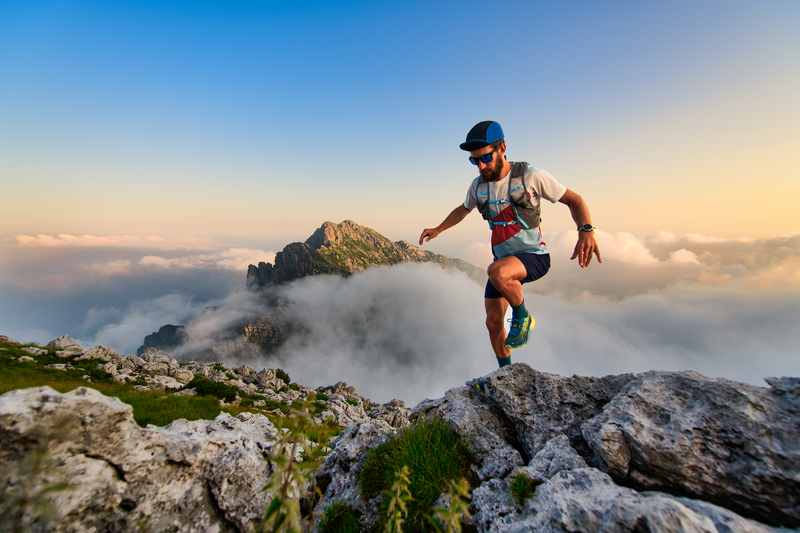
94% of researchers rate our articles as excellent or good
Learn more about the work of our research integrity team to safeguard the quality of each article we publish.
Find out more
ORIGINAL RESEARCH article
Front. Environ. Sci. , 14 October 2020
Sec. Water and Wastewater Management
Volume 8 - 2020 | https://doi.org/10.3389/fenvs.2020.570904
The presence of pathogens in industrial cooling towers has been identified as one of the causes of legionellosis, but the tangible hazard has been unrecognized. The major function of a cooling tower in the industries is to transfer heat into the environment through evaporation which leads to an unusual increment in the conductivity of water. Conductivity indicates dissolved solids of water which further facilitate the occurrence of corrosion within the cooling tower systems that may hamper the overall functioning of the cooling tower. The lab-scale study was conducted to developed and demonstrated an efficient membrane to control potential pathogen (Legionella sp.) associated with cooling tower water. In the present study, cooling tower water from various locations was collected and examined for physicochemical parameters (pH, conductivity, salinity, sulfate, and APS reductase) and microbial load. The phylogenetic analysis of the potential sample (with a maximum load of bacterial counts, P1) confirms the presence of Legionella sp., Pseudomonas sp., and Flavobacterium sp. In the present investigation, a unique membrane was developed to regulate the growth of pathogenic contaminants along with sulfate, alkalinity in the cooling tower water. The membrane was capable of reducing conductivity from 6.03 to 4.5 mS/cm and total microbial load reduced by 40% by passing through the liquid. The developed membrane was characterized through FTIR that indicated the presence of carbonyl, C–CH3 and C–O–C group whereas, thermal properties of the membrane was determined through TGA. Thus the developed membrane may be used for environmental monitoring and maintenance, including anti-scale treatment, and physical, chemical, and microbiological control, ensure the good performance of reducing the Legionella sp. risk for public health in cooling tower stations.
The cooling tower is commonly used in various industries, majorly in power plants in order to maintain cool environment during the thermoelectric power generation (Kenny et al., 2009). The assembly of cooling tower was depicted in Figure 1. In the cooling tower systems, the water keeps on circulating which led to the deposition of dissolved and suspended solids (calcium, magnesium, chloride, and silica) in the recirculating water, it may further cause scaling, corrosion within the cooling tower system (Bott, 2009; Altman et al., 2012). The major source of makeup water in cooling tower is either lakes or rivers. The composition of makeup water influences various factors such as biofouling, organic, inorganic and microbial load.
Researchers investigated that the quantity of total dissolved solids was directly correlated with the functioning of cooling tower (Yurudu, 2013). Scaling is one of the major problems that frequently occurred in cooling tower system due to the deposition of insoluble solids that forms hard scale on the surface of cooling tower pipes. The cooling tower water is an excellent source for the growth of microorganisms due to the presence of optimum temperature, micro and macro-nutrients and high surface ratio. Cooling tower is an important source for Legionella growth; they have a large volume of water open to the atmosphere and contain some elements. The genus Legionella is Gram negative bacteria which include approximately 61 species with at least 79 serogroups. The most dangerous species, in 90% of cases that causes legionellosis, is the Legionella pneumophila sero group 1 (Paranjape et al., 2019).
Various reports showed the abundant presence of pathogenic micro-organism such as L. pneumophila and Pseudomonas aeruginosa in the cooling towers (Wery et al., 2008; Iervolino et al., 2017; Paranjape et al., 2019).
These pathogenic bacteria directly affect the respiratory system of human being. Many researchers has reported the strategies to eliminate the pathogenic contaminants using zinc based biocides that further facilitate the growth of microorganism due to the vigorous availability of nutrients (Duda, 2010). To overcome such problems of pathogenic species, various approaches have been employed involving ultra and micro-filtration (Shannon et al., 2008).
