, respectively).
The interquartile range of the onset of oviposition across all treatments (n = 263 colonies) was 10–21 days (mean = 16.8 days, median = 18 days post-treatment). Because this interval followed the time when the most pronounced mortality occurred for the HK-Sm and Live-Sm treated individuals (Figures 3, 4), we are confident our experimental design fostered trade-offs between immunity and reproduction.
Figure 4. Top (A,B) Onset of oviposition curves derived from model 1 as a function of king treatment (A) and queen treatment (B). The legend in the top left corresponds to treatment for all four graphs. The black bars overlaid on the X-axis show the period during which the steepest decline in reproductive survival occurred (Figure 3). This period coincided with the time at which parents are allocating resources to the various demands. For both parental treatments, there was no significant difference in the onset of oviposition. Onset of oviposition was primarily influenced by intrinsic traits (mass, COO, Table 1). Bottom (C,D) depict the likelihood of oviposition as a function of king treatment (C) and queen treatment (D). Colonies headed by saline-injected kings were significantly more likely to oviposit than Live-Sm kings (C). HK-Sm queens were significantly more likely to oviposit than saline and Live-Sm queens (D). In D, the Live-Sm and saline curves are overlapping.
The onset of oviposition was primarily driven by the king and queen's intrinsic traits (COO, mass; Supplemental Table 1). King treatment was a significant overall predictor of the onset of oviposition (W = 7.7, df = 3, p = 0.05; Supplemental Table 1), mostly driven by the saline treatment. This statistical significance is likely not biologically meaningful as the onset of oviposition curves track each other closely (Figure 4A). Queen treatment and the interaction queen treatment × queen mass were not significant predictors of the onset of oviposition (W = 3.1, df = 3, p = 0.4; W = 3.4, df = 3, p = 0.3, respectively; Figure 4B). We were unable to detect combined effects between queen and king treatments on onset of oviposition because of the unbalanced experimental design (Supplemental Figure 1).
Our second model indicated that the queen's intrinsic traits were significant predictors of whether she laid at least one egg by day 30 post-treatment (COO: W = 62.6, df = 13, p < 0.001; mass: W = 5.2, df = 1, p = 0.02; Supplemental Table 1). In addition to the queen's traits, the immunological status of both the king and queen were significant and independent predictors of the likelihood of oviposition (W = 9, df = 3, p = 0.03, Figure 4C; W = 12, df = 3, p = 0.007; Figure 4D; Supplemental Table 1), although their effects appeared to be sex-specific. Queens paired with Live-Sm kings were the least likely to oviposit (Figure 4C, Supplemental Table 2). Queens mated to saline kings and naïve kings were 2 and 1.4 times, respectively, as likely to oviposit compared to those mated to Live-Sm kings (W = 7.9, df = 1, p = 0.005; Figure 4C, Supplemental Table 2; W = 3.9, df = 1, p = 0.05, respectively). HK-Sm queens were twice as likely to oviposit as saline-injected or Live-Sm queens (W = 7.3, df = 1, p = 0.007; W = 8.7, df = 1 p = 0.003, respectively, Figure 4D, Supplemental Table 2). No other pairwise comparisons between king or queen treatments were significantly different (Figures 4C,D; Supplemental Table 2).
The analysis of log-transformed egg totals found no significant main effect of king and queen treatment on egg production (Supplemental Table 3). The only significant main effect was the onset of oviposition (F = 28.8, df = 1, 222, p < 0.001), with a 4.2% decrease in the number of eggs produced for each additional day that oviposition was delayed (Supplemental Table 3). There was, however, a significant interaction between queen treatment and queen mass (F = 3.3, df = 1, 223, p = 0.02; Supplemental Table 3). This effect was mainly driven by the Live-Sm-injected queens, which showed a 4.2% increase in the number of eggs laid per milligram of mass, compared to saline-injected queens whose egg production was not influenced by their mass (t = 2.6, df = 218.4, p = 0.01). Similarly, queen mass did not impact the number of eggs laid for the HK-Sm and naïve queens (t = 0.5, df = 222.5, p = 0.6; t = 1.7, df = 220.2, p = 0.09, respectively). Even though the interaction of queen treatment by king treatment approached significance (F = 2.3, df = 3, 215.9, p = 0.08), none of the pairwise comparisons were significant. Thus, when both parents are pathogenically stressed, there appears to be no combined effects on the number of eggs produced by day 30. There was a significant interaction between queen treatment and king death (F = 2.9, df = 3, 215, p = 0.04; Supplemental Table 3). King death negatively influenced the number of eggs produced, but only when kings were paired with naïve queens. Naïve queens whose kings lived produced 49% more eggs than naïve queens whose mates died (t = 2.0, df = 208.2, p = 0.05).
