- 1Akvaplan-niva, Tromsø, Norway
- 2Department: Environment and New Resources, SINTEF Ocean, Trondheim, Norway
- 3NILU, Norwegian Institute for Air Research, Tromsø, Norway
Crumb rubber granulate (CRG) produced from end of life tires (ELTs) is commonly applied to synthetic turf pitches (STPs), playgrounds, safety surfaces and walkways. In addition to fillers, stabilizers, cross-linking agents and secondary components (e.g., pigments, oils, resins), ELTs contain a range of other organic compound and heavy metal additives. While previous environmental impact studies on CRG have focused on terrestrial soil and freshwater ecosystems, many sites applying CRG in Norway are coastal. The current study investigated the organic chemical and metal additive content of ‘pristine’ and ‘weathered’ CRG and their seawater leachates, as well as uptake and effects of leachate exposure using marine copepods (Acartia and Calanus sp.). A combination of pyrolysis gas chromatography mass spectrometry (py-GC-MS) and chemical extraction followed by GC-MS analysis revealed similar organic chemical profiles for pristine and weathered CRG, including additives such as benzothiazole, N-1,3-dimethylbutyl-N′-phenyl-p-phenylenediamine and a range of polycyclic aromatic hydrocarbons (PAHs) and phenolic compounds (e.g., bisphenols). ICP-MS analysis revealed g kg–1 quantities of Zn and mg kg–1 quantities of Fe, Mn, Cu, Co, Cr, Pb, and Ni in the CRG. A cocktail of organic additives and metals readily leached from the CRG into seawater, with the most abundant leachate components being benzothiazole and Zn, Fe, Co (metals), as well as detectable levels of PAHs and phenolic compounds. Concentrations of individual components varied with CRG source material and CRG to seawater ratio, but benzothiazole and Zn were typically the organic and metal components present at the highest concentrations in the leachates. While organic chemical concentrations in the leachates stabilized within days, metals continued to leach out over the 30-day period. Marine copepods exposed to high CRG leachate concentrations exhibited high mortalities within 48 h. The smaller lipid-poor Acartia had a higher sensitivity to leachates than the larger lipid-rich Calanus, indicating species-specific differences in vulnerability to leachates. The effect on survival was alleviated at lower leachate concentrations, indicating a dose-response relationship. Benzothiazole and its derivatives appear to be of concern owing to their proven toxicity, while bisphenols are also known to be toxic and were enriched in the leachates relative to the other compounds in the CRG.
Introduction
In 2016, global production of natural and synthetic rubber reached 27.3 million tons (54% synthetic) (International Rubber Study Group, 2017), with ∼70% used in the manufacture of vehicle tires. It is estimated that there are 1 billion end-of-life tires (ELTs) generated globally each year (Wbscd, 2015). Despite the EU banning ELTs from landfill (European Community Directive 1999/31/EC and Waste Framework Directive 2006/12/EC) due to the risk of pollutant release, production of crumb rubber granulate (CRG) from ELTs has been considered an acceptable way of utilizing this waste material and is often considered recycling. Common CRG applications include outdoor artificial sports fields, playgrounds, general safety surfaces and on trails and walkways (Simon, 2010), where CRG is subject to weathering and transportation into the surrounding environment. It is estimated that 100–120 tons of CRG are used on a full-sized artificial football field (equivalent to ∼25000 ELTs) and that 1.5–2.5 tons is lost annually (Lassen et al., 2015). The European Chemicals Agency (ECHA) estimates there will be ∼21,000 full size and ∼72,000 mini-sized synthetic turf pitches in the EU by 2020, corresponding to 30% of all ELT usage (ECHA, 2017).
Car tire rubber and CRG from ELTs contains a broad range of additives including filler systems (carbon black, clays, silicas, calcium carbonate), stabilizer systems (antioxidants, antiozonants, waxes), cross-linking agents (sulfur, accelerators, activators) and secondary components such as pigments, oils, resins and short fibers. Chemical classes associated with car tires include polyaromatic hydrocarbons (PAHs), phthalates, sulfenamides, guanidines, thiazoles, thiuams, dithiocarbamates, sulfur donors, phenolics, phenylenediamines and heavy metals (Smolders and Degryse, 2002; ChemRisk Inc., 2008; Bocca et al., 2009; Llompart et al., 2013; Ruffino et al., 2013; Cheng et al., 2014; Canepari et al., 2017). Many of these chemicals can cause environmental impacts and pose risks to human health (Sadiktsis et al., 2012; Rodgers and Waddell, 2013; Ruffino et al., 2013; Cheng et al., 2014; Canepari et al., 2017; Halle et al., 2020).
In Europe, standards for Environmental Compatibility regulate the content of dissolved organic carbon (DOC), extractable organic halogens (EOX), Pb, Cd, Cr, Hg, Zn and Sn (DIN 18035-7:2002-06 and NF P90-112). Furthermore, EU REACH regulations (Annex XVII entry 28) require that carcinogens such as the EU-8 PAHs are not to be supplied to the general public above certain concentration limits (0.01–0.1% by weight; 100–1000 mg kg–1), while the concentration of individual PAHs may not exceed 0.0001% (1 mg kg–1) when present as PAH mixtures in consumer products (REACH Annex XVII entry 50). These concentrations are, however, regularly reached or exceeded for certain chemicals and metals in CRG derived from ELTs given the inhomogeneous nature of CRG sources (Diekmann et al., 2019). Identified compounds leaching from CRG into water include benzothiazoles, phthalates and phenols, where benzothiazole is typically observed in the highest quantities (Li et al., 2010; Llompart et al., 2013). In addition to being the largest contributor to the organic fraction of CRG leachates, benzothiazoles have also been identified as toxic to aquatic species including fish (He et al., 2011). Heavy metals leaching from CRG are also of concern, especially zinc (Zn) as it is present in quantities up to 1–2% (mass) and can leach in mg quantities over long periods of time, even after deposition in the environment (Rhodes et al., 2012).
Most environmental studies into the effects of CRG have focused on terrestrial soil and freshwater ecosystems, where leaching into rainwater and runoff via waterways occurs (Wik and Dave, 2009; Wagner et al., 2018; Halle et al., 2020). Regulated metals (As, Ag, Ba, Cd, Cr, Hg, Pb, and Se) and organic contaminants in freshwater leachates of tire rubber have been shown to have concentrations below their respective regulatory limits (Cheng et al., 2014). Laboratory studies with cladocerans (Daphnia magna) and algae (Pseudokirchneriella subcapitata) indicated the major toxic constituent in freshwater leachates is Zn, with minor contributions from organic compounds (Gualtieri et al., 2005; Wik et al., 2009). A recent study reported only small fractions of the heavy metals and PAHs present were bioavailable for freshwater benthic macroinvertebrates (Redondo-Hasselerharm et al., 2018). However, many urban areas are located on the coast, making the marine environment an additional likely sink for CRG as it is transported through the environment. For example, Norway has a number of artificial turfs using CRG as turf infill located near the coast or fjords, and also storage and production facilities for CRG adjacent to ports and the open sea (Figure 1; Møllhausen et al., 2017). There is very limited knowledge regarding the behavior and fate of CRG in the marine environment. Ecotoxicological metrics are often the starting point for environmental risk assessment. Risk assessment procedures include various metrics of species tolerance to chemicals (Forbes and Calow, 2002; Calow and Forbes, 2003). Survival, quantified through standardized laboratory toxicity tests, is a widely used expression of species tolerance to chemical exposures. The most common testing protocol is performed by exposing biota to several different concentrations of chemicals.
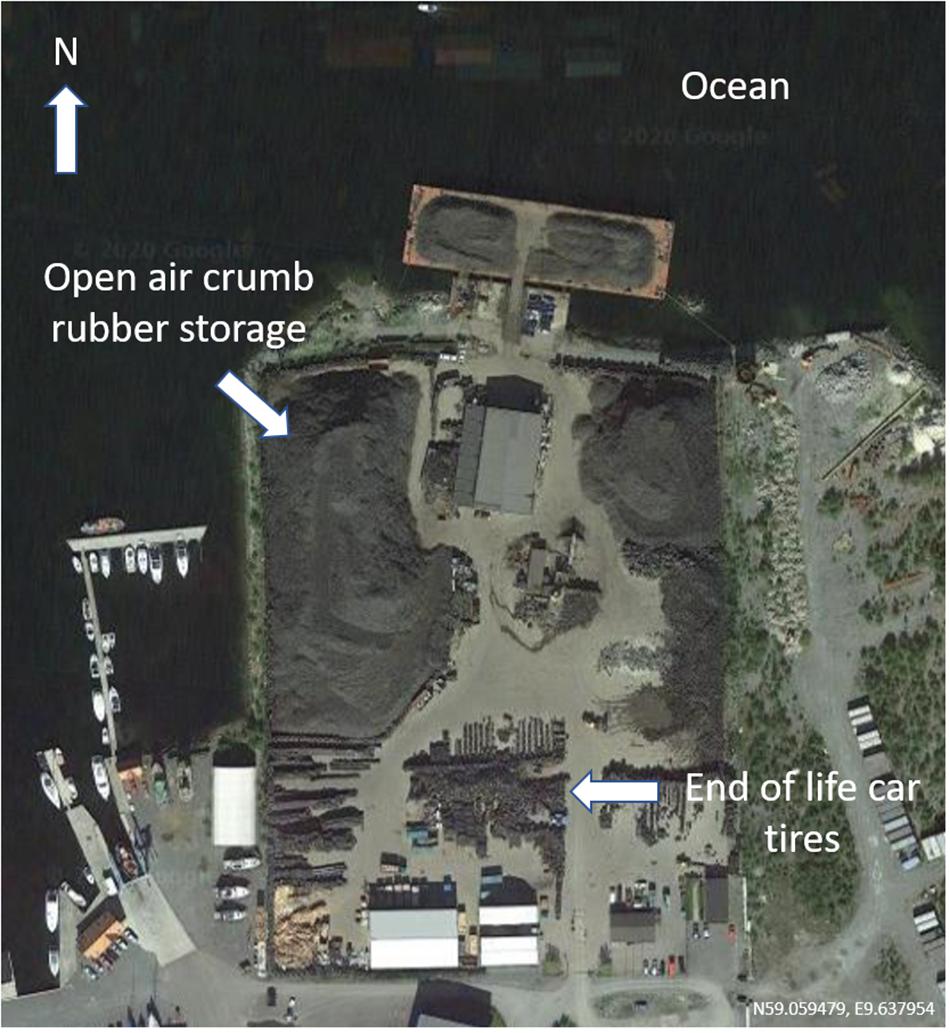
Figure 1. Example of CRG storage and production facilities located adjacent to port and open sea areas near Porsgrunn, Norway. Aerial image generated using Google Maps (2020).
