- 1Department of Functional Morphology and Biomechanics, Zoological Institute, Kiel University, Kiel, Germany
- 2Manaaki Whenua—Landcare Research, Auckland, New Zealand
- 3School of Biological Sciences, University of Auckland, Auckland, New Zealand
- 4Johann-Friedrich-Blumenbach-Institute of Zoology and Anthropology, University of Göttingen, Göttingen, Germany
Insects have developed specialized structures on their feet for adhering to surfaces, with stick and leaf insects or Phasmatodea exhibiting an unexpectedly high diversity of these structures. In Phasmatodea, attachment on different substrates is achieved by two types of pads on the legs: the euplantulae on the tarsomeres and the arolium on the pretarsus. The euplantulae are adhesive structures capable of adaptability to the substrate profile and generation of the required attachment strength. The diversity of euplantular microstructures of 56 species that represent all major lineages recognized within Phasmatodea and the whole biogeographical distribution of the group are examined using scanning electron microscopy (SEM). Nine different types of attachment structures can be distinguished whereby one, the nubby type, can be further divided into three different distinct types based on the specific ratio of each conical outgrowth. We mapped the morphological data from the SEM onto a phylogenetic tree we reconstructed based on molecular data. Previously, the evolution of different adhesive microstructures (AMs) on these pads has been suggested to reflect phylogenetic groups. However, different types of AMs are found within monophyletic groups, and our ancestral character state reconstruction suggests smooth euplantulae in the ground pattern of Euphasmatodea and multiple independent origins of other forms. The type of AM appears to be strongly associated with ecomorphs, e.g., smooth euplantular surfaces are more frequently found in tree-dwellers than in ground-dwellers, whilst the attachment pads of ground-dwelling species primarily bear conical cuticular outgrowths (nubby euplantulae).
Introduction
The mesodiverse Phasmatodea are widespread, inhabiting nearly all temperate and tropical ecosystems worldwide (Günther, 1953; Bedford, 1978; Brock et al., 2017), but have only limited dispersal capabilities (Bradler et al., 2015). These exclusively phytophagous insects are well-camouflaged in their preferred habitats due to their masquerade imitating leafs or twigs (Bradler, 2009). While undergoing a fast radiation since the Cretaceous (Bradler et al., 2015) the slowly migrating phasmids adapted to very local environmental settings. In a wide distributional range various ecological adaptations occurred independently in different lineages, causing different species inhabiting similar environments to display similar adaptations (e.g., Buckley et al., 2009; Dennis et al., 2015).
Since the wide distribution and occupancy of similar ecological niches has led to many convergences in Phasmatodea, many distinct morphological adaptations, such as specialized egg deposition modes, are more likely to form ecomorphological groups within this lineage than to reflect phylogenetic relationships (Buckley et al., 2009; Bradler et al., 2015; Goldberg et al., 2015). For instance, the exclusively ground dwelling tree lobsters (e.g., Droycoccelus, Eurycantha, Canachus) are shown to be polyphyletic, but adapted to specific environmental conditions, leading to many parallel evolvements. These formerly taxonomically associated species exhibit a very similar habitus, which is robust, dorsoventrally depressed and includes sturdy, armed legs. They dwell on the ground and all bury their eggs therein, usually with a secondary ovipositor (Buckley et al., 2009). Such a distinct ecological specification is attended by dissimilar substrata, species dwell on in their preferred habitats, and most probably includes adaptations of the attachment devices of these insects (Gottardo et al., 2015).
In order to adhere securely in the inhabited environment, phasmids possess specialized structures on their tarsi (Figure 1). Especially species living in the canopy depend on secure attachment to avoid potential damage from dropping to the ground (Schmitt et al., 2018). In addition to the pretarsal claws two main types of attachment pads are used in phasmids. The pretarsus is equipped with a pan-shaped toe pad (arolium) in every species. This particular attachment pad is suggested to create adhesion, if the tarsus is pulled from the surface (Labonte and Federle, 2013). On the proximal tarsomeres a small number of heel pads (euplantulae) are situated. Most species possess one euplantula on each of the four proximal tarsomeres enabling adjustment to the surface profile. In some species, a fifth euplantula is found on the distal tarsomere as well (Vallotto et al., 2016). In contrast to the arolium, the euplantulae generate large friction coefficients, when they are pressed onto the substrate due to shear forces, but create negligible adhesion (Busshardt et al., 2011, 2012; Labonte and Federle, 2013; Labonte et al., 2014).
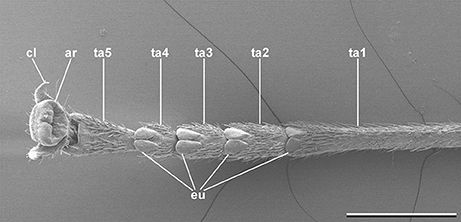
Figure 1. Typical phasmatodean tarsus, Clonaria conformans (Gratidiini). cl, tarsal claw; ar, arolium; eu, euplantula; ta1-5, tarsomeres 1-5. Scale bar: 1 mm.
Attachment in insects is achieved in general by adaption to the surface profile in order to maximize the actual contact area. This is accomplished either by flexible setose structures, or by soft cuticle layers (Gorb, 2001, 2005; Bennemann et al., 2014). Both principles appear in different groups of insects (Beutel and Gorb, 2001; Grohmann et al., 2015). Hairy systems, consisting of deformable adhesive setae, are common in spiders (Gorb et al., 2006), beetles (Stork, 1980; Gorb and Gorb, 2002; Bullock and Federle, 2011), earwigs (Haas and Gorb, 2004), and flies (Bauchhenss, 1979; Gorb, 1998; Niederegger et al., 2002; Friedemann et al., 2014). The other system consists of cuticular pads, which bear no prominent ornamentation, such as setae, but might bear different adhesive microstructures (Beutel and Gorb, 2008). This type is common in Phasmatodea (Büscher and Gorb, 2017) and also found in Orthoptera (Gorb et al., 2000; Perez Goodwyn et al., 2006), Hymenoptera (Federle et al., 2001, 2002; Frantsevich and Gorb, 2004), and Blattodea (Clemente and Federle, 2008).
