- Australian Institute of Marine Science (AIMS), Townsville, QLD, Australia
Given current concerns regarding the extent of microplastic contamination in the environment, routine monitoring for microplastics in biological tissues is becoming increasingly common place. However, complex sample matrices, such as lipid-rich tissues, require multiple pre-treatment steps which may lead to increased sample processing time and costs, and a reduction in microplastic recovery rates thereby hindering monitoring efforts. Lipid-rich (fat) tissues often pose difficulties for traditional potassium hydroxide (KOH) digestion methods due to saponification. This reaction produces a suspension of glycerol and fatty acids (soaps), which may entrap microplastics inhibiting their recovery and clog filters thus reducing the efficiency of the filtration or inhibiting it altogether. In this study, the incorporation of 100% ethanol (EtOH) to existing KOH digestion methods was found to completely redissolve the viscous saponified gel formed in these reactions, with a digestion efficiency greater than 97% for all treated lipid-rich tissue samples. Recovery of spiked polyethylene and polystyrene fragments, and rayon and polyester fibers, ranged from 93% to 100%. The addition of EtOH did not induce physical or chemical degradation on these polymers. The inclusion of an ad hoc decision-making tool within the digestion workflow reduced pre-processing time for samples and allowed for solid saponified samples to be completely redissolved. This validated workflow facilitates high through-put sampling of biota, by enabling lipid-rich tissues to be filtered with a high degree of efficiency thereby successfully separating microplastics from their gelatinous matrix.
Introduction
Once considered an emerging contaminant, microplastics (MPs; a heterogenous mixture of plastic particles <5 mm), have proliferated in the global marine environment (Akdogan and Guven, 2019). As such reliable and regular monitoring for marine MPs is now advocated by many national and international agencies (Lusher et al., 2017a; GESAMP, 2019) to better understand the extent, sources, fate and impacts of contamination throughout a diverse array of marine ecosystems. Typical matrices of interest for these monitoring programs include seawater, sediments and marine biota.
A variety of methods have been developed to separate and identify MPs present in these environmental matrices (Miller et al., 2017). In general, for marine biota, the most common method to verify MP intake is to dissect the gastrointestinal tract (GIT) and chemically digest the tissue, leaving only indigestible remains, including putative MPs (Lusher et al., 2017b; Hermsen et al., 2018; GESAMP, 2019). Alkali tissue digestions using potassium hydroxide (KOH) are recommended as the ideal method to dissolve tissue and retain the integrity of ingested microplastics (GESAMP, 2019). Notably, KOH has minimal impact on most common polymer types under ambient to moderate temperatures (<40°C) for short periods (<2 weeks) (Dehaut et al., 2016; Karami et al., 2017), is inexpensive and is low risk to laboratory users. As such, KOH has been utilized in many MP studies for multiple marine organisms (Lusher et al., 2017b), but most commonly for fish. Further, many studies have sought to optimize KOH digestions to increase digestion efficiency whilst decreasing reaction times (e.g., Dehaut et al., 2016; Karami et al., 2017; Kühn et al., 2017). Digestion periods typically range from 24 hr (Dehaut et al., 2016) up to 2–3 weeks (Foekema et al., 2013; Munno et al., 2018), with tested reaction temperatures ranging from 18°C (Kühn et al., 2017) through to 60°C (Dehaut et al., 2016; Karami et al., 2017; Munno et al., 2018). If significant indigestible material remains following the KOH digestion step, the solution can be further clarified with a density separation (Coppock et al., 2017; Karami et al., 2017; Herrera et al., 2018). The solution is then passed through a suitable pore-sized filter to isolate the intact putative MPs for subsequent physical and chemical analysis (Hermsen et al., 2018; Lusher and Hernandez-Milian, 2018).
Marine biota with a high lipid (fat) content pose a challenge for separation of MPs from their tissues. Fatty tissues can be excised from the GIT prior to MP separation (e.g., Caron et al., 2018a), however, this lipid-rich tissue may itself be of interest for examination, such as liver tissue (e.g., Collard et al., 2017). Lipids in the presence of an aqueous alkali salt undergo a saponification reaction to form soap (Kent, 2003; Mabrouk, 2005). Sodium-based salts form a hard soap while potassium-based salts form soft (liquid suspension) soaps (Konkol and Rasmussen, 2015). In a typical 10% aqueous KOH digestion, saponification will occur if the lipid content is sufficiently high within the solution, even at room temperature. This has led to some authors suggesting that 10% aqueous KOH digestions should not be used on samples with high lipid content or with large fat deposits (Bessa et al., 2019).
This study optimized the alkali KOH digestion method to facilitate recovery of MPs from lipid-rich tissues prone to saponification. There are many variations of the KOH digestion method reported throughout the literature, with digestion time and temperatures being the primary parameters considered (Dehaut et al., 2016; Kühn et al., 2017). In this study, two digestion methods, one using room temperature (Foekema et al., 2013) and the other using 40°C heating (Karami et al., 2017), were optimized for lipid-rich fish tissue. Ethanol (EtOH) is a versatile polar solvent capable of dissolving polar (due to its hydroxyl group) and many non-polar (due to its ethyl group) substances, but has been shown to have minimal impact on MPs (Courtene-Jones et al., 2017; Herrera et al., 2018). Therefore, a simple treatment with EtOH post-KOH digestion was investigated as a means of dispersing and redissolving the resultant gelatinous soap suspension. Specifically, the objectives were to design a suitable workflow to expedite the recovery of MPs from lipid-rich saponified biota samples. The workflow was optimized using farmed fish due to their fatty composition and regulated homogenous diet, and then applied to wild caught fish with unknown lipid content and multifarious diet. Both wild and farmed fish are regularly consumed by humans globally and are of particular interest for studies evaluating MPs in human seafood.
Materials and Methods
Materials
Potassium Hydroxide pellets (Thermo Fisher Scientific UNILAB CAS No. 1310-58-3) were dissolved with Milli-Q (Millipore) water to form a 10% w/v aqueous solution. Absolute ethanol AR grade (Thermo Fisher Scientific CAS No. 64-17-5) was used undiluted. Stainless steel (316 grade) filters (547 μm pore size - 55 mm Ø, Plain Weave; 263 μm pore size - 19 mm Ø, Plain Weave; and 26 μm pore size - 19 mm Ø; Twill Weave) were punched from woven mesh. Polypropylene filters (30 μm - 47 mm Ø Millipore) were sourced from Merck.
Irregular polyethylene (PE) particles were cut into nominal 1 mm lengths from a green laboratory rope using a scalpel. Irregular polystyrene (PS) particles (1–2 mm) were purchased from Sigma Aldrich. Polyester (PES) fibers (1–5 mm) were abraded from a fluorescent safety vest using commercial sandpaper. Commercial rayon thread (Gütermann CA 02776) was cut into nominal 1 mm lengths and individual fibers were then teased apart. All polymers had their chemical composition confirmed using Fourier Transform Infrared Spectroscopy (FTIR) with a PerkinElmer Spectrum 100 universal ATR (16 scans at 4 cm–1 resolution, wavenumber range = 4000–600 cm–1). Spectra were searched against a commercial library (NICODOM) following Kroon et al. (2018).
Lipid-Rich Fish Tissue
Local fish shops provided adult farmed barramundi (Lates calcarifer) samples for analysis. These were either viscera removed from the fish frame (n = 3), or whole fish frames with viscera intact (n = 6). Frames and viscera were transported to the laboratory then frozen at −20°C for storage until processing. Samples were defrosted at ambient room temperature (RT; ∼22°C) and fish frames were dissected to extract the viscera. The swim bladder was removed from all samples. Viscera were then either analyzed whole (n = 6) (Experiment A and C) or dissected further to separate the stomach and intestine (n = 3) (Experiment B). Visual assessment of Experiment C samples revealed they contained extremely large solid lipid deposits when compared with samples prepared for Experiment A and B. Therefore, some deposits that were not well attached to the viscera were removed to ensure samples were comparable between the three experiments (see Supplementary Figure S1). All tissues were weighed individually and placed in glass vessels for digestion.
KOH Digestion of Lipid-Rich Fish Tissues
To examine the applicability of EtOH resuspension to both RT and elevated temperature (40°C) digestion methods, two different aqueous KOH digestions were conducted (Table 1). For the RT method (Foekema et al., 2013), the whole viscera (Experiment A), or the individual stomach and intestine (Experiment B), were digested with 10% (w/v) KOH solution with a final concentration of 1:4 (tissue weight/KOH volume) (Supplementary Table S1) at ambient RT for 14 days. In Experiment B, the stomach and intestine from each sample were analyzed separately. Sample vessels (1 L glass Schott bottles or a 1 L beaker) were gently swirled by hand once a week throughout the digestion period.
