- 1Laboratory of Fundamental and Applied Research in Chemical Ecology, Institute of Biology, University of Neuchâtel, Neuchâtel, Switzerland
- 2Escuela Nacional de Estudios Superiores Unidad Morelia, Universidad Nacional Autónoma de México, Morelia, Mexico
- 3Campo Experimental Uruapan, Instituto Nacional de Investigaciones Forestales, Agrícolas y Pecuarias, Uruapan, Mexico
Many parasitic wasps use volatiles emitted by plants under herbivore attack to find their hosts. It has therefore been proposed that these inducible plant volatiles serve an indirect defense function by recruiting parasitoids and other natural enemies. This suggested function remains controversial because there is little evidence that, in terms of fitness, plants benefit from the actions of natural enemies, particularly parasitoids, which do not immediately kill their hosts. We aimed to address this controversy in a semi-natural field experiment in Mexico, where we used large screen tents to evaluate how parasitoids can affect plant performance. The tritrophic study system comprised teosinte (Zea spp.), the ancestor of maize, Spodoptera frugiperda Smith (Lepidoptera: Noctuidae) and Campoletis sonorensis Cameron (Hymenoptera: Ichneumonidae), which have a long evolutionary history together. In tents without parasitoids, S. frugiperda larvae inflicted severe damage to the plants, whereas in the presence of parasitoid wasps, leaf damage was reduced by as much as 80%. Parasitoids also mitigated herbivore-mediated mortality among young teosinte plants. Although these findings will not resolve the long-standing debate on the adaptive function of herbivore-induced plant volatiles (HIPVs), they do present strong support for the hypothesis that plants can benefit from the presence of parasitoid natural enemies of their herbivores.
Introduction
Plant defense traits are commonly recognized as important determinants of the tremendous success and diversity of plants in natural ecosystems. Insects are important evolutionary drivers of these defenses, selecting for numerous chemical and physical traits that plants use to defend themselves against herbivory (Howe and Jander, 2008). Several defense responses are specifically induced by herbivore attack (Karban and Balwin, 1997). In addition to attributes such as leaf toughness, toxic compounds and digestibility reducers, which have direct negative effects on the herbivores, plants possess traits that have been interpreted as indirect defenses, because they act through the recruitment of natural enemies of herbivores (Dicke and Sabelis, 1988; Turlings et al., 1995; Heil, 2008).
The current study addresses a well-known phenomenon that has been interpreted as an indirect plant defense: the attraction of natural enemies to insect-damaged plants through the emission of volatile attractants (Turlings and Fritzsche, 1999; Heil, 2008). It remains unclear, however, whether these volatile attractants confer fitness benefits to the plants, and the specific function of herbivore-induced plant volatiles (HIPVs) is still hotly debated in the literature (Allison and Hare, 2009; Dicke and Baldwin, 2010; Agrawal, 2011; Hare, 2011; Kessler and Heil, 2011; Steiger et al., 2011; Kaplan, 2012; Heil, 2014; Poelman, 2015). Yet, it is evident that HIPVs are attractive to parasitoids and predators. The best studied examples of HIPV induction involve spider mites (Dicke and Sabelis, 1988; Takabayashi et al., 1994; De Boer and Dicke, 2004), and caterpillars (Turlings et al., 1990; Geervliet et al., 1996; De Moraes et al., 1998; Turlings and Fritzsche, 1999; Kessler and Baldwin, 2001). In the case of caterpillars, several elicitors contained in their oral secretions have been found to trigger or greatly enhance the emission of HIPVs (Alborn et al., 1997; Schmelz et al., 2006). These HIPVs provide natural enemies with potentially reliable cues, which for instance allow Cardiochiles nigriceps Viereck (Hymenoptera: Braconidae), a highly specialized parasitoid, to distinguish plants attacked by hosts from plants attacked by non-hosts (De Moraes et al., 1998). Despite general agreement that HIPVs are used by predators and parasitoids to locate hosts, it remains to be demonstrated that, from the plant's perspective, this interaction is adaptive.
Predators have the potential to significantly reduce plant damage, because they normally kill herbivorous prey immediately. HIPVs are effective in attracting predators in the field (Bernasconi Ockroy et al., 2001) and this may increase predation of insect herbivores (Kessler and Baldwin, 2001). Indeed, several field studies have shown that predators can reduce plant damage, resulting, in some cases, in positive plant fitness effects (Clark et al., 1994; Moran et al., 1996; Bartlett, 2008). In the context of plant volatile-mediated predator attraction, we are only aware of one study that specifically focuses on plant performance. In this study, the attraction of Geocoris spp. predators (Hemiptera: Geocoridae) to HIPVs was shown to positively affect the flower production of wild tobacco, which is likely to increase seed production (Schuman et al., 2012).
Herbivore-induced plant volatiles are also effective in attracting parasitoids in the field (Bernasconi Ockroy et al., 2001), which may increase parasitism of insect herbivores (Khan et al., 1997; De Moraes et al., 1998; Thaler, 1999). However, the effects of parasitic wasps on plant performance are not as straightforward as for predators. Parasitoids ultimately kill their hosts, but the effect on herbivore damage is often delayed. While parasitism by solitary parasitoids usually leads to a significant decrease in feeding by the host (Hoballah et al., 2004), certain gregarious parasitoids may stimulate growth of the host, possibly leading to more plant damage (Coleman et al., 1999; Harvey, 2005), but this probably does not affect plant reproduction (Van Loon et al., 2000). Current evidence for plant fitness benefits mediated by parasitoid wasps is limited to a few studies. One is a parasitoid exclusion experiment with seed-weevils, where parasitoid activity was shown to enhance seed production (Gomez and Zamora, 1994). A study on yucca plant-yucca moth-parasitoid interactions yielded opposite plant fitness effects for two subsequent study years. This is explained by the fact that yucca moth caterpillars damage the plants, but the adults serve as pollinators (Crabb and Pellmyr, 2006). A study in Citrus revealed that adding parasitoids to enclosures containing plants and scale insects reduced herbivore numbers and increased plant biomass (Matsumoto et al., 2003). A study in charlock mustard revealed that parasitism of Pieris brassicae L. (Lepidoptera: Pieridae) caterpillars, which feed on both leaves and flowers, may have positive fitness consequences for the plant (Gols et al., 2015); the parasitoid used in this study is attracted to volatiles from caterpillar-infested plants (Steinberg et al., 1992; Geervliet et al., 1996; Desurmont et al., 2015). Tooker and Hanks (2006) studied the impact of a gall wasp on growth and reproduction of the prairie perennial Silphium laciniatum L. and found that the presence of a parasitoid had a positive effect on seed size. The females of this parasitoid are known to be attracted to the volatiles emitted by galled plants (Tooker and Hanks, 2006). A few other studies reported similar findings, but these involved plants and insects that did not coevolve together and/or were conducted under relatively artificial conditions (Van Loon et al., 2000; Fritzsche Hoballah and Turlings, 2001; Smallegange et al., 2008).
As is evident from multiple recent critical reviews (Hare, 2011; Kaplan, 2012; Heil, 2014; Poelman, 2015), none of the above studies has been fully accepted as providing conclusive evidence for fitness benefits conferred to plants by parasitoids under realistic field conditions. Here we present a study conducted to seek such evidence for a tropical tritrophic system involving teosinte, the wild ancestor of maize, and herbivorous and parasitoid insects that have interacted with this plant over evolutionary time.
Maize is one of the most studied plants when it comes to HIPV emissions, particularly in the context of parasitoid attraction (Turlings et al., 1990; Turlings and Fritzsche, 1999). Maize responds strongly to herbivory, and caterpillar-damaged plants produce large quantities of a blend of predominantly green leaf volatiles, terpenoids and aromatic compounds (Turlings et al., 1995, 1998; Degen et al., 2004). The blend is highly attractive to various parasitoids (Tamò et al., 2006), although it remains unclear which of the compounds in the blend are of key importance for the attraction (D'Alessandro and Turlings, 2006). The wild ancestors of maize are teosintes, a small species complex of Zea grasses found in Mexico, Guatemala and Nicaragua (Matsuoka et al., 2002). Although there are substantial differences, various species and sub-species of teosinte release a very similar bouquet of volatiles as has been reported for maize (Gouinguené et al., 2001; De Lange et al., 2016). Artificial selection of crop plants, including maize (Rosenthal and Dirzo, 1997; Dávila-Flores et al., 2013; De Lange et al., 2016; Gaillard et al., 2018), for increased yield and improved quality can negatively influence other important traits, such as resistance to herbivores (Chen et al., 2015; Whitehead et al., 2017). Wild teosinte species and the insects associated with them are therefore ideal for a study that aims to test if a plant derives fitness benefits from attracting parasitoids.
Here, we studied teosintes in relation to the herbivore Spodoptera frugiperda Smith (fall armyworm) (Lepidoptera: Noctuidae) and its natural enemy Campoletis sonorensis Cameron (Hymenoptera: Ichneumonidae) (Figure S1). Larvae of the moth S. frugiperda are polyphagous but particularly well-adapted to feed on grasses, constituting one of the most devastating maize pests in North-America. S. frugiperda larvae are also reported to feed on teosintes (Luginbill, 1928; De Lange et al., 2014). C. sonorensis is one of the main natural enemies of S. frugiperda, and frequently parasitizes S. frugiperda in agricultural areas in Mexico (Hoballah et al., 2004; Jourdie et al., 2008; Bahena-Juárez et al., 2010; Von Mérey et al., 2012). This solitary koinobiont endoparasitoid wasp attacks the early caterpillar instars, which then feed and grow much less than unparasitized caterpillars. The parasitoid larvae emerge long before their host has reached full size potential, and the host dies at this point (Turlings and Fritzsche, 1999).