For hexapods in general, the structural diversity of the attachment devices has been demonstrated to reflect phylogenetic relationships and, for the Phasmatodea and their controversely discussed sister groups, potential evolutionary scenarios were postulated based on these traits (Beutel and Gorb, 2001, 2006, 2008). The presence of smooth arolia and euplantulae, without macroscopic adhesive structures, has been discussed with respect to a phylogenetic placement of Phasmatodea as sister group to Mantophasmatodea, emphasizing the morphological similarity of adhesive systems in both groups (Beutel and Gorb, 2008). Nevertheless, recent morphological and molecular approaches support the sister group relation of Embioptera and Phasmatodea (Ishiwata et al., 2011; Friedemann et al., 2012; Letsch et al., 2012; Letsch and Simon, 2013; Misof et al., 2014). Although the attachment pads of stick insects appear to be smooth on a macroscopical level, the basal splitting of Timema and Euphasmatoda (the remaining Phasmatodea excluding Timema), is supported by the ultrastructure of the arolium, which is covered with pointed acanthae in Timema and entirely smooth in the latter. This sister group relationship of Timema and Euphasmatodea is considered well supported (e.g., Bradler, 2009). Beutel and Gorb (2008) distinguished the pointed acanthae on the euplantulae of Timema and the nubby adhesive microstructures (AM) of the euphamatodeans Aretaon asperrimus and Neohirasea maerens. The nubs found in these two taxa were referred to as low aspect-ratio acanthae, but subsequent discussion regarding their structure used various different terms, including microtrichia (Gottardo and Heller, 2012) and bumps (Zill et al., 2014) without considering the cellular origin of these structures. Similar AM are found on the euplantulae of stick insects in a considerable diversity, as revealed in various taxonomic descriptions, biomechanic studies, and phylogenetic approaches. Five types of AM have been found so far: in addition to the pointed acanthae in Timema, nubs with two different aspect ratios are found in taxa from different lineages of euphasmatodeans. Carausius morosus possesses comparatively long nubs (Busshardt et al., 2012), while other stick insects from different lineages bear shorter nubs (Beutel and Gorb, 2008; Gottardo and Heller, 2012; Büscher and Gorb, 2017). Some taxa possess entirely smooth euplantulae without any micromorphological ornamentation (Busshardt et al., 2012; Gottardo and Vallotto, 2014; Gottardo et al., 2015; Vallotto et al., 2016; Büscher and Gorb, 2017), and Dallaiphasma eximius revealed a hexagonal or plateau pattern (Gottardo, 2011). Although the structural diversity of phasmatodean AMs is known (Büscher et al., 2018) and the phylogenetic clustering of similar AMs is supposed to partly reflect the evolution of Phasmatodea (Gottardo et al., 2015; Büscher and Gorb, 2017), there is no recent study approaching the phylogenetic relationships of the different AMs. We therefore examine the diversity of euplantular AMs within Phasmatodea in correlation with the phylogeny of the species examined.
Materials and Methods
Morphology
The euplantulae of 56 adult female specimens were examined using SEM. These species represent the major lineages currently recognized in Phasmatodea (see Supplementary Data 1: Supplementary Table S1 for detailed information). Living specimens were bred in captivity until they reached adulthood. The insects were then anesthetized with CO2 and then decapitated. The tarsi were cut off and fixated in a 2.5% solution of glutaraldehyde in PBS buffer and stored on ice on a shaker for 24 h. In the case, when no living specimens were available, dried specimens from collections were used. In order to restore the original condition of attachment pads, the cuticle has been softened. Therefore, the tarsi were removed and stored in a relaxing chamber for 24 h. Afterwards the tarsi were put into a 10% solution of lactic acid for 24–48 h until the attachment pads were restored into an adequate condition and then fixed in a 2.5% solution of glutaraldehyde in PBS buffer (Gladun and Gumovsky, 2006). Then specimens were stored in 70% ethanol, dehydrated in an ascending alcohol series and critical-point dried. Dried samples were mounted on aluminum stubs and sputter-coated with a 15 nm thick layer of gold-palladium. Samples were observed in the scanning electron microscope (SEM) Hitachi S4800 (Hitachi High-Technologies Corp., Tokio, Japan) at 7 kV of acceleration voltage.
Phylogenetic Analysis
DNA has been extracted and sequenced for 14 stick insect specimens. We combined these data with previously sequenced taxa (Buckley et al., 2009; Goldberg et al., 2015). Sampled taxa were chosen with a focus on the different ecomorphs, covering the entire geographic distribution of Phasmatodea and all extant stick insect lineages. We amplified regions of the mitochondrial cytochrome c-oxidase subunit I (COI) and II (COII) genes, and the nuclear histone subunit 3 (H3) and ribosomal large subunit RNA gene (28S) using methods described previously (Buckley et al., 2009). DNA sequences were edited in Geneious R10 (Kearse et al., 2012). Alignments were made using Muscle (Edgar, 2004) as implemented in Geneious R10. The alignments were partitioned into four sets of characters; mitochondrial 1st and 2nd codon positions, mitochondrial 3rd codon positions, H3 gene, and 28S gene. Use of a partitioned model allows us to account for the typically different substitution patterns between different genes and codon positions, especially the increased rate at the third codon positions. Model selection using the AIC in JModelTest v.2.1.3 (Darriba et al., 2012) was then performed independently on each partition. Phylogenetic reconstruction was then performed using MrBayes v3.2.6 (Ronquist et al., 2012). For prior distributions we used a flat Dirichlet distribution (1,1,1,1) on “ratepr,” informative Dirichlet distribution (1,2,1,1,2,1) on “revmatpr,” beta distribution (1,1) on “tratiopr,” exponential distribution (1) on “shapepr,” and an unconstrained exponential distribution (1) on “brlens,” State frequencies were fixed at empirical values. We ran five independent analyses of 10 million generations, four chains, a sample frequency of 1000, and an MCMCMC temperature of 0.2. Output files were inspected in Tracer v1.6.0 (http://tree.bio.ed.ac.uk/software/tracer/) to ensure all effective sample sizes were >200 and MCMC chains were mixing appropriately. We also reconstructed phylogenetic relationships using maximum likelihood as implemented in Garli (Zwickl, 2006). We used the same partitioned substitution model as in the Bayesian analysis. The data were bootstrapped with 100 replicates and 1 search replicate per bootstrap. Ancestral character states were reconstructed using the Mkv model (Lewis, 2001) as implemented in Mesquite v3.4 (Maddison and Maddison, 2018). Ancestral states were estimated on the topology and branch lengths from the Bayesian analysis. The alignment used for the phylogenetic reconstruction is provided as a Nexus input file as Supplementary Data Sheet 1.
Results
Morphology
The tarsus of stick insects generally consists of five tarsomeres (Figure 1). The pretarsus is equipped with two claws and an arolium. Tarsomeres 1–4 bear distal euplantulae in all species studied. In some species, an accessory euplantula is found on the fifth tarsomere. The whole tarsal morphology reveals no intraspecific difference, neither between different specimens, nor in the micromorphological features within the arolium and euplantulae, nor between different legs of the same individual, nor between different euplantulae on the same tarsus. We found nine types of AM among the examined species. Adult females of exemplary species for the corresponding AMs are shown in Figure 2. Smooth euplantular surfaces are the most frequently observed forms (Figure 3A), followed by conical outgrows of different aspect ratios (nubby, Figures 3F–H). Rare forms include flat pads (found in Necroscia annulipes, Figure 3B), plateaus (present in Epidares nolimetangere and Dajaca monilicornis, Figures 3C,L), coarse (found in Kalokorinnis and Oreophoetes, Figure 3D), maze (found in Leiophasma, Figure 3E), ridges (found in the Lanceocercata taxa Argosarchus horridus and Megacrania phelaus, Figure 3I), acanthae (only found in Timema, Figure 3J, Beutel and Gorb, 2008), and hairs (setae, present only in Dinophasma saginatum, Figure 3K). The AMs of every of these species is illustrated and described in detail in Büscher et al. (2018).