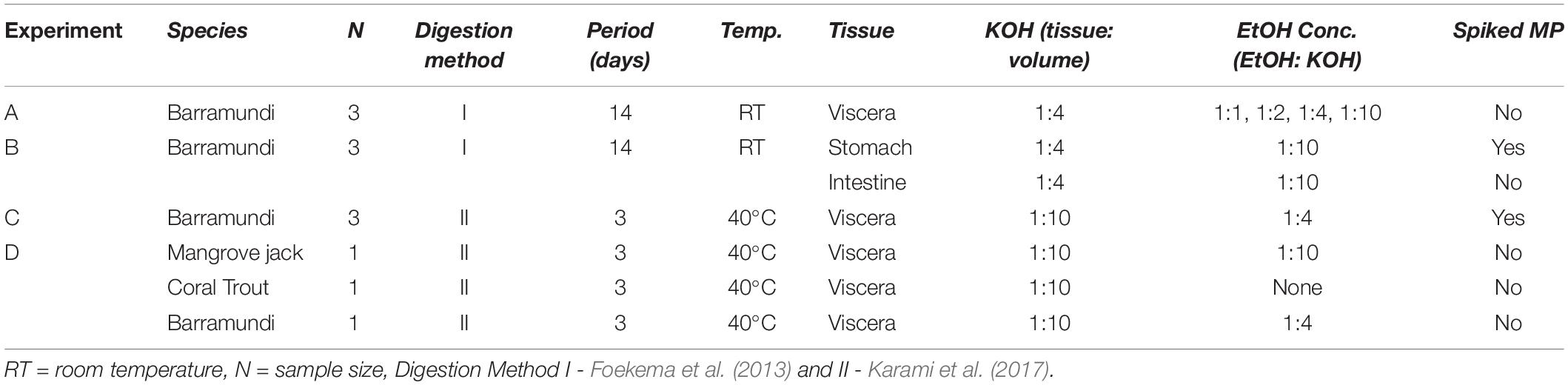
Table 1. Methodological approach used to optimize potassium hydroxide (KOH) digestion of lipid-rich fish tissues and recovery of spiked microplastics.
For the elevated temperature method (Karami et al., 2017) (Experiment C), viscera were digested in 10% (w/v) KOH solution with a final concentration of 1:10 (tissue weight/KOH volume) and incubated at 40°C for 3 days in an Innova 40 benchtop incubator. Sample vessels (3 L glass volumetric flasks) were gently swirled by hand after 48 h.
Treatment of Saponified Solutions
Lipids in the presence of KOH undergo saponification to form a soft weak gel (Kent, 2003) that exhibits no flow when in the steady-state (Ferry, 1980). All samples from Experiment A, B and C were visually inspected at the beginning and midway through the digestion period for the formation of a viscous soft gel at the solution’s surface, signifying saponification. Experiments A, B and C were performed sequentially, with the results and general observations influencing the design of the subsequent experiments. For each of the three experiments, the methods used to redissolve the gel were optimized (Figure 1), then incorporated into a generic workflow applicable to any lipid-rich tissue sample.
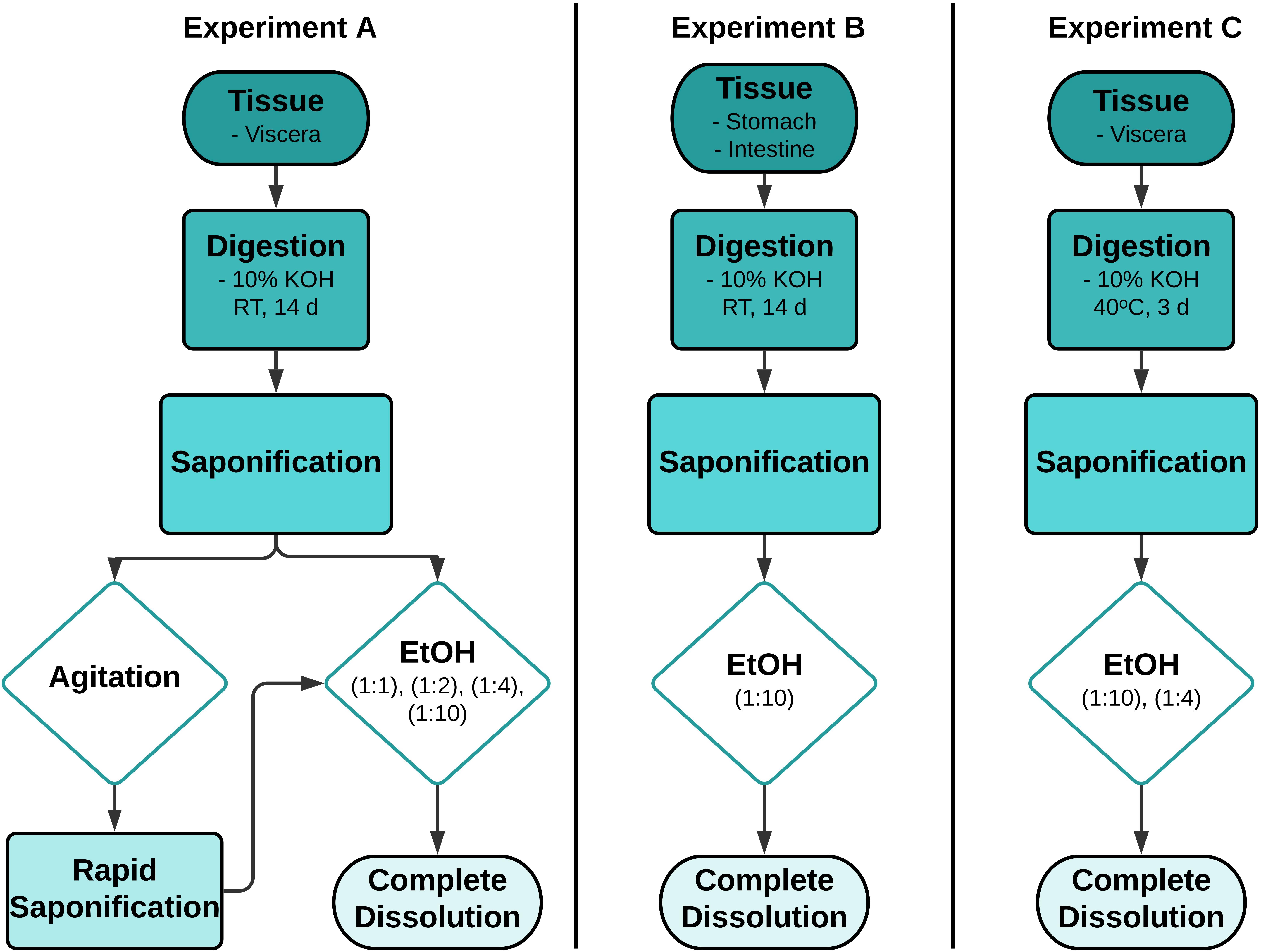
Figure 1. Workflows outlining the process of optimizing the treatment of 10% potassium hydroxide (KOH) digested lipid-rich fish tissues. RT -room temperature, EtOH - ethanol, d- day, solvent ratios - 100% EtOH: Biota/KOH solution, v/v.
The objective of Experiment A was to establish a suitable method to redissolve saponified gel present in lipid-rich samples whilst still achieving high digestion efficiencies (> 95%), typical of 10% KOH digestions where saponification does not occur. For samples presenting with a viscous gel, two methods of gel resuspension were tested sequentially. First, samples were vigorously agitated by stirring with a glass rod in an effort to mechanically breakdown the gel. However, this process proved counterproductive to the desired result and was discontinued. EtOH treatments post-KOH digestion were then examined as a means of redissolving the resultant soap (Table 1). To determine the lowest possible concentration of EtOH needed to achieve complete resuspension of the viscous gel, 5 subsamples (approximately 5 ml) of gel were taken from each sample. Subsamples were diluted with either a 0 (control), 1:1, 1:2, 1:4, or 1:10 volume of EtOH (100% EtOH/gel subsample, v/v), respectively. After adding the EtOH, subsamples were stirred with a glass rod until dispersed or up to 1 min at RT, then visually assessed for the presence of a homogenous liquid, indicating resuspension. Subsamples were then vacuum filtered through a 26 μm stainless steel filter. The control subsamples were not treated with EtOH before filtration. The remainder of each of the three samples was then treated with the lowest EtOH concentration required to achieve redissolution, stirred briefly and filtered, sequentially through a number of pre-weighted stainless steel filters to determine digestion efficiency, as described below.