Teosinte, S. frugiperda and C. sonorensis share a long evolutionary history, and it is well- established that C. sonorensis and other parasitoid species exploit Spodoptera-induced plant volatiles in their search for hosts (Tamò et al., 2006; De Lange et al., 2016). For the current study, we first conducted a survey of different teosinte populations in Mexico to confirm this association and found both herbivore and parasitoid on teosinte plants (described in detail in File S1, Table S1, Figure S2). Seeds from three teosinte populations, Tzitzio (Zea mays L. ssp. parviglumis Iltis & Doebley), Cuitzeo (Z. mays ssp. mexicana (Schrader) Iltis), and Hidalgo (Z. mays ssp. mexicana), were used to grow plants in large screen tents. Previous studies identified differences in volatile emission between the different teosinte subspecies (Gouinguené et al., 2001; De Lange et al., 2016). The young (6–7-week-old) teosinte plants in each tent were exposed to realistic levels of S. frugiperda infestation, and different parasitoid densities in the tents were used as a proxy for differential parasitoid attraction. Tents without herbivores and tents with herbivores but without parasitoids served as controls. Plants in similar plots, but without tent coverage, served to assess recruitment of naturally present parasitoids. Our results show that the presence of parasitoid wasps in tents reduces plant damage. This may be a matter of life or death for young teosinte plants, as the presence of parasitoids also reduced plant mortality. The different teosinte varieties displayed different volatile signatures, but this did not translate into differential parasitoid attractiveness or mortality in plots without tent coverage. Overall, the outcome of this study supports the hypothesis that plants can benefit from the presence of parasitoid natural enemies of their herbivores.
Materials and Methods
Plants
Seeds of annual teosintes were obtained from three different locations in the state of Michoacán, Mexico: near Tzitzio (Z. mays ssp. parviglumis; N 19°34.310′, W 100°55.358′; altitude 1,354 m), near Cuitzeo (Z. mays ssp. mexicana; N 19°59.742′, W 100°53.397′; altitude 2,096 m) and near Ciudad Hidalgo (Z. mays ssp. mexicana; N 19°41.696′, W 100°37.544′; altitude 2,086 m). The seeds were collected from private land with permission from the landowners. Our studies did not involve endangered or protected species. We used three different teosintes to assess whether they differed in volatile emissions that might affect parasitoid recruitment. Z. mays ssp. parviglumis is considered the wild ancestor of modern maize (Matsuoka et al., 2002). This species occurs in the Balsas river valley in southern and western Mexico, where the mean annual temperature is between 20 and 25°C and annual rainfall ranges from 125 to 200 cm. It grows at an altitude between 400 and 1700 m. Closely related Z. mays ssp. mexicana grows on the plains and valleys of central and northern Mexico at altitudes of 1800 to 2500 m. Here, mean annual temperature varies from 15 to 20°C and rainfall ranges from about 50 to 100 cm per year (Sanchez Gonzalez and Ruiz Corral, 1995). Previous studies identified differences in volatile emission between these plants (Gouinguené et al., 2001; De Lange et al., 2016).
Teosinte seeds were sown in biodegradable pots filled with a mixture of soil and vermiculite in three batches on July 12, July 28, and August 19, 2010. All seedlings were kept in a greenhouse for 10–20 days before being planted in a field on the UNAM (Universidad Nacional Autónoma de México) campus in Morelia, Michoacán, Mexico (N 19°38.960′, W 101°13.636′; altitude 1966 m). As the field experiment was replicated three times, the field was divided into three parcels. Each parcel was divided into eight plots with at least 60 cm between plots. Within each parcel, at five randomly chosen positions, a tent was placed (12′ × 12′ Dual Identity Screenhouse and Canopy, Gigatent, Wayne, NJ, USA) to enclose the plants with specifically chosen insect treatments and to exclude other insects (Figure 1A). The sides of the tents were burrowed 15 cm into the soil to stabilize them against strong winds and rain. In addition, two plots (open plots) remained without a tent. Hence, there were six (replicate 1) or seven (replicates 2 and 3) plot treatments per parcel (Figure 1A), which are described below. In each plot, plants were arranged in nine mini-plots of six teosinte plants each, with the different populations randomly distributed in a three-by-three Latin square design (i.e., 54 plants per tent). Plants were spaced at least 25 cm apart within a mini-plot with 50 cm between mini-plots (Figure 1B). Plants were only irrigated at the end of the rainy season, in September (Figure S3). Weeds were regularly removed by hand. Prior to insect treatments (see below), the numbers of plants present and absent in each plot were scored. The very few plants that failed to establish after transplantation were excluded from the analyses.
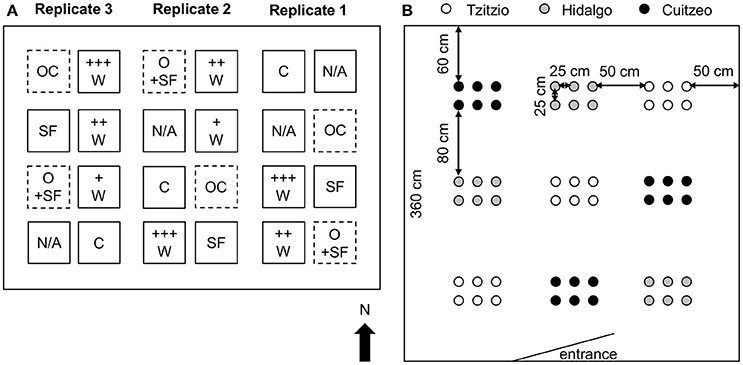
Figure 1. Field design. (A) Layout for the three replicate parcels. Teosinte plants were placed in 360 × 360 cm plots covered by a tent (C, SF, +W, ++W, +++W) or in open plots not covered by a tent (OC, O+SF). The labels refer to experimental treatments: tents not infested with herbivores (treatment C), tents infested with Spodoptera frugiperda larvae in the absence (SF) and in the presence of increasing densities of Campoletis sonorensis parasitoid wasps (+W, ++W, +++W), and natural parasitism of S. frugiperda larvae in plots not covered by a tent (OC, O+SF). N/A: Not applicable (these plots were covered by a tent, but involved a separate experiment). The arrow points north. The drawing is not on scale. (B) Layout within each plot. Plants from three different teosinte populations, Tzitzio (Zea mays ssp. parviglumis), Hidalgo (Z. mays ssp. mexicana), and Cuitzeo (Z. mays ssp. mexicana), were placed in a tent in nine mini-plots in a randomized three-by-three Latin square, with six plants per mini-plot. Plants were placed in an identical arrangement in the open plots that were not covered by a tent.
Insect Rearing
Rearing colonies of S. frugiperda originated from insect collections in various maize fields in the state of Michoacán, Mexico, in the summer of 2010 (Bahena-Juárez et al., 2010). The larvae were kept at room temperature and were reared on fresh castor bean (Ricinus communis L.) leaves, as this diet tends to reduce cannibalism (Cave, 2000; Valicente et al., 2013; Martínez-Martínez et al., 2015). Pupae were sexed and adults were kept in mating boxes at a 50:50 sex ratio, with cotton wool soaked in honey-water solution as a food source. Females were allowed to oviposit on paper lining the walls of the boxes. The rearing colony of the solitary koinobiont endoparasitoid C. sonorensis originated from wasps that emerged from S. frugiperda larvae that were collected in the summer of 2010. To maintain the colony, 20 second-instar S. frugiperda caterpillars were offered to two mated female wasps for 5 h in a small plastic box. The parasitized caterpillars were kept on castor bean leaves until the parasitoid larvae emerged and formed cocoons, which were kept in petri dishes until adult emergence. The adult wasps were sexed and housed in cages (30 × 30 × 30 cm) with a sex ratio of 25:75 (male: female), with cotton wool soaked in honey-water solution as a food source. For experiments, second-instar S. frugiperda larvae and 2–7-day-old mated female wasps were used. The females were naïve, which means that as adults they had never been in contact with a plant or a host before.
Treatments
Five different treatments were applied to the plants in the tents: C - control, no insects; SF - infestation with five second-instar S. frugiperda larvae per plant, but no release of parasitoids; and three treatments (+W, ++W and +++W) that also involved infestation with five second-instar S. frugiperda larvae per plant, but in these cases, we added female C. sonorensis wasps at different densities, of 3, 9, or 54 wasps per tent, respectively. The lowest wasp density treatment (+W) was absent from the first replicate and was added to the next two replicates because the medium wasp density treatment (++W) resulted in an unexpectedly high parasitism rate. We employed different amounts of parasitoids to mimic plant differential recruitment strength. Two plots without tents (open plots) with the same numbers of plants were included to assess actual recruitment of naturally present parasitoids. One of the open plots received no insects (OC) and the other received five second-instar S. frugiperda larvae per plant (O+SF) (Figure 1).
Infestation with S. frugiperda larvae took place on August 23, September 6 and October 6, 2010, for replicate one, two, and three, respectively. Five larvae were placed in the whorl of each plant with a fine brush. S. frugiperda moths readily lay batches that may contain hundreds of eggs. Of the larvae that hatch from these eggs, only few survive the first stages, with cannibalism being an important mortality factor (Luginbill, 1928; Chapman et al., 1999). Five second-instar larvae per plant are within the range that is naturally observed on teosinte (File S1, Figure S2) and maize plants (Von Mérey et al., 2011). Under controlled laboratory conditions, a single C. sonorensis female can lay eggs in more than 40 larvae per 24 h (Isenhour, 1985). In the field, parasitism of S. frugiperda on maize plants is highly variable among regions and seasons (Jourdie et al., 2008; Bahena-Juárez et al., 2010; Von Mérey et al., 2012). We considered the presence of 90, 30, and 5 hosts per wasp an estimate of low, intermediate and high plant attractiveness to parasitoids, respectively.
Parasitoid wasps were released in three equally sized batches on days 1, 2, and 3 following larval infestation (i.e., 1, 3, and 18 wasps were released on each of three consecutive days for the low, medium and high wasp density treatments, respectively). Inside each tent, cups with cotton wool soaked in honey-water solution were provided as a food source for the wasps.