In present investigation, an efficient membrane capable of filtering the pathogenic micro-organisms was developed. These methods physicochemical analysis of water was performed in order to determine the chemical content (chloride, sulfate and total dissolved solids) available in cooling tower water. Further, it was analyzed for the presence of general aerobic bacteria, sulfate reducing bacteria (SRB) and pathogenic species such as Legionella. The Legionella presence was further confirmed through biochemical test (oxidase and gelatin hydrolysis essay). The sample was genetically characterized through 16 S rRNA sequencing. To regulate the contaminants in cooling tower system, in house membrane was developed and characterized through FTIR and TGA. The efficiency of the developed membrane was investigated in 10 liter laboratory scale that confirms the reduction in various contaminants in cooling tower water.
The water samples of cooling tower was provided by NTPC Limited from five different power stations P1 water sample was collected from Faridabad location with latitude of 28.4089°N and 77.3178°E, P2 sample from Unchahar with latitude of 25.9041°N and 81.3014°E, P3 sample was collected from Dadri with latitude of 28.5462°N and 77.5561°E, P4 sample was acquired from Jhajjar with latitude of 28.6055°N and 76.6538°E and P5 sample was collected from different area of Faridabad with latitude of 28.3486°N and 77.3623°E.
The samples were collected in sterile containers and transported to the laboratory (TERI) situated in New Delhi for investigation. Further, samples were stored at 4°C for future analysis. Ten liters of cooling water were collected in sterile polytetrafluoroethylene (PTFE) bottles containing a sodium thiosulfate solution (10%, v/v) in order to neutralize any residual disinfectant. All water samples were analyzed for Legionella spp., P. aeruginosa, and heterotrophic aerobic bacterial counts.
Water samples were determined for hydrogen ion concentration and conductivity using pH meter, conductivity meter at, respectively. pH meter was calibrated with different pH buffers (4.0, 7.0 and 9.0) whereas conductivity meter was calibrated with standard KCl solution.
The chemical oxygen demand of water samples were estimated through standard protocols of American Society for Testing and Materials (American Society for Testing and Materials [ASTM], 1995). 0.25 g of MgSO4 was added in COD tubes with the help of spatula. Around 10 ml of water samples, 5 ml of 0.25N K2Cr2O7 and 15 ml of COD acid was added into the tubes. The solution was digested for 2 h at 150°C. Further, the digested samples were titrated against 0.1 N FeH8N2O8S2 until wine red color appeared.
The concentration of sulfate was determined through turbidity method. The samples were mixed with ZnC4H6O4, buffers and BaCl2 (Morais et al., 2001). The absorbance of turbid solution was observed at 420 nm. The calibration was performed with sodium sulfate standards (10, 20, 30, 40 mg/l) and R2 value was around 0.99. The chloride content of samples was estimated through AgNO3 titration where K2CrO4 was used as an indicator (Shukla and Arya, 2018).
The QuickChek SRB kit employed immunoassay technology to detect SRB in samples. The antibodies found in kit were against adenosine 5′-phosphosulfate reductase (APS) enzyme which was prominently present in all strains of SRB that convert sulfate into sulfide as shown in Figure 2. The antibody and enzyme layer become blue in the presence of chromagen. The general aerobic heterotrophic broth (GHB) comprise of 0.5 g/l of yeast extract, 0.5 g/l of proteose peptone, 0.5 g/l of cas-amino acids, 0.5 g/l of glucose, 0.5 g/l of starch. 0.3 g/l of C3H3NaO3, 0.3 g/l of K2HPO4, and 0.05 g/l of MgSO4.7H2O was prepared according to NACE guidelines and sterilized for 15 min at 121°C. The water samples were incubated at a rate of 1% into broth and inoculated at 37°C for 24 h. Based on the total microbial load, sample (P1) was selected for detailed genetic characterization and identification for the presence of pathogenic species.
To facilitate the growth of Legionella, water sample was diluted in KCl-HCl solution (1:10) with pH of 2.2. The diluted water sample was plated on buffered charcoal yeast agar supplemented with L-cysteine. The plates were incubated at 37 ± 1°C for 24 h (Parr et al., 2015).
The colonies were morphologically characterized through gram staining. The bacteria were biochemically tested for the presence of cytochrome c enzyme. The colonies were swirled on the glass slide with 1% of N, N, N, N-tetramethyl-p-phenylenediamine dihydrochloride reagent whereas, in gelatin hydrolysis test, gelatin agar slants comprises of 12% gelatin, 0.5% of peptone and 0.3% of beef extract was prepared and inoculated with P1 sample (Ditommaso et al., 2015).
Antibiotic sensitivity test was performed through disc diffusion method using Muller Hinton agar (2 g of beef extract, 17.5 g of acid hydrolyzate of casein, 1.5 g of starch and 17 g of agar). The antibiotic used in the study were Cefuroxime (30), Amikacin (10 μg), Co-trimoxazole (25 μg), Lincomycin (15 μg), Cefoperazone (75 μg), Chloramphenicol (25 μg), Penicillin G (10 units), Nalidixic acid (30 μg), and Colistin (10 μg). The culture plates were placed with antibiotic disc and incubated at 37°C for 24 h (Jarraud et al., 2012; Kaiser et al., 2017).