The current study aimed to investigate the organic chemical and metal profiles in CRG materials and their associated seawater leachates, as well as assessing the toxicity of CRG leachates to two coastal arctic species of copepod (Acartia longiremis and Calanus sp.). CRG test materials were sourced both directly from a commercial supplier (‘pristine’) and collected from outdoor sports fields in Trondheim and Tromsø (‘weathered’). Additionally, the commercial material was cryomilled into small particle size fractions. The organic chemical content of the CRG materials was determined by a combination of non-target and target analysis using gas chromatography mass spectrometry (GC-MS) techniques, while metals were determined using inductive coupled plasma mass spectrometry (ICP-MS). Leachate studies were performed over a 30-day period and target organics and metals determined using the same techniques. CRG leachates were also produced and used to investigate their toxicity to marine copepods (Acartia longiremis and Calanus sp.).
Materials and Methods
Chemicals and Materials
Pristine CRG (RGS) was supplied by RagnSells, Norway, pre-use CRG (TOS), produced by JOGRA, Steinindustri AS, Norway and weathered CRG (TRD) was collected directly from an outdoor sports field in Trondheim, Norway. All organic solvents and salts were of analytical grade and their purity verified in-house prior to use. Dichloromethane (DCM) was supplied by Rathburn (United Kingdom), ethyl acetate (EtOAc) was supplied by Fluka (Germany) and methanol was supplied by MERCK (Norway). Deionized water was produced from a MilliPore® MilliQ water system. Natural seawater was collected from 90 m depth in Trondhjemsfjorden and 60 m depth in Sandnessund (Tromsø), filtered to remove coarse particles and then sterile filtered (0.22 μm Sterivex®) prior to use in the experiments. Reference organic chemical standards were supplied by Chiron AS (Trondheim, Norway) and Sigma-Aldrich (Darmstadt, Germany). Reference inorganic chemical standards were supplied by Inorganic Ventures (Christiansburg, VA, United States). The suite of tire-derived CRG reference materials comprised a ‘pristine’ CRG procured from a commercial supplier (RGS) and two field collected samples representing ‘pre-use’ (TOS) and ‘weathered’ (TRD) CRG materials (Table 1). The pristine RGS CRG material (1.0–2.8 mm) was further cryomilled into <1500, <1000 and <250 μm fractions.
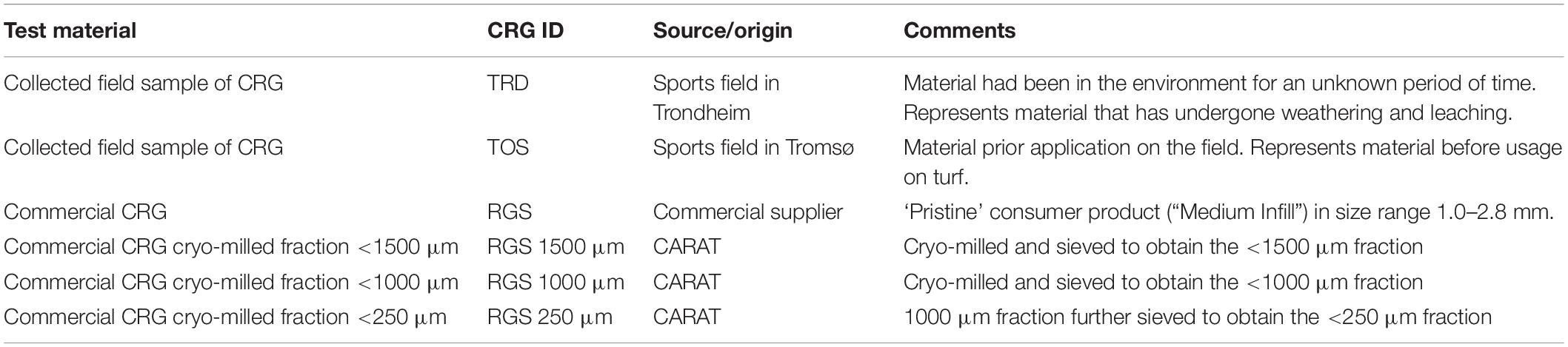
Table 1. Overview of tire-derived crumb rubber granulate (CRG) reference materials used in the studies.
Characterization of CRG Materials
Prior to use in leaching and toxicity studies, the metal and organic chemical content of the CRG samples was determined using a combination of non-target and target analytical chemical methods; conventional gas- and liquid chromatography mass spectrometry (GC- and LC-MS), pyrolysis-GC-MS and inductively coupled plasma mass spectrometry (ICP-MS). For conventional non-target GC-MS analysis, triplicate samples of CRG (∼100 mg) were solvent extracted with DCM and triplicate samples were extracted using EtOAc. For target analysis of phthalates, duplicates were extracted using DCM/hexane (1:1, v/v), where 4 mL solvent and a mixture of surrogate organic chemical internal standards (DEP-d4, DIBP-d4, DHXP-d4, DBzP-d4, DEHP-d4) was added to each sample prior to extraction. Extraction of all samples was conducted using bath sonication for 30 min (Bandelin Sonorex Super RK 510H, 640W, 35 kHz) at either room temperature (DCM and DCM/hexane) or 65°C (EtOAc) to bath sonication for 30 min. Solvent extracts were then filtered through a pipette packed with Bilson cotton and a small amount of anhydrous Na2SO4 to remove particulates and moisture. Extracts were then concentrated by solvent evaporation (40°C under a gentle flow of N2) to approximately 500 μL and recovery internal standards (fluorene-d10, acenapthene-d10 or DOP-d4 depending on the target chemicals) added prior to analysis by GC- and LC-MS. Phenolic compounds in CRG were determined by extracting a sub sample (0.1 g) twice with 2 mL of distilled methanol under 15 min of ultrasonication (USC-THD, VWR, Norway). Internal standards (13C-labeled BPA, BPB, BPE, 4,4′-BPF, BPP, 4,4′-BPS, 2,4′-BPS, BPZ, BPAF, TBBPA, non-ylphenol, octylphenol and D-labeled 2,2′-BPF, and BPAP) were added prior to extraction. The extracts were combined and concentrated to 0.5 mL, followed by centrifugation to remove any suspended particulate material prior to analysis by LC-MS. For pyrolysis GC-MS, CRG samples were analyzed directly without any pre-treatment. The samples (a few mg of each) were placed in a 45 μL glass vial, which was subsequently heat-sealed. Samples were analyzed using both thermal desorption and pyrolysis approaches.
Leaching of Chemicals From CRG
The influence of CRG particle size, CRG concentration and natural weathering (both field-collected samples and samples placed in ocean for 12 months) on the metal and organic chemical profile of the resulting leachates was investigated. To generate leachates for chemical characterization, CRG samples were shaken (orbital shaker) at 250 rpm in sterile-filtered seawater at room temperature (approximately 20°C) in the dark. The leachate studies investigated the influence of CRG concentration (1, 10 and 100 g L–1), exposure time (1–30 days), the influence of CRG origin (pristine, pre-use, weathered) and the influence of CRG particle size (medium infill particles (1.0–2.8 mm) and cryomilled particles: 250, 1000, and 1500 μm) on the resulting leachate composition. To generate leachates for toxicity testing, a standard exposure time (14 days), CRG concentration (100 and 10 g L–1, respectively), and size (medium infill) was applied. Dilutions of filtered leachate were prepared directly in sterile filtered seawater (salinity 34–35 psu, pH 8.0–8.2).
On sampling, the leachates were isolated from the CRG material using a glass fiber filter (GF/F or GF/C, 0.7–1.2 μm nominal pore size) and then sub-sampled for metal and organics analysis. For GC-MS analysis, surrogate internal standards (same as above) were added to the aqueous leachates, which were then acidified (HCl, pH∼2). The samples were extracted three times with either DCM only or with a mixture of DCM and n-hexane (1:1, v/v) in appropriate volumes depending on the sample size. The combined extracts were gently evaporated to approximately 500 μL and a recovery internal standard (same as above) added immediately prior to analysis by GC-MS. For LC-MS analysis of the phenolic compounds, 20 μL of each leachate was mixed into 80 mL of HCl and an internal standard (13C-labeled BPA, BPB, BPE, 4,4′-BPF, BPP, 4,4′-BPS, 2,4′-BPS, BPZ, BPAF, TBBPA, non-ylphenol, octylphenol and D-labeled 2,2′-BPF and BPAP) added. An equal amount of methanol was added to an aliquot of the acidified mixture and thoroughly mixed prior to analysis by LC-MS. Aliquots of the leachates were set aside for metal analysis by ICP-MS.
Analytical Methods
Both the thermal desorption and full pyrolysis methods used to analyses the CRG materials employed an Agilent 7890A GC coupled to an Agilent 5975C MS, fitted with a ZB5-MSplus column (60 m × 0.25 mm × 0.25 μm) and an EI source operated at 230°C and 70 eV. The CRG samples were introduced into the pyrolysis chamber at 230°C, and the chamber temperature rapidly increased to the final temperature (300°C or 600°C), before the vial was manually broken and the analytes released onto a cryogenic (liquid nitrogen) trap. The pyrolysis chamber was heated to 300°C (2 min hold) for thermal desorption analysis and heated to 600°C (2 min hold) for full pyrolysis. After the hold time, the analytes are released onto the analytical column with helium as the carrier gas. The GC temperature was held at 40°C (1 min), ramped to 320°C at 12°C min–1 (12 min hold). The MS was operated in full scan mode (m/z 50–500) and analytes identified based on >90% match to NIST 2017 library spectra.
Each CRG material and corresponding leachate extract was analyzed by three different GC-MS approaches: (i) a non-target full-scan analysis to identify all GC-amenable additive chemicals, (ii) a selected ion monitoring (SIM) method specifically targeted toward PAHs, and (iii) a SIM method targeted toward benzothiazole. All analyses were performed with a GC-MS system comprising an Agilent 7890A GC equipped with an Agilent 5975C Mass Selective Detector (MSD) fitted with an EI ion source. A detailed overview of the instrumental conditions is provided in the Supplementary Information. After initial inspection of chromatograms, peaks were deconvoluted using Unknowns algorithms and best hits from NIST 2017 library were extracted. Compounds were filtered based on observed presence in at least 3 of 6 replicates and >90% match to NIST 2017 library mass spectra. Biogenic compounds or compounds of possible biogenic origin were removed from the data set. All compounds found in control samples were removed from the data set. For target analyte quantification, a 6-level calibration curve was applied to calculate concentrations after normalizing response to internal standards.