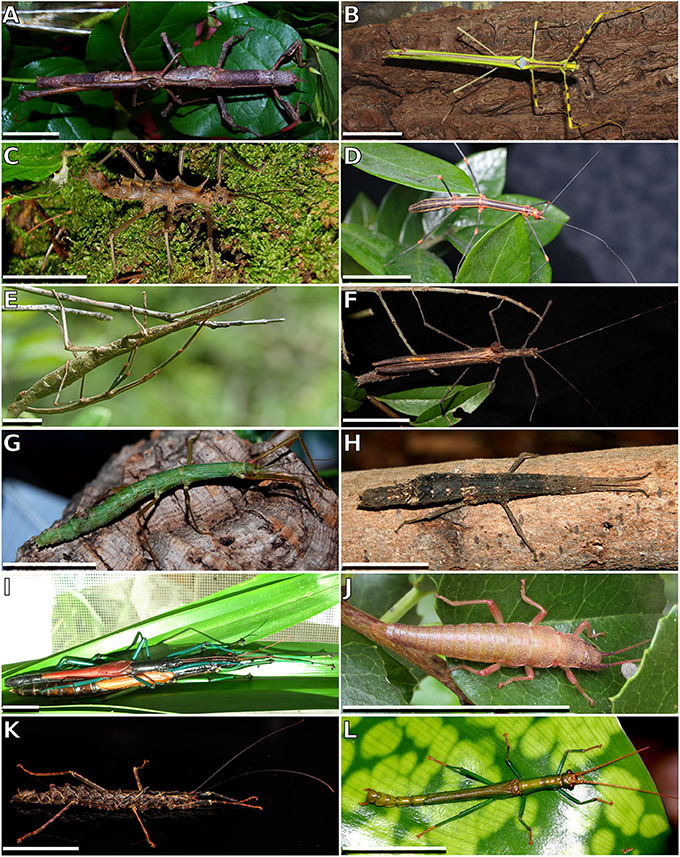
Figure 2. Exemplary members of Phasmatodea. (A) Cigarrophasma tessulatum, couple, image provided by Daniel Dittmar. (B) Necroscia annulipes, female, image provided by Holger Dräger. (C) Epidares nolimetangere, female. (D) Oreophoetes peruana, female. (E) Leiophasma sp., couple, image provided by Paul Bertner. (F) Pseudophasma velutinum, female, image provided by Holger Dräger. (G) Xylica oedematosa, female, image provided by Daniel Dittmar. (H) Orestes mouhotii, female. (I) Megacrania phelaus, couple, image provided by Bruno Kneubühler. (J) Timema sp., female, image provided by Royce Cumming. (K) Dinophasma saginatum, female, image provided by Bruno Kneubühler. (L) Dajaca monilicornis, male, image provided by Luis Mata. Scale bars: 20 mm.
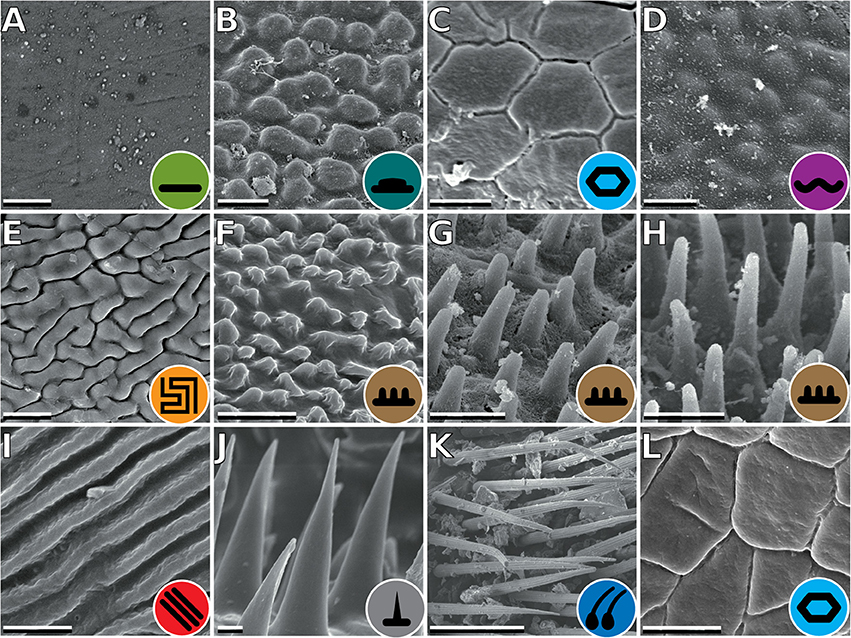
Figure 3. Types of attachment microstructures in Phasmatodea. (A) Smooth, Cranidium gibbosum. (B) Flat pads, Necroscia annulipes. (C) Plateaus, Epidares nolimetangere. (D) Coarse, Kalocorinnis wegneri. (E) Maze, Leiophasma sp. (F) Nubby (small), Pseudophasma velutinum. (G) Nubby (median), Xylica oedematosa. (H) Nubby (long), Orestes mouhotii. (I) Ridges, Megacrania phelaus. (J) Acanthae, Timema sp. (K) Hairs, Dinophasma saginatum. (L) Plateaus, Dajaca monilicornis. Scale bars: (A–J). 2 μm; (K). 50 μm.