The P1 sample was filtered through 0.45 μm pore size, genomic DNA was extracted through PowerSoil DNA isolation kit following the manufacturer’s protocol. Further, DNA was amplified through polymerase chain reaction using universal primer. The cycling program consisted of an initial denaturation step of 95°C for 2 min, followed by 25 cycles of 95°C for 20 s, 55°C for 15 s, and 72°C for 5 min, and a final elongation of 10 min at 72°C. The amplified product was cloned and transformed as described by Sharma et al., 2018. The transformed colonies were sequenced and sequences were compared with database of Basic Local Alignment Search Tool (BLAST) and deposited in Genbank. Phylogenetic tree was constructed by neighbor-joining method using MEGA version 6.06 programs.
A 10% (w/v) of cellulose acetate (Sigma Aldrich) solution was prepared in acetone. Around 20 g of CaCO3 and 9 g of glycerol was added in the suspension. The solution was stir well for 10 min and was casted on glass plate plastered with scotch tape to provide adequate thickness to the membrane. The membrane was air dried for 5–10 min. To remove CaCO3 particles from the membrane, it was dipped in 0.24 M HCl solution that showed appearance of gas bubbles (Bott, 2009).
A permeation test was performed by employing cross flow membrane test unit (comprised of pump, relief valves and pressure guage). The effective membrane area used for permeation measurement was 17.3 cm2. The trans-membrane pressure in fouling test and pure water flux was kept constant at 400 mm Hg. Membrane flux was determined in terms of volume of permeate per unit time (in hours) and area. The membrane flux was expressed in lm–2h–1 (Celik et al., 2011).
Time invested during the filtration process and area of membrane filter was determined using vernier caliper.
The functional groups present in in-house membrane were characterized by Fourier Transform Infrared Spectroscopy (Nicolet, 6700) with a scanning wave number from 4000 to 600 cm–1. The resolution was set at 4 cm–1 with 64 scans per spectrum. An alteration in chemical and physical properties of membrane was analyzed as a function of raise temperature by thermal gravimetric analysis (TGA, Perkin Elmer) under the nitrogen atmosphere with heating rate of temperature 10°C/min.
A commercial membrane (Millipore; Durapore) of pore size 0.22 μm was used to compare efficiency of in-house membrane. The filtration was performed under aseptic conditions.
The filtered water was assessed for hydrogen ions concentration, chemical oxygen demand, salinity, alkalinity and conductivity. The alkalinity of water was determined through sulfuric acid titration. The samples were titrated against 0.02N sulfuric acid with phenolphthalein indicator until light pink color appeared. The microbial load (including Legionella sp.) was also estimated using the spread plate technique and colony forming unit (CFU/ml) was determined.
The surface morphology of used in-house membrane was observed by Scanning electron microscopy (Zeiss EVO MA 10 scanning electron microscope). Micrographs of the membrane were captured digitally using digital image transfer recognition program of Zeiss. The dried sample of the membrane was coated with gold and mounted onto aluminums stabs with adhesive tape and examined at 10 KV (Sharma et al., 2018).
A statistical analysis was performed using Microsoft Excel 2010. One-way analysis of variance (ANOVA) was performed to identify significant difference between the efficiency of retaining contaminants by commercial and in-house membrane. The probability (p) < 0.05 represent the significant difference among the variables.
Scale up study was performed in a column separated with in-house membrane as depicted in Figure 3. The column was attached with the vacuum pump. A 10 liter of sample was added into the chamber; filtrate was collected in sterile collector and was analyzed for various microbial and physico-chemical parameters.
The pH of water samples (P1, P2, P3, P4, and P5) were near neutral. The neutral pH of water may facilitate the growth of various micro-organisms. The conductivity of samples was recorded. Among all, P1 showed maximum conductivity of 6.03 ± 0.015 mS/cm whereas 5.26 ± 0.2, 0.80 ± 0.02, 0.66 ± 0.02, and 0.25 ± 0.01 mS/cm was observed in P2, P3, P4, and P5, respectively. Similarly, total dissolved solids were maximum (4.01 × 103 ppm) in P1 sample. The chemical oxygen demand was significantly higher (96 ± 02 mg/l) in P1 sample as compared to P2 whereas, other samples does not showed any chemical oxygen demand or it may be below the detection limit. The chloride and alkalinity content of water samples were in the range of 45 ± 0.1–85 ± 0.2 and 22.3 ± 0.5–1379.7 ± 15 mg/l, respectively (Table 1). Maximum content of conductivity, total dissolved solids and chloride may accelerate the metal corrosion in cooling tower system (Samimi, 2013).
Diverse group of microbes were vigorously found in water sample, including aerobes, anaerobes and SRB. General aerobic bacteria were estimated according to NACE guidelines, the results were described in Table 2. APS reductase data depicted the presence of SRB’s in four water samples except P2 where the concentration of SRB’s were significantly less (Supplementary Figure S1). The P1 sample was appeared to be highly contaminated with excessive general aerobic bacteria and other contaminants. Further, studies were performed with P1 sample.