Experiment B was designed to assess whether a reduction in sample weight, by removal of non-target tissue and solid lipid deposits prior to digestion, prevented or reduced the likelihood of saponification. Therefore, all non-target tissue surrounding the GIT was excised and only the individual stomach and individual intestines were digested separately with KOH as described above. The EtOH concentration, determined from Experiment A, was added to each of the samples 24 h before termination of the reaction. For these samples EtOH was not stirred with a glass rod, but the vessel was swirled gently by hand for <1 min to evenly distribute the EtOH. After 24 h, the suspension was then filtered to determine digestion efficiency, as described below.
Experiment C was designed to examine whether EtOH treatment could successfully redissolve saponified samples generated during a 10% KOH digestion conducted at elevated temperatures. Viscera were digested at 40°C for 3 days. The EtOH concentration determined from Experiment A was added to the digestion solution 1 h before termination of the reaction. The vessels were gently swirled by hand for <1 min to circulate the EtOH, then left stationary for the remainder of digestion period. The resuspended solution was then filtered to determine digestion efficiency, as described below.
Digestion Efficiency
All samples treated with 100% EtOH were filtered sequentially onto 547 μm (55 mm Ø), 263 and 26 μm (19 mm Ø) pre-weighed stainless steel filters. Using a Teflon wash bottle, these were then flushed liberally and sequentially with Milli-Q water and 80% EtOH, to reduce the possibility of particulates adhering to the sides of the funnel and to remove any chemical residue. Filters and the filtration system were flushed at least 3x or until there were no particulates adhering to the system. Filters were dried overnight in an oven at 40°C then stored in a desiccator, thereafter weights were recorded to calculate the digestion efficiency (Equation 1).
where Wi = Initial weight of lipid-rich materials (w.w), Wa = Weight of dry filter membrane after filtration (d.w), and Wb = Weight of dry filter membrane before filtration (d.w).
The initial sample weight was measured with a Sartorius TE3102S balance (2 d.p). Filter weights before and after filtration were measured with a Sartorius CP224S balance (4 d.p). The samples were allowed to stabilize on the balance before recording the final weight.
Effect of KOH and EtOH on Microplastics
Model MPs were exposed to KOH alone or combined KOH and EtOH to examine whether one or both reagents would significantly degrade polymers, potentially inhibiting recovery and identification. To account for any KOH-induced degradation of the spiked polymers, MPs were exposed to 10% KOH for 14 days at RT or 10% KOH for 3 days at 40°C, in the absence of biological tissue and EtOH. Model MPs comprised of 10 black rayon fibers, 10 green PE fragments, 10 transparent PS fragments and 10 yellow PES fibers.
Lipid-rich tissues from Experiment B and C were spiked with model MPs (Table 1) to investigate whether the additional EtOH treatments would result in MP degradation when combined with KOH. Individual barramundi stomachs (Experiment B) were spiked with 5 black rayon fibers, 5 transparent PS irregular fragments and 5 green PE irregular fragments. Additionally, Experiment C viscera were also spiked with 5 yellow PES fibers per sample as PES fibers are known to degrade following KOH digestion at elevated temperatures, particularly above 60°C (Karami et al., 2017).
Following exposure, all samples were vacuum filtered onto stainless steel filters and retained MPs were assessed for physical and chemical changes by comparing color, shape and infrared spectral profiles with non-treated virgin MPs. MPs were visually identified using a Leica Stereo microscope MZ16A. Polymer spectra of virgin (n = 3) and exposed particles were acquired using ATR-FTIR. Spectral comparisons were conducted using the COMPARE analysis in the PerkinElmer Spectrum IR software (Kroon et al., 2018). Further, carbonyl indices for both non-treated virgin MPs and MPs exposed to KOH and EtOH (Experiment B and C) were compared (A detailed description of the spectral analysis is provided in Supplementary Material).
Establishing a Treatment Workflow
Results obtained throughout Experiments A, B and C were used to develop a tailored workflow to ameliorate the digestion of lipid-rich tissues. The workflow utilized the following three criteria to establish the complete dissolution of the gel post EtOH treatment: (i) solution appeared homogenous (see Table 2 for guidance in visual assessment), (ii) ability of the treated sample to pass through a 26 μm pore size stainless steel filter in <5 min, and (iii) a digestion efficiency of equal efficacy to KOH digestion without saponification (i.e., >95%). The criteria used to verify the workflow were: (iv) recovery rates of spiked MPs above 90%, and (v) no EtOH-induced changes in physical or chemical characteristics in recovered MPs.
Application of the Workflow: Case Study Using Wild Caught Fish (Experiment D)
The tailored workflow, based on the results of Experiment A, B, and C, was validated using wild fish (Figure 2). Wild fish were hypothesized to be less fatty than farmed fish and were used to determine if the workflow was suitable for analyzing fish with variable and unknown lipid content. The viscera of coral trout (Plectropomus leopardus), barramundi (Lates calcarifer), and mangrove jack (Lutjanus argentimaculatus), were obtained from fish shops or provided by local fishers from Townsville, Australia.
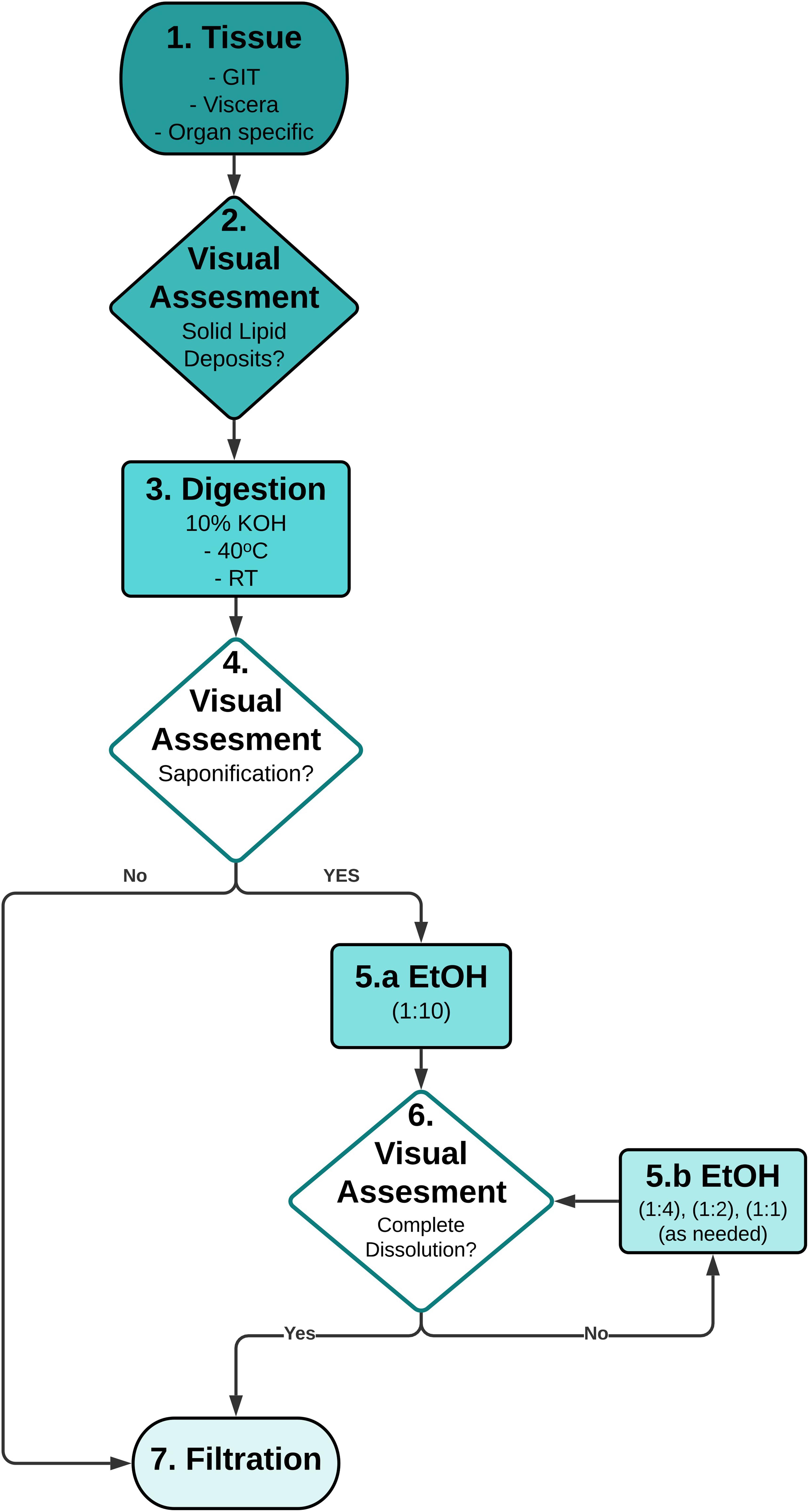
Figure 2. Recommended workflow for dissolution of the gel formed in saponified potassium hydroxide (KOH) digestions. 1. Method is applicable to any desired fish tissue; 2. Optional: Removal of any excess solid lipid (fat) deposits attached to the tissue is recommended to reduce KOH/EtOH reaction volume; 3. Method is valid for both ambient and elevated temperature (≤40°C) KOH digestions; 4. Stage-gate using visual assessment (Table 2) to process sample via filtration, or if saponification has occurred to treat with EtOH; 5a. Lowest concentration of EtOH is added; 6. Stage-gate using visual assessment to process homogenous solution via filtration, or if gel is still evident treat with EtOH; 5b. Sequentially add excess EtOH to dissolve the gel. 7. Filtration of homogeneous solution over suitable pore size filters to retain microplastics. GIT – gastrointestinal tract, EtOH – ethanol, RT - room temperature, solvent ratios - 100% EtOH: Biota/KOH solution, v/v.