Phylogenetic Relationships
The DNA sequence alignment consisted of 762 base pairs (bp), 695, 328, and 615 from the COI, COII, H3, and 28S genes respectively. The maximum likelihood relative substitutions rates of mitochondrial 1st + 2nd codon positions, mitochondrial 3rd codon positions, H3 and 28S genes were 0.035, 4.732, 0.065, and 0.077 respectively. We observed strong support [Bayesian posterior probability (BPP) = 1] for several monophyletic groups that were also recovered in previous studies (Figure 4), including Necrosciinae, Phylliinae, Aschiphasmatinae, Pseudophasmatidae, Lanceocercata, and Anisacanthidae (Leiophasma + Parectatosoma). However, bootstrap (BS) values were somewhat lower with only Phylliinae, Aschiphasmatinae, and Anisacanthidae (Leiophasma + Parectatosoma) receiving 100% BS support. Clades not supported, but also monophyletic in accordance with previous studies (Bradler et al., 2014, 2015; Goldberg et al., 2015) are Clitumninae (BPP = 0.54, BS < 50%), Diapheromerinae (BPP = 0.53, BS = 72%), and Heteropteryginae (BPP = 0.74, BS < 50%). The three subgroups of the latter, Dataminae (Epidares + Pylaemenes + Orestes), Heteropteryginae (Haaniella + Heteropteryx), and Obriminae (Aretaon + Sungaya) are each highly supported with BPP values of 1 and BS values of 98%, 100% and 100% respectively. Dataminae and Heteropteryginae are placed as sister taxa (BPP = 0.71, BS < 50%), which is in contrast to previous studies that suggest alternative topologies for these groups (Bradler et al., 2015; Goldberg et al., 2015). Further noteworthy results include a rather robust topology for Lanceocercata that largely corroborates earlier analyses, including a clade of taxa from New Caledonia (Canachus) + New Zealand (Argosarchus + Clitarchus) (BPP = 1, BS = 93%) and a sister group relationship between Dimorphodes and all remaining Lanceocercata, and with Cladomorphinae being sister clade to Stephanacridini (Macrophasma) + Lanceocercata (Buckley et al., 2009, 2010; Bradler et al., 2014, 2015). Necrosciinae also comprises Korinninae (Kalokorinnis) as suggested before (Goldberg et al., 2015) and Conlephasma, which has been discussed as being either related to Necrosciinae or Pseudophasmatidae (Gottardo and Heller, 2012) and is here unambiguously placed as sister group to Neohirasea + Phaenopharos (BPP = 1, BS = 92%) within Necrosciinae. A Malagasy clade consisting of Achrioptera + Anisacanthiade is well supported (BPP = 1, BS = 68%) as recovered before by Bradler et al. (2015). A further noteworthy result is the placement of the enigmatic African Bacillinae taxon Xylica as sister group to Aschiphasmatinae (BPP = 1, BS = 59%), which corroborates the finding of Buckley et al. (2009). One surprising result is our recovery of Phylliinae nested within Lonchodinae, albeit weakly supported. This is at odds with all previous phylogenetic analyses observing monophyletic Lonchodinae (Buckley et al., 2009; Bradler et al., 2014, 2015; Goldberg et al., 2015), and we attribute this result to a shortage of taxon sampling within these two subfamilies. The deeper nodes, e.g., the radiation of major phasmatodean lineages and enigmatic longstanding taxa like Agathemera, Bacillus, and Bactrododema, are poorly resolved and largely unsupported, which is a common observation for phylogenetic studies of Phasmatodea (Buckley et al., 2009; Bradler et al., 2014, 2015; Goldberg et al., 2015).
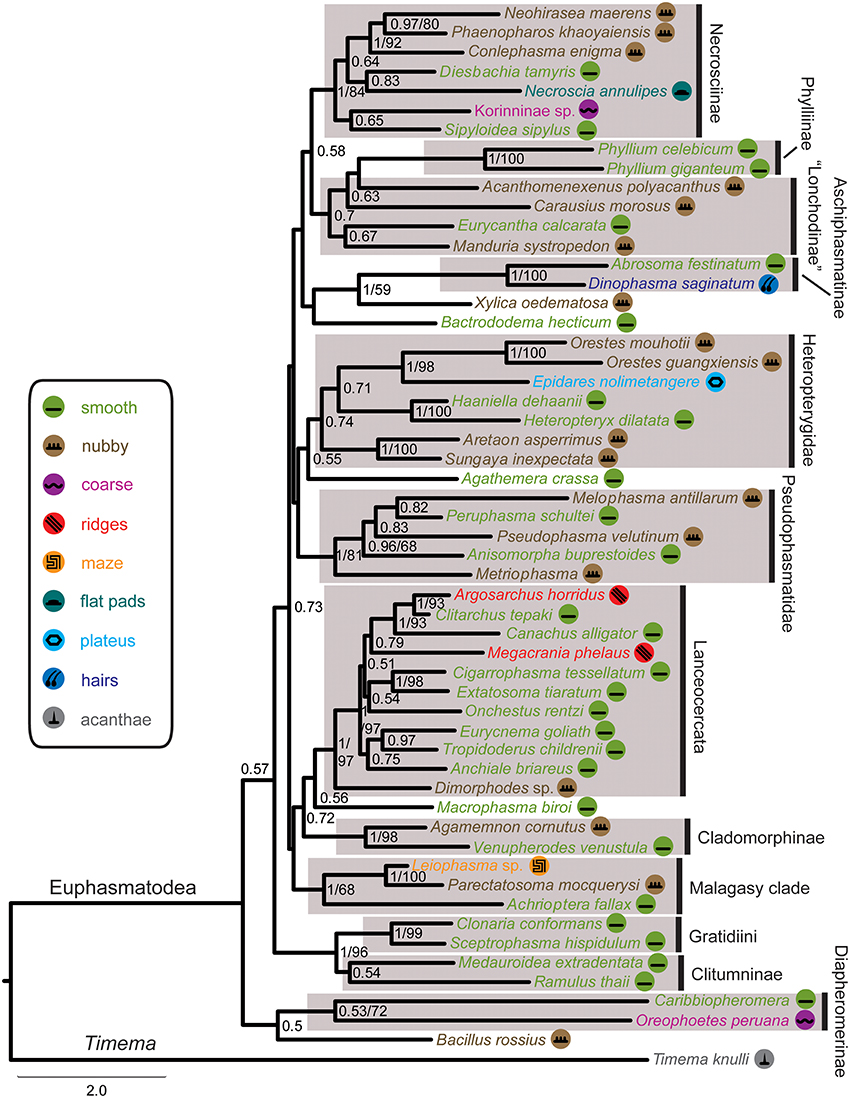
Figure 4. Majority-rule consensus tree of investigated Phasmatodea resulting from Bayesian analysis of the combined molecular data. Posterior probabilities followed by bootstrap percentages are given at nodes. Symbols mapped on taxa and color code of tarsal structures/euplantulae type according to figure legend on the left.