Report of Subbaram et al. (2017) demonstrated that L. pneumophila was the most virulent species as it showed 100% mortality in suckling mice during pathogenicity studies whereas its presence in the water bodies may pose public health risk. Various studies represented outbreak of Legionellosis in developed countries (Walser et al., 2014). To confirm the presence of Legionella in p1 sample, selective media (buffered charcoal yeast agar) was used. Isolated bacterial colony was appeared to be gram negative, rod shaped as described by Sharma et al., 2019. Colonies were oxidase and gelatinase positive (Supplementary Figure S2).
Further, to determine the pathogenicity of isolated Legionella species antibiotic sensitivity assay was performed where nine different antibiotics were used viz. amikacin, co-trimoxazole, cefuroxime, lincomycin, cefroperazone, chloramphenicol, penicillin G, nalidixic acid, colistin. Legionella species shows resistant against two antibiotics (Lincomycin and Peniciliin G). The Legionella species was susceptible against other antibiotics (Supplementary Figure S3). The diameter of zone of inhibition was lying in a range of 0.9 ± 0.03 to 2.6 ± 0.09 cm (Table 3). The results were performed in triplicates and data points were average of the triplicate ± standard deviation (less than 5% of average).
Uncultured based 16S rRNA sequencing study was characterized in Figure 4. The sequences were available under accession number MK 910197-MK910199. Phylogenetic profile indicates the bacterial genera found in P1 sample was Legionella sp., Pseudomonas sp. and Flavobacterium sp. Legionella species does not shows any antagonistic characteristic in reference with Pseudomonas and Flavobacterium sp. (Bertelli et al., 2018). Previous reports suggested that Pseudomonas, Blastomonas, Methylobacterium, and unclassified genera from Bukholderiaceae family constitute the core micro- biome of cooling tower (Paranjape et al., 2019).
Figure 4. Phylogenetic tree of bacterial community of P1 sample was constructed using neighbor-joining through bacterial 16S rRNA gene sequences. Numbers at nodes indicated the bootstrap values >50% from, 1000 replicates. The scale bar indicates sequence divergence.
Various studies shows pathogenic bacterial genera intensely detected in cooling tower system have been accompanied with biofilm formation i.e., Legionella, Pseudomonas, Mycobacterium, Leptospira, Achromobacter, and Bosea (Greub and Raoult, 2004; Thomas et al., 2010). Legionella pneumophila has been shown to be one of the utmost abundant members of Legionella community persist in a cooling tower water for a long period of time (Carvalho et al., 2007; Wery et al., 2008; Parthuisot et al., 2010).
The cellulose acetate membrane was prepared in-house (manually). The presence of calcium carbonate in the membrane slurry makes it fragile. The pores in the membrane were generated after hydrochloric acid treatment, which reacts with the calcium carbonate leading to the release of carbon dioxide;
The glycerol serves as the key ingredients that regulate pore size and gradient along the cross section of the membrane (Hess et al., 2015). The membrane was appeared white in color with robust nature. Figure 5 illustrated the scanning electron micrographs of membrane with the pore size of 0.027–3.0 μm. Further, permeation study demonstrated gradually reduction in sample when compared with pure water due to the presences of dust, silt and other impurities.
Figure 5. Scanning electron micrographs of cellulose acetate membrane cross sections (A) represents the presence of calcium carbonate in the membrane, (B–D) represents membrane on the left side and its scanning micrograph after the removal of calcium carbonate at different magnifications.
The membrane was characterized by FTIR spectroscopy where spectrum showed distinctive acetate peak at 1737 cm–1 that was associated with the symmetric stretching of carbonyl group (C = O). The characteristic peaks at 1369, 1219 cm–1 wavenumber corresponds to C-CH3 and C-O-C stretching frequencies, respectively (Gopiraman et al., 2013). The intense peak at 3281.52 corresponds to O-H stretching as shown in Figure 6.
The TGA thermogram of the in-house membrane was shown in Figure 7. The volatization of volatile matter was occurred in the initial phase between 25 and 330°C. The significant thermal degradation of in house membrane was monitored between 330 and 450°C. The overall weight loss of membrane was around 70–80% (Arthanareeswaran et al., 2004).
Membrane filtration was employed in cooling tower systems as a treatment option that would allow water re-circulation back to the system. The performance of membranes was tested for sulfate and salinity removal from the water sample of cooling tower (Feng, 2008). Generally, higher the salinity of water, higher will be the energy required for its removal (DiFilippo, 2004; Altman et al., 2012). Membrane systems in general have lower water recovery, but are more economical as compared to other alternative methods.
After the membrane treatment, the filtrate of P1 sample was investigated for various parameters (microbial and physico-chemical). The untreated P1 sample serves as a control. The filtrate shows slight reduction in pH and around 25% reduction in conductivity, 28% in salinity, 48% reduction in sulfate and 47% in alkalinity. The microbial load of control sample was 1.8 × 105 CFU/ml that drastically reduced up to 40% in treated P1 sample whereas, the CFU/ml of Legionella was also reduced. To compare the efficiency of in-house membrane, the P1 sample was treated with commercial membrane of 0.22 μm pore size. The commercial membrane also showed reduction in all the parameters such as pH, salinity, conductivity, alkalinity etc. (Figure 8). The outcomes of commercial membrane illustrated similar pattern as obtained in case of in-house membrane. The in-house membrane appeared to be cost effective. The cost of overall experiment of membrane generation was relatively low.