Viscera was digested in 10% KOH as per Experiment C (3 days at 40°C) in glass Schott bottles. One hour before termination of the digestion, the reaction solution was visually assessed and if a solid gel had developed (Table 2), was processed using the tailored workflow. Using this workflow, EtOH was added to samples with a saponified gel in 1:10 EtOH:KOH ratio. After gently swirling by hand, the samples were returned to the incubator for 1 h. If the gel was visually assessed as having completely dissolved, it was filtered as described above, if not, then additional EtOH was added to achieve a concentration of 1:4 EtOH:KOH. The samples were gently swirled, then visually assessed again. If necessary, the process was repeated using a 1:2 or a 1:1 concentration of EtOH, until the solution presented as a homogenous liquid. Only after complete resuspension was achieved, based on visual assessment, was the sample filtered to assess digestion efficiency.
Copious amounts of prey items and sediment were present in the wild fish GIT, which was visible as a thick layer of sediment in the bottom of the Schott bottle. Thus, these were filtered onto larger 47 mm Ø filters (30 μm pore size). The increased surface area accommodated a larger retentate load before clogging. Digestion efficiency was a calculated for these samples as outlined above.
QA/QC
The objective of this study was to assess recovery of spiked polymers, and not to quantify environmental MP burdens. Therefore, although dissection and analyses followed recommended clean laboratory procedures to prevent laboratory contamination, procedural blanks were not collected. These procedures included wearing a 100% cotton lab coat, cleaning glassware and utensils with detergent (if needed) and Milli-Q water before use and between samples, the use of plastic utensils was avoided, laboratory benches were cleaned with 70% EtOH and samples were kept covered with aluminum foil (Lusher and Hernandez-Milian, 2018). Samples were not processed inside a clean air device (Wesch et al., 2017). To apply this workflow to environmental samples, it is recommended to follow established QA/QC method, such as those described in Karami et al. (2017), Bråte et al. (2018), or Hermsen et al. (2018).
Results
Ethanol Workflow for Resuspension of Saponified Fish Fat
All farmed barramundi tissue subjected to 10% KOH digestion resulted in the formation of a viscous gel, which visually resembled gelatin (jelly) (Table 2 and Figure 3). These saponified samples (Experiment A: viscera; Experiment B: stomach/intestines; Experiment C: viscera) could not be easily poured from the glass vessels in this state.
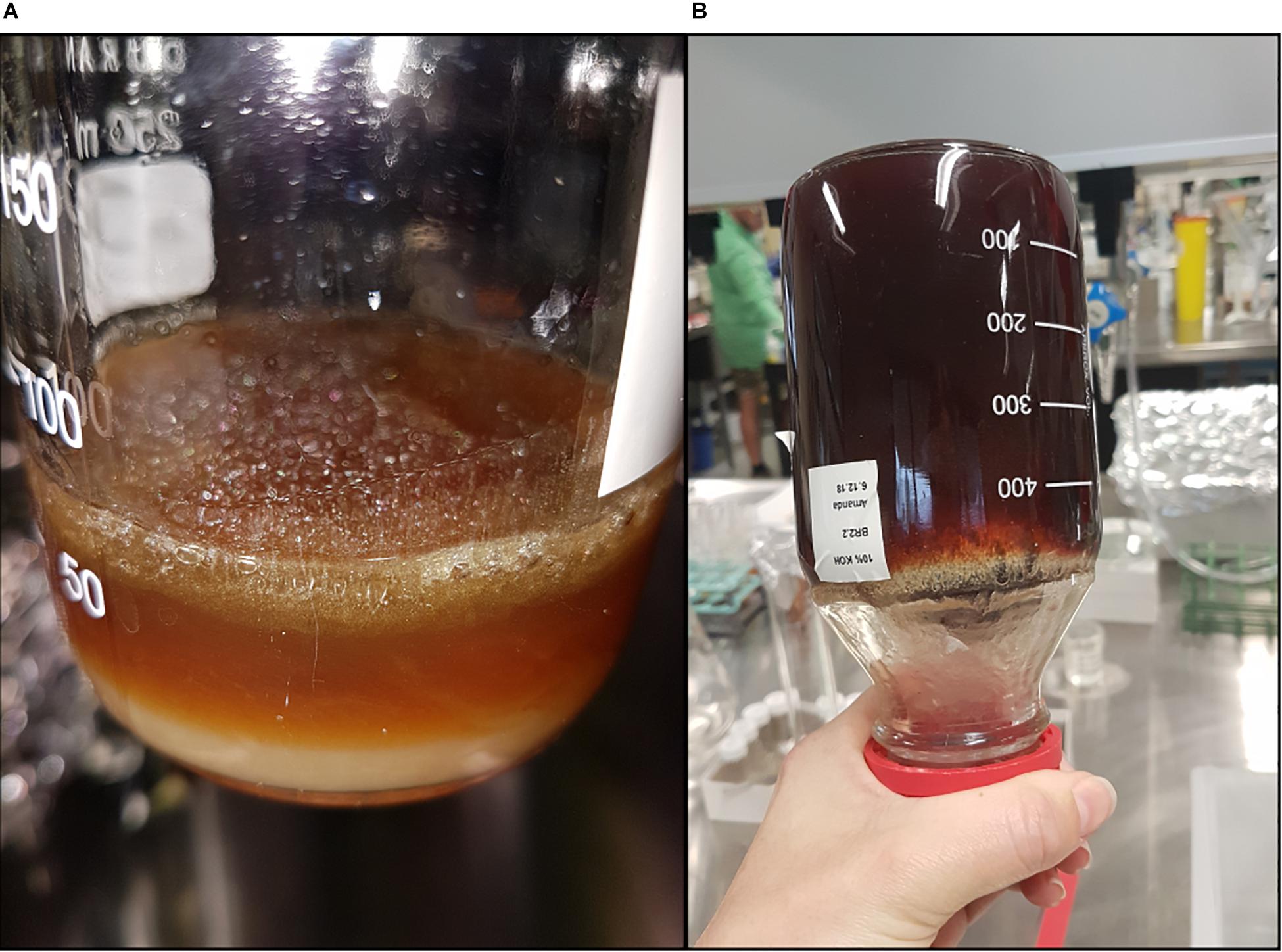
Figure 3. Saponified viscera samples of barramundi (Lates calcarifer) (Experiment A) after treatment with 10% potassium hydroxide (KOH) at ambient room temperature (∼22°C) for 14 days, (A) sample with a single layer of gel floating atop a liquid layer, gel is approximately 0.5 cm thick, and (B) entire sample (∼ 500 mL) has saponified due to agitation and formed a solid soft gel.
In Experiment A, agitation in the form of shaking and inverting, resulted in the gel breaking into smaller clumps, however, it could not be redissolved. Further, the gel layer reformed shortly after shaking ceased. Continued vigorous stirring with a clean glass rod induced an extremely rapid saponification reaction, with the physical properties of the entire sample changing from a biphasic mixture (lower liquid layer, and upper floating gel), to a homogenous thick gel (Figure 3 and Supplementary Figure S2), which could not be poured, nor broken apart with vigorous shaking. Attempted vacuum filtration of the control (untreated) subsample through a 26 μm filter was unsuccessful, thus no further analysis of untreated saponified samples was carried out. Treatment of the gel subsamples with EtOH (1:1, 1:2, 1:4, and 1:10) resulted in complete dissolution, with all resulting solutions able to pass through the 26 μm filter, with only minimal gelatinous residue being retained. Thus, the lowest dilution, 1:10, was selected to redissolve the remainder of the samples from Experiment A, and for Experiments B and C.