Discussion
Evolution of Attachment Structures
The recovered phylogeny was largely consistent with current phylogenetic hypotheses on stick and leaf insects with the exception of the placement of Phylliinae within Lonchodinae, which is not well supported and probably an artifact due to the limited taxon sampling of the present study. The poorly resolved radiation among the major phasmatodean lineages impedes a reliable ancestral character state reconstruction of euplantular adhesive structures for stick and leaf insects at the moment. Furthermore, the condition for this character complex in Timema (acanthae) is unlike in any of the investigated members of its sister group Euphasmatodea, which consequently does not allow for an outgroup comparison. We are confident that one of the most frequent forms, smooth and nubby, which are also present in closely related groups like Orthoptera, Blattodea, and Embioptera (Gorb et al., 2000; Perez Goodwyn et al., 2006; Clemente and Federle, 2008; Büscher et al., 2018), represents the ground pattern of AM in Euphasmatodea, and indeed the ancestral state reconstructions favor smooth (Figure 5). We also conclude that the rare AM types are derived for the respective taxa, e.g., coarse for Kalokorinnis and Oreophoetes, plateaus for Epidares, maze for Leiophasma, and hairs (adhesive setae) for Dinophasma, and this is supported by the ancestral state reconstructions. Moreover, the euplantular structure ridges evolved twice within Lanceocercata, in Argosarchus and Megacrania. Our reconstructions support independent origins of the nubby types (Figure 5). In general, unambiguously monophyletic groups exhibit a diversity of structures, e.g., in Aschiphasmatinae the smooth (Abrosoma), hairy (Dinophasma), and plateaus (Dajaca) forms are found. While some closely related taxa possess the same euplantular types, such as nubby AMs in Neohirasea, Phaenopharos, and Conlephasma in Necrosciinae, or smooth AMs in Anchiale, Eurycnema, and Tropidoderus in Lanceocercata, the occurrence of specific AMs do not generally reflect phylogenetic relationships. Besides Clitumninae and Phylliinae, none of the well-supported major clades bears a uniform AM type. The type of AM appears to be associated with ecomorphs, e.g., smooth euplantular surfaces are more frequently found in slender tree-dwellers than in stout ground-dwellers, whilst the attachment pads of ground-dwelling species more often bear conical cuticular outgrowths with different aspect ratios. However, this does not entirely explain the distribution of AMs across phasmid taxa. For instance, Heteropterygidae are uniformly ground-dwelling phasmids, including egg-deposition into the soil, yet they exhibit varying types of adhesive structures: In Obriminae (Aretaon, Sungaya), the euplantular surface is nubby, whereas smooth euplantulae are found in Heteropteryginae (Heteropteryx, Haaniella). Yet, characterization of distinct ecomorphs still needs to be addressed based on substantiated criteria such as morphometrics and niche specialization. One further potential explanation of the presence of certain AMs is body size. Bigger species might exhibit smooth euplantulae more frequently, e.g., the large Bactrododema, Eurycnema, Achrioptera, and Eurycantha. Assumption of this potential trend gains further support by the fact that the smaller juvenile Eurycantha individuals still have nubby euplantulae while those of adults are smooth (Gottardo et al., 2015). Heteropteryginae bearing smooth euplantulae are generally bigger than Obriminae with nubby euplantulae. Then again, the ground-dwelling Orestes and Epidares are of the same small size and still possess different AMs.
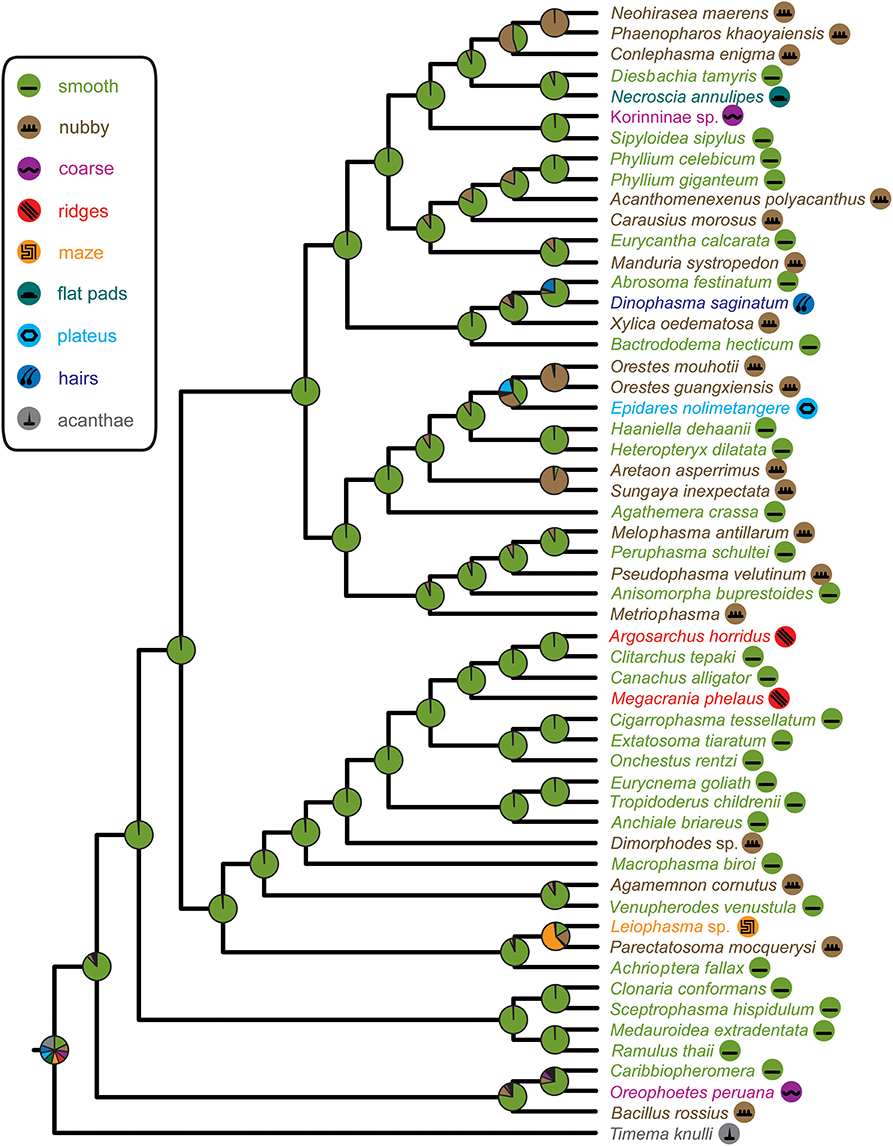
Figure 5. Phasmatodea phylogeny and ancestral character state reconstruction of euplantular microstructures with pie charts representing relative ML support at ancestral nodes according to color code in figure legend.
Functional Relevance of Attachment Microstructures
The functional properties of the different AMs in Phasmatodea are only partially known so far. Experimental data are available for Phasmatodea only for the nubby and the smooth type (Busshardt et al., 2012; Labonte and Federle, 2013; Labonte et al., 2014), hence, the other remain hypothetical or are inferred from other taxa. The nubby AM is shown to be load sensitive and less susceptible to different surface roughnesses (Busshardt et al., 2012; Labonte and Federle, 2013; Labonte et al., 2014). Nubby attachment microstructures, if they are not the ground type of AMs, possibly evolved convergently in the different lineages as a response to a broad range of surfaces in the preferred habitats. In contrast, smooth AMs perform better on smooth surfaces (Busshardt et al., 2012). Many species possessing this AM live in trees and often are food plant specialists. These species possibly face rather smooth surfaces, whilst species with nubby structures possibly find a broader range of surface roughnesses on the ground. Other types of AMs might have evolved due to insect-plant interactions (e.g., Friedemann et al., 2015) or specific environmental conditions, e.g., specialized structured surfaces or wet surfaces (Grohmann et al., 2015). On wet surfaces, splitting the contact surface like in the flat pads, plateaus, and maze AMs possibly reduces hydroplaning and stick-slip motions. This effect has been shown for mushroom shaped and hexagonal bioinspired artificial surfaces (Varenberg and Gorb, 2007, 2009). Irregularly shaped microstructures, such as ridges, might have evolved due to food plant specialization. The anisotropy of the AM causes dissimilar adhesive forces with and against the structures (Filippov and Gorb, 2013) and might be used for generating propulsion on structured surfaces (Clemente et al., 2009). Megacrania phelaus feeds exclusively on plants with a parallel leaf venation (Hsiung, 2007). This food plant association possibly initiated the development of the structured AM in this species. The hairy system of attachment pads, herein only represented by D. saginatum, is reported to possess similar adhesion and friction forces to the smooth system (Bullock et al., 2008). The comparison of the adhesive pads of C. morosus and the dock beetle Gastrophysa viridula (Coleoptera: Chrysomelidae) suggested that fibrillary adhesive systems may be more efficient in terms of self-cleaning than smooth ones (Clemente et al., 2010).