Across all parental treatments, egg volume ranged from 0.14 mm3 to 0.55 mm3 with a mean of 0.28 mm3 ± 0.002 SE. The mixed effects analysis revealed significant variation of egg volume depending on the queen's COO (W = 2.15, df = 1, p = 0.03). No other factor, including king (F = 0.9, df = 3, 179.3, p = 0.9) and queen treatment (F = 1.3, df = 3, 178.7, p = 0.3), nor their combined effects (F = 0.1, df = 3, 179.7, p = 0.08), influenced average egg volume (Supplemental Tables 4, 5).
The main objective of our study was to test the putative impacts of bacterial infection on several fitness parameters of Z. angusticollis during early colony life. There is widespread empirical evidence that immunity and reproduction are “mutually constrained” (Schwenke et al., 2016). The presence of pathogens often induce immune responses, threatens the survival of the infected individual, and depletes stored metabolic resources (Schmid-Hempel, 1998). Consequently, pathogenic exposure prevents individuals from reaching their theoretical maximum reproductive output. Alternatively, individuals can potentially optimize their reproductive output by changing their reproductive tactics in response to infection (Figure 1). For example, short-lived and/or oviparous animals with no parental care (a combination of traits recently coined under the concept of “pace-of-life syndromes”; Dammhahn et al., 2018), may engage in terminal investment (i.e., egg dumping; Sullivan et al., 2016; Duffield et al., 2017). In contrast, long-lived and/or iteroparous organisms that exhibit parental care may delay reproduction until conditions for them and their offspring are favorable, thus increasing future reproductive opportunities (Dani and Kondandaramaiah, 2017; Duffield et al., 2017; Figure 1). Given that termites fall into the latter category, we had anticipated most reproductive milestones and fitness measures to be negatively affected by pathogenic stress, particularly because parental resources are limited until a worker force is established (Chouvenc and Su, 2017). In our attempt to control for other variables that could potentially influence (or interact with) parental immunological treatment, our experimental design and analyses revealed a more complex picture. The dynamics between infection and reproduction are context-dependent and appear to be modulated by intrinsic and extrinsic factors.
We propose a new model for early colony development that combines these data with previous observations and identified reproductive milestones of incipient colonies that are either plastic or refractive to the effects of pathogens (Figure 5). Newly established termite colonies appeared to transition through two sequential all-or-nothing fitness “checkpoints” followed by an initial growth phase in which the first brood (i.e., the first cohort of eggs) is produced. This latter stage is not equivalent to the ergonomic growth phase of a social insect colony (Oster and Wilson, 1978; Figure 5).
Figure 5. Ontogeny of colony foundation under pathogenic stress. T = treatment was given on the same day as colony establishment. Each checkpoint depicts the overall percent of colonies that either did (YES) or did not (NO) successfully pass through that checkpoint: ‡, percent at day 10; +, percent at day 20; *, percent at day 30. Checkpoint 1, characterized by whether kings and queens survive pathogenic exposure, is the first hurdle in the successful establishment of a colony. The steepest decline in survival was in the first 10 days post-establishment when 25% of all reproductives died. By day 30, 41% of all reproductives were dead. Checkpoint 2 is characterized by whether a queen engages in egg-laying. Surviving queens oviposited between days 10 and 20 post-establishment. If a queen successfully surpassed checkpoints 1 and 2, then fitness-related costs due to pathogenic stress were evident in a context dependent manner during the initial phase of colony growth: light queens injected with Live-Sm produced fewer eggs by day 30 relative to heavy queens who were similarly treated. = indicates the negative contributions of pathogenic stress on fitness-related parameters.
Our results indicate that kings and queens experience altered and context-dependent resource investments during the first two checkpoints, with measurable consequences that become evident at the initial growth phase (Figure 5). The following discussion, based on the present empirical results, is organized according to the progression across these significant phases (Figure 5).