Figure 8. Described the significant reduction in various parameters in control, in-house treated and commercially treated P1 sample.
Further, study was confirmed through scanning electron microscopy, at magnification of 25,000×. The micrograph of used in-house membrane evidently represents rod shaped contaminants that were retained on the surface of membrane (Figure 9).
Figure 9. Scanning micrograph of microbial contaminants retained on the surface of in-house membrane.
The treated water was analyzed for various parameters (conductivity, salinity, alkalinity, COD, and CFU/ml). The treated sample showed 30–50% reduction in COD, alkalinity and salinity. Conductivity was found in traces in treated water sample. The filtration tends to reduce the microbial load, biofilm, and other contaminants in significant amount.
The in-house membrane has capability to reduce particulate matter that can cause clogging and fouling of cooling tower systems. The pore size of filter plays an important role in eliminating the entire particulate matter. Due to this, there will be a reduction in water consumption as water being re-circulated through the system for longer duration. This tends to reduce the energy consumption as filtration reduces the occurrence of scale and fouling on heat exchangers. Due to filtration, biological growth can be controlled and reduction can mitigate potential health problems caused by pathogenic species (Legionella sp.) (Latzer, 2012).
The environmental studies of Legionnaires infection associated with the cooling tower have revealed poorly sustained systems, lack of control procedures, and failure of system equipment. Various investigators and guidelines recommended the regular maintenance of the cooling tower to control the fouling, scaling occurred due to deposition of salts, microbial growth. Additionally, routine examination and implementation of corrective measures have been recommended to reduce the water stagnation. Further measures involve the cleaning of all internal parts, such as the heat exchanger, and removing dirt, dust, dissolved solids, and organic materials.
As described by Krumholz et al., 2013, a great number of the cooling tower did not have a risk assessment and management plan in place, an operational manual available, or standardized cleaning and maintenance procedures, indicating that the Cooling tower operation was not being systematically monitored. The present study was focused on the removal of pathogenic micro-organisms present in the cooling tower water through the developed membrane. Krumholz et al., 2013 described Desulfovibrio species used sulfate as substrate and converted it to adenosyl phosphorsulfate which involved ATP. Further, APS reductase facilitates the reduction of APS to sulfite and AMP. The production of H2S gas tends to accelerate the corrosion in the industrial cooling tower systems (Krumholz et al., 2013).
We hypothesized that the resident microbial population influences the colonization, survival, and proliferation of L. pneumophila in cooling towers. The source of the cooling water P1 was the main factor explaining the difference in the microbial composition of the cooling towers in our study.
The Legionella isolated from the hot water systems were commonly identified as L. pneumophila, in line with the other researchers such as Bargellini et al. (2011). Although more than 50 species of genus Legionella was found in the natural environments, most of the species are pneumophila and other lower reservoirs of other species as anysa, longbeachae and micdadei have been described by Edagawa et al., 2008 and Mouchtouri et al., 2007.
A researcher (Han et al., 2012) showed the presence of highly dynamic taxa that suggests the abundance of biofilm forming and biofilm associated microbes in the cooling tower basin (FeiTsao et al., 2019). Another report shows the probability of presence of pathogenic Legionella sp. was around 40% in Asia, Australia and Europe. Similarly, recent outbreak in New York demonstrated the presence of 38% of L. pneumophila and 25% of its isolates (Mouchtouri et al., 2010).
Another report represents that 72% of samples were contribute L. pneumophila that indicate a possible correlation between diversity of Legionella sp. in water and culturability of L. pneumophila (Llewellyn et al., 2017). Additionally, all the Legionella sp. identified so far in the various investigations are considered to be potentially pathogenic (Burillo et al., 2017). A study emphasized that cooling towers of pharmaceutical plants and oil refinery and found high levels of Proteobacteria, such as Rhodobacteraceae, Sphingomonadaceae, Bradyrhizobiaceae, as well as Cyanobacteria, but no Pseudomonas Bargellini et al. (2011) and Sharmin et al. (2013). Thus, the community composition seems to be influenced by the intrinsic properties of a cooling tower and its geographic location.
The presence of bacterial phyla and Proteobacteria families within cooling towers remains homogenous across all US climate regions. This study represents that climate and geography may have little impact on the microbial communities. A study conducted in Queensland, Australia reported Proteobacteria and Bacteriodetes were the abundant phyla in cooling towers industries (Sharma et al., 2015).
Pseudomonas species, the most prevalent in seawater and industrial water, were reported to cause corrosion on the mild steel, stainless steel and aluminum alloy in industrial habitats (Gubner and Beech, 2000). Pseudomonas has also been shown to cause the corrosion of metal and pitting on the metal surface of cooling tower system. Busalmen et al. (2002) described that corrosion rate tends to accelerate by cathodic reduction caused by catalase enzyme produced by Pseudomonas. In the phylogenetic tree, the isolates exhibited high nucleotide sequence similarity with P. aeruginosa and P. species with high similarity values of greater than 98%.