Volatile Collection and Analysis
We collected volatiles from all three teosinte populations to potentially explain differences in parasitoid recruitment. Plant volatiles were collected as described previously (Von Mérey et al., 2012). Briefly, plants were enveloped in Nalophan sleeves (Omya AG, Oftringen, Switzerland) of 150 mm diameter and 50 cm length and the top and bottom of the sleeves were closed with a plastic seal. The sleeves were attached to a metal wire to prevent the plants from falling over. Tubular glass parts (23 × 17 × 12 mm) with an open screw cap were attached to the bag to insert a trapping filter containing 25 mg of 80–100 mesh SuperQ adsorbent (Alltech Associates, Inc., Deerfield, IL, USA). Air was pulled through the trapping filters using an air-sampling pump (SKC 222 series, Blanc Labo S.A., Lonay, Switzerland) for 6 h at 0.6 L/min. The volatiles were eluted from the filters with 150 μl dichloromethane and the extracts were stored at −20°C before analysis. For each replicate, we simultaneously collected volatiles from all three teosinte varieties, in tents with plants infested with S. frugiperda larvae without parasitoids (treatment SF). In most maize varieties, volatile emission is herbivore-inducible, and non-induced plants emit hardly any compounds (Turlings et al., 1995; Degen et al., 2004). Collections started one day after larval infestations and were repeated on 4, 5, or 6 consecutive days, using different plants [n = 14 (Tzitzio), 15 (Hidalgo), and 16 (Cuitzeo)].
Volatiles were analyzed using a gas chromatograph (Agilent 6890 Series GC System G1530A) coupled to a mass spectrometer (Agilent 5973 Network Mass Selective Detector; transfer line 280°C, source 230°C, ionization potential 70 eV). An aliquot of 2 μl was injected in split mode (65.8:1) onto an apolar capillary column (HP-Ultra 2 ms, 30 m, 0.25 mm ID, 0.25 μm film thickness; Agilent J&W Scientific, USA). Helium at constant flow (1.1 ml/min) was used as the carrier gas. The column temperature was maintained at 40°C and increased after injection at 8°C/min to 150°C followed by a post-run of 2 min at 300°C. The detected volatiles were identified by comparing their: I) mass spectra with those of the NIST05 library, II) mass spectra and retention times with those of authentic standards, and III) retention times with those from previous analyses (D'Alessandro and Turlings, 2005). Volatiles that met only one of those criteria are labeled as tentatively identified. Comparisons of volatile quantities between the three teosinte populations were based on peak areas. These data were corrected for the volume of air that was pulled through the trapping filters. We subtracted compounds that were also present in blank collections (volatile collections that were performed in a similar way as described above, but without a plant, n = 3).
Parasitism
Seven days after the larval infestations, the shoot of every second plant in each plot was harvested, placed in a paper bag, and transported to the nearby laboratory to evaluate insect presence and herbivory (n = 52–80). Nine to ten days later, the tents were removed to allow the remaining plants to continue to develop under natural conditions. The wasps were expected to disperse at this point, and naturally present herbivores and natural enemies could reach the plants. We argue that young plants are most vulnerable to herbivory and would benefit most from parasitoid presence, so we standardized parasitoid presence (through the use of tents) only for young plants.
In the laboratory, right after harvest, the harvested plants were carefully removed from the paper bags and the number of caterpillars present on each plant was counted. Individual larvae were weighed (an average was calculated per plant) and subsequently placed in individual compartments of a 24-well ELISA plate. Larvae were supplied with artificial maize-based diet and reared at room temperature until adult emergence or, in the case of parasitism, until parasitoid emergence occurred (Hoballah et al., 2004). The adult parasitoids, dead parasitoid larvae and cocoons were individually preserved in 70% ethanol in Eppendorf tubes. The wasps were visually examined to identify the species (Cave, 1995). We calculated the parasitism rate by dividing the number of parasitized larvae by the number of collected larvae (total number of collected larvae minus the number of larvae that escaped or died for unknown reasons). Since we did not obtain parasitoid larvae or cocoons at the time of harvest, we assumed that the parasitoids had not yet emerged from their hosts at this point.
Herbivory and Shoot Dry Biomass
After removing the larvae, the leaves of the harvested plants were scanned and herbivore damage was assessed using NIH ImageJ 1.44l software (Rasband, 1997–2012). A minimal amount of plant damage was observed in control tents, where herbivores were excluded (treatment C), mostly due to occasional grasshoppers in the tents. Tents were inspected each morning and grasshoppers were quickly removed so that extensive damage by herbivores other than the experimentally released individuals was prevented. Herbivory was only analyzed for plants in tents; damage levels on plants in plots without tents, subjected to herbivory from unknown species of herbivores, were considered irrelevant for the scope of this study. After scanning, plant shoots were dried in an oven at 80°C for 2 days and their dry weight was recorded.
Plant Survival
Two weeks after removing the last tents, the numbers of plants present and absent in each plot were scored as a measure of plant survival (corrected for the plants that were harvested according to experimental protocols). Individual plants were scored as 0 (dead) or 1 (alive) (n = 51–80). On November 8, 2010, all plants died during an early freezing event (Figure S3). Due to this frost event before plant maturity, we could not fully assess the true consequences of the treatments for plant fitness.
Statistical Analysis
Data were recorded for each individual plant. Outcomes for most recorded parameters were analyzed adjusting for teosinte population and/or replicate, as appropriate. For the +W treatment, there were only two replicates, while for all other treatments, there were three replicates. Comparisons between treatments were targeted, i.e., we compared treatments based on our hypotheses rather than comparing all treatments for all measured parameters.
Data for larval weight, parasitism rate, plant damage, and shoot dry weight were analyzed using a generalized linear model (GLM) based on a Gaussian distribution, with variety and replicate as adjustments. For larval weight and parasitism rate, data from both the OC and the O+SF treatment were combined as one value for open plots. Data for larval retrieval were analyzed using a GLM based on a Poisson distribution, with variety and replicate as adjustments. Data for plant mortality were analyzed using a GLM based on a binomial distribution, with variety and replicate as adjustments. The models' adequacy was assessed through likelihood ratio statistics and examination of residuals.
Volatiles were classified based on biosynthetic origin [green leaf volatiles (GLVs), acyclic hydrocarbons, alcohols (other than GLVs), esters (other than GLVs), aldehydes, monoterpenes, homoterpenes, sesquiterpenes, aromatics, and unidentified compounds]. They were then tested for normality and homogeneity of variance, and analyzed by groups using multivariate analysis of variance (MANOVA). Only groups consisting of two or more compounds were included in the analysis. A significant effect was followed by GLM based on a Gaussian distribution, with replicate as adjustment. Total volatile emissions were also analyzed using a GLM based on a Gaussian distribution, with replicate as adjustment. To visualize potential differences between the volatile patterns of the different plants, the total volatile profiles were analyzed using principal component analysis (PCA).
To evaluate the strength of correlations between different parameters, we averaged values per miniplot and calculated the coefficient of determination (R2). The data were fitted with a GLM to calculate the significance of the correlation. Statistical analyses were performed using Minitab Version 18 (http://www.minitab.com), SigmaPlot Version 13.0 (https://systatsoftware.com/), and R Version 3.3.1 (R Core Team, 2016).
Results
Survival and Parasitism of Caterpillars
To test whether the presence of parasitoid wasps influenced larval survival, we compared larval recovery in tents in the presence and absence of wasps. Overall, 1 week after larval infestations, we recovered 39 ± 4.0% (average ± SE) of all released larvae in the absence of wasps, while we recovered only 19 ± 2.0% of all released larvae in the presence of wasps. Significantly more larvae were retrieved from tents in the presence of herbivores only than from tents in the presence of the two highest wasp quantities (GLM, χ2 = 122.6 df = 7, 274, P < 0.001; Figure S4A). More larvae were retrieved from teosinte varieties Hidalgo and Cuitzeo than from variety Tzitzio. More larvae were retrieved in the first replicate than in replicates two and three. Overall, these results confirm that parasitoids contribute to early, premature larval mortality. The weight of individual larvae was lower in the tents where wasps were present than in the tents where wasps were absent (Figure S4B), indicating that in the presence of wasps, the larvae were parasitized and/or suffered from non-consumptive disturbances by the parasitoids (GLM, F = 30.707, df = 7, 153, P < 0.001). Larval weight was higher in the first replicate than in replicates two and three. There were no significant differences in larval weight between teosinte varieties. When the recovered larvae were reared on artificial diet, larvae from tents without wasps developed into healthy adult moths, indicating that no naturally present parasitoid wasps had entered the tents. Most of the larvae recovered from tents with wasps were found to be parasitized. In the presence of the two highest numbers of wasps, parasitism rate was significantly higher than in the presence of the low number of wasps (GLM, F = 5.3463, df = 6, 67, P < 0.001). The +W treatment probably shows a higher standard error than the ++W and +++W treatment because the sample size was lower (Figure 2). There were no significant differences between the three teosinte varieties, and in replicate 3 parasitism rates were lower than in replicates 1 and 2. Note that all treatments were replicated three times, except for the +W treatment, which was absent from the first replicate (absolute no. of parasitized larvae: +W = 14, ++W = 39, +++W = 38, OC = 2, O+SF = 9).
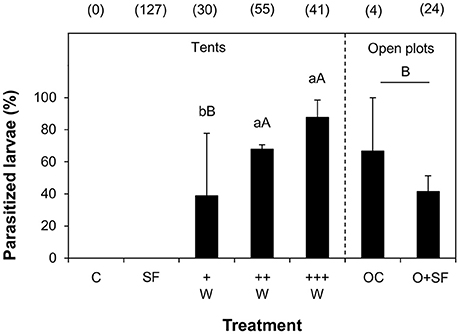
Figure 2. Parasitism of Spodoptera frugiperda larvae. Average percentage of parasitized larvae (+SE) on teosinte plants. The larvae came from tents that were not infested with herbivores (treatment C) or that were infested with larvae in the absence (SF) or in the presence of different numbers of Campoletis sonorensis parasitoid wasps (+W, ++W, +++W), or from uncovered plots (OC, O+SF). The total number of larvae retrieved from each plot is given in brackets (total number of collected larvae minus the number of larvae that escaped or died for unknown reasons). Different letters indicate statistically significant differences (GLM, P < 0.05). Lowercase letters represent significant differences between plots in tents in which different wasp numbers were released. Uppercase letters represent significant differences between all treatments where wasps were present; both open plots were combined as a single “open plot” treatment for statistical analysis.