The survival data points to three main findings. First, our data established the timeframe for the first all-or-nothing fitness check-point. The steepest decline in survival occurred in the first 10 days, after which mortality leveled off (Figure 3). The mortality of the untreated, naïve, reproductives was high (26.5% by day 30, Figure 3), but consistent with previous studies of termite colony foundation (e.g., in Z. angusticollis, Rosengaus and Traniello, 1993b; Calleri et al., 2006a; in Coptotermes formosanus, Fei and Henderson, 2003; in Nasutitermes corniger, Hartke and Rosengaus, 2013). Given that the steepest decline in survival occurred early on, prior to oviposition, many kings and queens die before having the chance to reproduce, thus resulting in a complete loss of fitness. Second, the survival data strongly support the notion that our immunological treatments resulted in pathogenic stress just as intended. The Live-Sm-injected reproductives experienced the steepest mortality relative to all other treatments. Their additional mortality relative to saline and HK-Sm treatments is attributed to the negative impact of the active pathogen. Such decline in survivorship during the first 10 days occurred just prior to the onset of oviposition (Figures 3, 4), when queens had high energetic demands for mating, oogenesis and excavating/sanitizing their copularium. All of these costly activities likely fostered trade-offs between reproductive investment and immune activation (Schwenke et al., 2016), which appear to have resulted in cascading fitness-related consequences. Third, immunological treatment also had indirect effects on the mates of treated individuals. Kings or queens mated to a Live-Sm-treated individual were more likely to die than those mated to naïve or control partners. This may be the result of either disease transmission between the reproductives (e.g., Rosengaus et al., 2000) or the absence of a partner leading to diminished or a complete lack of social interactions required to reach reproductive status (e.g., allogrooming and proctodeal exchanges; Shellman-Reeve, 1990; Brent et al., 2007). Moreover, infected individuals often turn “lethargic” and may not fully contribute to the multiple tasks associated with colony establishment, forcing their mates to assume a higher energetic burden and thus, increase their mortality.
Results from both the onset and likelihood of oviposition helped delineate the parameters of checkpoint 2. This stage was characterized by whether or not queens laid eggs rather than when they laid those eggs: parental treatment had no effect on the onset of oviposition and only 68% of surviving queens oviposited by day 30 (Figures 4A,C). Of these, regardless of parental treatment, two-thirds began oviposition between days 10 and 20, defining the timeframe of this checkpoint (Figure 5). The possibility exists that the remaining 32% of queens who survived but had yet to oviposit by day 30 may have done so if the census had been continued beyond this time. However, preliminary data from an ongoing, more long-term study indicate that most queens in this situation failed to ever produce eggs (personal observations).
That the onset of oviposition was not delayed by parental treatment was unexpected given that Z. angusticollis queens who received a nylon implant (eliciting a cellular immune response) had a significantly delayed onset of oviposition (Calleri et al., 2007). However, the microinjections used here were likely less invasive than the introduction of nylon implants, which remained inside of the hemocoel for 4 days. The fixed reproductive schedule under bacterial exposure may represent a compromise between generating an immune response and producing a functional worker cohort as quickly as possible (Matsuura and Kobayashi, 2010). An un-altered reproductive schedule may allow Z. angusticollis reproductives to simultaneously allot limited resources toward both immunity and the production of a worker force, albeit a diminished one (discussed below).
Interestingly, the effects of pathogenic exposure on the likelihood of oviposition were both treatment- and sex-specific. These divergent outcomes likely arose from pathogen-induced sex-specific dynamics between treatment and available metabolic resources. Nitrogen is a severely limited nutrient in cellulose-consuming termites (Nalepa, 1994), and previous work showed that nitrogen supplementation increases the likelihood that a founding pair reproduces (Shellman-Reeve, 1990). Zootermopsis kings are known to provision their queens with nitrogenous secretions during colony foundation (Shellman-Reeve, 1990), and excess nitrogen is known to be stored in the fat body (reviewed by Slaytor and Chappell, 1994). Taken together—along with the observations that mass serves as a reliable proxy for overall stored resources in insects (e.g., Berger et al., 2012; McConnel and Judge, 2018), and that queen mass was a predictor of both the likelihood and onset of oviposition in our experiment (Supplementary Table 2)—these facts point to the significance of having adequate resources for the successful establishment of a termite colony. We found that a queen's likelihood of oviposition was significantly lower when her mate received an injection of Live-Sm. Live-Sm injections may have compromised the king's ability to supplement the queen's resources. The sub-lethal infection may have also altered the rate at which kings interact with their queen, an important trigger for the queen's sexual maturation (Brent et al., 2007). Additionally, the queen may be able to sense the male is sick, although this is unlikely as Rosengaus et al. (2011b) found no evidence of mate choice by Z. angusticollis queens when presented with a choice of Metarhizium anisopliae infected and uninfected kings. Any or all of these scenarios would result in the queen having less or no access to the essential resources and/or social interactions that promote oogenesis.