The microfiltration and ultrafiltration impartial processes and their combination tends to work efficiently in order to remove the suspended particles, macromolecules and oil as a pretreatment step whereas the combination of ultra-low-pressure nanofiltration and reverse osmosis has been implemented to treat the produced water for greater water quality standards which are potable and for irrigation (Le and Nunes, 2016).
The desalination process occurred in the cooling tower water during the membrane distillation technology is a promising solution. The technology appeared to be much more resistant to the fouling than the pressure driven desalination. The foremost advantage of utilizing membrane distillation despite of nanofiltration was that it involves the saving of energy, make up water and chemicals (Koeman-Stein et al., 2016). Thus, the developed membrane can be used for the industrial application.
Cooling tower are highly efficiency air handling systems, extensively used in the thermal power plants. Our data reveals that the preventive measures, including cleaning, disinfection treatments, physico chemical and microbial analyses was undertaken during the entire operation. Scaling and corrosion of the tower components can affect its functionality and increase the risk of the release of airborne microorganisms, such as Legionella. In the present study the presence of pathogenic bacteria viz. Legionella sp., Pseudomonas sp. and Flavobacterium sp in the most contaminated water sample (P1) through 16S rRNA sequencing. The membrane appeared to be an alternative aspirant which eliminate pathogens in a chemicals free approach and the developed of cellulose membrane was easy and economical which can be implemented in the industrial scale. The results in this study promote a preventive approach for the surveillance of Legionella in cooling tower stations. This may be an important step that will help stakeholders adopt a risk assessment plan, supporting the environmental monitoring studies, and allow for the rapid identification of outbreak sources during epidemiological investigations.
The original contributions presented in the study are included in the article/Supplementary Material, further inquiries can be directed to the corresponding author.
NS and ML conceived and designed the experiments. ML and BL provided all the resources for performing experiments. NS performed the experiments and analyzed the data. All authors drafted the work, revised it critically for important intellectual content, and final approval of the version to be published.
The authors declare that the research was conducted in the absence of any commercial or financial relationships that could be construed as a potential conflict of interest.
The authors are thankful to the Director General, TERI for providing infrastructural facilities and encouragement to execute the present study. The authors are also grateful to S. K. Dube for providing fruitful guidance.
The Supplementary Material for this article can be found online at: https://www.frontiersin.org/articles/10.3389/fenvs.2020.570904/full#supplementary-material
Altman, S. J., Jensen, R. P., Cappelle, M. A., and Sanchez, A. L. (2012). Membrane treatment of side-stream cooling tower water for reduction of water usage. Desalination 285, 177–183. doi: 10.1016/j.desal.2011.09.052
American Society for Testing and Materials [ASTM] (1995). Standard Methods for Chemical Oxygen Demand (Dichromate Oxygen Demand of Water D1252-95. Philadelphia, PA: ASTM.
Arthanareeswaran, G., Thanikaivelan, P., Srinivasn, K., and Mohan, D. (2004). Synthesis, characterization and thermal studies on cellulose acetate membranes with additive. Eur. Polym. J. 40, 2153–2159. doi: 10.1016/j.eurpolymj.2004.04.024
Bargellini, A., Marchesi, I., Righi, E., and Ferrari, A. (2011). Parameters predictive of Legionella contamination in hot water systems: association with trace elements and heterotrophic plate counts. Water 45, 2315–2321. doi: 10.1016/j.watres.2011.01.009
Bertelli, C., Courtois, S., Rosikiewicz, M., and Pirion, P. (2018). Reduced chlorine in drinking water distribution systems impacts bacterial biodiversity in biofilms. Front. Microbiol. 9:2520. doi: 10.3389/fmicb.2018.02520
Bott, T. R. (2009). Biofouling control in cooling water. Int. J. Chem. Eng. Res. 9, 1–4. doi: 10.1155/2009/619873
Burillo, A., Pedro-Botet, M. L., and Bouza, E. (2017). Microbiology and epidemiology of Legionnaire’s disease. Infect. Dis. Clin. N. Am. 31, 7–27.
Busalmen, J. P., Vazquez, M., and De Sanchez, S. R. (2002). New evidences on the catalase mechanism of microbial corrosion. Electrochim. Acta 47, 1857–1865. doi: 10.1016/s0013-4686(01)00899-4
Carvalho, F. R. S., Vazoller, R. F., Foronda, A. S., and Pellizari, V. H. (2007). Phylogenetic study of Legionella species in pristine and polluted aquatic samples from a tropical Atlantic forest ecosystem. Curr. Microbiol. 55, 288–293. doi: 10.1007/s00284-006-0589-1
Celik, E., Park, H., Choi, H., and Choi, H. (2011). Carbon nanotube blended polyethersulfone membranes for fouling control in water treatment. Water Res. 45, 274–282. doi: 10.1016/j.watres.2010.07.060
DiFilippo, M. N. (2004). Use of Produced Water in Recirculating Cooling Systems at Power Generating Facilities. Palo Alto, CA: National Energy Technology Laboratory.
Ditommaso, S., Ricciardi, E., Giacomuzzi, M., and Arauco, S. R. (2015). Legionella in water samples: how can you interpret the results obtained by quantitative PCR? Mol. Cell Prob. 29, 7–12. doi: 10.1016/j.mcp.2014.09.002
Duda, S. M. (2010). Biological Control in Cooling Water Systems Using Nonchemical Water Treatment Devices, Master’s thesis, University of Pittsburgh, Pittsburgh, PA.