To address actual recruitment, we assessed the parasitism rate of larvae in the three teosinte populations in plots without tents. In open plots that were infested with larvae, we recovered significantly fewer larvae per plant than in tent-covered plots in the absence and presence of wasps (GLM, χ2 = 148.66, df = 9, 345, P < 0.001) (Figure S4A). In the two open plots, individual larval weight was highly variable, but overall lower than larval weight in tent-covered plots in the absence of wasps (GLM, F = 30.269, df = 8, 187, P < 0.001; Figure S4B). These results indicate that the larvae in open plots suffered the effects of natural enemies and/or adverse environmental factors. Also, there may have been natural infestation with caterpillars of variable age. Parasitism rates in the two open plots were low; over all three replicates, a total of 11 parasitized larvae were found. Although parasitism was lower in open plots exposed to naturally present parasitoids than in plots in tents in the presence of medium and high number of wasps, there were no significant differences in parasitism between plots in tents in the presence of the lowest number of wasps and open plots, (GLM, F = 7.968, df = 7, 91, P < 0.001), indicating that parasitism rates in these tents more or less mimicked natural parasitism rates (Figure 2). C. sonorensis accounted for 55% of the parasitized larvae retrieved from the open plots; for the remaining parasitized larvae, the responsible species was not identified.
Herbivory and Shoot Dry Biomass
In the absence of wasps, larvae consumed significantly more leaf area than in the presence of wasps, indicating that parasitism and/or non-consumptive disturbances by the parasitoids reduced larval feeding (GLM, F = 24.581, df = 7, 273, P < 0.001; Figure 3). Damage levels were lowest in the presence of the highest wasp quantity. No significant differences were found among the teosinte populations. There was a significant effect of replicate; damage levels were highest in the first replicate. There was a significant positive correlation between the total larval biomass per plant and the amount of leaf damage (R2 = 0.88, P < 0.001, Figure S5). Overall, these results imply that parasitoid wasps can significantly reduce herbivory.
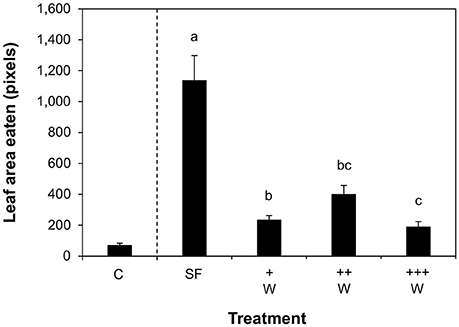
Figure 3. Damage inflicted by Spodoptera frugiperda larvae. Average amount of damage inflicted (+SE) on teosinte plants in tents that were not infested with herbivores (treatment C) or that were infested with larvae in the absence (SF) and in the presence of increasing densities of Campoletis sonorensis parasitoid wasps (+W, ++W, +++W). Different letters indicate statistically significant differences (GLM, P < 0.05). Only plots where larvae were released were included in the statistical analyses.
For tent-covered plots, plant biomass (shoot dry weight) was higher in plots exposed to herbivores in the absence of wasps (treatment SF) than in plots exposed to the medium wasp density (++W) and control, no-insect conditions (C) (GLM, F = 11.955, df = 8, 352, P < 0.001; Figure S6), indicating compensatory growth (Agrawal, 2000) under the maximum experimental herbivore pressure. Indeed, a recent study with wild lima bean plants confirmed that parasitoids can attenuate plant responses that lead to compensatory growth (Cuny et al., 2018). There were significant differences among teosinte populations, with Hidalgo having the highest biomass and Tzitzio the lowest. There were also biomass differences between replicates, with plant biomass being significantly higher in the first replicate than in the second and third replicate. There was a significant positive correlation between herbivory and plant biomass (R2 = 0.52, P < 0.001, Figure S7), as well as between larval retrieval and plant biomass (R2 = 0.53, P < 0.001, Figure S8). This is again best explained as a consequence of compensatory growth.
For open plots, there were no significant differences in shoot dry weight between treatments (GLM, F = 9.9939, df = 5, 144, P < 0.001; Figure S6). There was also no significant correlation between larval retrieval and plant biomass (R2 = 0.16, P = 0.249), which may be explained by the low numbers of larvae that were retrieved from the open plots.
Volatile Emission
To compare volatile emission for the different teosinte populations, we collected volatiles from individual herbivore-infested plants. Total volatile emissions differed significantly between different populations [Total volatiles (average ± SE) Tzitzio: 53,780 ± 14,477.5a; Hidalgo: 16,188 ± 3,313.8b; Cuitzeo: 11,317 ± 2,984.4b, where the unit is peak area (the sum of total ion counts of all integrated peaks), and different letters indicate statistically significant differences (GLM, F = 10.180, df = 4, 40, P < 0.001)]. Also, there were significant differences in volatile profiles (Table 1, Table S2, Figure S9), which are reflected in the PCA. The first two principal components explained 32% of the total variance (Figure 4). The observed compounds are typical for herbivore-induced maize and teosintes (Gouinguené et al., 2001; De Lange et al., 2016). We observed emission of green leaf volatiles, such as (Z)-3-hexenol and (Z)-3-hexenyl acetate, that are frequently associated with parasitoid attraction (Turlings and Erb, 2018), and maize mutants that do not emit GLVs display dramatic reductions in attractiveness to parasitoids (Christensen et al., 2013). As expected, the teosintes also released various terpenoids, such as linalool, 4,8-dimethyl-1,3,7-nonatriene, (E)-β-caryophyllene and (E)-β-farnesene, that have also been linked to the attraction of parasitoids (Schnee et al., 2006; Köllner et al., 2008; Fontana et al., 2011). There was a significant positive correlation between leaf area eaten and total volatile emission (R2 = 0.47, P = 0.019, Figure S10). Indeed, the more damage that caterpillars inflict, the more volatiles are emitted, and the more maize is attractive to parasitoids (Turlings et al., 2004).
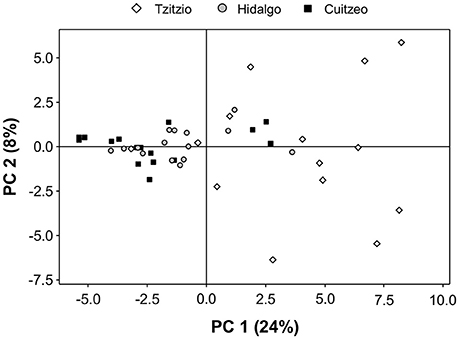
Figure 4. Principal component analysis (PCA) of herbivore-induced plant volatile emissions. Plants from three different teosinte populations were compared: Tzitzio (Zea mays ssp. parviglumis), Hidalgo (Z. mays ssp. mexicana), and Cuitzeo (Z. mays ssp. mexicana). Volatiles were collected from plants that were infested with Spodoptera frugiperda larvae in the absence of parasitoid wasps (treatment SF). The PCA shows the first and second principal components (PC) with the explained variance in brackets.
Plant Survival
After harvest, the tents were removed and the remaining plants were allowed to continue their development under natural conditions. Two weeks after removing the last tents, we monitored plant survival in each plot. There was a significant difference in plant mortality between treatments in plots previously covered with tents (GLM, χ2 = 66.589, df = 8, 353, P < 0.001). Plant mortality was 28 ± 5.3% (average ± SE) in the presence of only herbivores, while mortality was an overall 3 ± 1.0% in the other plots (Figure 5). It should be noted that mortality was considerably more severe in replicate 2 than in replicates 1 and 3. There was no significant difference in mortality between different teosinte varieties. Hence, a positive effect of parasitoid presence on plant survival was observed.
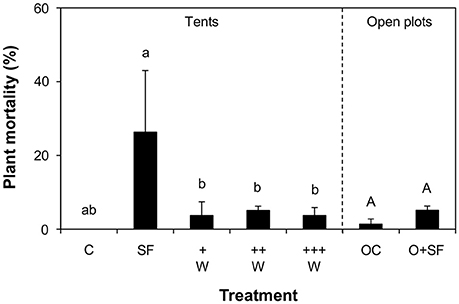
Figure 5. Plant mortality. Shown is the average number of teosinte plants that died before the end of the experiment (+SE) in plots that were initially covered with tents that were not infested with herbivores (treatment C) or that were infested with larvae in the absence (SF) and in the presence of increasing densities of Campoletis sonorensis parasitoid wasps (+W, ++W, +++W), and in open plots, never covered by a tent (OC, O+SF). Different letters indicate statistically significant differences (GLM, P < 0.05). Lowercase letters represent significant differences between plots initially covered with tents. Uppercase letters represent significant differences between open plots.
Mortality was 3 ± 1.4% in plots that were never covered with tents, and there were no significant differences between the two open plot treatments (GLM, χ2 = 3.0831, df = 5, 149, P = 0.6872; Figure 5).
Plant mortality did not correlate with total volatile emission by plants infested with non-parasitized S. frugiperda larvae (R2 = 0.11, P = 0.601), herbivory (R2 = −0.12, P = 0.191), initial plant biomass (tents: R2 = 0.12, P = 0.173; open plots: R2 = −0.01, P = 0.969), or parasitism rate (tents in which parasitoids were released: R2 = −0.16, P = 0.277; open plots: R2 = −0.12, P = 0.642).
Discussion
Whether or not plants benefit from attracting natural enemies has been heavily debated and is the topic of several reviews (Hare, 2011; Kaplan, 2012; Heil, 2014; Poelman, 2015). These reviews emphasize that, currently, there is only marginal evidence that attracting natural enemies has any consequences for plant fitness. Yet, a study with transformed tobacco plants has convincingly shown that plants produce fewer flowers when they are unable to recruit predatory bugs (Schuman et al., 2012). Also, a recent study in Brassica nigra L. showed that the exclusion of natural enemies, both predators and parasitoids, reduced plant reproductive output in the presence of insect herbivores (Lucas-Barbosa et al., 2017). The present study addresses the benefits to plants of attracting parasitic wasps, which do not immediately kill their hosts. These wasps rely heavily on HIPVs for host location (Turlings et al., 1990; Geervliet et al., 1996; De Moraes et al., 1998; Tamò et al., 2006). Using a tritrophic system with organisms that have a longstanding evolutionary history together, we mimicked plants with differential attractiveness to parasitoids under realistic field conditions and found a beneficial effect of parasitism on plant damage and survival (Figures 3, 5).