Phenolic compounds were analyzed using the Agilent 1290 UHPLC coupled to Agilent 6550 HR-QTOF system operated in a negative electrospray ionization mode. Separation of bisphenols was achieved with the use of a Waters HSS T3 column (1.8 μm, 150 × 3.0 mm) with a gradient of water and methanol used as a mobile phase. The phthalate extract was measured directly without further pre-treatment by LC-MS (Vantage, Thermo Fisher Scientific, United States) using a Waters UPLC column with a BEH Phenyl 100 × 2.1 mm, 1.8 μm phase. A solvent gradient of A: 0.1% Formic acid in water and B: 0.1% formic acid in methanol was applied as the mobile phase. For quantification of bisphenols and phthalates, the isotopic dilution method was applied. Detection limits were calculated based on the instrumental sensitivity of control samples. All data are blank corrected.
Metal concentrations in the CRG extracts and leachate extracts were determined for different experiments at two laboratories using two slightly different, but comparable ICP-MS approaches. A detailed overview of the sample preparation approach and instrumental conditions are provided in the Supplementary Information. Briefly, the first approach involved digestion of the samples using HNO3, HCl and H2O2 at 220°C for 20 min, followed by dilution in MilliQ and addition of 103Rh and 115In internal standards. Analysis was performed using an Agilent 8800 Triple Quadrupole ICP-MS (ICP-QQQ) fitted with a SPS 4 Autosampler. In the second approach, samples were digested in 5 mL HNO3 and 3 mL deionized water at 250°C for 65 min, followed by dilution in MilliQ and addition of 115In as an internal standard. Analysis was performed using an Agilent 7700x ICP-MS.
Exposure of Marine Copepods to Seawater CRG Leachates
Zooplankton was collected in Balsfjord and Håkøybotn near Tromsø (Norway, 69.67°N 18.79°E) with a WP2 net fitted with a 180 μm mesh and a non-filtering cod end. The organisms were diluted in ambient seawater and brought to the laboratory for acclimation in 50 L tanks aerated with silicone tubes. Individual adult female copepods were sorted into small bowls and kept at ambient temperature (8°C) prior to use. For exposure experiments, a series of seawater CRG leachate stock solutions were produced using the method described above. The stock solutions represented leachates derived from (i) 100 g L–1 of TOS CRG, (ii) 10 g L–1 TOS CRG, (iii) 10 g L–1 TRD CRG, and (iv) 10 g L–1 RGS CRG. The leachates were isolated by passing the sample through a glass fiber filter (GF/C, 1.2 μm nominal pore size). For toxicity testing, the stock solutions were diluted with filtered seawater to the desired concentrations (0.01–100 g L–1). The corresponding CRG mass concentrations for each leachate dilution are presented in Supplementary Table S1.
A pilot study (Experiment 1) to determine general concentration ranges leading to copepod mortality was conducted with 24 individuals of two coastal arctic species (Acartia longiremis females and Calanus sp. pre-adult copepodite stage 5 (C5) and adult females) sorted from the field samples collected at Håkøybotn. The organisms were exposed to seawater leachate solutions of CRG TOS (100 g L–1) at 100 (i.e., undiluted) and 50 g L–1 dilution in 5 mL wells on two 12-multiwell plates (n = 24). Mortalities were recorded in 4 h intervals (Acartia only) and at the end of the 24 h exposure period. In a second study (Experiment 2), groups of copepods (n = 10) were incubated in 3 replicate 500 mL blue cap glass bottles (total volume 620 mL) containing filtered seawater, microalgae food (Tetraselmis sp. >5000 cells mL–1) and a range of leachate concentrations corresponding to 5–35 g L–1 of CRG (TOS only). Control exposures contained only copepods, algae and filtered seawater (no leachate). The bottles were attached onto a plankton wheel (Supplementary Figure S1) and rotated slowly (0.26 rpm) for up to 17 days (or until all individuals in the exposure bottles had died) while submersed in seawater at 8°C. A third study (Experiment 3) used the same approach as Experiment 2, but with lower leachate concentrations (representing 0.01, 0.1 and 1 g L–1 of CRG) and for 3 different CRG types (TOS, TRD and RGS). Survival was monitored daily over a 2-week period.
To study the effect of the leachates on the survival of the copepods, effect sizes were calculated as mean differences, subtracting average mortality in the corresponding controls from the mortality recorded in the leachate dilutions:
The variance was estimated as the pooled standard deviation (Rosnow and Rosenthal, 1996):
The pooled standard deviation was then multiplied with 1.96, representing 95% of the area under a normal distribution curve, to plot vertical error bars of the mean differences. Error bars above (and not crossing) the zero line denote significantly higher mortality in the exposures than in the controls.
Results and Discussion
CRG Characterization
Non-target Screening Analysis of CRG
An overview of the organic compounds detected in the CRG extracts by non-target analysis is presented in Supplementary Table S2. In total, 19 different compounds were identified with a ≥90% match to NIST 2017 library mass spectra. Compounds included PAHs (pyrene and phenanthrene), benzothiazoles (benzothiazole, 2-mercaptobenzothiazole), phenols (4-tert-octylphenol, 3-tert-butylphenol), methyl stearate, quinolines and amines (N-(1,3-dimethylbutyl)-N′-phenyl-1,4-benzenediamine, diphenylamine) amongst others. PAHs and benzothiazoles are well-known components of CRG, with many classified as environmental and human toxins (ChemRisk Inc., 2008; ECHA, 2017). However, some of the other compounds identified represent classes of chemicals that are less commonly reported and less is known about their potential risks (Rogge et al., 1993; Llompart et al., 2013; Wagner et al., 2018).
Quantitative Analysis of Target Organic Compounds in CRG
A summary of the concentrations of the target 16 EPA PAHs (presented as total PAHs), phenolics, benzothiazole and other selected compounds in the CRG extracts (TRD, TOS and RGS) is presented in Table 2. Concentrations of individual detectable compounds ranged from 0.0004 mg kg–1 (4,4′-bisphenol S in TRD) to 540 mg kg–1 (acetophenone in TOS) of CRG. Total PAH concentrations across the 3 different CRG materials were largely consistent and ranged from 47 mg kg–1 (TOS) to 58 mg kg–1 (TRD). The most prevalent PAH was pyrene at 24–25 mg kg–1, followed by fluoranthene and phenanthrene at 8–7 mg kg–1 and 3.8–6.5 mg kg–1, respectively. These findings are well within the range of concentrations reported in CRG by ECHA (9.12–58.2 mg kg–1) and by the US EPA (mean of 41 mg kg–1; n = 27) (ECHA, 2017; US EPA and CDC/ATSDR, 2019). Benzothiazole exhibited high concentrations in all CRG materials, but with greater variation than the PAHs, ranging from 37 mg kg–1 (TRD) to 110 mg kg–1 (TOS). These values are slightly higher to those reported previously by the US EPA of 11 mg kg–1 (US EPA and CDC/ATSDR, 2019). Acetophenone and phthalide were present in very low concentrations in the TRD and RGS materials (0.22–0.37 and 0.1–0.4 mg kg–1, respectively), but were present in very high concentrations in the TOS material (78–540 mg kg–1, respectively). In general, phenolic compounds were present in very low concentrations ranging from 0.0004 mg kg–1 to 4 mg kg–1, with seven of the twelve target phenols not being found in any of the CRG materials. Only 2,4-bisphenol A, 2,4-bisphenol F, 4,4′-bisphenol F and trace amounts of 4,4′-bisphenol S and 4,4′-bisphenol A were detected. Sum Bisphenol concentrations ranged from at 2.26 mg kg–1 (TOS) to 6.33 mg kg–1 (TRD), with 2,4-bisphenol F as the major contributor at 0.61–1.21 mg kg–1, followed by 4,4′-bisphenol F at 0.38–0.83 mg kg–1 and 2,4-bisphenol A with 0.16–0.18 mg kg–1. Interestingly, there was quite a large degree of variation in the concentrations of some compounds between the different CRG samples. This may reflect different source materials used in the preparation or, in the case of TRD samples which had been exposed in the environment, changes due to weathering. While there does not appear to be any studies comparing the chemical composition of a wide range of different tires, an ecotoxicological assessment of the leachates from 25 different tires indicated a range of EC50 values, suggesting varying chemical compositions (Wik and Dave, 2006).
Of the 14 analyzed phthalates, only 7 could be detected in CRG (Table 2). DEHP dominated with 17.7 mg/kg followed by DiNP, DiBP and DnBP (10.1, 2.94, 2.60, and 2.06 mg/kg). The total phthalate load in CRG is similar to the PAH load and benzothiazole (47–58 mg kg–1 and 37–110 mg/kg–1, respectively). A previous study found lower average concentrations for all four of these phthalates in CRG sampled directly from artificial pitches, but the values from the current study are well within the variation of the data reported (RIVM, 2016). Four phthalates (DiBP, DBP, BBP and DEHP) are classified by ECHA as toxic to reproduction in category 1B (may damage the unborn child and are suspected of damaging fertility), with BBP and DBP also categorized as toxic to the aquatic environment. In addition, ECHA’s Member State Committee (MSC) has unanimously confirmed that these four phthalates are endocrine disruptors related to human health (although they did not agree unanimously that they were of equivalent concern) and that DEHP is an endocrine disruptor in the environment. All four phthalates are registered as substances of very high concern (SVHC) (ECHA, 2017). Both phthalates and their metabolites were found in marine species such as turtles and porpoises, indicating existing exposure to these rubber and plastic additives. After being taken up by the organisms, they are metabolized relatively fast, forming stable metabolites with unknown toxicity (Savoca et al., 2018; Rian et al., 2020).
CRG Characterization by Pyrolysis GC-MS
Thermal desorption chromatograms and pyrograms are presented in Supplementary Table S3. The pyrograms are complex but reveal similar “fingerprints” between pristine infill (RGS) and weathered CRG (TRD). This is not surprising, given that most of the compounds revealed by this type of analysis are large molecules and small fragments typically produced by the pyrolysis process. Additive-related compounds identified included benzothiazole and its methylated isomers, N-(1,3-dimethylbutyl)-N′-phenyl-1,4-benzenediamine, d-limonene, and quinolines. Other compounds identified included small aliphatic (alkanes, alkenes and cyclic compounds) and aromatic hydrocarbons (BTEX (benzene, toluene, ethylbenzene, xylenes), C4-C6 alkylbenzenes, styrene, indanes, PAHs) and small ketones. The smaller hydrocarbons are expected to be products of partial fragmentation of the styrene butadiene rubber (SBR) in the CRG, while the other compounds are mostly known rubber additives. A number of additional additives were identified by chemical extraction followed by fullscan GC-MS analysis of the CRG (Supplementary Table S2), with benzothiazole being the most pronounced additive peak, alongside N-(1,3-dimethylbutyl)-N′-phenyl-1,4-benzenediamine, which was also identified in the pyrograms.