The importance of possessing adequate resources is further highlighted by our observation that HK-Sm injected queens were more likely to produce eggs than any other group. We hypothesize that the boiled bacteria emulated nutrient supplementation (Figure 4D). Heat-killed bacterial injections, which consist of a crude suspension of boiled bacteria, could have provided metabolites such as amino acids and/or glucose in addition to PAMPs. To the best of our knowledge, no study has tested whether boiled suspensions of bacteria have nutritional value, and we are currently testing this possibility. That the likelihood of oviposition for Live-Sm queens was nearly identical to that of saline-injected queens (60.9 and 60.1%, respectively) strongly suggests that oviposition is not directly impacted by pathogenic stress per se, but rather by its consequences on the availability of resources. It is possible that only robust (i.e., heavy) queens survived the Live-Sm treatment, thereby masking the direct effects of pathogens on oviposition. This is unlikely, however, because if we inadvertently selected for robust queens, mass should have been a significant predictor of survival, and this was not the case. Finally, there is no reason to assume that heat-killed bacteria and live bacteria produce equivalent physiological outcomes. Heat-killed E. coli was found to initiate a negative feedback loop that resulted in a truncated immune response in Drosophila, compared to live E. coli (Neyen et al., 2016). If these findings are applicable to termites, the burden of immunological responses would have been greater for Live-Sm queens than for HK-Sm queens, which would explain why the latter has a higher likelihood of oviposition. In short, queens likely need to meet some threshold of stored resources in order to produce eggs.
Ideally, reproductive pairs should strive to quickly produce as many offspring as possible to secure a worker force that will emancipate kings and queens from the energy requirements of nest maintenance and brood care (Matsuura and Kobayashi, 2010). However, the presence of pathogens likely prevents reproductives from achieving their maximum reproductive potential. We have shown that the onset of oviposition in Z. angusticollis is refractive to the effects of pathogens (Figures 4A,B). Also, terminal investment in this species is highly unlikely due to the need for extensive parental care. Based on these observations, we envision that infected parents “opt” to simultaneously divide their resources toward both reproduction and immunity (Figure 1). Consequently, at this stage, they have two possible tactics: either decrease investment in each individual egg, or decrease the number of eggs they produce (Figure 1; Schwenke et al., 2016). Previous research found a positive correlation between egg size and resilience to environmental stressors (Fox and Czesak, 2000). Moreover, there is robust evidence across taxa for an inverse relationship between egg size and offspring number, even when parents are not resource-limited (Dani and Kondandaramaiah, 2017). In animals and plants, egg number is typically more variable than egg size (Dani and Kondandaramaiah, 2017). Our data are in accordance with these three generalizations: in the incipient stages of colony foundation, termite queens “opted” to maintain egg quality at the expense of egg quantity when they were both pathogenically stressed and resource-limited. This study did not take into account egg laying order. However, if Z. angusticollis queens lay increasingly smaller eggs, as reported in Reticulitermes speratus (Matsuura and Kobayashi, 2010), then the average egg volume should have been lower (accounting for the decrease in egg volume for each subsequent egg) than that of queens who laid fewer eggs. Furthermore, any effects of pathogens on the pattern of egg volume over time would have yielded consistent differences in the average egg volume of those queens who were infected. Neither of these outcomes were observed and, therefore, it is reasonable to conclude that exposure to S. marcescens did not influence the average egg volume or alter the typical pattern of egg volume as a function of laying order.
The number of eggs produced was significantly influenced by queen mass, but only when queens were injected with live S. marcescens. For example, queens laid 4.2% more eggs per every additional mg of mass. The queens in this study ranged between 20 and 60 mg. Thus, a 20 mg Live-Sm queen who lays two eggs would have laid 10.4 eggs if she was 60 mg instead. The facts that heavier Live-Sm queens laid a similar number of eggs as the naïve, control and HK-Sm queens and that light Live-Sm queens were less likely to oviposit, point to the importance of having adequate metabolic resources to cope with both the demands of immunity and reproduction (Figures 1, 5). Similar resource-based, context-dependent trade-offs between immunity and reproduction have been reported in other taxa (e.g., crickets, Shoemaker et al., 2006; lizards, French et al., 2007; rodents, Xu et al., 2012).
In conclusion, our empirical study identified several intrinsic and extrinsic factors (including pathogenic stress) that influence resource allocation decisions by termite reproductives and, ultimately, the successful establishment of a colony. In the face of disease, new queens are more likely to survive and avoid reproductive costs of infection when they are heavier and mated to uninfected, heavy kings. Costs of infection appear to be mediated via the queen's direct and indirect access to metabolic resources, resulting in a context-dependent reduction of offspring number (but not quality) during colony foundation. Given the apparent importance of adequate resources for the successful foundation of a colony, termite kings and queens may have been (and continue to be) selected to (a) be larger (i.e., heavier), (b) choose robust mates following swarming and (c) have long-lived kings who both provision the queen and participate in biparental care.