In the tent experiments, we observed that the presence of parasitic wasps led to significant parasitism of S. frugiperda (Figure 2), and increasing the number of wasps resulted in higher parasitism rates. Even at the lowest wasp density (i.e., three) most larvae were parasitized (Figure 2). These results imply that the wasps very efficiently located and parasitized their hosts. Most of the larvae retrieved from the plants were in their second- and third-instar; indeed C. sonorensis prefers to lay eggs in second- and third-instar S. frugiperda larvae (Isenhour, 1985; Cave, 1995). Larval retrieval rates were lower in the presence of wasps than in the absence of wasps (Figure S4A). Since we can assume that the parasitoids had not yet emerged from their hosts at this point, reduced retrieval rates may be due to larval mortality after excessive stinging and superparasitism (Van Lenteren et al., 2006), or because of non-consumptive effects of the natural enemies (Johnson et al., 2007; Thaler and Griffin, 2008; Fill et al., 2012). Although we did not specifically address non-consumptive effects of natural enemies directly in this study, it is known that the mere presence of parasitoids can cause larvae to drop off the plants in order to evade them, and not all larvae will be able to find a plant again (Luginbill, 1928). Also, the parasitoids could disturb herbivore feeding and change other behaviors. These non-consumptive effects of natural enemies may be considerable and should be considered in order to determine the complete beneficial effects of parasitoids for plants.
We observed that the presence of parasitic wasps reduced the amount of damage inflicted by S. frugiperda larvae (Figure 3), and larvae in the presence of wasps had a lower body weight (Figure S4B), confirming laboratory experiments showing that parasitized larvae consume and grow considerably less than healthy larvae (Fritzsche Hoballah and Turlings, 2001). We should note that the lower larval body weight may in part have resulted from non-consumptive effects of the natural enemies, by disturbing larval feeding (Luginbill, 1928; Johnson et al., 2007; Thaler and Griffin, 2008; Fill et al., 2012). The presence of herbivores alone was clearly detrimental for plant survival. This important negative effect on plant fitness was significantly reduced in the presence of parasitoids.
It should be noted that C. sonorensis is a solitary koinobiont endoparasitoid that typically causes its host to consume and grow less (Harvey et al., 1999; Fritzsche Hoballah and Turlings, 2001; Harvey, 2005). In contrast, gregarious parasitoids may stimulate the growth of their host, depending on clutch size (Beckage and Alleyne, 1997; Coleman et al., 1999; Harvey, 2005). Therefore, the effects of parasitoid natural enemies on their hosts and associated host plants cannot be generalized.
Tent experiments have their limitations, but they also offer several advantages. The experimental design enabled precise control of herbivore and parasitoid densities and avoided other potentially confounding factors, biotic or abiotic, among treatments. Moreover, upon assessment of parasitism a week after wasp release, the tents were removed and the plants were exposed to the same natural conditions as the plants in the open plots. The use of tents did not affect plant survival compared to plots without tents (Figure 5).
To complement the tent study, we set up experimental treatments in open plots to evaluate the effects of actual parasitoid recruitment on plant survival under true field conditions. Larval recovery rates were rather low (Figure S4A), but C. sonorensis was found to parasitize the larvae also in the open plots. In these plots, larvae must have faced a number of additional natural enemies, not just C. sonorensis. Nevertheless, parasitism rates in open plots fell within the same range as parasitism rates in herbivore-infested tents with the lowest number of wasps (Figure 2). In agricultural areas, in the absence of pesticides, parasitism rates can be very high (Hoballah et al., 2004; Jourdie et al., 2008; Bahena-Juárez et al., 2010; Von Mérey et al., 2012), and their positive effects on plant performance can be expected to be much more significant.
All plants emitted a considerable amount of HIPVs in response to herbivory (Figure 4, Figure S9, Table S2), and they may have served as cues for the parasitoid wasps to find their hosts. Although differences in volatile quantity (total amount) and quality (the relative importance of the different compounds in the composition of the odor blend) have been reported for different teosinte varieties (Gouinguené et al., 2001; De Lange et al., 2016), and we found differences in emission of individual HIPVs as well as total HIPV quantities in the three teosinte populations used in this study (Figure S9, Table S2), the plants emitted comparable overall volatile blends (Figure 4). Overall, teosinte variety Tzitzio emitted higher total HIPV quantities than the two other varieties. We observed differences in the emission of individual compounds, but could not correlate those to the attractiveness of the plants or the effectiveness of the parasitoids, due to the low occurrence of parasitism in the open plots.
Other studies have also attempted to determine the impact of plant HIPVs on parasitoid recruitment. For maize, Degen et al. (2012) used a similar approach to evaluate larval parasitism on lines with distinct differences in total odor emissions under field conditions in Mexico. They observed no clear-cut correlation between volatile emissions and parasitism rates. The simplistic notion that the more volatiles are released by a plant the more attractive they are to parasitoids has been clearly refuted (D'Alessandro and Turlings, 2006; Sobhy et al., 2012). Moreover, the link between the action of parasitoids and plant performance is not just limited to the emission of HIPVs, and differential herbivore and plant performance will be affected by other phenotypic differences among plant genotypes. The fact that it remains largely unknown which volatiles are of key importance for parasitoid attraction (D'Alessandro and Turlings, 2006) complicates the choice of appropriate genotypes for such studies. A transgenic approach (Schuman et al., 2012) and the use of elicitors (Alborn et al., 1997; Thaler, 1999; Von Mérey et al., 2012), inhibitors (D'Alessandro et al., 2006; Bruinsma et al., 2010) or pure compounds (De Boer and Dicke, 2004; Von Mérey et al., 2011) to manipulate volatile emissions have also been very useful to demonstrate the role of volatiles in predator or parasitoid attraction, but they present similar problems: the treatments may have other unknown effects on the performance of the plants, herbivores or parasitoids. We therefore assert, depending on the research objectives, that such approaches do not offer advantages over those used in the present study: a combination of tent studies and open plots.
Because of logistical constraints, planting of teosinte in the field occurred with a gap between replicates, causing notable variability in results. Also, the teosintes were planted relatively late in the season, and all plants were hit and killed by an early frost, before seed set. This very unfortunate event limits the conclusive power of this study, as we cannot say anything about the ultimate fitness effects of the treatments, which could be best measured in terms of seed number and quality. Nevertheless, the overall results allow us to conclude that the herbivore by itself was highly detrimental for plant survival, and that this important negative effect on plant fitness was significantly reduced in the presence of parasitoids (Figure 5).
In conclusion, we found that larvae of S. frugiperda cause severe damage to teosinte plants, which can result in substantial mortality among seedlings. The presence of parasitoid wasps reduced larval damage and significantly decreased plant mortality. Although our findings will not resolve the long-standing debate on the many possible functions of HIPVs, the study presents support for the hypothesis that plants can benefit from recruiting parasitoid natural enemies.
Author Contributions
EdL, KO, and TT: designed the study. EdL, KF, TD, BG, RA-R, and FB-J: performed the study; EdL and KF: analyzed the data; EdL and TT: wrote the first version of the manuscript, which was edited and approved by all authors.
Funding
This study was supported by a grant from the Swiss National Science Foundation (grant 3100A0-1221132/1).
Conflict of Interest Statement
The authors declare that the research was conducted in the absence of any commercial or financial relationships that could be construed as a potential conflict of interest.
Acknowledgments
We thank Víctor Rocha-Ramírez for his valuable support in the field, and Yolanda Magdalena García-Rodríguez for assistance with gas chromatography. Thanks to Selene Ramos-Ortiz for technical assistance. We thank Matthias Held, Peter Morin, and Dinesh Kumar Barupal for advice on statistical analysis. Thanks to Marianne Duployer for help with the analysis of herbivore damage. We are grateful to George Mahuku, Carlos Muñoz, and Suketoshi Taba at the International Maize and Wheat Improvement Center (CIMMYT), Mexico, for technical assistance. Thanks to Gaylord Desurmont, Gaétan Glauser, Emily Bick, and Ian Kaplan for many useful comments on an earlier version of this manuscript. This study first appeared as a PhD thesis chapter (De Lange, 2014).
Supplementary Material
The Supplementary Material for this article can be found online at: https://www.frontiersin.org/articles/10.3389/fevo.2018.00055/full#supplementary-material
Figure S1. Study organisms. (A) Teosintes (Zea mays ssp. parviglumis and Z. mays ssp. mexicana) in the experimental field. (B) A female Campoletis sonorensis wasp attacking a Spodoptera frugiperda larva. Photograph credits: Elvira de Lange (A) and Matthias Held (B).
Figure S2. Plant height, herbivore and parasitoid presence in eight teosinte populations in Mexico. (A) Plant height (+SE) at the time of the survey. (B) Average number of leaves (+SE) per plant, including small expanding leaves. (C) Average number of Spodoptera frugiperda larvae (+SE) found per plant in each population. (D) Total number of Campoletis sonorensis wasps that emerged from the S. frugiperda larvae that were collected. For an overview of the sites, see Table S1. Seeds from two of the surveyed sites were used for the field experiments in 2010: site 6 corresponds to Cuitzeo and site 7 corresponds to Tzitzio.
Figure S3. Weather data. (A) Minimum and maximum temperatures. (B) Precipitation. The weather station was localized in Cointzio, Michoacán, Mexico, at a distance of about 5 km from the experimental field. The date the temperature reached 0°C corresponds exactly to the date the plants were lost to freezing.