Metals in CRG
The results of the metals analysis of pristine (RGS), pre-use (TOS) and weathered (TRD) CRG, as well as cryomilled CRG of different size fractions, are given in Table 3. Zinc was the most abundant metal in all samples, ranging from 22601 mg kg–1 (TOS) to 12544 mg kg–1 (TRD). Mg ranged from 1046 mg kg–1 (TRD) to 273 mg kg–1 (RGS), Al ranged from 1305 mg kg–1 (TRD) to 1066 mg kg–1 (RGS), Fe ranged from 1214 mg kg–1 (TRD) to 729 mg kg–1 (TOS), Co ranged from 84 mg kg–1 (RGS) to 36.5 mg kg–1 (TRD) and Cu ranged from 85 mg kg–1 (TOS) to 18 mg kg–1 (TRD). All other metals (Cr, Mn, Ni, Cd, Sb and Pb) were below 25 mg kg–1 in all CRG samples. The variation in individual metal concentrations between TRD, TOS and RGS was typically less than an order of magnitude (Table 3). The observed variation appears to reflect differences in the source materials for the different CRG materials as the concentration of some metals was highest in the weathered TRD material (Mg, Al, Cr, Mn, Fe, Ni). However, Zn was lower in TRD than either TOS or RGS and may indicate loss of this metal through leaching in the environment.
Leaching of CRG Chemicals Into Seawater
The pilot study investigating the effect of exposure time (1–30 days) on metal and organic additive composition and concentration in seawater leachates indicated that an exposure time of 14 days was sufficient to generate stable leachate concentrations of organic chemicals in a static system (Figure 2). However, Zn concentrations in the seawater leachate continued to increase until the end of the experiment, which lasted for 30 days. This is in line with previous studies on Zn leaching from tire rubber, which showed that continued leaching in a flow-through system did not deplete the Zn reservoir in the granulate significantly (Rhodes et al., 2012). Based on these data, an exposure time of 14 days was used to generate leachates for the remaining leachate studies and toxicity studies.
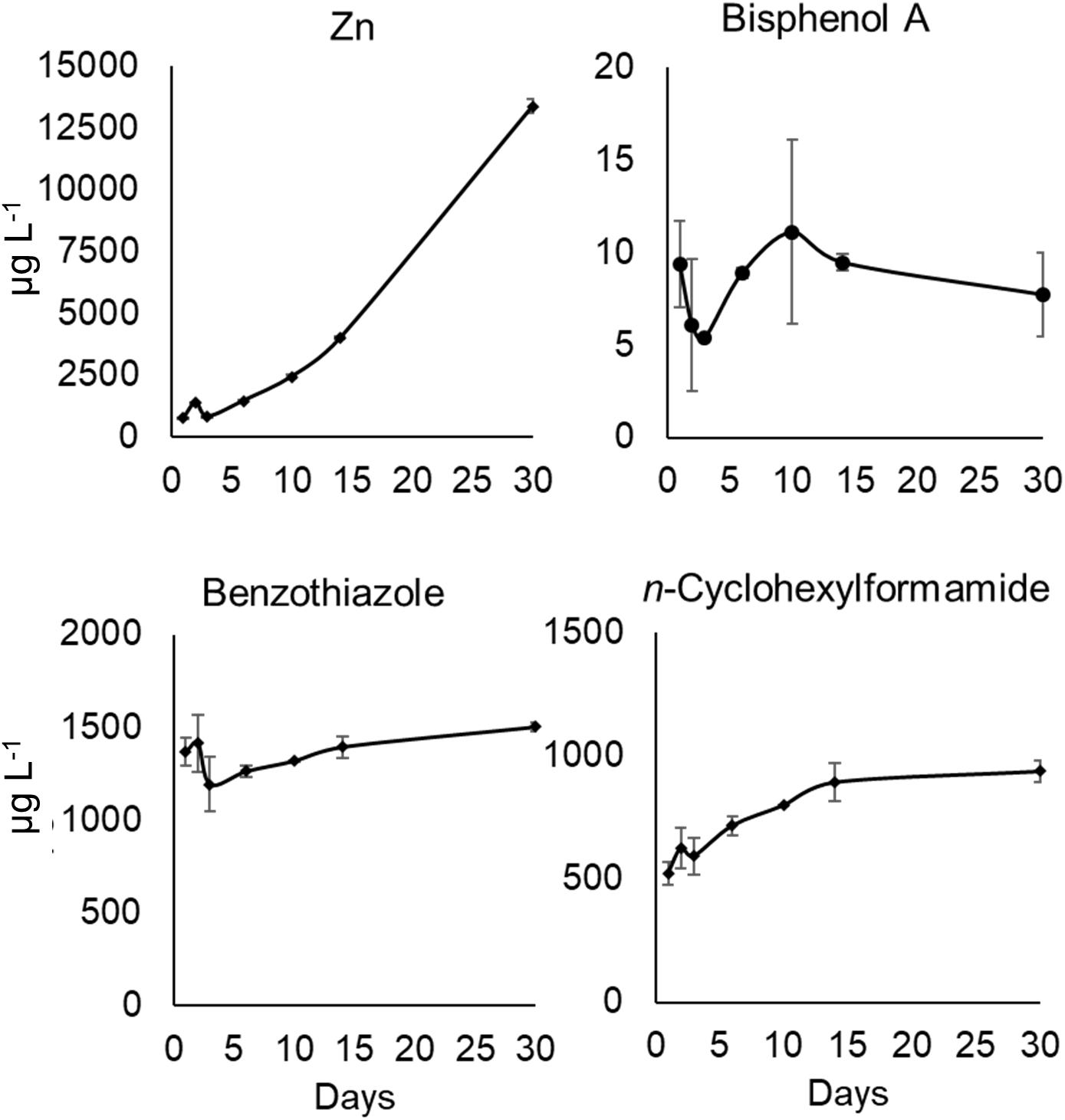
Figure 2. Leaching of zinc, bisphenol A, benzothiazole and n-cyclohexylformamide from pristine crumb rubber granulate (RGS) into seawater over 30 days at a crumb rubber concentration of 100 g L–1.
After 14 days, a distinct coloration of the seawater was clearly visible, indicating leaching and dispersion of fine CRG particulates (Supplementary Figure S2). Target analysis of the leachates revealed that a number of organic (Tables 4, 5) and metal (Table 6) additives leached from the CRG into the seawater. Benzothiazole was the organic compound with the highest concentration in all CRG leachates, irrespective of the CRG to water ratio used to generate the leachate, while Zn was the metal with the highest concentration in all leachates. The most abundant organic and metal components measured in the original CRGs materials were also most abundant in the corresponding leachates. Benzothiazole concentrations in leachates from the three different CRGs differed but were consistent with the distributions in the source CRG materials where the lowest concentrations were determined for TRD CRG and the corresponding leachate (Table 4). This may reflect inherent variability of the CRG composition or that this low molecular weight compound (MW 135) preferentially leaches out of CRG in the natural environment. However, benzothiazole concentrations in the leachates closely reflected those in the respective source CRGs. Previous studies have reported benzothiazole CRG leachate concentrations of 293–578 μg L–1 (Nilsson et al., 2008), 526 μg L–1 (Ly and Walker, 2009), 18 μg L–1 (Celeiro et al., 2014), which are comparable to the values determined in the current study (Table 4). The concentrations of benzothiazole and Zn in seawater leachates showed a linear relationship with the amount of CRG added to the seawater (Figure 3), supporting the suitability of direct dilution of stock leachates for the toxicity study.
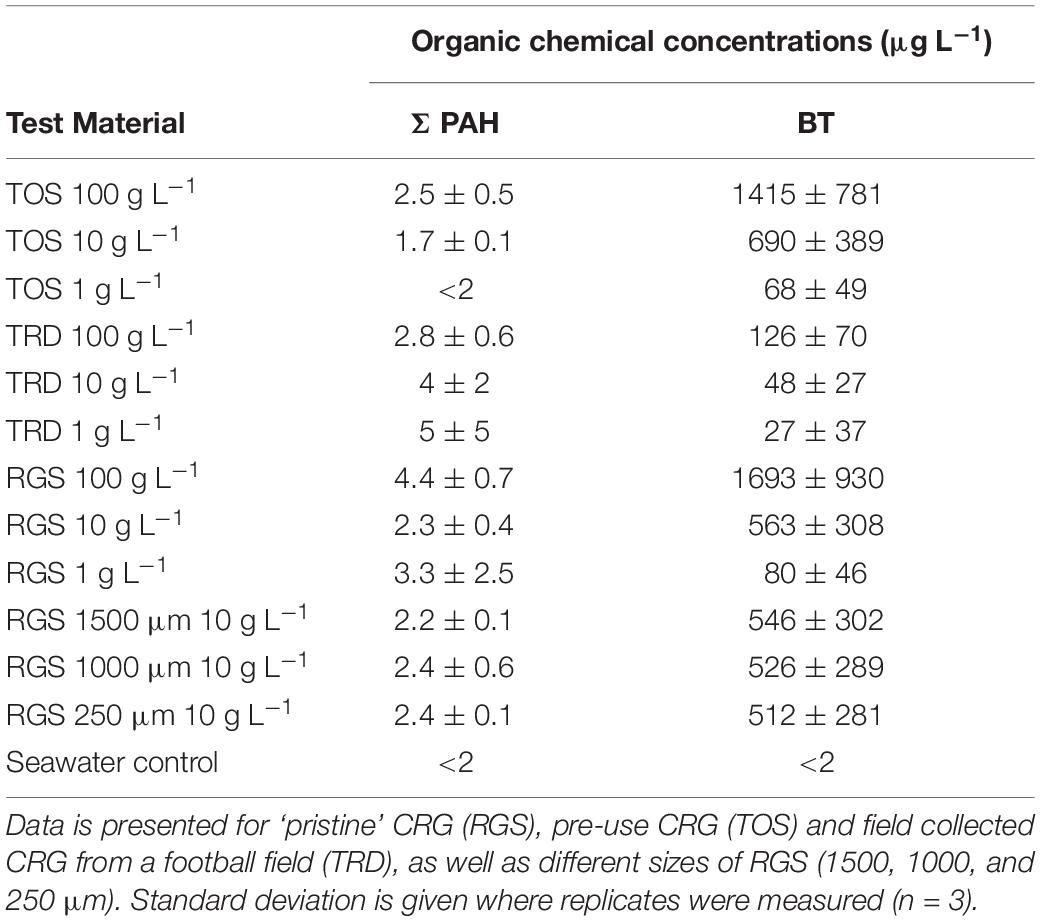
Table 4. Concentration of benzothiazole and total PAHs in seawater leachates as a function of CRG concentration.