EC collected mature colonies from the field, designed and conducted the experiment, generated statistical models and wrote the manuscript. II generated statistical models and helped write the manuscript. RR collected mature colonies from the field, designed the experiment, wrote the manuscript and funded this research.
This work was supported by the 2013 Nutting Grant provided by the North American Section of the International Union for the Study of Social Insects.
The authors declare that the research was conducted in the absence of any commercial or financial relationships that could be construed as a potential conflict of interest.
We thank the administrators of the Redwood East Bay Regional Parks for their permission to collect termite colonies, the North American Section of the International Union for the Study of Social Insects for funding through the Nutting Grant, Dr. T. Gouhier for guidance with statistics, and Drs. C. S. Brent, W. Smith, and B. Roitberg for comments and suggestions in preliminary versions of this manuscript. We appreciate the time and comments of the reviewers which increased the clarity and quality of this manuscript. We also give thanks to the many undergraduates, especially B. Lejeune, E. Parikh, and A. Adesina, for assistance with the census.
The Supplementary Material for this article can be found online at: https://www.frontiersin.org/articles/10.3389/fevo.2018.00103/full#supplementary-material
Adams, L., and Raj, B. (2005). Isolation and characterization of enteric bacteria from the hindgut of the Formosan termite. Bioresour. Technol. 96, 1592–1598. doi: 10.1016/j.biortech.2004.12.020
Berger, D., Olofsson, M., Friberg, M., Karlsson, B., Wiklund, C., and Gotthard, K. (2012). Intraspecific variation in body size and the rate of reproduction in female insects–adaptive allometry or biophysical constraint? J. Anim. Ecol. 81, 1244–1258. doi: 10.1111/j.1365-2656.2012.02010.x
Bignell, D. E., Roisin, Y., and Lo, N. (2011). Biology of Termites: a Modern Synthesis. Dordrecht: Springer.
Brent, C. S., and Traniello, J. F. A. (2001). Influence of sex-specific stimuli on ovarian maturation in primary and secondary reproductives of the dampwood termite Zootermopsis angusticollis. Physiol. Entomol. 26, 239–247. doi: 10.1046/j.1307-69622.2001.00243.x
Brent, C. S., and Traniello, J. F. A. (2002). Effects of enhanced dietary nitrogen on reproductive maturation of the Termite Zootermopsi angusticollis (Isoptera: Termopsidae). Popul. Ecol. 31, 313–318. doi: 10.1603/0046-225X-31.2.313
Brent, C. S., Schal, C., and Vargo, E. L. (2007). Endocrine effects of social stimuli on maturing queens of the dampwood termite Zootermopsis angusticollis. Physiol. Entomol. 32, 26–33. doi: 10.1111/j.1365-3032.2006.00536.x
Calleri, D. V. II, McGrail Reid, E., Rosengaus, R. B., Vargo, E. L., and Traniello, J. F. A. (2006a). Inbreeding and disease resistance in a social insect: effects of heterozygosity on immunocompetence in the termite Zootermopsis angusticollis. Proc. R. Soc. B 273, 2633–2640. doi: 10.1098/rspb.2006.3622
Calleri, D. V. II. Rosengaus, R. B., and Traniello, J. F. (2005). Disease and colony foundation in the dampwood termite Zootermopsis angusticollis: the survival advantage of nestmate pairs. Naturwissenschaften 92, 300–304. doi: 10.1007/s00114-005-0630-4
Calleri, D. V. II., Rosengaus, R. B., and Traniello, J. F. (2007). Immunity and reproduction during colony foundation in the dampwood termite, Zootermopsis angusticollis. Physiol. Entomol. 32, 136–142. doi: 10.1111/j.1365-3032.2007.00559.x
Calleri, D. V. II. Rosengaus, R. B., and Traniello, J. F. A. (2006b). Disease and colony establishment in the dampwood termite Zootermopsis angusticollis: survival and fitness consequences of infection in primary reproductives. Insectes Soc. 53, 204–211. doi: 10.1007/s00040-005-0859-0
Chouvenc, T., and Su, N.-Y. (2017). Irreversible transfer of brood care duties and insights into the burden of caregiving in incipient subterranean termite colonies. Ecol. Entomol. 42, 777–784. doi: 10.1111/een.12443
Chouvenc, T., Su, N-Y., and Grace, J. K. (2011). Fifty years of attempted biological control of termites – Analysis of a failure. Biol. Control 59, 69–82. doi: 10.1016/j.biocontrol.2011.06.015
Contreras-Garduño, J., Rodríguez, M. C., Rodríguez, M. H., Alvarado-Delgado, A., and Lanz-Mendoza, H. (2014). Cost of immune priming within generations: trade-offs between infection and reproduction. Microbes Infect. 16, 261–267. doi: 10.1016/j.micinf.2013.11.010
Cornelius, M. L., and Osbrink, W. L. (2009). Bioassay design and length of time in the laboratory affect intercolonial interactions of the Formosan subterranean termite (Isoptera, Rhinotermitidae). Insectes Soc. 56, 203–211. doi: 10.1007/s00040-009-0014-4
Cremer, S., Pull, C. D., and Fürst, M.A (2018). Social immunity: emergence and evolution of colony-level disease protection. Annu. Rev. Entomol. 63, 105–123. doi: 10.1146/annure-ento-020117-043110
Crosland, M. W. J., Lok, C. M., Wong, T. C., Shakarad, M., and Traniello, J. F. A. (1996). “Temporal polyethism in the lower termite, Reticulitermes fukienensis (Isoptera: Rhinotermitidae),” in Proceedings of the International Congress of Entomology, 408.