Figure S4. Larval retrieval and weight. The number of Spodoptera frugiperda larvae that were retrieved (a) and larval weight (b). The larvae were obtained from teosinte plants in tents in the absence (SF) and in the presence of different numbers of Campoletis sonorensis parasitoid wasps (+W, ++W, +++W), or from teosinte plants in open plots not covered by a tent (OC, O+SF). Initially, five second-instar larvae were placed on each plant in each treatment plot. Different letters indicate statistically significant differences (GLM, P < 0.05). Lowercase letters represent significant differences between plots in tents. Uppercase letters represent significant differences between all treatments in which herbivores were released. For (a), only plots where larvae were released were included in the statistical analyses. For (b), both open plots were combined as a single “open plot” treatment for statistical analysis. Box plots display median (a line within the box), the 25th percentile (the boundary of the box closest to zero), and the 75th percentile (the boundary of the box farthest from zero). Error bars below and above the box indicate the 10th and the 90th percentile, respectively. Outlying points are shown as solid circles.
Figure S5. Correlation between total larval biomass and herbivore-inflicted damage. Parameters were measured in tents that were infested with Spodoptera frugiperda larvae in the absence (SF) and in the presence of increasing densities of Campoletis sonorensis parasitoid wasps (+W, ++W, +++W), for all three teosinte varieties, in all three replicates of the experiments. R2 = 0.88, P < 0.001. The dashed line represents a linear trendline.
Figure S6. Plant shoot biomass at first harvest. Shoot dry biomass of teosinte plants. Plants were obtained from tents that were not infested with herbivores (treatment C) or that were infested with larvae in the absence (SF) or in the presence of different numbers of Campoletis sonorensis parasitoid wasps (+W, ++W, +++W), or in open plots not covered by a tent (OC, O+SF), 7 days after herbivore infestations. Different letters indicate statistically significant differences (GLM, P < 0.05). Minuscule letters represent significant differences between plots in tents. Major letters represent significant differences between open plots. Box plots display median (a line within the box), the 25th percentile (the boundary of the box closest to zero), and the 75th percentile (the boundary of the box farthest from zero). Error bars below and above the box indicate the 10th and the 90th percentile, respectively. Outlying points are shown as solid circles.
Figure S7. Correlation between herbivore-inflicted damage and plant shoot biomass. Parameters were measured in tents that were infested with Spodoptera frugiperda larvae in the absence (SF) and in the presence of increasing densities of Campoletis sonorensis parasitoid wasps (+W, ++W, +++W), for all three teosinte varieties, in all three replicates of the experiments. R2 = 0.52, P < 0.001. The dashed line represents a linear trendline.
Figure S8. Correlation between larval retrieval and plant shoot biomass. Parameters were measured in tents that were infested with Spodoptera frugiperda larvae in the absence (SF) and in the presence of increasing densities of Campoletis sonorensis parasitoid wasps (+W, ++W, +++W), for all three teosinte varieties, in all three replicates of the experiments. R2 = 0.53, P < 0.001. The dashed line represents a linear trendline.
Figure S9. Chromatograms of volatiles collected from herbivore-damaged teosinte plants. (A) Teosinte population Tzitzio (Zea mays ssp. parviglumis). (B) Teosinte population Hidalgo (Z. mays ssp. mexicana). (C) Teosinte population Cuitzeo (Z. mays ssp. mexicana). Volatile emissions were measured in tents that were infested with Spodoptera frugiperda larvae in the absence of parasitoid wasps (SF). Collections started 1 day after larval infestations and were repeated on 4, 5, or 6 consecutive days, using different plants. The labeled compounds are among the most abundant volatiles: (1) heptanal; (2) α-pinene; (3) 2-methyl-2-hepten-6-one; (4) (Z)-3-hexenyl acetate; (5) hexyl acetate*; (6) limonene; (7) (Z)-β-ocimene; (8) linalool; (9) (3E)-4,8-dimethyl-1,3,7-nonatriene; (10) 2-ethylhexyl acetate*; (11) phenylmethyl acetate*; (12) 2-phenylethyl acetate; (13) indole; (14) (E)-β-caryophyllene; (15) (E)-α-bergamotene; (16) β-cedrene*; (17) (E)-β-farnesene; (18) β-sesquiphellandrene*; (19) caryophyllene-oxide*; (20) methyl salicylate. * = tentative identification.
Figure S10. Correlation between herbivore-inflicted damage and total volatile emission. Parameters were measured in tents that were infested with Spodoptera frugiperda larvae in the absence of parasitoid wasps (SF), for all three teosinte varieties, in all three replicates of the experiments. R2 = 0.47, P = 0.019. The dashed line represents a linear trendline. HIPV, herbivore-induced plant volatiles.
Table S1. Survey of eight teosinte populations in Mexico in 2009.
Table S2. Absolute amounts of volatiles collected from herbivore-damaged teosinte plants (in peak area ± SE).
File S1. Survey of teosinte, herbivores, and parasitoids.
References
Agrawal, A. A. (2000). Overcompensation of plants in response to herbivory and the by-product benefits of mutualism. Trends Plant Sci. 5, 309–313. doi: 10.1016/S1360-1385(00)01679-4
Agrawal, A. A. (2011). Current trends in the evolutionary ecology of plant defence. Funct. Ecol. 25, 420–432. doi: 10.1111/j.1365-2435.2010.01796.x
Alborn, H. T., Turlings, T. C. J., Jones, T. H., Stenhagen, G., Loughrin, J. H., and Tumlinson, J. H. (1997). An elicitor of plant volatiles from beet armyworm oral secretions. Science 276, 945–949.
Alleyne, M., and Beckage, N. E. (1997). Parasitism-induced effects on host growth and metabolic efficiency in tobacco hornworm larvae parasitized by Cotesia congregata. J. Insect Physiol. 43, 407–424. doi: 10.1016/S0022-1910(96)00086-8
Allison, J. D., and Hare, J. D. (2009). Learned and naive natural enemy responses and the interpretation of volatile organic compounds as cues or signals. New Phytol. 184, 768–782. doi: 10.1111/j.1469-8137.2009.03046.x
Bahena-Juárez, F., De Lange, E., Farnier, K., Cortez-Mondaca, E., Sánchez-Martínez, R., García-Pérez, F., et al. (2010). “Parasitismo en gusano cogollero del maíz Spodoptera frugiperda (J. E. Smith) (Lepidoptera: Noctuidae) en el centro de México”, in Proceedings: XXXIII Congreso Nacional de Control Biológico (Uruapan), 204–209.
Bartlett, R. (2008). Negative interactions between chemical resistance and predators affect fitness in soybeans. Ecol. Entomol. 33, 673–678. doi: 10.1111/j.1365-2311.2008.01028.x
Bernasconi Ockroy, M. L., Turlings, T. C. J., Edwards, P. J., Fritzsche-Hoballah, M. E., Ambrosetti, L., Bassetti, P., et al. (2001). Response of natural populations of predators and parasitoids to artificially induced volatile emissions in maize plants (Zea mays L.). Agric. For. Entomol. 3, 201–209. doi: 10.1046/j.1461-9555.2001.00107.x
Bruinsma, M., Van Broekhoven, S., Poelman, E. H., Posthumus, M. A., Müller, M. J., Van Loon, J. J., et al. (2010). Inhibition of lipoxygenase affects induction of both direct and indirect plant defences against herbivorous insects. Oecologia 162, 393–404. doi: 10.1007/s00442-009-1459-x
Cave, R. D. (1995). Manual para el reconocimiento de parasitoides de plagas agrícolas en América Central. El Zamorano: Zamorano Academic Press.
Cave, R. D. (2000). Biology, ecology and use in pest management of Telenomus remus. Biocontrol News Inform. 21, 21N−26N.
Chapman, J. W., Williams, T., Escribano, A., Caballero, P., Cave, R. D., and Goulson, D. (1999). Fitness consequences of cannibalism in the fall armyworm, Spodoptera frugiperda. Behav. Ecol. 10, 298–303. doi: 10.1093/beheco/10.3.298
Chen, Y. H., Gols, R., and Benrey, B. (2015). Crop domestication and its impact on naturally selected trophic interactions. Annu. Rev. Entomol. 60, 35–58. doi: 10.1146/annurev-ento-010814-020601
Christensen, S. A., Nemchenko, A., Borrego, E., Murray, I., Sobhy, I. S., Bosak, L., et al. (2013). The maize lipoxygenase, ZmLOX10, mediates green leaf volatile, jasmonate and herbivore-induced plant volatile production for defense against insect attack. Plant J. 74, 59–73. doi: 10.1111/tpj.12101
Clark, M. S., Luna, J. M., Stone, N. D., and Youngman, R. R. (1994). Generalist predator consumption of armyworm (Lepidoptera: Noctuidae) and effect of predator removal on damage in no-till corn. Environ. Entomol. 23, 617–622. doi: 10.1093/ee/23.3.617
Coleman, R. A., Barker, A. M., and Fenner, M. (1999). Parasitism of the herbivore Pieris brassicae L. (Lep., Pieridae) by Cotesia glomerata L. (Hym., Braconidae) does not benefit the host plant by reduction of herbivory. J. Appl. Entomol. 123, 171–177.
Crabb, B. A., and Pellmyr, O. (2006). Impact of the third trophic level in an obligate mutualism: do yucca plants benefit from parasitoids of yucca moths? Int. J. Plant Sci. 167, 119–124. doi: 10.1086/497844
Cuny, M. A. C., Gendry, J., Hernández-Cumplido, J., and Benrey, B. (2018) Changes in plant growth seed production in wild lima bean in response to herbivory are attenuated by parasitoids. Oecologia doi: 10.1007/s00442-018-4119-1. [Epub ahead of print].