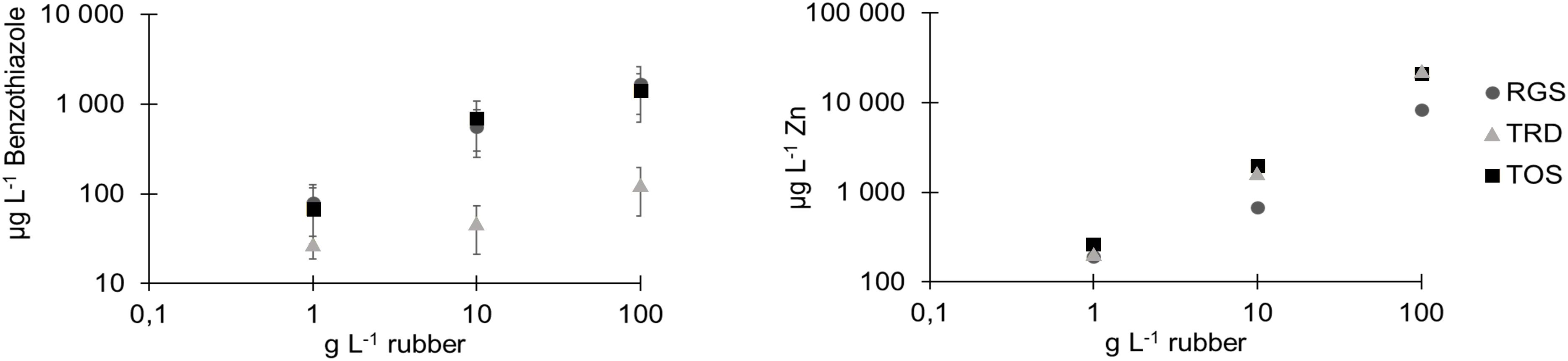
Figure 3. Increasing concentrations of benzothiazole (left) and zinc (right) in seawater leachates (μg L–1) as a function of CRG concentration. The graphs show averages and standard deviations for 3 replicates of leachate produced from ‘pristine’ CRG (RGS), pre-use CRG (TOS) and field collected CRG from a football field (TRD).
The total PAH concentrations in the different seawater leachates were generally low, ranging between <LOD (for the leachate produced from TOS at 1 g L–1) and 4.4 μg L–1 (for the leachate produced from RGS at 100 g L–1) (Table 4). In contrast to benzothiazole and metals, there was no clear increase in the total PAH concentration relative to increasing CRG exposure concentration. Phenolic compounds were found in low abundance in the TOS leachate, dominated by 2,4-bisphenol F and 4,4′-bisphenol F (11.9 and 6.2 μg L–1), while phthalates were not found in the TOS leachate at all (Table 5). After Zn, the metals present at the highest concentration in the different CRG leachates (from CRG at 100 g L–1) were Fe (126–377 μg L–1), Mn (25–79 μg L–1), Cu (39–66 μg L–1) and Co (13.57 μg L–1) (Table 6). All other target metals (Cr, Ni, Cd, Sb and Pb) were present at concentrations of <10 μg L–1 in all leachates. The metal profiles in the leachates largely reflect those in the CRG materials (Table 3), with metals at higher concentrations in the parent CRG materials also being present at higher concentrations in the resulting leachates.
In the samples investigating the influence of particle size on leachate composition, the concentrations of individual organic chemicals and metals showed different patterns (Tables 4–6). In general, the concentrations of specific organic chemicals were similar for all three of the different particle sizes studied (250, 1000, 1500 μm) at CRG concentrations of 10 g L–1. Total PAHs ranged from 2.2–2.4 μg L–1 and benzothiazole ranged from 512–546 μg L–1, which was also comparable to the non-cryomilled material at the same exposure concentration (2.7 and 563 μg L–1, respectively). A similar pattern was observed for the metals Cr (4.2–5.0 μg L–1) and Pb (3.0–3.6 μg L–1), which also compared to the non-cryo-milled material (4.0 and 2.7 μg L–1, respectively). The other metals generally exhibited an increase in leachate concentration with a corresponding decrease in CRG particle size, although this was more pronounced for some metals than others. For example, metal concentrations more than doubled in leachates derived from 250 μm CRG particles compared those from 1500 μm CRG particles, where Zn increased from 1.7 mg L–1 to 4.1 mg L–1, Cu increased from 23 to 33 μg L–1, Mn increased from 4 μg L–1 to 20 μg L–1 and Co increased from 2.3 μg L–1 to 11.4 μg L–1. Smaller particles have a greater surface area to volume ratio, which is known to promote leaching to aqueous media. The increased leaching of Zn from CRG of decreasing particle size observed in the current study has previously been demonstrated (Rhodes et al., 2012). Very few studies have investigated the release of other metals from CRG or tire wear particles of different sizes, but the available literature indicates that the leaching of many metals is independent of particle size (Selbes et al., 2015). While it would have been interesting to normalize the leached metal concentration data by surface area to identify size effects, the size distribution of the test materials was actually quite broad and therefore the estimated surface area became a ‘surface area range’. Furthermore, the particles in the current study were very irregular in shape with detailed surface morphologies, meaning that estimation of surface area based on the assumption of spherical particles is too far away from an accurate surface area estimation to be sufficiently robust. Dissolved organic carbon leaching from SBR particles has been shown to increase with smaller particle sizes (Selbes et al., 2015), which contrasts the observations for specific organic compounds in the current study. A comprehensive review of tire wear particles in the environment concluded that the influence of particle size on leaching is inconclusive (Wagner et al., 2018). The results of the current study suggest leaching of CRG components is influenced by particle size and the partitioning coefficient of individual organics and metals between the CRG and aqueous phase, as well as the background concentration of the compounds in the surrounding water (direction of concentration gradient to reach equilibrium). The values recorded in leachates here were all above EU guideline thresholds for marine and freshwater (EU DIRECTIVE 2008/105/EC), where Environmental Quality Standards (EQS) of 0.28, 1.0 and 7.8 μg L–1 have been defined for cobalt, copper and zinc, respectively. Leached concentrations were exceeding these concentrations by up to three orders of magnitude (Table 6), with Zn over the recommended threshold by a factor of >2500.
Phthalic anhydride and n-cyclohexylformamide were observed in the CRG leachates but not in the original CRG materials by any of the extraction and analysis techniques (Supplementary Table S2). Compounds observed in CRG leachates and not in solvent extracts or pyrograms of the original CRG materials could reflect the differing water solubilities of organic chemicals present in car tire rubber. Both phthalic anhydride and n-cyclohexylformamide are highly polar, low molecular weight compounds (MW 148 and 127, respectively). Such compounds may be present in low levels in the CRG, but preferentially leach into aqueous solution. n-Cyclohexylformamide has previously been identified in fumes of ethylene propylene diene rubber, suggesting it could be a component of CRG (Forrest, 2019).
Toxicity of CRG Leachates to Marine Copepods
As relevant marine environmental concentrations are currently unknown, three exposure experiments using CRG TOS tested a wide selection of leachate concentrations, ranging from high in Experiment 1 (100 and 50 g L–1), via medium in Experiment 2 (5–35 g L–1), to low in Experiment 3 (1-0.01 g L–1). Mortality was chosen as the endpoint for the two copepods, the smaller Acartia longiremis and the larger Calanus sp. CRG TOS was selected as test material due to immediate availability of sufficient CRG amounts to produce the leachates. Mortality in control bottles was variable over time and between experiments, but did not mask the dose response in the treatments, except at the lowest concentrations where mortality in controls was higher than in the exposures in some instances. This may simply be due to stochastic variation in the data since we do not expect a beneficial effect of low leachate doses on the copepods. We cannot, however, rule out that non-lethal doses of one or several of the measured contaminants trigger a physiological defense response in the exposed copepods, which may increase their survival compared to non-exposed counterparts. This possibility would have to be investigated further and the nature of the defense mechanism studied with suitable methods (e.g., gene expression mapping). Cumulative mortality over time is presented in Figure 4 for each experiment and both copepod species. At high leachate concentrations (Experiment 1), all copepods died within 24 h. This was studied in more detail for A. longiremis, showing a slower deterioration at 50 g L–1 than at 100 g L–1 after 4, 8 and 12 h of incubation (Figure 4A). Medium leachate concentrations (Experiment 2) induced a clear dose-response in both species, but also demonstrated higher sensitivity in Acartia than in Calanus, where Acartia reached 100% mortality much faster than Calanus at all three leachate concentrations (Figure 4B). The LC50 values at 48 h were 35 g L–1 for Calanus compared to <5 g L–1 for Acartia. At the lowest concentrations (Experiment 3), mortalities were higher in the controls than in the leachate exposures for both species and final mortalities after 2 weeks were ≤50% for exposed copepods (Figure 4C). For Calanus, only one low TOS concentration (0.1 g L–1) was tested, where survival was 72% on day 14. The low leachate concentrations did therefore not induce negative effects in either species (Figure 4C). Experiment 3 (low concentrations; 1-0.01 g L–1) was repeated with two more CRG types, the weathered TRD and the pristine RGS (Supplementary Figure S3). Again, copepod mortalities in leachate exposures were similar to those in the controls, except for TRD at 1 g L–1, for which elevated mortalities were observed in both copepod species (Supplementary Figure S3b).