In Experiment B, the complete removal of the stomach and intestines from lipid-rich organs (e.g., liver) and solid lipid deposits did not prevent saponification. The individual stomachs and intestines all presented with floating soft weak gels when digested with KOH. The samples were treated with 1:10 EtOH and visual assessment determined that gel was redissolved. As dissection was not an effective method of preventing or reducing the level of saponification, complete viscera were used in all following tests to reduce sample preparation time.
The formation of a solid gel following KOH digestion was also observed in Experiment C (viscera 1:10 tissue:KOH (w/v) 40°C) (Figure 4A). Visual assessment revealed this solid gel did not completely dissolve after initially adding EtOH (1:10). Increasing the volume of EtOH (1:4) was successful in redissolving the remaining gel and allowed for filtration without any gel residue (Figure 4B).
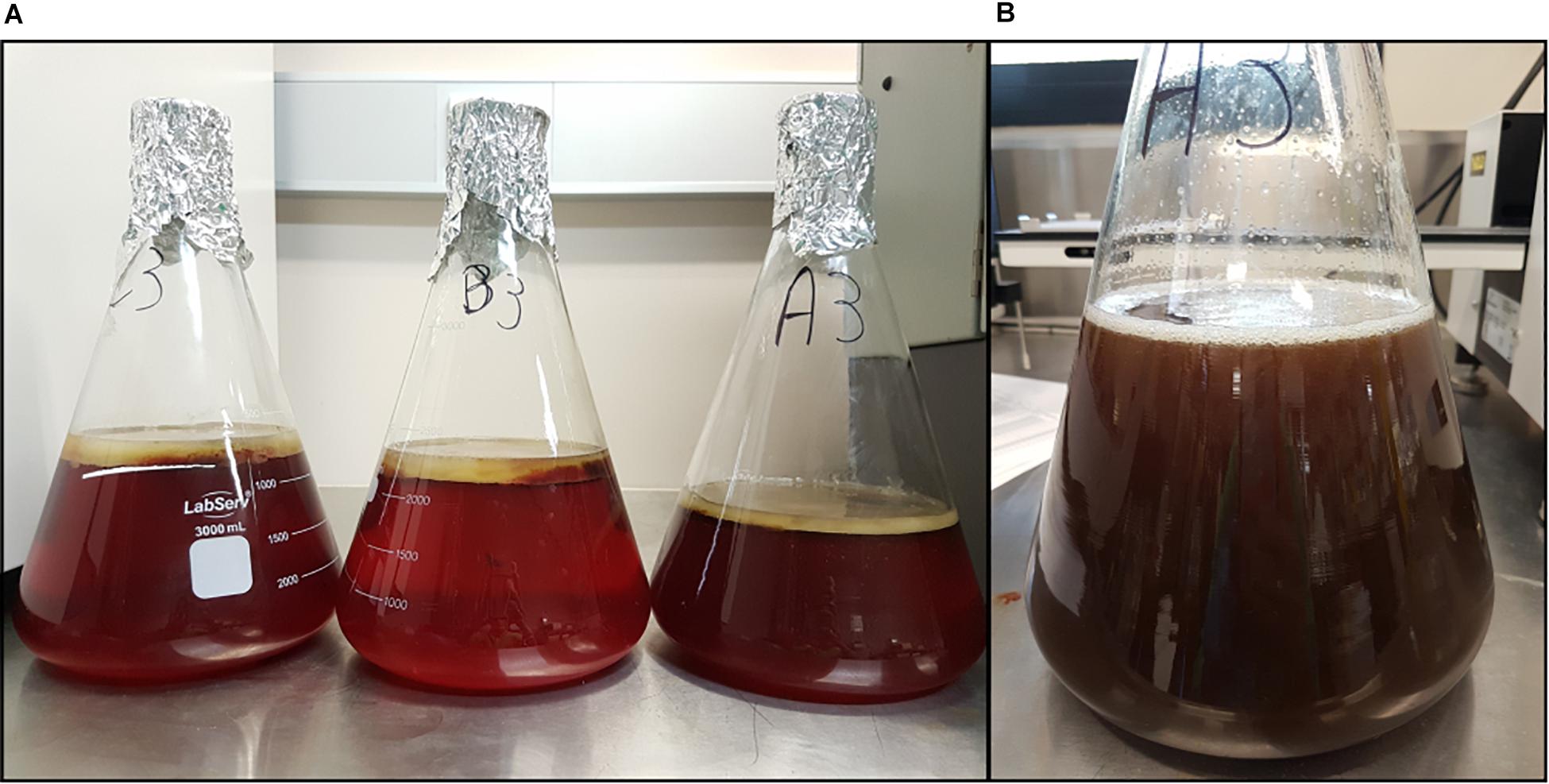
Figure 4. Saponified and resuspended samples of barramundi (Lates calcarifer) viscera (Experiment C) after digestion with 10% potassium hydroxide (KOH) at 40°C for 3 days, (A) samples showing the formation of a white gel floating on top of the KOH digested tissue (brown underlaying liquid), gel is approximately 2 cm thick, and (B) the same sample after the addition of ethanol (1:4), the floating gel is completely resuspended.
The results of Experiment A, B and C demonstrated that an ad hoc visual assessment of the physical appearance of the sample (Table 2) was a simple and effective method to establish the presence of saponified gels. Visual inspection was also effective at verifying dissolution, with all samples that were judged redissolved able to pass through a 26 μm filter after EtOH treatment. Hence, visual assessment combined with EtOH treatment was applied in the wild fish case study (Experiment D).
Inspection of wild-caught coral trout viscera found it to be relatively free of fat deposits. Digestion of this tissue under Experiment D conditions yielded a clear solution with no obvious saponified gel observed. As a result, addition of EtOH was deemed unnecessary and the sample was filtered as is. Wild-caught mangrove jack and barramundi viscera were observed to have solid fat deposits (although notably less than farmed barramundi, Supplementary Figure S3). Digestion with 10% KOH formed a viscous gel, therefore both samples were further treated with EtOH (1:10, as per the tailored workflow). Gel-like solids in the mangrove jack sample dissolved completely resulting in a clarified solution that was easily filtered. Treatment of the wild-caught barramundi sample with EtOH (1:10) resulted in only partial dissolution of the gel, as was also noted in Experiment C. Increasing the volume of EtOH to 1:4 redissolved the remaining gel, and the solution was filtered.
Digestion Efficiency
The digestion efficiency, notwithstanding the limited sample replication, for all farmed barramundi (Experiment A, B, and C) was greater than 99% (Table 3). Very little prey and sediment residue was present on the filters for these samples (Supplementary Figure S4). Conversely, the wild-caught fish (Experiment D) contained substantial prey items in their GIT, including whole animals as well as bones, crustacean exoskeletons, shells and algae, all of which were retained on the filter. Sediments were also observed in the digested viscera samples obtained from mangrove jack and barramundi. Thus, viscera samples from wild fish required multiple large (47 mm Ø; 30 μm pore size) filters to prevent clogging (Supplementary Figure S5). Although the digestion efficiency was still considered acceptable for fish tissues (>97%), and the gel was able to be resuspended by the EtOH, the abundance of material remaining on the filter would impede visual examination of the digestate for MPs.