Cruse, A. (1998). Termite Defenses Against Microbial Pathogens. Ph.D. dissertion, Macquarie University. Sydney, NSW.
Dammhahn, M., Dingemanse, N. J., Niemel,ä, P. T., and Réale, D. (2018). Pace-of-life syndromes: a framework for the adaptive integration of behavior, physiology and life history. Behav. Ecol. Sociobiol. 72, 1–8. doi: 10.1007/s00265-018-2473-y
De Bach, P. H., and Mcomie, W. A. (1939). New disease of termites caused by bacteria. Ann. Entomol. Soc. Am. 32, 137–146.
Duffield, K. R., Bowers, E. K., Sakaluk, S. K., and Sadd, B. M. (2017). A dynamic threshold model for terminal investment. Behav. Ecol. Sociobiol. 71:185. doi: 10.1007/s00265-017-2416-z
Fei, H. X., and Henderson, G. (2003). Comparative study of incipient colony development in the Formosan subterranean termite, Coptotermes formosanus Shiraki (Isoptera, Rhinotermitidae). Insectes Soc. 50, 226–233. doi: 10.1007/s00040-003-0666-4
Fox, C. W., and Czesak, M. E. (2000). Evolutionary ecology of progeny size in arthropods. Annu. Rev. Entomol. 45, 341–369. doi: 10.1146/annurev.ento.45.1.341
French, S. S., Johnston, G. I. H., and Moore, M. C. (2007). Immune activity suppresses reproduction in food-limited female tree lizards Urosaurus ornatus. Funct. Ecol. 21, 1115–1122. doi: 10.1111/j.1365-2435.2007.01311.x
Hamilton, W. D., Axelrod, R., and Tanese, R. (1990). Sexual reproduction as an adaptation to resist parasites (a review). Proc. Natl. Acad. Sci. U.S.A. 87, 3566–3573. doi: 10.1073/pnas.87.9.3566
Hamilton, W. D., and Zuk, M. (1982). Heritable true fitness and bright birds: a role for parasites? Science 218, 384–387. doi: 10.1126/science.7123238
Han, Y. S., Chun, J., Schwartz, A., Nelson, S., and Paskewitz, S. M. (1999). Induction of mosquito hemolymph proteins in response to immune challenge and wounding. Dev. Comp. Immunol. 23, 555–562. doi: 10.1016/S0145-305X(99)00047-6
Hartke, T. R., and Rosengaus, R. B. (2013). “Costs of pleometrosis in a polygamous termite.” Proc. Biol. Sci. 280:20122563. doi: 10.1098/rspb.2012.2563
Hoffmann, A. A., and Ross, P. A. (2018). Rates and patterns of laboratory adaptation in (mostly) insects. J. Econ. Entomol. 111, 501–509. doi: 10.1093/jee/toy024
Krautz, R., Arefin, B., and Theopold, U. (2014). Damage signals in the insect immune response. Front. Plant Sci. 5:342. doi: 10.3389/fpls.2014.00342
Matsuura, K., and Kobayashi, N. (2010). Termite queens adjust egg size according to colony development. Behav. Ecol. 21, 1018–1083. doi: 10.1093/beheco/arq101
McConnel, M. W., and Judge, K. A. (2018). Body size and lifespan are condition dependent in the mealworm beetle, Tenebrio molitor, but not sexually selected traits. Behav. Ecol. Sociobiol. 72, 32. doi: 10.1007/s00265-018-2444-3
Mirabito, D., and Rosengaus, R. B. (2016). A double-edged sword? The cost of proctodeal trophallaxis in termites. Insectes Soc. 63, 135–142. doi: 10.1007/s00040-015-0448-9
Morris, D. M. (1987). Optimal allocation of parental investment. Oikos 49, 332–339. doi: 10.2307/3565769
Nalepa, C. A. (1994), “Nourishment and the origin of termite eusociality,” in Nourishment and Evolution in Insect Societies, eds J. H. Hunt and N. C. A. Boulder (Boulder, CO: Westview Press), 57–104.