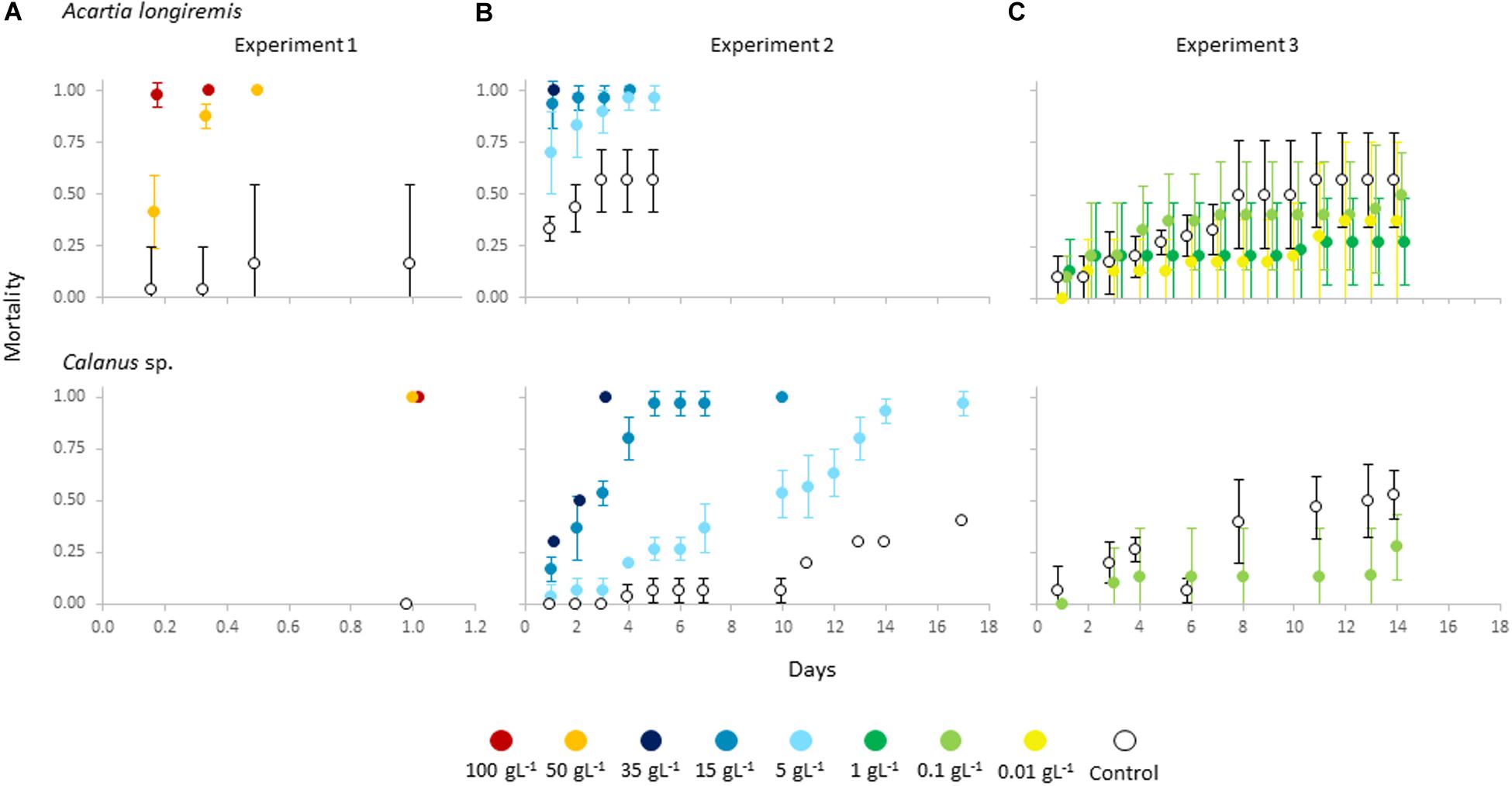
Figure 4. Mortality of Acartia longiremis (upper panels) and Calanus sp. (lower panels) exposed to a range of TOS CRG leachate concentrations in three experiments; (A) Experiment 1 (50 and 100 g L–1), (B) Experiment 2 (35, 15 and 5 g L–1), (C) Experiment 3 (1, 0.1 and 0.01 g L–1). Experiments 1, 2, and 3 ran for 1, 17, and 14 days, respectively.
To test for significant differences in mortality between exposed and unexposed copepods, effect sizes were calculated for three selected time points: day 1, day 8 and day 14. Significant effect sizes were recorded for all exposure concentrations ≥5 g L–1 of CRG TOS (Figure 5). The lower exposure concentrations (0.01–1 g L–1) were not different from controls, including for TRD at 1 g L–1, despite the elevated mortalities mentioned above (Supplementary Figure S3b). Nonetheless, it can be hypothesized that the increased weathering state of this rubber appeared to contribute to the observed increase in toxicity. It is suggested that the partial weathering alters the rubber properties, for example making it more brittle and increasing the available surface area, leading to a higher degree of contaminant release from the material.
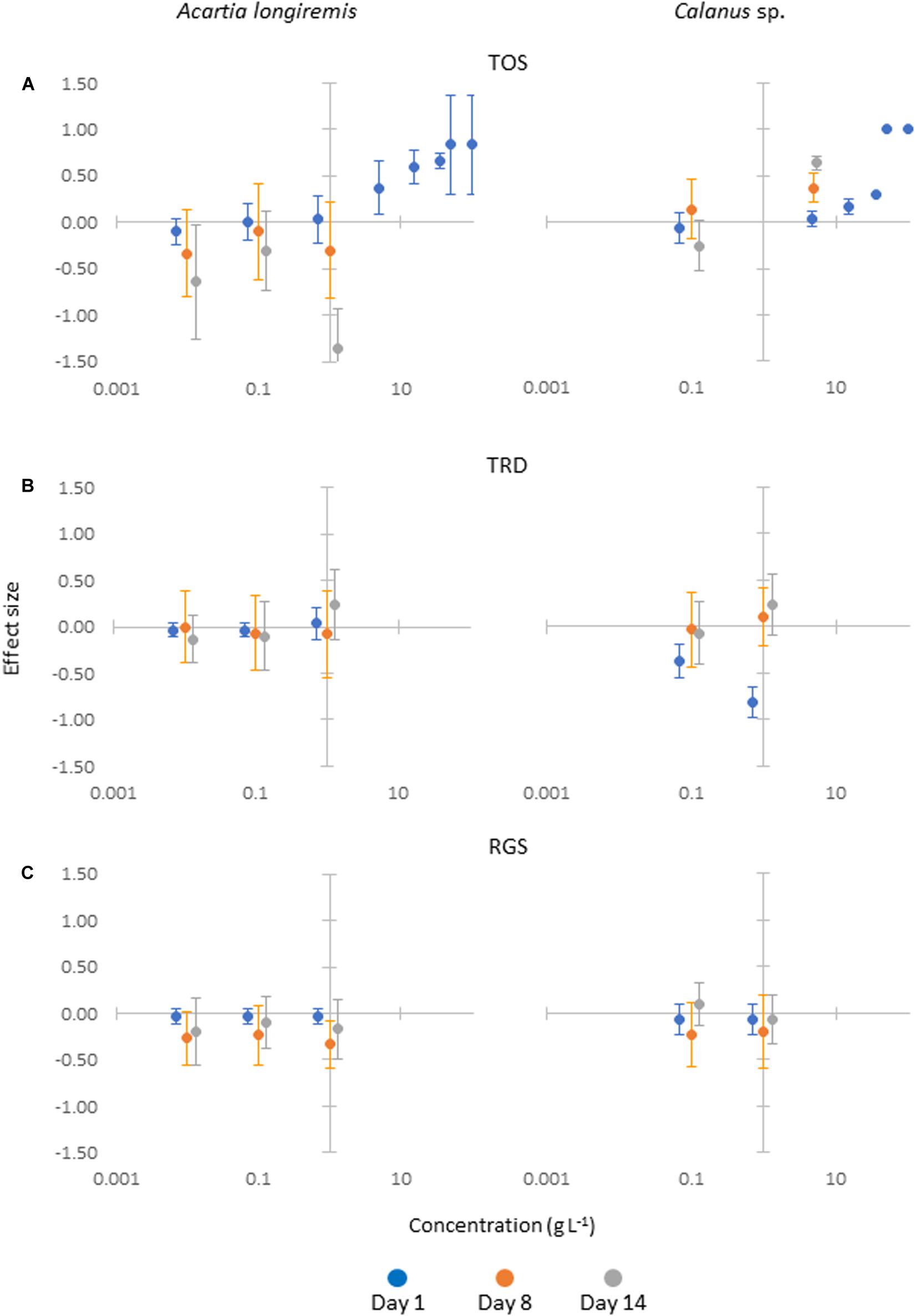
Figure 5. Effect sizes of different CRG concentrations for Acartia longiremis (left) and Calanus sp. (right) for three CRG types: (A) TOS, (B) TRD, and (C) RGS. Positive values with error bars not crossing the zero line indicate significantly higher mortality in leachate treatments than in controls.
Species-Specific Toxicity
The study demonstrates different sensitivities of the two copepods studied: Acartia responded with higher mortality more quickly than Calanus at a given CRG concentration. This may be explained by (a) the difference in body size (Neumann et al., 2005), where the smaller Acartia may receive higher doses via a larger surface to volume ratio than the larger Calanus, or (b) ingest more toxin due to higher clearance rates (volume of water filtered per time unit), or (c) due to differences in defense/repair mechanisms or internal toxin pathways. For example, Calanus C5 stages have lipid reserves that may help them to ‘buffer’ toxic molecules and remove them from their metabolism, while Acartia lacks this option and may be more exposed to oxidative stress (Hansen et al., 2018; Sørensen et al., 2020). The toxicity of CRG leachates on aquatic organisms has been reviewed in Wik and Dave (2009) and Halle et al. (2020). Effect concentrations ranged widely and depended on the type (e.g., abrasion method), origin (e.g., summer versus winter tires) and state (e.g., weathering, UV exposure) of the CRG applied. To our knowledge, no other studies on marine zooplankton have been performed to date, except for one study on the brackish Eurytemora affinis (Hall et al., 1993), where leachate exposure resulted in 100% mortality. Effect concentrations for freshwater cladocerans (daphnids), comparable to the pelagic marine copepods studied here, ranged widely but seemed to be generally lower than those recorded here. The reported 48 h EC50 values for D. magna ranged from 0.25 g L–1 to 10 g L–1 (Wik and Dave, 2005, 2006), while another study found an LC50 of 25 g L–1 after 72 h of incubation (Goudey and Barton, 1992), which is a relatively high value compared to the LC50 values (48 h) between 5 and 35 g L–1 determined in the current study. It has been proposed that tire-derived leachates exhibit reduced toxicity with increasing salinity (Hartwell et al., 2000) and the current study supports this. When leachates were eluted at pH values <7, toxicity increased in parallel with increasing Zn concentrations in the eluate (Gualtieri et al., 2005), indicating that the leachability of contaminants is variable and depends on the state of the leaching rubber (e.g., weathering state) and the prevailing leaching conditions. UV exposure of the rubber also seemed to play a role for the level of toxicity of the resulting leachate (Wik and Dave, 2006).
What Are the Drivers of CRG Leachate Toxicity?
Ingestion of CRG particles by marine organisms is known to occur (Redondo-Hasselerharm et al., 2018; Khan et al., 2019), leading to potential exposure through leaching during gut transit. However, exposure of marine organisms to additive chemicals in CRG is likely to be more widespread through leaching to the aqueous phase, especially as some of these additives exhibit persistency in the environment (Halle et al., 2020). The variety of organic additives present in CRG make it extremely challenging to determine which chemical groups are of most interest for assessing the potential environmental impacts and risks associated with CRG. Leachate toxicity studies with TWP and CRG material have been conducted in different aquatic environments with a variety of species, resulting in large variations in effects that have been attributed to differences in tire composition, leachate generation method and species sensitivity (Wik and Dave, 2009; Wagner et al., 2018). However, full elucidation of the components of TWP and CRG leachates driving toxicological responses in aquatic environments has yet to be achieved. Furthermore, comparison of CRG/TWP toxicity data is compromised by a lack of standard methods for generating leachates, for characterizing the additive chemical composition and for measuring their hazard potential, although adjustment of already existing guidelines on soluble contaminants could be fine-tuned to suit a leachate guideline (Khan et al., 2017). It will also be important going forward to established methods for distinguishing between particle effects and those deriving from additive chemicals reaching from CRG/TWP (Wik and Dave, 2009; Wagner et al., 2018; Halle et al., 2020).