D'Alessandro, M., Held, M., Triponez, Y., and Turlings, T. C. (2006). The role of indole and other shikimic acid derived maize volatiles in the attraction of two parasitic wasps. J. Chem. Ecol. 32, 2733–2748. doi: 10.1007/s10886-006-9196-7
D'Alessandro, M., and Turlings, T. C. J. (2005). In situ modification of herbivore-induced plant odors: a novel approach to study the attractiveness of volatile organic compounds to parasitic wasps. Chem. Senses 30, 739–753. doi: 10.1093/chemse/bji066
D'Alessandro, M., and Turlings, T. C. (2006). Advances and challenges in the identification of volatiles that mediate interactions among plants and arthropods. Analyst 131, 24–32. doi: 10.1039/B507589K
Dávila-Flores, A. M., DeWitt, T. J., and Bernal, J. S. (2013). Facilitated by nature and agriculture: performance of a specialist herbivore improves with host-plant life history evolution, domestication, and breeding. Oecologia 173, 1425–1437. doi: 10.1007/s00442-013-2728-2
De Boer, J. G., and Dicke, M. (2004). The role of methyl salicylate in prey searching behavior of the predatory mite Phytoseiulus persimilis. J. Chem. Ecol. 30, 255–271. doi: 10.1023/B:JOEC.0000017976.60630.8c
Degen, T., Bakalovic, N., Bergvinson, D., and Turlings, T. C. (2012). Differential performance and parasitism of caterpillars on maize inbred lines with distinctly different herbivore-induced volatile emissions. PLoS ONE 7:e47589. doi: 10.1371/journal.pone.0047589
Degen, T., Dillmann, C., Marion-Poll, F., and Turlings, T. C. (2004). High genetic variability of herbivore-induced volatile emission within a broad range of maize inbred lines. Plant Physiol. 135, 1928–1938. doi: 10.1104/pp.104.039891
De Lange, E. S. (2014). Tritrophic Interactions on Cultivated Maize and Its Wild Ancestor, Teosinte. Ph.D. thesis, University of Neuchâtel, Neuchâtel. Available online at: http://doc.rero.ch/record/232964
De Lange, E. S., Balmer, D., Mauch-Mani, B., and Turlings, T. C. J. (2014). Insect and pathogen attack and resistance in maize and its wild ancestors, the teosintes. New Phytol. 204, 329–341. doi: 10.1111/nph.13005
De Lange, E. S., Farnier, K., Gaudillat, B., and Turlings, T. C. J. (2016). Comparing the attraction of two parasitoids to herbivore-induced volatiles of maize and its wild ancestors, the teosintes. Chemoecology 26, 33–44. doi: 10.1007/s00049-015-0205-6
De Moraes, C. M., Lewis, W. J., Paré, P. W., Alborn, H. T., and Tumlinson, J. H. (1998). Herbivore-infested plants selectively attract parasitoids. Nature 393, 570–573.
Desurmont, G. A., Laplanche, D., Schiestl, F. P., and Turlings, T. C. (2015). Floral volatiles interfere with plant attraction of parasitoids: ontogeny-dependent infochemical dynamics in Brassica rapa. BMC Ecol. 15:17. doi: 10.1186/s12898-015-0047-7
Dicke, M., and Baldwin, I. T. (2010). The evolutionary context for herbivore-induced plant volatiles: beyond the ‘cry for help’. Trends Plant Sci. 15, 167–175. doi: 10.1016/j.tplants.2009.12.002
Dicke, M., and Sabelis, M. W. (1988). How plants obtain predatory mites as bodyguards. Neth. J. Zool. 38, 148–165.
Fill, A., Long, E. Y., and Finke, D. L. (2012). Non-consumptive effects of a natural enemy on a non-prey herbivore population. Ecol. Entomol. 37, 43–50. doi: 10.1111/j.1365-2311.2011.01333.x
Fontana, A., Held, M., Fantaye, C. A., Turlings, T. C., Degenhardt, J., and Gershenzon, J. (2011). Attractiveness of constitutive and herbivore-induced sesquiterpene blends of maize to the parasitic wasp Cotesia marginiventris (Cresson). J. Chem. Ecol. 37, 582–591. doi: 10.1007/s10886-011-9967-7
Fritzsche Hoballah, M. E., and Turlings, T. C. J. (2001). Experimental evidence that plants under caterpillar attack may benefit from attracting parasitoids. Evol. Ecol. Res. 3, 553–565.
Gaillard, M. D. P., Glauser, G., Robert, C. A. M., and Turlings, T. C. J. (2018). Fine-tuning the ‘plant domestication-reduced defense’ hypothesis: specialist vs generalist herbivores. New Phytol. 217, 355–366. doi: 10.1111/nph.14757
Geervliet, J. B. F., Vet, L. E. M., and Dicke, M. (1996). Innate responses of the parasitoids Cotesia glomerata and C. rubecula (Hymenoptera: Braconidae) to volatiles from different plant-herbivore complexes. J. Insect. Behav. 9, 525–538.
Gols, R., Wagenaar, R., Poelman, E. H., Kruidhof, H. M., van Loon, J. J. A., and Harvey, J. A. (2015). Fitness consequences of indirect plant defence in the annual weed, Sinapis arvensis. Funct. Ecol. 29, 1019–1025. doi: 10.1111/1365-2435.12415
Gomez, J. M., and Zamora, R. (1994). Top-down effects in a tritrophic system: parasitoids enhance plant fitness. Ecology 75, 1023–1030. doi: 10.2307/1939426
Gouinguené, S., Degen, T., and Turlings, T. C. J. (2001). Variability in herbivore-induced odour emissions among maize cultivars and their wild ancestors (teosinte). Chemoecology 11, 9–16. doi: 10.1007/PL00001832
Hare, J. D. (2011). Ecological role of volatiles produced by plants in response to damage by herbivorous insects. Annu. Rev. Entomol. 56, 161–180. doi: 10.1146/annurev-ento-120709-144753
Harvey, J. A. (2005). Factors affecting the evolution of development strategies in parasitoid wasps: the importance of functional constraints and incorporating complexity. Entomol. Exp. Appl. 117, 1–13. doi: 10.1111/j.1570-7458.2005.00348.x
Harvey, J. A., Jervis, M. A., Gols, R., Jiang, N., and Vet, L. E. M. (1999). Development of the parasitoid, Cotesia rubecula (Hymenoptera: Braconidae) in Pieris rapae and Pieris brassicae (Lepidoptera: Pieridae): evidence for host regulation. J. Insect Physiol. 45, 173–182. doi: 10.1016/S0022-1910(98)00113-9
Heil, M. (2008). Indirect defence via tritrophic interactions. New Phytol. 178, 41–61. doi: 10.1111/j.1469-8137.2007.02330.x
Heil, M. (2014). Herbivore-induced plant volatiles: targets, perception and unanswered questions. New Phytol. 204, 297–306. doi: 10.1111/nph.12977
Hoballah, M. E., Degen, T., Bergvinson, D., Savidan, A., Tamò, C., and Turlings, T. C. J. (2004). Occurrence and direct control potential of parasitoids and predators of the fall armyworm (Lepidoptera: Noctuidae) on maize in the subtropical lowlands of Mexico. Agric For Entomol 6, 83–88. doi: 10.1111/j.1461-9555.2004.00207.x
Howe, G. A., and Jander, G. (2008). Plant immunity to insect herbivores. Annu. Rev. Plant Biol. 59, 41–66. doi: 10.1146/annurev.arplant.59.032607.092825
Isenhour, D. J. (1985). Campoletis sonorensis [Hym.: Ichneumonidae] as a parasitoid of Spodoptera frugiperda [Lep.: Noctuidae]: host stage preference and functional response. Entomophaga 30, 31–36.
Johnson, M. L., Armitage, S., Scholz, B. C. G., Merritt, D. J., Cribb, B. W., and Zalucki, M. P. (2007). Predator presence moves Helicoverpa armigera larvae to distraction. J. Insect Behav. 20, 1–18. doi: 10.1007/s10905-006-9048-x
Jourdie, V., Alvarez, N., and Turlings, T. C. J. (2008). Identification of seven species of hymenopteran parasitoids of Spodoptera frugiperda, using polymerase chain reaction amplification and restriction enzyme digestion. Agric. For. Entomol. 10, 129–136. doi: 10.1111/j.1461-9563.2008.00362.x
Kaplan, I. (2012). Trophic complexity and the adaptive value of damage-induced plant volatiles. PLoS Biol. 10:e1001437. doi: 10.1371/journal.pbio.1001437
Karban, R., and Balwin, I. T. (1997). Induced Responses to Herbivory. Chicago, IL: Chicago University Press.
Kessler, A., and Baldwin, I. T. (2001). Defensive function of herbivore-induced plant volatile emissions in nature. Science 291, 2141–2144. doi: 10.1126/science.291.5511.2141
Kessler, A., and Heil, M. (2011). The multiple faces of indirect defences and their agents of natural selection. Funct. Ecol. 25, 348–357. doi: 10.1111/j.1365-2435.2010.01818.x
Khan, Z. R., Ampong-Nyarko, K., Chiliswa, P., Hassanali, A., Kimani, S., Lwande, W., et al. (1997). Intercropping increases parasitism of pests. Nature 388, 631–632.
Köllner, T. G., Held, M., Lenk, C., Hiltpold, I., Turlings, T. C. J., Gershenzon, J., et al. (2008). A maize (E)-β-caryophyllene synthase implicated in indirect defense responses against herbivores is not expressed in most American maize varieties. Plant Cell 20, 482–494. doi: 10.1105/tpc.107.051672
Lucas-Barbosa, D., Dicke, M., Kranenburg, T., Aartsma, Y., van Beek, T. A., Huigens, M. E., et al. (2017). Endure and call for help: strategies of black mustard plants to deal with a specialized caterpillar. Funct. Ecol. 31, 325–333. doi: 10.1111/1365-2435.12756
Martínez-Martínez, L., Padilla-Cortes, E., Jarquín-López, R., Sánchez-García, J. A., and Cisneros-Palacios, M. E. (2015). Performance of fall armyworm, Spodoptera frugiperda (Smith), fed with corn and castor bean leaves. Entomol. Mexicana 2, 397–403.
Matsumoto, T., Itioka, T., and Nishida, T. (2003). Cascading effects of a specialist parasitoid on plant biomass in a Citrus agroecosystem. Ecol Res 18, 651–659. doi: 10.1111/j.1440-1703.2003.00586.x
Matsuoka, Y., Vigouroux, Y., Goodman, M. M., Sanchez, G. J., Buckler, E., and Doebley, J. (2002). A single domestication for maize shown by multilocus microsatellite genotyping. Proc. Natl. Acad. Sci. U.S.A. 99, 6080–6084. doi: 10.1073/pnas.052125199
Moran, M. D., Rooney, T. P., and Hurd, L. E. (1996). Top-down cascade from a bitrophic predator in an old-field community. Ecology 77, 2219–2227. doi: 10.2307/2265715
Poelman, E. H. (2015). From induced resistance to defence in plant-insect interactions. Entomol. Exp. Appl. 157, 11–17. doi: 10.1111/eea.12334
Rasband, W. S. (1997–2012). “ImageJ”. (Bethesda, MD: U.S. National Institutes of Health). Available online at: http://imagej.nih.gov/ij/
R Core Team (2016). “R: a Language and Environment for Statistical Computing”. Vienna: R Foundation for Statistical Computing.