Conclusion
Stick and leaf insects have more diverse euplantular microstructures than previously reported (Beutel and Gorb, 2008). Nine different types can be distinguished whereby the nubby type can be further divided into three different distinct types based on the specific ratio of each conical outgrowth. Hereby the different types of AMs do not follow a phylogenetic pattern, but rather depend on the ecological niche a species inhabits or its body size. Large canopy-dwellers more frequently appear to exhibit smooth euplantulae while smaller ground-dwellers apparently show nubby AMs. The morphological diversity found in each clade of phasmids suggests the convergent evolution or reversal of certain euplantular types. A high number of species is already illustrated and described in a comprehensive comparative analysis of the tarsal morphology in Phasmatodea (Büscher et al., 2018). To reliably reconstruct the evolution of these adhesive types, a denser taxon sampling and a better resolved phylogeny of these taxa are necessary.
Data Availability Statement
All datasets analyzed for this study are included in the manuscript and the Supplementary Material. The DNA sequences used for the phylogenetic reconstruction can be found at GenBank [https://www.ncbi.nlm.nih.gov/genbank/] under the accession numbers listed in Supplementary Table 1. The alignment used for the phylogenetic reconstruction is provided as a Nexus input file as Supplementary Data Sheet 1.
Author Contributions
THB, CG, SG, and SB designed research. THB generated SEM data and photographs. TRB obtained and analyzed molecular data. THB and SB wrote manuscript. TRB, CG, and SG contributed to manuscript editing. All authors have approved the final version of the manuscript.
Funding
This study was supported by the German Science Foundation (DFG grants BR 2930/3-1, BR 2930/4-1 and BR 2930/5-1 to SB).
Conflict of Interest Statement
The authors declare that the research was conducted in the absence of any commercial or financial relationships that could be construed as a potential conflict of interest.
Acknowledgments
We thank Esther Appel and Alexander Kovalev (Department of Biomechanics and Functional Morphology, Kiel University, Germany) for support in microscopy and preservation. Bruno Kneubühler (Lucerne, Switzerland), Daniel Dittmar (Berlin, Germany), Royce Cumming (San Diego Natural History Museum, San Diego, USA), Paul Bertner (Winfield, Canada), Luis Mata (RMIT University, Melbourne, Australia), and Holger Dräger (Schwerin, Germany) are acknowledged for providing specimens and photographs. We acknowledge financial support by Land Schleswig-Holstein within the funding programme Open Access Publikationsfonds.
Supplementary Material
The Supplementary Material for this article can be found online at: https://www.frontiersin.org/articles/10.3389/fevo.2018.00069/full#supplementary-material
Supplementary Table 1. Taxon sampling.
Supplementary Data Sheet. Nexus input file.
References
Bauchhenss, E. (1979). Die Pulvillen von Calliphora erythrocephala Meig. (Diptera, Brachycera) als Adhäsionsorgane. Zoomorphologie 93, 99–123. doi: 10.1007/BF00994125
Bedford, G. O. (1978). Biology and ecology of the Phasmatodea. Ann. Rev. Entomol. 23, 125–149. doi: 10.1146/annurev.en.23.010178.001013
Bennemann, M., Backhaus, S., Scholz, I., Park, D., Mayer, J., and Baumgartner, W. (2014). Determination of the Young's modulus of the epicuticle of the smooth adhesive organs of Carausius morosus using tensile testing. J. Exp. Biol. 217, 3677–3687. doi: 10.1242/jeb.105114
Beutel, R. G., and Gorb, S. N. (2001). Ultrastructure of attachment specializations of hexapods (Arthropoda). Evolutionary patterns inferred from a revised ordinal phylogeny. J. Zool. Syst. Evol. Res. 39, 177–207. doi: 10.1046/j.1439-0469.2001.00155.x
Beutel, R. G., and Gorb, S. N. (2006). A revised interpretation of the evolution of attachment structures in Hexapoda with special emphasis on Mantophasmatodea. Arthropod Syst. Phylogeny 64, 3–25.
Beutel, R. G., and Gorb, S. N. (2008). Evolutionary scenarios for unusual attachment devices of Phasmatodea and Mantophasmatodea (Insecta). Sys. Ent. 33, 501–510. doi: 10.1111/j.1365-3113.2008.00428.x
Bradler, S. (2009). Die Phylogenie der Stab- und Gespenstschrecken (Insecta: Phasmatodea). Göttingen: Universitätsverlag. 139.
Bradler, S., Cliquennois, N., and Buckley, T. R. (2015). Single origin of Mascarene stick insects: ancient radiation on sunken islands? BMC Evol. Biol. 15, 196. doi: 10.1186/s12862-015-0478-y
Bradler, S., Robertson, J. A., and Whiting, M. F. (2014). A molecular phylogeny of Phasmatodea with emphasis on Necrosciinae, the most species-rich subfamily of stick insects. Sys. Ent. 39, 205–222. doi: 10.1111/syen.12055
Brock, P. D., Büscher, T. H., and Baker, E. (2017). “Phasmida species file online: phasmida species file version 5.0/5.0,” in Species 2000 and ITIS Catalogue of Life, eds eds Y. Roskov et al. (Leiden: Species 2000: Naturalis). Available online at: www.catalogueoflife.org/col
Buckley, T. R., Attanayake, D., and Bradler, S. (2009). Extreme convergence in stick insect evolution: phylogenetic placement of the Lord Howe Island tree lobster. Proc. R. Soc. Lond. B 276, 1055–1062. doi: 10.1098/rspb.2008.1552
Buckley, T. R., Attanayake, D., Nylander, J. A. A., and Bradler, S. (2010). The phylogenetic placement and biogeographical origins of the New Zealand stick insects (Phasmatodea). Sys. Ent. 35, 207–225. doi: 10.1111/j.1365-3113.2009.00505.x
Bullock, J. M., and Federle, W. (2011). Beetle adhesive hairs differ in stiffness and stickiness: in vivo adhesion measurements on individual setae. Naturwissenschaften 98, 381–387. doi: 10.1007/s00114-011-0781-4
Bullock, J. M. R., Drechsler, P., and Federle, W. (2008). Comparison of smooth and hairy attachment pads in insects: friction, adhesion and mechanisms for direction-dependence. J. Exp. Biol. 211, 3333–3343. doi: 10.1242/jeb.020941
Büscher, T. H., and Gorb, S. N. (2017). Subdivision of the neotropical Prisopodinae Brunner von Wattenwyl 1893 based on features of tarsal attachment pads (Insecta: Phasmatodea). Zookeys 645, 1–11. doi: 10.3897/zookeys.645.10783
Büscher, T. H., Grohmann, C., Bradler, S., and Gorb, S. N. (2018). Tarsal Attachment Pads in Phasmatodea (Hexapoda: Insecta). Zoologica. Stuttgart: Schweizerbart Science Publishers.