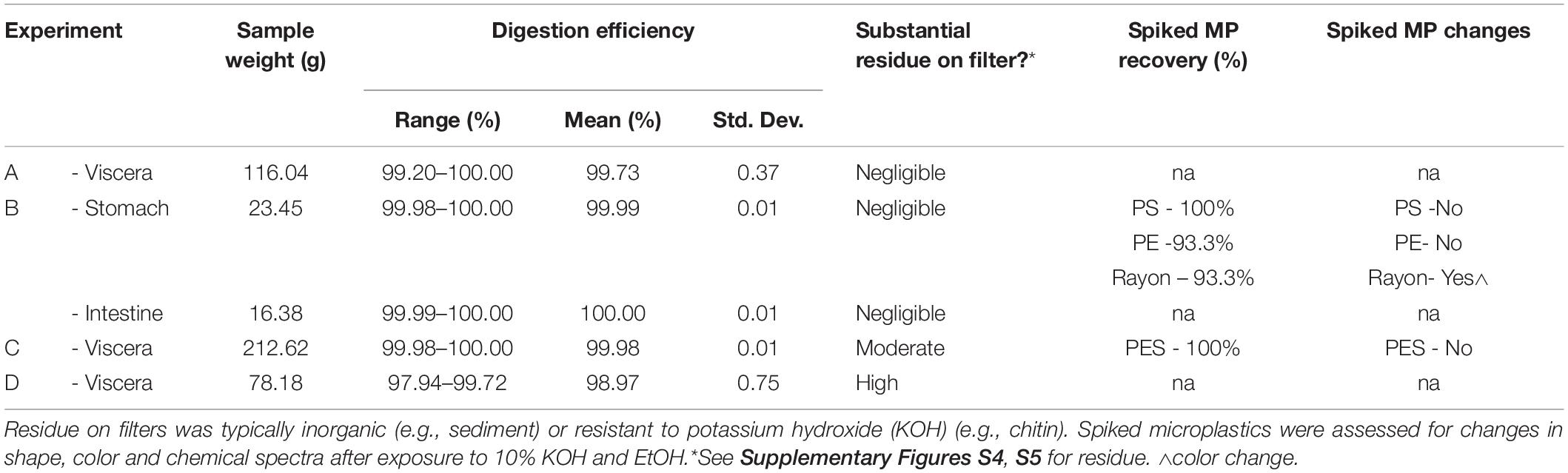
Table 3. The digestion efficiency and recovery of spiked MPs (polyethylene PE, polystyrene PS, polyester PES and rayon) in the four Experiments (A–D).
Effect of KOH and EtOH on Microplastics
Examination of the MPs exposed to 10% KOH for 14 days at RT found there was no change in color or shape of PS or PE. However, black rayon fibers were bleached and changed from opaque to transparent. Chemical analysis using FTIR found no substantial changes in the spectral profiles compared to virgin plastics (PE – 99.0%, PS- 98.7%, Rayon – 94.9.0% match). Despite a slightly lower % match for rayon, it was still above the accepted similarity threshold of > 70% (Lusher et al., 2013; Kroon et al., 2018). Exposure to 10% KOH at 40°C for 3 days also did not result in significant changes in the chemical spectra for the four polymers (PE – 98.5%, PS- 99.6%, Rayon – 97.9%, PES – 97.6% match). There were no changes in color and shape, apart from rayon, which underwent similar color changes as per the RT KOH exposure.
Recovery of spiked MPs from KOH digested and EtOH treated barramundi tissue was 100% for PS particles, 93.3% for PE particles, 93.3% for rayon fibers, and 100% for PES fibers (Table 3). All spiked samples that produced saponified gels were treated with EtOH. Thus, there were no attempts to recover MPs directly from the gel itself. For all MPs, shape was unchanged after the combined KOH and EtOH exposure. Color was also unaffected by the combined exposure for PS, PE and PES. However, rayon fibers became transparent, likely due to KOH exposure prior to the addition of EtOH (Table 3). Spectral analysis revealed no major change in profile compared to MPs exposed to KOH only. All polymer spectra returned correlations > 90% with the virgin MP after the combined KOH and EtOH exposure (PE – 98.6%, PS- 99.3%, Rayon – 97.1%, PES - 98.0% (Supplementary Table S2). Although not all recovered MPs were able to be FTIR analyzed. Carbonyl indices for all polymers treated with KOH and EtOH were not significantly different to untreated virgin MPs (PE: Mann–Whitney U = 15, n1 = 14, n2 = 3, p > 0.05, two tailed) (PS: Mann–Whitney U = 16, n1 = 15, n2 = 3, p > 0.05, two tailed) (PES: Mann–Whitney U = 10, n1 = 8, n2 = 3, p > 0.05, two tailed) (Rayon: Mann–Whitney U = 11, n1 = 8, n2 = 3, p > 0.05, two tailed) (Supplementary Table S3).
Discussion
Saponification (or alkaline hydrolysis) of triglycerides (e.g., lipids/animal fat) in the presence of a strong base (e.g., KOH) is a reaction where both reactants are consumed to form the salt of a carboxylic acid (otherwise known as soap) and an alcohol (Mercantili et al., 2014). This process has not been widely addressed in the literature concerning tissue digestion in terms of impacting MP extraction. It is possible that this is because few studies have attempted to extract MPs from large lipid-rich biota, where saponification is more likely to occur. Studies that have investigated larger biota (e.g., tuna, turtle, dolphin, whale) have either focused on stomach contents and not the stomach tissue (Besseling et al., 2015; Lusher et al., 2015; Chagnon et al., 2018; Hernandez-Gonzalez et al., 2018) or strategically avoided digestion with KOH (Caron et al., 2018b). One of the few studies to note the occurrence of saponification reported that KOH digestion of cod stomachs produced a “Layer of black/brown slime afloat” and recommended using a combined KOH: NaClO digestion (Enders et al., 2016). However, implementation of this method relies on prior knowledge that saponification will occur in the samples. One advantage of the workflow presented here is that it uses an existing method for tissue digestion (10% KOH) (Lusher and Hernandez-Milian, 2018; GESAMP, 2019), does not rely on prior knowledge of sample lipid content or the propensity for saponification, and can be applied to samples with the option of modification only if saponification occurs.
Larger biota such as barramundi and mangrove jack, with a high lipid content complicate the application of recommended KOH digestion methods (Enders et al., 2016; Karami et al., 2017; Bessa et al., 2019; GESAMP, 2019). Agitation and stirring the saponified fish sample did not clarify the solution, rather it accelerated saponification producing a homogenous soft gel (Supplementary Video). This can be explained in terms of reaction kinetics, where stirring and agitation have a positive effect on the conversion and rate constant (Mercantili et al., 2014; Danish et al., 2015). The addition of EtOH was effective at resuspending the gel within all samples that were initially deemed intractable, including those in which the entire volume (∼500 mL) had completely saponified due to agitation. Thus, EtOH treatment was found to be a suitable process to redissolve saponified samples and as such was included in the tailored workflow for treating gels formed in KOH digestions. A workflow based on visual assessments allowed for the flexibility required to process variable samples. Simple visual assessment was demonstrated to be a satisfactory decision tool for determining whether a sample, either treated or untreated, would pass through a fine pore filter with no gel residue. Thus, reducing the need for complex analytical testing of precious samples or troubleshooting after filtration has commenced. Further modification of the workflow, from a single predefined EtOH concentration (1:10), to a decision node triggered by visual assessment with clearly defined criteria, allowed for greater flexibility and the ability to handle samples of unknown complexity within a structured process. Each species of the wild fish exhibited varying levels of saponification (coral trout < mangrove jack < barramundi), and the workflow facilitated a tailored process for each species. Further, digestion efficiencies for all saponified, lipid-rich tissue samples were comparable to published values of biological materials using 10% KOH at 40°C without saponification. For example, reported digestion efficiencies include 96.8% for fish muscle and skin (Karami et al., 2017), 91.2% and 98.0% for bivalve tissue (Thiele et al., 2019). The addition of EtOH to saponified samples did not assist digestion or increase the efficiency, rather it decreased the sample viscosity, dissolving the solid gels and facilitated the final processing steps of filtration, which was not possible without treatment. Thereafter, putative MPs present on the filters can be further identified and characterized using established procedures [e.g., Kroon et al. (2018)].