Rosenthal, J. P., and Dirzo, R. (1997). Effects of life history, domestication and agronomic selection on plant defence against insects: evidence from maizes and wild relatives. Evol Ecol 11, 337–355. doi: 10.1023/A:1018420504439
Sanchez Gonzalez, J. J., and Ruiz Corral, J. A. (1995). “Teosinte distribution in Mexico,” in Proceedings of a Forum - Gene Flow Among Maize Landraces, Improved Maize Varieties, and Teosinte: Implications for Transgenic Maize, eds J. A. Serratos, M. C. Willcox, and F. Castillo (Mexico: CIMMYT), 18–39.
Schmelz, E. A., Carroll, M. J., LeClere, S., Phipps, S. M., Meredith, J., Chourey, P. S., et al. (2006). Fragments of ATP synthase mediate plant perception of insect attack. Proc. Natl. Acad. Sci. U.S.A. 103, 8894–8899. doi: 10.1073/pnas.0602328103
Schnee, C., Köllner, T. G., Held, M., Turlings, T. C., Gershenzon, J., and Degenhardt, J. (2006). The products of a single maize sesquiterpene synthase form a volatile defense signal that attracts natural enemies of maize herbivores. Proc. Natl. Acad. Science. U.S.A. 103, 1129–1134. doi: 10.1073/pnas.0508027103
Schuman, M. C., Barthel, K., and Baldwin, I. T. (2012). Herbivory-induced volatiles function as defenses increasing fitness of the native plant Nicotiana attenuata in nature. Elife 1:e00007. doi: 10.7554/eLife.00007
Smallegange, R. C., Van Loon, J. J. A., Blatt, S. E., Harvey, J. A., and Dicke, M. (2008). Parasitoid load affects plant fitness in a tritrophic system. Entomol. Exp. Appl. 128, 172–183. doi: 10.1111/j.1570-7458.2008.00693.x
Sobhy, I. S., Erb, M., Sarhan, A. A., El-Husseinu, M. M., Mandour, N. S., and Turlings, T. C. (2012). Less is more: treatment with BTH and laminarin reduces herbivore-induced volatile emissions in maize but increases parasitoid attraction. J. Chem. Ecol. 38, 348–360. doi: 10.1007/s10886-012-0098-6
Steiger, S., Schmitt, T., and Schaefer, H. M. (2011). The origin and dynamic evolution of chemical information transfer. Proc. R. Soc. B 278, 970–979. doi: 10.1098/rspb.2010.2285
Steinberg, S., Dicke, M., Vet, L. E. M., and Wanningen, R. (1992). Response of the braconid parasitoid Cotesia (= Apanteles) glomerata to volatile infochemicals: effects of bioassay set-up, parasitoid age and experience and barometric flux. Entomol. Exp. Appl. 63, 163–175. doi: 10.1111/j.1570-7458.1992.tb01571.x
Takabayashi, J., Dicke, M., and Posthumus, M. A. (1994). Volatile herbivore-induced terpenoids in plant-mite interactions: variation caused by biotic and abiotic factors. J. Chem. Ecol. 20, 1329–1354. doi: 10.1007/BF02059811
Tamò, C., Ricard, I., Held, M., Davison, A. C., and Turlings, T. C. J. (2006). A comparison of naïve and conditioned responses of three generalist endoparasitoids of lepidopteran larvae to host-induced plant odours. Anim. Biol. 56, 205–220. doi: 10.1163/157075606777304177
Thaler, J. S. (1999). Jasmonate-inducible plant defences cause increased parasitism of herbivores. Nature 399, 686–688.
Thaler, J. S., and Griffin, C. A. M. (2008). Relative importance of consumptive and non-consumptive effects of predators on prey and plant damage: the influence of herbivore ontogeny. Entomol. Exp. Appl. 128, 34–40. doi: 10.1111/j.1570-7458.2008.00737.x
Tooker, J. F., and Hanks, L. M. (2006). Tritrophic interactions and reproductive fitness of the prairie perennial Silphium laciniatum Gillette (Asteraceae). Environ. Entomol. 35, 537–545. doi: 10.1603/0046-225X-35.2.537
Turlings, T. C. J., Davison, A. C., and Tamò, C. (2004). A six-arm olfactometer permitting simultaneous observation of insect attraction and odour trapping. Physiol. Entomol. 29, 45–55. doi: 10.1111/j.1365-3032.2004.0362.x
Turlings, T. C. J., and Erb, M. (2018). Tritrophic interactions mediated by herbivore-induced plant volatiles: mechanisms, ecological relevance, and application potential. Annu. Rev. Entomol. 63, 433–452. doi: 10.1146/annurev-ento-020117-043507
Turlings, T. C. J., and Fritzsche, M. E. (1999). “Attraction of parasitic wasps by caterpillar-damaged plants,” in Insect-Plant Interactions and Induced Plant Defence, eds D. J. Chadwick and J.A. Goode (Chicheste: John Wiley & Sons), 21–38.
Turlings, T. C. J., Lengwiler, U. B., Bernasconi, M. L., and Wechsler, D. (1998). Timing of induced volatile emissions in maize seedlings. Planta 207, 146–152. doi: 10.1007/s004250050466
Turlings, T. C., Loughrin, J. H., McCall, P. J., Rose, U. S., Lewis, W. J., and Tumlinson, J. H. (1995). How caterpillar-damaged plants protect themselves by attracting parasitic wasps. Proc. Natl. Acad. Sci. U.S.A. 92, 4169–4174. doi: 10.1073/pnas.92.10.4169
Turlings, T. C., Tumlinson, J. H., and Lewis, W. J. (1990). Exploitation of herbivore-induced plant odors by host-seeking parasitic wasps. Science 250, 1251–1253.
Valicente, F. H., Tuelher, E. S., Pena, R. C., Andreazza, R., and Guimarães, M. R. F. (2013). Cannibalism and virus production in Spodoptera frugiperda (J.E. Smith) (Lepidoptera: Noctuidae) larvae fed with two leaf substrates inoculated with Baculovirus spodoptera. Neotrop. Entomol. 42, 191–199. doi: 10.1007/s13744-013-0108-6
Van Lenteren, J. C., Cock, M. J. W., Hoffmeister, T. S., and Sands, D. P. A. (2006). “Host specificity in arthropod biological control, methods for testing and interpretation of the data”, in Environmental Impact of Invertebrates for Biological Control of Arthropods, eds F. Bigler, D. Babendreier and U. Kuhlmann (Wallingford: CABI Publishing), 38–63.
Van Loon, J. J. A., De Boer, J. G., and Dicke, M. (2000). Parasitoid-plant mutualism: parasitoid attack of herbivore increases plant reproduction. Entomol. Exp. Appl. 97, 219–227. doi: 10.1046/j.1570-7458.2000.00733.x
Von Mérey, G. E., Veyrat, N., De Lange, E., Degen, T., Mahuku, G., López Valdez, R., et al. (2012). Minor effects of two elicitors of insect and pathogen resistance on volatile emissions and parasitism of Spodoptera frugiperda in Mexican maize fields. Biol. Control. 60, 7–15. doi: 10.1016/j.biocontrol.2011.09.010
Von Mérey, G., Veyrat, N., Mahuku, G., López Valdez, R., Turlings, T. C., and D'Alessandro, M. (2011). Dispensing synthetic green leaf volatiles in maize fields increases the release of sesquiterpenes by the plants, but has little effect on the attraction of pest and beneficial insects. Phytochemistry 72, 1838–1847. doi: 10.1016/j.phytochem.2011.04.022
Keywords: Campoletis sonorensis, parasitoid wasp, Spodoptera frugiperda, tritrophic interactions, volatiles, Zea mays
Citation: de Lange ES, Farnier K, Degen T, Gaudillat B, Aguilar-Romero R, Bahena-Juárez F, Oyama K and Turlings TCJ (2018) Parasitic Wasps Can Reduce Mortality of Teosinte Plants Infested With Fall Armyworm: Support for a Defensive Function of Herbivore-Induced Plant Volatiles. Front. Ecol. Evol. 6:55. doi: 10.3389/fevo.2018.00055
Received: 27 October 2017; Accepted: 16 April 2018;
Published: 18 May 2018.
Edited by:
Marie Bengtsson, Swedish University of Agricultural Sciences, SwedenReviewed by:
Amanuel Tamiru, International Centre of Insect Physiology and Ecology, KenyaAntonino Cusumano, Wageningen University & Research, Netherlands
Copyright © 2018 de Lange, Farnier, Degen, Gaudillat, Aguilar-Romero, Bahena-Juárez, Oyama and Turlings. This is an open-access article distributed under the terms of the Creative Commons Attribution License (CC BY). The use, distribution or reproduction in other forums is permitted, provided the original author(s) and the copyright owner are credited and that the original publication in this journal is cited, in accordance with accepted academic practice. No use, distribution or reproduction is permitted which does not comply with these terms.
*Correspondence: Ted C. J. Turlings, dGVkLnR1cmxpbmdzQHVuaW5lLmNo
†Present Address: Elvira S. de Lange, Department of Entomology and Nematology, University of California Davis, Davis, CA, United States
Kevin Farnier, Biosciences Research Division, Department of Economic Development, Jobs, Transport and Resources, AgriBio, Centre for AgriBioscience, La Trobe University, Melbourne, VIC, Australia
Benjamin Gaudillat, UMR ‘Peuplements Végétaux et Bio-agresseurs en Milieu Tropical’, Pôle de Protection des Plantes, CIRAD/Université de La Réunion, Saint-Pierre, France