Busshardt, P., Gorb, S. N., and Wolf, H. (2011). Activity of the claw retractor muscle in stick insects in wall and ceiling situations. J. Exp. Biol. 214, 1676–1684. doi: 10.1242/jeb.051953
Busshardt, P., Wolf, H., and Gorb, S. N. (2012). Adhesive and frictional properties of tarsal attachment pads in two species of stick insects (Phasmatodea) with smooth and nubby euplantulae. Zoology 115, 135–141. doi: 10.1016/j.zool.2011.11.002
Clemente, C. J., and Federle, W (2008). Pushing versus pulling: division of labour between tarsal attachment pads in cockroaches. Proc. R. Soc. Lond. B 275, 1329–1336. doi: 10.1098/rspb.2007.1660
Clemente, C. J., Bullock, J. M., Beale, A., and Federle, W. (2010). Evidence for self-cleaning in fluid-based smooth and hairy adhesive systems of insects. J. Exp. Biol. 213, 635–642. doi: 10.1242/jeb.038232
Clemente, C. J., Dirks, J.-H., Barbero, D. R., Steiner, U., and Federle, W. (2009). Friction ridges in cockroach climbing pads: anisotropy of shear stress measured on transparent, microstructured substrates. J. Comp. Physiol. A 195, 805–814. doi: 10.1007/s00359-009-0457-0
Darriba, D., Taboada, G. L., Doallo, R., and Posada, D. (2012). JModelTest 2: more models, new heuristics and parallel computing. Nat. Methods 9, 772. doi: 10.1038/nmeth.2109
Dennis, A. B., Dunning, L. T., Sinclair, B. J., and Buckley, T. R. (2015). Parallel molecular routes to cold adaptation in eight genera of New Zealand stick insects. Sci. Rep. 5, 13965. doi: 10.1038/srep13965
Edgar, R. C. (2004). MUSCLE: multiple sequence alignment with high accuracy and high throughput. Nucleic Acids Res. 32, 1792–1797. doi: 10.1093/nar/gkh340
Federle, W., Brainerd, E. L., McMahon, T. A., and Hölldobler, B. (2001). Biomechanics of the movable pretarsal adhesive organ in ants and bees. Proc. Natl. Acad. Sci. U.S.A. 98, 6215–6220. doi: 10.1073/pnas.111139298
Federle, W., Riehle, M., Curtis, A. S., and Full, R. J. (2002). An integrative study of insect adhesion: mechanics and wet adhesion of pretarsal pads in ants. Integr. Comp. Biol. 42, 1100–1106. doi: 10.1093/icb/42.6.1100
Filippov, A., and Gorb, S. N. (2013). Frictional-anisotropy-based systems in biology: structural diversity and numerical model. Sci. Rep. 3, 1240. doi: 10.1038/srep01240
Frantsevich, L., and Gorb, S. (2004). Structure and mechanics of the tarsal chain in the hornet, Vespa crabro (Hymenoptera: Vespidae): implications on the attachment mechanism. Arthropod. Struct. Dev. 33, 77–89. doi: 10.1016/j.asd.2003.10.003
Friedemann, K., Kunert, G., Gorb, E. V., Gorb, S. N., and Beutel, R. G. (2015). Attachment forces of pea aphids (Acyrthosiphon pisum) on different legume species. Ecol. Entomol. 40, 732–740. doi: 10.1111/een.12249
Friedemann, K., Schneeberg, K., and Beutel, R. G. (2014). Fly on the wall – attachment structures in lower Diptera. Syst. Ent. 39, 460–473. doi: 10.1111/syen.12064
Friedemann, K., Wipfler, B., Bradler, S., and Beutel, R. G. (2012). On the head morphology of Phyllium and the phylogenetic relationships of Phasmatodea (Insecta). Acta Zool. 93, 184–199. doi: 10.1111/j.1463-6395.2010.00497.x
Gladun, D., and Gumovsky, A. (2006). The pretarsus in Chalcidoidea (Hymenoptera Parasitica). Functional morphology and possible phylogenetic implications. Zool. Scripta 35, 607–626. doi: 10.1111/j.1463-6409.2006.00245.x
Goldberg, J., Bresseel, J., Constant, J., Kneubühler, B., Leubner, F., Michalik, P., et al. (2015). Extreme convergence in egg-laying strategy across insect orders. Sci. Rep. 5, 7825. doi: 10.1038/srep07825
Gorb, S. N. (2001). Attachment Devices of Insect Cuticle. Dordrecht; Boston, MA; London: Kluwer Academic Publishers.
Gorb, E. V., and Gorb, S. N. (2002). Attachment ability of the beetle Chrysolina fastuosa on various plant surfaces. Entomol. Exp. Appl. 105, 13–28. doi: 10.1046/j.1570-7458.2002.01028.x
Gorb, S. N. (1998). The design of the fly adhesive pad. Distal tenent setae are adapted to the delivery of an adhesive secretion. Proc. R. Soc. Lond. B 265, 747–752. doi: 10.1098/rspb.1998.0356
Gorb, S. N. (2005). Uncovering insect stickiness: structure and properties of hairy attachment devices. Am. Entomol. 51, 31–35. doi: 10.1093/ae/51.1.31
Gorb, S., Jiao, Y., and Scherge, M. (2000). Ultrastructural architecture and mechanical properties of attachment pads in Tettigonia viridissima (Orthoptera Tettigoniidae). J. Comp. Physiol. A 186, 821–831. doi: 10.1007/s003590000135
Gorb, S. N., Niederegger, S., Hayashi, C. Y., Summers, A. P., Vötsch, W., and Walther, P. (2006). Biomaterials: silk-like secretion from tarantula feet. Nature 443, 407. doi: 10.1038/443407a
Gottardo, M. (2011). A new genus and new species of Philippine stick insects (Insecta: Phasmatodea) and phylogenetic considerations. C. R. Biol. 334, 555–563. doi: 10.1016/j.crvi.2011.04.003
Gottardo, M., and Heller, P. (2012). An enigmatic new stick insect from the Philippine Islands (Insecta: Phasmatodea). C. R. Biol. 335, 594–601. doi: 10.1016/j.crvi.2012.07.004
Gottardo, M., and Vallotto, D. (2014). External macro- and micromorphology of the male of the stick insect Hermarchus leytensis (Insecta: Phasmatodea) with phylogenetic considerations. C. R. Biol. 337, 258–268. doi: 10.1016/j.crvi.2014.02.005
Gottardo, M., Vallotto, D., and Beutel, R. G. (2015). Giant stick insects reveal unique ontogenetic changes in biological attachment devices. Arthropod. Struct. Dev. 44, 195–199. doi: 10.1016/j.asd.2015.01.001
Grohmann, C., Henze, M. J., Nørgaard, T., and Gorb, S. N. (2015). Two functional types of attachment pads on a single foot in the Namibia bush cricket Acanthoproctus diadematus (Orthoptera: Tettigoniidae). Proc. R. Soc. Lond. B 282, 20142976. doi: 10.1098/rspb.2014.2976
Günther, K. (1953). Über die taxonomische Gliederung und die geographische Verbreitung der Insektenordnung der Phasmatodea. Beitr. Entomol. 3, 541–563.