Neyen, C., Runchel, C., Schüpfer, F., Meier, P., and Lemaitre, B. (2016). The regulartory isoform rPGRP-LC induces immune resolution via endosomal degredation of receptors. Nat. Immunol. 17, 1150–1160. doi: 10.1038/ni.3536
Nutting, W. L. (1969). “Flight and colony foundation,” in Biology of Termites, Vol. 1, eds K. Krishna, and F. M. Weesner (New York, NY: Academic Press), 233–282.
Osbrink, W. L. A., Kelley, S. W., Connick, W. J. Jr., Wright, M. S., and Lax, A. R. (2001). Virulence of bacteria associated with the formosan subterranean termite (Isoptera: Rhinotermitidae) in New Orleans, LA. Environ. Entomol. 30, 443–448. doi: 10.1603/0046-225X-30.2.443
Oster, G. F., and Wilson, E. O. (1978). Caste and Ecology in Social Insects. Princeton, NJ: Princeton University Press.
Parker, J., and Johnston, L. A. (2006). The proximate determinants of insect size. J. Biol. 5, 1–4. doi: 10.1186/jbiol47
Rosengaus, R. B., and Traniello, J. F. A. (1993a). Disease risk as a cost of outbreeding in the termite Zootermopsis angusticollis. Proc. Nat. Acad. Sci. U.S.A. 90, 6641–6645. doi: 10.1073/pnas.90.14.664
Rosengaus, R. B., and Traniello, J. F. A. (1993b). Temporal polytheism in incipient colonies of the primitive termite Zootermopsis angusticollis: a single multiage caste. J. Insect Behav. 6, 237–252. doi: 10.1007/bf01051507
Rosengaus, R. B., and Reicheld, J. L. (2016). Phenoloxidase activity in the infraorder Isoptera: unraveling life-hisotry correlates of immune investment. Sci. Nat. 103, 2–10. doi: 10.1007/s00114-016-1338-3
Rosengaus, R. B., James, L. T., Hartke, T. R., and Brentm, C. S. (2011b). Mate preference and disease risk in Zootermopsis angusticollis (Isoptera: Termopsidae). Environ. Entomol. 40, 1554–1556. doi: 10.1603/EN11055
Rosengaus, R. B., Maxmen, A. B., Coates, L. E., and Traniello, J. F. A. (1998). Disease resistance: a benefit of sociality in the dampwood termite Zootermopsis angusticollis (Isoptera: Termopsidae). Behav. Ecol. Sociobiol. 44, 125–134. doi: 10.1007/s002650050523
Rosengaus, R. B., Traniello, J. F. A., Chen, T., Brown, J. J., and Karp, R. D. (1999). Immunity in a social insect. Naturwissenschaften 86, 588–591. doi: 10.1007/s001140050679
Rosengaus, R. B., Traniello, J. F. A., Lefebvre, M. L., and Carlock, D. M. (2000). The social transmission of disease between adult male and female reproductives of the dampwood termite Zootermopsis angusticollis. Ethol. Ecol. Evol. 12, 419–433. doi: 10.1080/08927014.2000.9522796
Rosengaus, R. B., and Traniello, J. F. A. (2001). Disease susceptibility and the adaptive nature of colony demography in the dampwood termite Zootermopsis angusticollis. Behav. Ecol. Sociobiol. 50, 546–556. doi: 10.1007/s002650100394
Rosengaus, R. B., Traniello, J. F. A., and Bulmer, M. S. (2011a). “Ecology, behavior, and evolution of diesease resistance in termites,” in Biology of Termites: A Modern Synthesis, eds D. E. Bignell, R. Yves, and N. Lo (Dordrecht: Springer). 165–191.