In the current study, it was not possible to clearly establish which components of the CRG leachates were driving the observed toxicity and the complexity of the leachates meant that it was only possible to quantify a subset of the organic chemicals present. Benzothiazole and its derivatives appear to be strong candidates for contributing to the observed effects based on the high leachate concentrations and established toxicity. Acute and chronic toxicity of benzothiazole and its derivatives was shown for the daphnid C. dubia (Nawrocki et al., 2005). Benzothiazole produced EC50s at 24.6 mg L–1 in acute (24 h) exposures and at 54.9 mg L–1 in chronic 1-week exposures, respectively, while several of the derivatives (including 2-mercaptobenzothiazole) had much higher toxicity. Although the concentrations of benzothiazole measured in our leachates remained below these values, with a range of 0.068 to 1.42 mg L–1 (Table 4), they may well have contributed to the overall toxicity observed in this study. The benzothiazole derivate 2-mercaptobenzothiazole was detected in CRG, but not in the leachates with the available method (Supplementary Table S2). Future studies should include this group of contaminants with higher resolution in the analytical chemistry to better pinpoint its contribution to CRG leachate toxicity.
In contrast to benzothiazole, PAHs in our CRG materials exceeded REACH Annex XVII entry 50 levels, but not EU REACH regulations (Annex XVII entry 28). Furthermore, PAHs only leached in limited quantities, suggesting they represent only a minor contribution to the observed toxicity. Similarly, phthalates did not leach significantly. While present in relatively small amounts in the original CRG material (3 mg kg–1), a range of bisphenols leached from CRG into seawater at relatively high concentrations compared to other organics (seemingly reported for the first time herein). Bisphenols have well-documented endocrine disrupting properties, with BPS and BPF being more potent than BPA (Chen et al., 2016). While very little data is available on the aquatic toxicity of these chemicals, a field study has shown that marine copepods accumulate bisphenols, especially in earlier developmental stages (Staniszewska et al., 2016). Bisphenols from CRG may thus contribute not only to toxic effects in the copepods themselves, but in addition pose a risk to secondary consumers in marine food webs. Zn was by far the most prevalent metal present in the CRG leachates and is often cited as the candidate most likely responsible for observed CRG/TWP leachate toxicity. For example, zinc toxicity was shown to relate to a disruption of calcium ion uptake in Daphnia magna (Muyssen et al., 2006) and similar mechanisms could apply for marine copepods.
Many other unidentified and unquantified organic compounds were also present in the leachates and may also be contributing to the overall toxicity. In general, the total concentrations of metals and organic pollutants are a limited means of assessing fate and transport. For example, metal toxicity depends not on total the concentration of a specific element, but on speciation, which is in turn controlled by environmental parameters such as redox, adsorption and interactions with dissolved organic matter. For organic pollutants, individual congeners of a group might exhibit a different toxic impact than others, as well as metabolization and bioaccumulation characteristics caused by variations in their molecular structure and the subsequent interactions with organisms and the environment. It is therefore important to consider that the most abundant compounds or metals in a leachate are not necessarily the most toxic and that additive toxicity may also occur. Depending on the mode(s) of action of single toxins and/or toxin mixtures, the effects may differ between marine habitats (e.g., sediment versus water column) and functional groups (e.g., feeding mode, foraging strategy, reproductive strategy, etc.). Although a previous study has indicated that leachates from car tire rubber materials are likely to be a greater threat to freshwater habitats than to estuarine or marine habitats (Hartwell et al., 2000), the current study suggests that impacts in the marine environment should also be considered, especially in regions with high emissions of TWP/CRG (e.g., urban runoff) and the Arctic, where some species may exhibit greater sensitivity than others. Beyond standardized laboratory-based effect concentrations, realistic environmental exposure scenarios are needed that study in situ CRG concentrations together with lethal and sublethal effects on individuals and populations in realistic concentration gradients from point sources (e.g., open-air storage facilities, coastal snow removal dumping sites). Long-term exposure of the daphnid Cerodaphnia dubia yielded EC50 values of 0.01–1.8 g L–1 (Wik et al., 2009), but any equivalent data for marine organisms is currently lacking. Finally, it will be important to pinpoint which leachate components are driving the observed toxicity and whether this varies across different marine species. This will provide the knowledge needed to develop risk assessments for ELTs and CRG, as well as providing industry with a priority list of additives that should be reduced or removed from rubber products.
Conclusion
The current work represents one of the first experimental studies to investigate the impacts of chemical additive leachates from CRG from ELTs on marine organisms. Detailed characterization of pristine and weathered CRG reference materials and their leachates indicated a complex mixture of organic chemical and metal additives were present in the materials and their corresponding leachates. Importantly, there were significant differences in additive profiles between CRG materials and their leachates, but pristine and weathered CRG materials had similar profiles, indicating that CRG particulates and the leaching chemicals will continue to pose a threat to wildlife long after their disposal. Marine copepods exhibited a dose-dependent response to CRG leachates, but species-specific differences were observed suggesting some organisms are more vulnerable to exposure than others. While benzothiazole and Zn were typically the organic and metal components identified at the highest concentrations in the leachates, further studies are necessary to elucidate which CRG leachate components are driving the observed toxicity. Furthermore, the long-term effects of CRG leachate exposure and sublethal endpoints should be studied in the future, also in combination with ingestion/exposure to the rubber particles.
Data Availability Statement
The raw data supporting the conclusions of this article will be made available by the authors, without undue reservation.
Author Contributions
CH, DH, AB, and LS contributed equally to the study. They all conceived and designed the study in collaboration. LS, DH, and AB conducted analysis of the samples. CH prepared samples and conducted the toxicity studies. All authors contributed equally to the preparation of the manuscript and approved its submission.
Funding
This work has been funded by the Fram Center Flagship Hazardous Substances (Framsenteret, Norway), project number 1002018.
Conflict of Interest
CH was employed by the company Akvaplan-niva. LS and AB were employed by the company SINTEF Ocean. DH was employed by the research foundation NILU. All authors declare that any commercial or financial relationships that could be construed as a potential conflict of interest had no influence on the findings of this research.
Acknowledgments
We are grateful to Itsasne Beitia Aguirre, Lisbet Støen, and Marianne Kjos at SINTEF and Mikael Harju, Pawel Rostkowski, and Marit Vadset at NILU for assistance with chemical analysis. We also acknowledge Kristine Hopland Sperre and Hector Andrade (Akvaplan-niva) for assistance with field sampling, exposure experiments, and plotting of toxicity data. We thank CARAT GmbH (Germany) for conducting cryomilling of the RGS CRG material.
Supplementary Material
The Supplementary Material for this article can be found online at: https://www.frontiersin.org/articles/10.3389/fenvs.2020.00125/full#supplementary-material
References
Bocca, B., Forte, G., Petrucci, F., Costantini, S., and Izzo, P. (2009). Metals contained and leached from rubber granulates used in synthetic turf areas. Sci. Total Environ. 407, 2183–2190. doi: 10.1016/j.scitotenv.2008.12.026
Calow, P., and Forbes, V. E. (2003). Peer reviewed: does ecotoxicology inform ecological risk assessment? Environ. Sci. Technol. 37, 146A–151A. doi: 10.1021/es0324003
Canepari, S., Castellano, P., Astolfi, M. L., Materazzi, S., Ferrante, R., Fiorini, D., et al. (2017). Release of particles, organic compounds, and metals from crumb rubber used in synthetic turf under chemical and physical stress. Environ. Sci. Pollut. Res. 25, 1448–1459. doi: 10.1007/s11356-017-0377-4
Celeiro, M., Lamas, J. P., Garcia-Jares, C., Dagnac, T., Ramos, L., and Llompart, M. (2014). Investigation of PAH and other hazardous contaminant occurrence in recycled tyre rubber surfaces. Case-study: restaurant playground in an indoor shopping centre. Int. J. Environ. Anal. Chem. 94, 1264–1271. doi: 10.1080/03067319.2014.930847
ChemRisk Inc. (2008). State of Knowledge Report for Tire Materials and Tire Wear Particles. San Francisco, CA: ChemRisk Inc.
Chen, D., Kannan, K., Tan, H., Zheng, Z., Feng, Y.-L., Wu, Y., et al. (2016). Bisphenol analogues other than BPA: environmental occurrence, human exposure, and toxicity—a review. Environ. Sci. Technol. 50, 5438–5453. doi: 10.1021/acs.est.5b05387
Cheng, H., Hu, Y., and Reinhard, M. (2014). Environmental and health impacts of artificial turf: a review. Environ. Sci. Technol. 48, 2114–2129. doi: 10.1021/es4044193
Diekmann, A., Giese, U., and Schaumann, I. (2019). Polycyclic aromatic hydrocarbons in consumer goods made from recycled rubber material: a review. Chemosphere 220, 1163–1178. doi: 10.1016/j.chemosphere.2018.12.111
ECHA (2017). An evaluation of the Possible Health Risks of Recycled Rubber Granules Used as Infill in Synthetic Turf Sports fields. Helsinki: ECHA.
Forbes, V. E., and Calow, P. (2002). Species sensitivity distributions revisited: a critical appraisal. Hum. Ecol. Risk Assess. Int. J. 8, 473–492. doi: 10.1080/10807030290879781
Forrest, M. J. (2019). Rubber Analysis: Characterisation, Failure Diagnosis and Reverse Engineering. Berlin: De Gruyter.
Google Maps (2020). Available online at: https://www.google.com/maps/
Goudey, J. S., and Barton, B. A. (1992). “Toxicity of scrap tire materials to selected aquatic organisms,” in Report to Souris Basin Development Authority, ed. R. Saskatchewan (Calgary, AB: Hydroqual Laboratories Limited and Environmental Management Associates).
Gualtieri, M., Andrioletti, M., Vismara, C., Milani, M., and Camatini, M. (2005). Toxicity of tire debris leachates. Environ. Int. 31, 723–730. doi: 10.1016/j.envint.2005.02.001
Hall, L. W., Ziegenfuss, M. C., and Anderson, R. D. (1993). Toxicity of Tire Leachate to Eurytemora Affinis. Queenstown, MD: University of Maryland.