Haas, F., and Gorb, S. (2004). Evolution of locomotory attachment pads in the Dermaptera (Insecta). Arthropod. Struct. Dev. 33, 45–66. doi: 10.1016/j.asd.2003.11.003
Hsiung, C. C. (2007). Revision of the genus Megacrania Kaup (Cheleutoptera: Phasmatidae). J. Orthoptera Res. 16, 207–221. doi: 10.1665/1082-6467(2007)16[207:ROTGMK]2.0.CO;2
Ishiwata, K., Sasaki, G., Ogawa, J., Miyata, D., and Su, Z.-H. (2011). Phylogenetic relationships among insect orders based on three nuclear protein-coding gene sequences. Mol. Phylogenet. Evol. 58, 169–180. doi: 10.1016/j.ympev.2010.11.001
Kearse, M., Moir, R., Wilson, A., Stones-Havas, S., Cheung, M., Sturrock, S., et al. (2012). Geneious basic: an integrated and extendable desktop software platform for the organization and analysis of sequence data. Bioinformatics 28, 1647–1649. doi: 10.1093/bioinformatics/bts199
Labonte, D., and Federle, W. (2013). Functionally different pads on the same foot allow control of attachment: stick insects have load-sensitive “heel” pads for friction and shear-sensitive “toe” pads for adhesion. PLoS ONE 8, e81943. doi: 10.1371/journal.pone.0081943
Labonte, D., Williams, J. A., and Federle, W. (2014). Surface contact and design of fibrillar 'friction pads' in stick insects (Carausius morosus): mechanisms for large friction coefficients and negligible adhesion. J. R. Soc. Interface 11, 20140034. doi: 10.1098/rsif.2014.0034
Letsch, H. O., and Simon, S. (2013). Insect phylogenomics: new insights on the relationships of lower neopteran orders (Polyneoptera). Sys. Ent. 38, 783–793. doi: 10.1111/syen.12028
Letsch, H. O., Meusemann, K., Wipfler, B., Schütte, K., Beutel, R., and Misof, B. (2012). Insect phylogenomics: results, problems and the impact of matrix composition. Proc. Roy. Soc. Lond. B 279, 3282–3290. doi: 10.1098/rspb.2012.0744
Lewis, P. O. (2001). A likelihood approach to estimating phylogeny from discrete morphological character data. Syst. Biol. 50, 913–925. doi: 10.1080/106351501753462876
Maddison, W. P., and Maddison, D. R. (2018). Mesquite: a Modular System for Evolutionary Analysis. Version 3.40. (Accessed April 12, 2018).
Misof, B., Liu, S., Meusemann, K., Peters, R. S., Donath, A., Mayer, C., et al. (2014). Phylogenomics resolves the timing and pattern of insect evolution. Science 346, 763–767. doi: 10.1126/science.1257570
Niederegger, S., Gorb, S., and Jiao, Y. (2002). Contact behaviour of tenent setae in attachment pads of the blowfly Calliphora vicina (Diptera, Calliphoridae). J. Comp. Physiol. A 187, 961–970. doi: 10.1007/s00359-001-0265-7
Perez Goodwyn, P., Peressadko, A., Schwarz, H., Kastner, V., and Gorb, S. (2006). Material structure, stiffness, and adhesion: why attachment pads of the grasshopper (Tettigonia viridissima) adhere more strongly than those of the locust (Locusta migratoria) (Insecta: Orthoptera). J. Comp. Physiol. A 192, 1233–1243. doi: 10.1007/s00359-006-0156-z
Ronquist, F., Teslenko, M., van der Mark, P., Ayres, D. L., Darling, A., Höhna, S., et al. (2012). MrBayes 3.2: efficient Bayesian phylogenetic inference and model choice across a large model space. Syst. Biol. 61, 539–542. doi: 10.1093/sysbio/sys029
Schmitt, M., Büscher, T. H., Gorb, S. N., and Rajabi, H. (2018). How does a slender tibia resist buckling? The effect of material, structural and geometric characteristics on the buckling behaviour of the hindleg tibia in the postembryonic development of the stick insect Carausius morosus. J. Exp. Biol. 221:jep.173047. doi: 10.1242/jeb.173047
Stork, N. E. (1980). Experimental analysis of adhesion of Chrysolina polita (Chrysomelidae: Coleoptera) on a variety of surfaces. J. Exp. Biol. 88, 91–108.
Vallotto, D., Bresseel, J., Heitzmann, T., and Gottardo, M. (2016). A black-and-red stick insect from the Philippines - observations on the external anatomy and natural history of a new species of Orthomeria. Zookeys 559, 35–57. doi: 10.3897/zookeys.559.6281
Varenberg, M., and Gorb, S. (2007). Shearing of fibrillar adhesive microstructure: friction and shear-related changes in pull-off force. J. R. Soc. Interface 4, 721–725. doi: 10.1098/rsif.2007.0222
Varenberg, M., and Gorb, S. N. (2009). Hexagonal surface micropattern for dry and wet friction. Adv. Mater. 21, 483–486. doi: 10.1002/adma.200802734
Keywords: euplantulae, tarsal attachment, adhesive microstructures, phylogeny, cuticle, functional morphology
Citation: Büscher TH, Buckley TR, Grohmann C, Gorb SN and Bradler S (2018) The Evolution of Tarsal Adhesive Microstructures in Stick and Leaf Insects (Phasmatodea). Front. Ecol. Evol. 6:69. doi: 10.3389/fevo.2018.00069
Received: 09 February 2018; Accepted: 04 May 2018;
Published: 24 May 2018.
Edited by:
Mariana Mateos, Texas A & M University, United StatesReviewed by:
Robert Friedman, University of South Carolina, United StatesBrian I. Crother, Southeastern Louisiana University, United States
Copyright © 2018 Büscher, Buckley, Grohmann, Gorb and Bradler. This is an open-access article distributed under the terms of the Creative Commons Attribution License (CC BY). The use, distribution or reproduction in other forums is permitted, provided the original author(s) and the copyright owner are credited and that the original publication in this journal is cited, in accordance with accepted academic practice. No use, distribution or reproduction is permitted which does not comply with these terms.
*Correspondence: Thies H. Büscher, dGJ1ZXNjaGVyQHpvb2xvZ2llLnVuaS1raWVsLmRlLg==