The addition of EtOH in this study had no discernible effects on the spiked polymers. Exposure of some polymers to alcohols, including EtOH, may cause stress cracking or stiffening due to the dissolution of plasticizers, but do not impact on the polymer itself (Harvey, 2012). The recovered PS, PES and PE particles were visually unchanged, and comparison of their infrared spectral profiles with that of non-treated polymers revealed a >97% match. Bleached rayon fibers were present in all treated samples including those exposed to only KOH; confirming that EtOH did not contribute to the bleaching process under the conditions used. Although the infrared spectral profile of the treated rayon was changed, likely due to the leaching of the dye, an acceptable spectral match of 86–99% to the untreated rayon was still achieved. A previous study also found no visual effect of 70% EtOH on rayon when used to preserve spiked wild mussel samples for 7 days (Courtene-Jones et al., 2017). They also reported no effect on the abundance or diversity of polymers able to be recovered. Similarly, Herrera et al. (2018) utilized EtOH for the separation of MPs from vegetal material with no effect on the recovered polymers. In the current study, recovery of rayon and PE from spiked farmed barramundi tissue was 93.3%. This slight reduction in recovery rate from the expected 100% is unlikely a result of either KOH or EtOH exposure, as established above, but more likely a result of mishandling the samples or insufficient rinsing of the filtration system with Milli-Q to ensure particles were not adhered to the walls. As the aim of this study was to establish a workflow to clarify KOH digested samples, there was no attempt to quantify the recovery of MPs from untreated saponified tissue samples. Low density MPs are likely to float in 10% KOH (specific gravity 1.09 g mL–1) and thus be incorporated into the gel. Given most saponified samples were too viscous to pour and the gel would not pass through a fine pore filter, MPs would need to be hand-picked, making this process impractical. Importantly, in this study KOH tissue digestions were limited to 40°C, as significant damage to some polymers has been observed at higher temperatures (Karami et al., 2017; Munno et al., 2018). As such, the effects of exposing polymers to heated EtOH (>40°C) were not investigated. Although it is unlikely that EtOH would exacerbate the effects already induced by heating KOH (Van Krevelen and Te Nijenhuis, 2009; Harvey, 2012), it would be prudent to conduct further validation before applying this workflow to KOH digestions > 40°C. Moreover saponification reactions are endothermic thus elevated temperatures increase the saponification reaction (Danish et al., 2015; Eze et al., 2015). Therefore, KOH digestions above 40°C should be avoided for lipid-rich tissue.
Following digestion of wild fish viscera, a surprising amount of biological material remained on the filters, reflecting their multifarious diet. This included crustacean exoskeletons that had remained almost completely intact, but their soft tissue was completely digested. In contrast, retentate from farmed barramundi were much cleaner suggesting their GITs contained little ingested material. Although not an objective of this study, the results also demonstrate that chitinous material is particularly resistant to 10% KOH, at both RT and 40°C. Although ingested MPs were not quantified in the wild fish, based on the abundance of KOH resistant material (e.g., chitin, bone) remaining post digestion, it is likely that visual separation of putative MPs from the GIT content would be difficult to achieve. To facilitate this, further treatment with a hypersaline solution (e.g., Coppock et al., 2017) to separate MPs from the undigested residue is recommended for future work with similarly complex samples.
Finally, digestion efficiency is calculated as a function of sample weight pre- and post-treatment and reported as a percentage. However, achieving a high efficiency can be an artifact of sample size. With large samples it becomes possible to achieve a high digestion efficiency and still have significant undigested materials retained on the filter. For example, a single prawn contains a significant amount of chitin, which is highly resistant to KOH digestion. A single prawn digested in KOH will have a lower digestion efficiency than a fish stomach containing the same single prawn, even though the amount of undigested residue retained on the filter would contain the same amount of chitin. This was observed in the wild barramundi and farmed barramundi samples, where there was <0.3% difference in the digestion efficiencies but marked visual differences in the retentate burden. For future studies, visual inspection of retentate coupled with calculated digestion efficiency may be an appropriate method to determine if further sample clarification is needed to facilitate MP extraction from digestate.
Conclusion
Following established workflows for estimating microplastic contamination in environmental samples is key to ensuring global consistency. Overall, potassium hydroxide is one of the most recommended, and utilized, digestion techniques for isolating microplastics from biological tissue, being highly effective on soft tissue. The workflow developed in this study proposes a variation of the traditional potassium hydroxide digestion, which is applied only when samples exhibit saponification. An ad hoc decision, based on visual assessment, to determine the extent of ethanol treatment was demonstrated to be a suitable workflow for resuspending saponified samples, with demonstrated applicability for a variety of experimental conditions. Using this method, all samples in this study achieved a digestion efficiency >97%. Treatment with ethanol was established to be an inexpensive, simple and relatively safe method to optimize existing potassium hydroxide digestion procedures, thus enabling filtration of lipid-rich saponified samples. This technique also allows for the whole viscera to be digested and filtered, negating the need to remove non-target tissue during the initial dissection, thus reducing sample preparation time and associated contamination risk. However, it is worth noting that for particularly fatty samples, such as large farmed fish, removal of some excess lipid deposits will decrease the working volume of both potassium hydroxide and ethanol and may decrease the potential for complete saponification. We further suggest that biota possessing substantial stomach content resistant to digestion be further refined using a density separation to allow for microplastic extraction and visual identification.
Data Availability Statement
The raw data supporting the conclusions of this article will be made available by the authors, without undue reservation.
Ethics Statement
Ethical review and approval was not required for the animal study because deceased animal parts were commercially purchased or donated.
Author Contributions
ALD conceptualized the study, carried out the laboratory analysis, analyzed the data, and wrote the original draft. CAM contributed to the methodology and reviewed and edited the manuscript. FJK acquired funding and contributed to reviewing and editing the manuscript. All authors contributed to the article and approved the submitted version.
Funding
This project was funded from the Key Program for International S&T Cooperation Projects: Sino-Australian Center for Healthy Coasts (No. 2016YFE0101500), as well as the Australia China Science Research Fund grant ACSRF 48162, Australian Government Department of Industry, Innovation and Science, and the Queensland Government Department of Environment and Science.
Conflict of Interest
The authors declare that the research was conducted in the absence of any commercial or financial relationships that could be construed as a potential conflict of interest.
Acknowledgments
We would like to thank the commercial and recreational fishers who provided wild fish for this study and acknowledge the Traditional Owners of the Girringun TUMRA area. We are thankful to Dr. S. Wild for providing valuable comments on the manuscript.
Supplementary Material
The Supplementary Material for this article can be found online at: https://www.frontiersin.org/articles/10.3389/fenvs.2020.563565/full#supplementary-material
References
Akdogan, Z., and Guven, B. (2019). Microplastics in the environment: a critical review of current understanding and identification of future research needs. Environ. Pollut. 254:113011. doi: 10.1016/j.envpol.2019.113011
Bessa, F., Frias, J., Kögel, T., Lusher, A., Andrade, J., Antunes, J., et al. (2019). Harmonized Protocol for Monitoring Microplastics in Biota. Belgium: JPI-Oceans.
Besseling, E., Foekema, E. M., Van Franeker, J. A., Leopold, M. F., Kühn, S., Bravo Rebolledo, E. L., et al. (2015). Microplastic in a macro filter feeder: humpback whale Megaptera novaeangliae. Mar. Pollut. Bull. 95, 248–252. doi: 10.1016/j.marpolbul.2015.04.007
Bråte, I. L. N., Hurley, R., Iversen, K., Beyer, J., Thomas, K. V., Steindal, C. C., et al. (2018). Mytilus spp. as sentinels for monitoring microplastic pollution in Norwegian coastal waters: a qualitative and quantitative study. Environ. Pollut. 243, 383–393. doi: 10.1016/j.envpol.2018.08.077
Caron, A. G. M., Thomas, C. R., Berry, K. L. E., Motti, C. A., Ariel, E., and Brodie, J. E. (2018a). Ingestion of microplastic debris by green sea turtles (Chelonia mydas) in the great barrier reef: validation of a sequential extraction protocol. Mar. Pollut. Bull. 127, 743–751. doi: 10.1016/j.marpolbul.2017.12.062
Caron, A. G. M., Thomas, C. R., Berry, K. L. E., Motti, C. A., Ariel, E., and Brodie, J. E. (2018b). Validation of an optimised protocol for quantification of microplastics in heterogenous samples: a case study using green turtle chyme. MethodsX 5, 812–823. doi: 10.1016/j.mex.2018.07.009
Chagnon, C., Thiel, M., Antunes, J., Ferreira, J. L., Sobral, P., and Ory, N. C. (2018). Plastic ingestion and trophic transfer between Easter Island flying fish (Cheilopogon rapanouiensis) and yellowfin tuna (Thunnus albacares) from Rapa Nui (Easter Island). Environ. Pollut. 243, 127–133. doi: 10.1016/j.envpol.2018.08.042
Collard, F., Gilbert, B., Compere, P., Eppe, G., Das, K., Jauniaux, T., et al. (2017). Microplastics in livers of European anchovies (Engraulis encrasicolus. L.). Environ. Pollut. 229, 1000–1005. doi: 10.1016/j.envpol.2017.07.089
Coppock, R. L., Cole, M., Lindeque, P. K., Queirós, A. M., and Galloway, T. S. (2017). A small-scale, portable method for extracting microplastics from marine sediments. Environ. Pollut. 230, 829–837. doi: 10.1016/j.envpol.2017.07.017
Courtene-Jones, W., Quinn, B., Murphy, F., Gary, S. F., and Narayanaswamy, B. E. (2017). Optimisation of enzymatic digestion and validation of specimen preservation methods for the analysis of ingested microplastics. Anal. Methods 9, 1437–1445. doi: 10.1039/c6ay02343f
Danish, M., Al Mesfer, M. K., and Rashid, M. (2015). Effect of operating conditions on CSTR performance: an experimental study. Int. J. Eng. Res. Appl. 5, 74–78.