Edagawa, A., Kimura, A., Doi, H., and Tanaka, H. (2008). Detection of culturable and nonculturable Legionella species from hot water systems of public buildings in Japan. J. Appl. Microbiol. 105, 2104–2114. doi: 10.1111/j.1365-2672.2008.03932.x
FeiTsao, H., Scheikl, U., Herbold, C., Indra, A., Walochnik, J., and Horn, M. (2019). The cooling tower water microbiota: Seasonal dynamics and co-occurrence of bacterial and protist phylotypes. Water Res. 159, 464–479. doi: 10.1016/j.watres.2019.04.028
Feng, Y. (2008). Management of Blowdown from Closed Loop Cooling Systems Using Impaired Waters. Master’s thesis, Renmin University of China, Beijing.
Gopiraman, M., Fujimor, K., Zeeshan, K., and Kim, B. S. (2013). Structural and mechanical properties of cellulose acetate/graphene hybrid nanofibers: spectroscopic investigations. Exp. Polym. Lett. 7, 554–563. doi: 10.3144/expresspolymlett.2013.52
Greub, G., and Raoult, D. (2004). Microorganisms resistant to free-living amoebae. Clin. Microbiol. Rev. 17, 413–433. doi: 10.1128/cmr.17.2.413-433.2004
Gubner, R., and Beech, I. B. (2000). The effect of extracellular polymeric substances on the attachment of Pseudomonas NCIMB 2021 to AISI 304 and 316 stainless steel. Biofouling 15, 25–36. doi: 10.1080/08927010009386295
Han, J., Kong, C., Heo, J., Yoon, Y., Lee, H., and Her, N. (2012). Removal of perchlorate using reverse osmosis and nanofiltration membranes. Environ. Eng. Res. 17, 185–190. doi: 10.4491/eer.2012.17.4.185
Hess, S. C., Kohll, A. X., Raso, R. A., and Schumacher, C. M. (2015). Template particle stabilized bicontinous emulsion yielding controlled assembly of hierarchical high flux filtration membrane. ACS Appl. Mater. Interf. 7, 611–617. doi: 10.1021/am506737n
Iervolino, M., Mancini, B., and Cristino, S. (2017). Industrial cooling tower disinfection treatment to prevent Legionella spp. Int. J. Environ. Res. Public Health 14:1125. doi: 10.3390/ijerph14101125
Jarraud, S., Descours, G., Ginevra, C., and Lina, G. (2012). Identification of Legionella in clinical samples. Methods Mol. Biol. 954, 27–56. doi: 10.1007/978-1-62703-161-5_2
Kaiser, W. J., Stark, N., and Grass, R. (2017). Rapid production of porous cellulose acetate membrane for water filtration using readily available chemicals. J. Chem. Educ. 94, 483–487. doi: 10.1021/acs.jchemed.6b00776
Kenny, J. F., Barber, N. L., Hutson, S. S., Linsey, K. S., Lovelace, J. K., and Maupin, M. A. (2009). Estimated Use of Water in the United States in 2005. Available online at: https://pubs.usgs.gov/circ/1344/pdf/c1344.pdf
Koeman-Stein, N. E., Creusen, R. J. M., Zijlstra, M., Groot, C. K., and van den Broek, W. B. P. (2016). Membrane distillation of industrial cooling tower blowdown water. Water Resour. Indust. 14, 11–17. doi: 10.1016/j.wri.2016.03.002
Krumholz, L. R., Wang, L., Beck, D. A. C., and Wang, T. (2013). Membrane protein complex of APS reductase and Qmo is present in Desulfovibrio vulgaris and Desulfovibrio alaskensis. Microbiology 159, 2162–2168. doi: 10.1099/mic.0.063818-0
Latzer, K. (2012). TechTalk. Available online at: http://www.chemtechwater.ca/attachments/Chemtech%20Sidestream%20Techtalk.pdf
Le, N. L., and Nunes, S. P. (2016). Materials and membrane technologies for water and energy sustainability. Sustain. Mater. Technol. 7, 1–28. doi: 10.1016/j.susmat.2016.02.001
Llewellyn, A. C., Lucas, C. E., Roberts, S. E., Brown, E. W., Nayak, B. S., and Raphael, B. H. (2017). Distribution of Legionella and bacterial community composition among regionally diverse US cooling towers. PLoS One 12:e0189937. doi: 10.1371/journal.pone.0189937
Morais, P. A., Rangel, A. O. S. S., and Souto, M. R. S. (2001). Determination of sulfate in natural and residual waters by turbidimetric flow-injection analysis. J. AOAC Int. 84, 59–64. doi: 10.1093/jaoac/84.1.59
Mouchtouri, V., Velonakis, E., Tsakalof, A., and Kapoula, C. (2007). Risk factors for contamination of hotel water distribution systems by Legionella species. Appl. Environ. Microbiol. 73, 1489–1492. doi: 10.1128/aem.02191-06
Mouchtouri, V. A., Goutziana, G., Kremastinou, J., and Hadjichristodoulou, C. (2010). Legionella species colonization in cooling towers: risk factors and assessment of control measures. Am. J. Infect. Control 38, 50–55. doi: 10.1016/j.ajic.2009.04.285
Paranjape, K., Bedard, E., Whyte, L. G., and Ronholm, J. (2019). Presence of Legionella spp. in cooling towers: the role of microbial diversity, Pseudomonas, and continuous chlorine application. bioRxiv [Preprint]. doi: 10.1101/540302
Parr, E., Whitney, A., and Berkelman, R. L. (2015). Legionellosis on the rise: a review of guidelines for prevention in the United States. J. Public Health Manag. Pract. 21, E17–E26.