Halle, L. L., Palmqvist, A., Kampmann, K., and Khan, F. R. (2020). Ecotoxicology of micronized tire rubber: past, present and future considerations. Sci. Total Environ. 706:135694. doi: 10.1016/j.scitotenv.2019.135694
Hansen, B. H., Olsen, A. J., Salaberria, I., Altin, D., Øverjordet, I. B., Gardinali, P., et al. (2018). Partitioning of PAHs between crude oil microdroplets, water, and copepod biomass in oil-in-seawater dispersions of different crude oils. Environ. Sci. Technol. 52, 14436–14444. doi: 10.1021/acs.est.8b04591
Hartwell, S. I., Jordahl, D. M., and Dawson, C. E. O. (2000). The effect of salinity on tire leachate toxicity. Water Air Soil Pollut. 121, 119–131.
He, G., Zhao, B., and Denison, M. S. (2011). Identification of benzothiazole derivatives and polycyclic aromatic hydrocarbons as aryl hydrocarbon receptor agonists present in tire extracts. Environ. Toxicol. Chem. 30, 1915–1925. doi: 10.1002/etc.581
International Rubber Study Group (2017). Statistical Summary of World Rubber Situation. Rubber Statistical Bulletin. Singapore: IRSG.
Khan, F. R., Halle, L. L., and Palmqvist, A. (2019). Acute and long-term toxicity of micronized car tire wear particles to Hyalella azteca. Aqu. Toxicol. 213:105216. doi: 10.1016/j.aquatox.2019.05.018
Khan, F. R., Syberg, K., and Palmqvist, A. (2017). Are standardized test guidelines adequate for assessing waterborne particulate contaminants? Environ. Sci. Technol. 51, 1948–1950. doi: 10.1021/acs.est.6b06456
Lassen, C., Hansen, S. F., Magnusson, K., Norén, F., Hartmann, N. B., Jensen, P. R., et al. (2015). Microplastics. Occurrence, Effects and Sources of Releases to the Environment in Denmark. Copenhagen: The Danish Environmental Protection Agency.
Li, X., Berger, W., Musante, C., and Mattina, M. I. (2010). Characterization of substances released from crumb rubber material used on artificial turf fields. Chemosphere 80, 279–285. doi: 10.1016/j.chemosphere.2010.04.021
Llompart, M., Sanchez-Prado, L., Pablo Lamas, J., Garcia-Jares, C., Roca, E., and Dagnac, T. (2013). Hazardous organic chemicals in rubber recycled tire playgrounds and pavers. Chemosphere 90, 423–431. doi: 10.1016/j.chemosphere.2012.07.053
Ly, L., and Walker, R. (2009). An assessment of Chemical Leaching, Releases to Air and Temperature at Crumb Rubber Infilled Synthetic Turf Fields. Albany, NY: New York State Department of Environmental Conservation.
Møllhausen, M., Thorsheim, F., and Herzke, D. (2017). “Rapport fra undersøkelser om svinn av gummigranulat fra kunstgressbaner, gjennomført av over 12 000 elever og spillere høsten 2017,” in Report for Forskningskampanjen, (Stockholm: Swedish Environmental Protection Agency).
Muyssen, B. T. A., De Schamphelaere, K. A. C., and Janssen, C. R. (2006). Mechanisms of chronic waterborne Zn toxicity in Daphnia magna. Aqu. Toxicol. 77, 393–401. doi: 10.1016/j.aquatox.2006.01.006
Nawrocki, S. T., Drake, K. D., Watson, C. F., Foster, G. D., and Maier, K. J. (2005). Comparative aquatic toxicity evaluation of 2-(Thiocyanomethylthio)benzothiazole and selected degradation products using ceriodaphnia dubia. Arch. Environ. Contaminat. Toxicol. 48, 344–350. doi: 10.1007/s00244-004-0105-1
Neumann, G., Veeranagouda, Y., Karegoudar, T. B., Sahin, Ö, Mäusezahl, I., Kabelitz, N., et al. (2005). Cells of Pseudomonas putida and Enterobacter sp. adapt to toxic organic compounds by increasing their size. Extremophiles 9, 163–168. doi: 10.1007/s00792-005-0431-x
Nilsson, N. H., Malmgren-Hansen, B., and Sognstrup Thomsen, U. (2008). “Mapping, emissions and environmental and health assessment of chemical substances in artificial turf,” in Survey of Chemical Substances in Consumer Products, (Taastrup: The Danish Technological Institute).
Redondo-Hasselerharm, P. E., De Ruijter, V. N., Mintenig, S. M., Verschoor, A., and Koelmans, A. A. (2018). Ingestion and chronic effects of car tire tread particles on freshwater benthic macroinvertebrates. Environ. Sci. Technol. 52, 13986–13994. doi: 10.1021/acs.est.8b05035
Rhodes, E. P., Ren, Z., and Mays, D. C. (2012). Zinc leaching from tire crumb rubber. Environ. Sci. Technol. 46, 12856–12863. doi: 10.1021/es3024379
Rian, M. B., Vike-Jonas, K., Gonzalez, S. V., Ciesielski, T. M., Venkatraman, V., Lindstrøm, U., et al. (2020). Phthalate metabolites in harbor porpoises (Phocoena phocoena) from Norwegian coastal waters. Environ. Int. 137:105525. doi: 10.1016/j.envint.2020.105525
RIVM (2016). Beoordeling Gezondheidsrisico’s Door Sporten op Kunstgrasvelden Met Rubbergranulaat. Netherlands: Kenniscentrum Sport & Bewegen.
Rodgers, B., and Waddell, W. (2013). “The science of rubber compounding,” in The Science and Technology of Rubber, 4th Edn, eds J. E. Mark, B. Herman, and C. M. Roland (Amsterdam: Elsevier), 417–470.
Rogge, W. F., Hildemann, L. M., Mazurek, M. A., Cass, G. R., and Simoneit, B. R. T. (1993). Sources of fine organic aerosol. 3. Road dust, tire debris, and organometallic brake lining dust: roads as sources and sinks. Environ. Sci. Technol. 27, 1892–1904. doi: 10.1021/es00046a019
Rosnow, R. L., and Rosenthal, R. (1996). Computing contrasts, effect sizes, and counternulls on other people’s published data: general procedures for research consumers. Pyschol. Methods 1, 331–340. doi: 10.1037/1082-989x.1.4.331
Ruffino, B., Fiore, S., and Zanetti, M. C. (2013). Environmental–sanitary risk analysis procedure applied to artificial turf sports fields. Environ. Sci. Pollut. Res. 20, 4980–4992. doi: 10.1007/s11356-012-1390-2
Sadiktsis, I., Bergvall, C., Johansson, C., and Westerholm, R. (2012). Automobile Tires—A potential source of highly carcinogenic dibenzopyrenes to the environment. Environ. Sci. Technol. 46, 3326–3334. doi: 10.1021/es204257d
Savoca, D., Arculeo, M., Barreca, S., Buscemi, S., Caracappa, S., Gentile, A., et al. (2018). Chasing phthalates in tissues of marine turtles from the Mediterranean sea. Mar. Pollut. Bull. 127, 165–169. doi: 10.1016/j.marpolbul.2017.11.069
Selbes, M., Yilmaz, O., Khan, A. A., and Karanfil, T. (2015). Leaching of DOC, DN, and inorganic constituents from scrap tires. Chemosphere 139, 617–623. doi: 10.1016/j.chemosphere.2015.01.042
Simon, R. (2010). Review of the Impacts of Crumb Rubber in Artificial Turf Applications. Oakland, CA: University of California.
Smolders, E., and Degryse, F. (2002). Fate and effect of zinc from tire debris in soil. Environ. Sci. Technol. 36, 3706–3710. doi: 10.1021/es025567p
Sørensen, L., Rogers, E., Altin, D., Salaberria, I., and Booth, A. M. (2020). Sorption of PAHs to microplastic and their bioavailability and toxicity to marine copepods under co-exposure conditions. Environ. Pollut. 258:113844. doi: 10.1016/j.envpol.2019.113844
Staniszewska, M., Nehring, I., and Mudrak-Cegiołka, S. (2016). Changes of concentrations and possibility of accumulation of bisphenol A and alkylphenols, depending on biomass and composition, in zooplankton of the Southern Baltic (Gulf of Gdansk). Environ. Pollut. 213, 489–501. doi: 10.1016/j.envpol.2016.03.004
US EPA, and CDC/ATSDR (2019). Synthetic Turf Field Tire Crumb Rubber Research Under the Federal Research Action Plan Final Report: Part 1 - Tire Crumb Characterization (Volumes 1 and 2). Washington, DC: U.S. Environmental Protection Agency.
Wagner, S., Hüffer, T., Klöckner, P., Wehrhahn, M., Hofmann, T., and Reemtsma, T. (2018). Tire wear particles in the aquatic environment - A review on generation, analysis, occurrence, fate and effects. Water Res. 139, 83–100. doi: 10.1016/j.watres.2018.03.051
Wik, A., and Dave, G. (2005). Environmental labeling of car tires—toxicity to Daphnia magna can be used as a screening method. Chemosphere 58, 645–651. doi: 10.1016/j.chemosphere.2004.08.103
Wik, A., and Dave, G. (2006). Acute toxicity of leachates of tire wear material to Daphnia magna—Variability and toxic components. Chemosphere 64, 1777–1784. doi: 10.1016/j.chemosphere.2005.12.045
Wik, A., and Dave, G. (2009). Occurrence and effects of tire wear particles in the environment – A critical review and an initial risk assessment. Environ. Pollut. 157, 1–11. doi: 10.1016/j.envpol.2008.09.028
Keywords: additives, organic compounds, metals, copepod, acute toxicity, zinc, benzothiazole, arctic
Citation: Halsband C, SØrensen L, Booth AM and Herzke D (2020) Car Tire Crumb Rubber: Does Leaching Produce a Toxic Chemical Cocktail in Coastal Marine Systems? Front. Environ. Sci. 8:125. doi: 10.3389/fenvs.2020.00125
Received: 30 April 2020; Accepted: 07 July 2020;
Published: 23 July 2020.
Edited by:
Antonia Praetorius, University of Amsterdam, NetherlandsReviewed by:
Nadia Valentina Martínez-Villegas, Instituto Potosino de Investigación Científica y Tecnológica (IPICYT), MexicoAmrika Deonarine, Texas Tech University, United States
Copyright © 2020 Halsband, Sørensen, Booth and Herzke. This is an open-access article distributed under the terms of the Creative Commons Attribution License (CC BY). The use, distribution or reproduction in other forums is permitted, provided the original author(s) and the copyright owner(s) are credited and that the original publication in this journal is cited, in accordance with accepted academic practice. No use, distribution or reproduction is permitted which does not comply with these terms.
*Correspondence: Claudia Halsband, Y2xhdWRpYS5oYWxzYmFuZEBha3ZhcGxhbi5uaXZhLm5v