Dehaut, A., Cassone, A.-L., Frère, L., Hermabessiere, L., Himber, C., Rinnert, E., et al. (2016). Microplastics in seafood: benchmark protocol for their extraction and characterization. Environ. Pollut. 215, 223–233. doi: 10.1016/j.envpol.2016.05.018
Enders, K., Lenz, R., Beer, S., and Stedmon, C. A. (2016). Extraction of microplastic from biota: recommended acidic digestion destroys common plastic polymers. ICES J. Mar. Sci. 74, 326–331. doi: 10.1093/icesjms/fsw173
Eze, V. C., Harvey, A. P., and Phan, A. N. (2015). Determination of the kinetics of biodiesel saponification in alcoholic hydroxide solutions. Fuel 140, 724–730. doi: 10.1016/j.fuel.2014.10.001
Foekema, E. M., De Gruijter, C., Mergia, M. T., van Franeker, J. A., Murk, A. J., and Koelmans, A. A. (2013). Plastic in North Sea Fish. Environ. Sci. Technol. 47, 8818–8824. doi: 10.1021/es400931b
GESAMP (2019). “Guidelines or the monitoring and assessment of plastic litter and microplastics in the ocean,” in (IMO/FAO/UNESCO-IOC/UNIDO/WMO/IAEA/UN/UNEP/UNDP/ISA Joint Group of Experts on the Scientific Aspects of Marine Environmental Protection), eds P. J. Kershaw, A. Turra, and F. Galgani (London: GESAMP), 130.
Harvey, J. A. (2012). “Chemical and physical aging of plastics,” in Handbook of Environmental Degradation of Materials, 2nd Edn, ed. M. Kutz (Oxford: William Andrew Publishing), 195–211. doi: 10.1016/b978-1-4377-3455-3.00006-7
Hermsen, E., Mintenig, S. M., Besseling, E., and Koelmans, A. A. (2018). Quality criteria for the analysis of microplastic in biota samples: a critical review. Environ. Sci. Technol. 52, 10230–10240. doi: 10.1021/acs.est.8b01611
Hernandez-Gonzalez, A., Saavedra, C., Gago, J., Covelo, P., Santos, M. B., and Pierce, G. J. (2018). Microplastics in the stomach contents of common dolphin (Delphinus delphis) stranded on the Galician coasts (NW Spain, 2005–2010). Mar. Pollut. Bull. 137, 526–532. doi: 10.1016/j.marpolbul.2018.10.026
Herrera, A., Garrido-Amador, P., Martínez, I., Samper, M. D., López-Martínez, J., Gómez, M., et al. (2018). Novel methodology to isolate microplastics from vegetal-rich samples. Mar. Pollut. Bull. 129, 61–69. doi: 10.1016/j.marpolbul.2018.02.015
Karami, A., Golieskardi, A., Choo, C. K., Romano, N., Ho, Y. B., and Salamatinia, B. (2017). A high-performance protocol for extraction of microplastics in fish. Sci. Total Environ. 578, 485–494. doi: 10.1016/j.scitotenv.2016.10.213
Kent, J. A. (2003). “Soap, fatty acids, and synthetic detergents,” in Riegel’s Handbook of Industrial Chemistry, ed. J. A. Kent (Boston, MA: Springer), 1098–1140.
Konkol, K. L., and Rasmussen, S. C. (2015). “An ancient cleanser: soap production and use in antiquity,” in Chemical Technology in Antiquity, ed. S. C. Rasmussen (Washington, DC: American Chemical Society), 245–266. doi: 10.1021/bk-2015-1211.ch009
Kroon, F., Motti, C., Talbot, S., Sobral, P., and Puotinen, M. (2018). A workflow for improving estimates of microplastic contamination in marine waters: a case study from North-Western Australia. Environ. Pollut. 238, 26–38. doi: 10.1016/j.envpol.2018.03.010
Kühn, S., van Werven, B., van Oyen, A., Meijboom, A., Bravo Rebolledo, E. L., and van Franeker, J. A. (2017). The use of potassium hydroxide (KOH) solution as a suitable approach to isolate plastics ingested by marine organisms. Mar. Pollut. Bull. 115, 86–90. doi: 10.1016/j.marpolbul.2016.11.034
Lusher, A., Hollman, P., and Mendoza-Hill, J. (2017a). “Microplastics in fisheries and aquaculture: status of knowledge on their occurrence and implications for aquatic organisms and food safety,” in FAO Fisheries and Aquaculture Technical Paper, Rome.
Lusher, A., Welden, N. A., Sobral, P., and Cole, M. (2017b). Sampling, isolating and identifying microplastics ingested by fish and invertebrates. Anal. Methods 9, 1346–1360. doi: 10.1039/c6ay02415g
Lusher, A. L., and Hernandez-Milian, G. (2018). Microplastic extraction from marine vertebrate digestive tracts, regurgitates and scats: a protocol for researchers from all experience levels. Bio Protoc. 8:e3087. doi: 10.21769/BioProtoc.3087
Lusher, A. L., Hernandez-Milian, G., O’Brien, J., Berrow, S., O’Connor, I., and Officer, R. (2015). Microplastic and macroplastic ingestion by a deep diving, oceanic cetacean: the True’s beaked whale Mesoplodon mirus. Environ. Pollut. 199, 185–191. doi: 10.1016/j.envpol.2015.01.023
Lusher, A. L., McHugh, M., and Thompson, R. C. (2013). Occurrence of microplastics in the gastrointestinal tract of pelagic and demersal fish from the English channel. Mar. Pollut. Bull. 67, 94–99. doi: 10.1016/j.marpolbul.2012.11.028
Mabrouk, S. T. (2005). Making usable, quality opaque or transparent soap. J. Chem. Educ. 82:1534. doi: 10.1021/ed082p1534
Mercantili, L., Davis, F., and Higson, S. P. J. (2014). Ultrasonic initiation of the alkaline hydrolysis of triglycerides (Saponification) without phase catalysis. J. Surfactants Deterg. 17, 133–141. doi: 10.1007/s11743-013-1450-8
Miller, M. E., Kroon, F. J., and Motti, C. A. (2017). Recovering microplastics from marine samples: a review of current practices. Mar. Polluti. Bull. 123, 6–18. doi: 10.1016/j.marpolbul.2017.08.058
Munno, K., Helm, P. A., Jackson, D. A., Rochman, C., and Sims, A. (2018). Impacts of temperature and selected chemical digestion methods on microplastic particles. Environ. Toxicol. Chem. 37, 91–98. doi: 10.1002/etc.3935
Thiele, C. J., Hudson, M. D., and Russell, A. E. (2019). Evaluation of existing methods to extract microplastics from bivalve tissue: adapted KOH digestion protocol improves filtration at single-digit pore size. Mar. Pollut. Bull. 142, 384–393. doi: 10.1016/j.marpolbul.2019.03.003
Van Krevelen, D. W., and Te Nijenhuis, K. (2009). “Chapter 22 - chemical degradation,” in Properties of Polymers, 4th Edn, eds D. W. Van Krevelen and K. Te Nijenhuis (Amsterdam: Elsevier), 779–786.
Keywords: potassium hydroxide (KOH), ethanol (EtOH), fat deposit, saponification, gel, Plectropomus leopardus, Lates calcarifer, Lutjanus argentimaculatus
Citation: Dawson AL, Motti CA and Kroon FJ (2020) Solving a Sticky Situation: Microplastic Analysis of Lipid-Rich Tissue. Front. Environ. Sci. 8:563565. doi: 10.3389/fenvs.2020.563565
Received: 19 May 2020; Accepted: 18 August 2020;
Published: 16 September 2020.
Edited by:
Cristina Panti, University of Siena, ItalyReviewed by:
Joana Correia Prata, University of Aveiro, PortugalQing Wang, Yantai Institute of Coastal Zone Research (CAS), China
Copyright © 2020 Dawson, Motti and Kroon. This is an open-access article distributed under the terms of the Creative Commons Attribution License (CC BY). The use, distribution or reproduction in other forums is permitted, provided the original author(s) and the copyright owner(s) are credited and that the original publication in this journal is cited, in accordance with accepted academic practice. No use, distribution or reproduction is permitted which does not comply with these terms.
*Correspondence: Amanda L. Dawson, YS5kYXdzb25AYWltcy5nb3YuYXU=