Rosengaus, R. B., Moustakas, J. E., Calleri, D. V., and Traniello, J. F. A. (2003). Nesting ecology and cuticular microbial loads in dampwood (Zootermopsis angusticollois) and drywood termites (Incisitermes minor, I. Schwarzi, Cryptotermes cavifrons). J. Insect Sci. 3, 1–6. doi: 10.1093/jis/3.1.31
Schmid-Hempel, P. (2003). Variation in immune defense as a question of evolutionary ecology. Proc. R. Soc. B 270, 357–366. doi: 10.1098/rspb.2002.2265
Schmid-Hempel, P. (2017). Parasites and their social hosts. Trends Parasitol. 33, 453–462. doi: 10.1016/j.pt.2017.01.003
Schwenke, R. A., Lazzaro, B. P., and Wolfner, M. F. (2016). Reproduction- immunity trade-offs in insects. Annu. Rev. Entomol. 61, 239–256. doi: 10.1146/annurev-ento-010715-023924
Shellman-Reeve, J. S. (1990). Dynamics of biparental care in the dampwood termite, Zootermopsis nevadensis (Hagen): response to nitrogen availability. Behav. Ecol. Sociobiol. 26, 389–397. doi: 10.1007/BF00170895
Shoemaker, K. L., Parsons, N. M., and Adamo, S. A. (2006). Mating enhances parasite resistance in the cricket Gryllus texensis. Anim. Behav. 71, 371–380. doi: 10.1016/j.anbehav.2005.05.007
Siva-Jothy, M. T., Moret, Y., and Rolff, J. (2005). Insect immunity: an evolutionary ecology perspective. Adv. In Insect Phys. 32, 1–48. doi: 10.1016/S0065-2806(05)32001-7
Slaytor, M., and Chappell, D. J. (1994). Nitrogen metabolism in termites. Comp. Biochem. Physiol. B 107B, 1–10. doi: 10.1016/0305-0491(94)90218-6
Dani, S. K. G., and Kondandaramaiah, U. (2017). Plant and animal reproductive strategies: lessons from offspring size and number tradeoffs. Front. Ecol. Evol. 5, 1–21. doi: 10.3389/fevo.2017.00038
Sullivan, K., Fairn, E., and Adamo, S. A. (2016). Sickness behavior in the cricket Gryllus texensis: comparison with animals across phyla. Behav. Process. 128, 134–143. doi: 10.1016/j.beproc.2016.05.004
Vargo, E., and Hussender, C. (2011). “Genetic structure of termite colonies and populations,” in Biology of Termites: A Modern Synthesis, eds D. E. Bignell, R. Yves, and N. Lo (Dordrecht: Springer), 321–347.
Wigby, S., Domanitskaya, E. V., Choffat, Y., Kubli, E., and Chapman, T. (2008). The effect of mating on immunity can be masked by experimental piercing in female Drosophilia melanogaster. J. Insect Physiol. 54, 414–420. doi: 10.1016/j.jinsphys.2007.10.010
Wilson, E. O. (1971). The Insect Societies. Cambridge: The Belknap Press of Harvard University Press.
Xu, Y. C., Yang, D. B., and Wang, D. H. (2012). No evidence for a trade-off between reproductive investment and immunity in a rodent. PLoS ONE 7:e37182. doi: 10.1371/journal.pone.0037182
Keywords: parental effects, pathogenic stress, trade-offs, immunity, termites, colony foundation, fitness, stress ecology
Citation: Cole EL, Ilieş I and Rosengaus RB (2018) Competing Physiological Demands During Incipient Colony Foundation in a Social Insect: Consequences of Pathogenic Stress. Front. Ecol. Evol. 6:103. doi: 10.3389/fevo.2018.00103
Received: 11 May 2018; Accepted: 29 June 2018;
Published: 25 July 2018.
Edited by:
Peter Schausberger, Universität Wien, AustriaReviewed by:
Thomas Chouvenc, University of Florida, United StatesCopyright © 2018 Cole, Ilieş and Rosengaus. This is an open-access article distributed under the terms of the Creative Commons Attribution License (CC BY). The use, distribution or reproduction in other forums is permitted, provided the original author(s) and the copyright owner(s) are credited and that the original publication in this journal is cited, in accordance with accepted academic practice. No use, distribution or reproduction is permitted which does not comply with these terms.
*Correspondence: Rebeca B. Rosengaus, ci5yb3NlbmdhdXNAbm9ydGhlYXN0ZXJuLmVkdQ==
Disclaimer: All claims expressed in this article are solely those of the authors and do not necessarily represent those of their affiliated organizations, or those of the publisher, the editors and the reviewers. Any product that may be evaluated in this article or claim that may be made by its manufacturer is not guaranteed or endorsed by the publisher.
Research integrity at Frontiers
Learn more about the work of our research integrity team to safeguard the quality of each article we publish.