Parthuisot, N., West, N. J., Lebaron, P., and Baudart, J. (2010). High diversity and abundance of Legionella spp. in a pristine river and impact of seasonal and anthropogenic effects. Appl. Environ. Microbiol. 76, 8201–8210. doi: 10.1128/aem.00188-10
Samimi, A. (2013). Micro-organisms of cooling tower problems and how to manage them. Int. J. Basic Appl. Sci. 1, 705–715.
Shannon, M. A., Bohn, P. W., Elimelech, M., and Georgiadis, J. G. (2008). Science and Technology for water purification in the coming decades. Nature 45, 2301–2310.
Sharma, C., Sharma, A. K., Mullick, S. C., and Kandpal, T. C. (2015). Assessment of solar thermal power generation potential in India. Renewable and Sustainable Energy Reviews 42, 902–912. doi: 10.1016/j.rser.2014.10.059
Sharma, N., Lavania, M., Kukreti, V., and Rana, D. P. (2018). Laboratory investigation of indigenous consortia TERIJ-188 for incremental oil recovery. Front. Microbiol. 8:2357. doi: 10.3389/fmicb.2018.2357
Sharma, N., Lavania, M., and Lal, B. (2019). Distribution of pathogenic Legionella species in the cooling towers: a possible cause of Legionnaire’s disease. Biomed. J. Sci. Tech. Res. 21, 15820–15823.
Sharmin, F., Wakelin, S., Huygens, F., and Hargreaves, M. (2013). Firmicutes dominate the bacterial taxa within sugar-cane processing plants. Sci. Rep. 3:3107.
Shukla, M., and Arya, S. (2018). Determination of chloride ion (Cl-1) concentration in Ganga river water by mohr method at Kanpur, India. Green Chem. Tech. Lett. 4, 06–08. doi: 10.18510/gctl.2018.412
Subbaram, K., Kannan, H., and Masadeh, M. M. A. (2017). Isolation, identification, characterization and antibiotic sensitivity profile of pathogenic Legionella pneumophila isolates from different water sources Asian Pac. Asian Pac. J. Trop. Biomed. 7, 411–415. doi: 10.1016/j.apjtb.2016.12.023
Thomas, V., McDonnell, G., Denyer, S. P., and Maillard, J. Y. (2010). Free-living amoebae and their intracellular pathogenic microorganisms: risks for water quality. FEMS Microbiol. Rev. 34, 231–259. doi: 10.1111/j.1574-6976.2009.00190.x
Walser, S. M., Gerstner, D. G., Brenner, B., Holler, C., Liebl, B., and Herr, C. E. W. (2014). Assessing the environmental health relevance of cooling towers–a systematic review of legionellosis outbreaks. Int. J. Hyg. Environ. Health 217, 145–154. doi: 10.1016/j.ijheh.2013.08.002
Wery, N., Bru-Adan, V., Minervini, C., Delgenes, J. P., Garrelly, L., and Godon, J. J. (2008). Dynamics of Legionella spp. and bacterial populations during the proliferation of L. pneumophila in a cooling tower facility. Appl. Environ. Microbiol. 74, 3030–3037. doi: 10.1128/aem.02760-07
Keywords: APS reductase analysis, conductivity (mixed), Legionella sp., FTIR, cooling towers, membranes
Citation: Sharma N, Lavania M and Lal B (2020) Development and Demonstration of Membrane to Control Potential Pathogen (Legionella sp.) Associated With Cooling Towers. Front. Environ. Sci. 8:570904. doi: 10.3389/fenvs.2020.570904
Received: 05 August 2020; Accepted: 24 September 2020;
Published: 14 October 2020.
Edited by:
Michael Gormley, Heriot-Watt University, United KingdomReviewed by:
Constantinos V. Chrysikopoulos, Technical University of Crete, GreeceCopyright © 2020 Sharma, Lavania and Lal. This is an open-access article distributed under the terms of the Creative Commons Attribution License (CC BY). The use, distribution or reproduction in other forums is permitted, provided the original author(s) and the copyright owner(s) are credited and that the original publication in this journal is cited, in accordance with accepted academic practice. No use, distribution or reproduction is permitted which does not comply with these terms.
*Correspondence: Meeta Lavania, bWVldGFsQHRlcmkucmVzLmlu
Disclaimer: All claims expressed in this article are solely those of the authors and do not necessarily represent those of their affiliated organizations, or those of the publisher, the editors and the reviewers. Any product that may be evaluated in this article or claim that may be made by its manufacturer is not guaranteed or endorsed by the publisher.
Research integrity at Frontiers
Learn more about the work of our research integrity team to safeguard the quality of each article we publish.