- 1Soil Biology Department, Institute of Soil Science and Land Evaluation, Faculty of Agricultural Sciences, University of Hohenheim, Stuttgart, Germany
- 2Ecology Group, Institute of Biology, Humboldt-Universität zu Berlin, Berlin, Germany
- 3Research Unit Biodiversity and Scientific Diving, Institute of Biomaterials and Biomolecular Systems, Faculty of Energy, Process and Biotechnology, University of Stuttgart, Stuttgart, Germany
- 4Biogeophysics Department, Institute of Soil Science and Land Evaluation, Faculty of Agricultural Sciences, University of Hohenheim, Stuttgart, Germany
Microplastics (MP) are pervasive in the environment. There is ample evidence of negative MP effects on biota in aquatic ecosystems, though little is known about MP effects in terrestrial ecosystems. Given numerous entry routes of MP into soils, soil organisms are likely to be exposed to MP. We compared potential toxicological effects of MP from (i) low-density polyethylene (LDPE) (mean diameter ± standard deviation: 57 ± 40 μm) and (ii) a blend of biodegradable polymers polylactide (PLA) and poly(butylene adipate-co-terephthalate) (PBAT) (40 ± 31 μm) on the reproduction and body length of the soil-dwelling bacterivorous nematode Caenorhabditis elegans. Feed suspensions without (control) or with MP (treatments) at concentrations of 1, 10, and 100 mg MP L–1 were prepared and nematodes were exposed to those suspensions on agar plates until completion of their reproductive phase (∼6 days). Using Nile red-stained PLA/PBAT MP particles and fluorescence microscopy, we demonstrated the ingestion of MP by C. elegans into pharynges and intestines. Under MP exposure, nematodes had fewer offspring (up to 22.9%) compared to nematodes in the control group. This decline was independent on the plastic type. We detected a tendency toward greater decreases in offspring at higher concentrations. Despite hints of negative effects on nematode body length under MP exposure, we could not derive a consistent pattern. We conclude that in MP-contaminated soils, the reproduction of nematodes, central actors in the soil food web, can be affected, with potentially negative implications for key soil functions, e.g., the regulation of soil biogeochemical cycles.
Introduction
Microplastics (MP) have only recently been recognized as an environmental threat to terrestrial ecosystems. MP are plastic particles smaller than 5 mm, and with different shapes such as spheres, fibers, and fragments (de Souza Machado et al., 2018; Rillig et al., 2019). Although reliable data on the prevalence of MP in soils is scarce (Watteau et al., 2018), soils are presumably large sinks for MP and MP may harm soil organisms (Bläsing and Amelung, 2018; Hurley and Nizzetto, 2018).
It has been shown for aquatic organisms such as mussels, langoustines, copepods, short crabs, and lugworms that the ingestion of MP can lead to negative effects on growth, reproduction and survival (Galloway et al., 2017; Foley et al., 2018; Franzellitti et al., 2019). These detrimental effects can be nutritional, a result of lower food intake resulting in energy deficiencies (Franzellitti et al., 2019), but also physical, due to lacerations and inflammations (Horton et al., 2017). In contrast, little is known about MP effects on soil fauna (Chae and An, 2018). Early studies on earthworms under MP exposure indicated that some biological functions could be inhibited (Huerta Lwanga et al., 2016; Cao et al., 2017). One study documented histopathological damage, including congestion fibrosis and inflammatory infiltrates in earthworms in response to MP exposure, although no biological functions were affected (Rodriguez-Seijo et al., 2017). Wang et al. (2019) found oxidative stress in earthworms in response to artificially high MP exposure only. Translocation of MP by earthworms (Huerta Lwanga et al., 2017a; Rillig et al., 2017b) and collembola (Maaß et al., 2017) could increase the exposure of other soil-dwelling species to MP. In addition, evidence on the accumulation of MP from soil to earthworms to chicken feces (Huerta Lwanga et al., 2017b) indicates that MP may enter terrestrial food webs through trophic transfers.
Nematodes (roundworms) live in any terrestrial habitat, exhibit high diversity across soils (Yeates and Bongers, 1999), and have a wide range of diets (Yeates et al., 1993; Orgiazzi et al., 2016). By regulating biogeochemical cycles and ecosystem processes, including mineralization and decomposition of organic matter (Griffiths et al., 1998; Bardgett et al., 1999; Ferris, 2010), they are key organisms in the soil food web. The soil-dwelling bacterivorous nematode Caenorhabditis elegans, distributed world-wide, is a well-established model organism for ecotoxicological tests of different kinds of pollutants such as mycotoxins, persistent organic pollutants and endocrine-disrupting compounds (Leung et al., 2008; Keller et al., 2018; Chen et al., 2019) and has been used for biosafety assessments of nanoparticles (Wu et al., 2019). Fang-Yen et al. (2009) observed that C. elegans can ingest polystyrene beads that can further be transported into the intestine, a possible entry route of MP into the soil food web (Rillig et al., 2017a). An early study on exposure of C. elegans to MP reported that MP could lead to inhibition of survival rates, body length, and reproduction, as well as intestinal damage and oxidative stress. While MP effects were independent on plastic type, MP effects were dependent on particle size (Lei et al., 2018b): MP particles of one μm led to the strongest effects when three different sizes (0.1, 1, and 5 μm) were compared.
Biodegradable plastics are considered an environmentally friendly alternative to conventional plastics, as they theoretically can be completely metabolized by microorganisms without leaving plastic residues in the environment (Bandopadhyay et al., 2018; Sander, 2019). For instance, polylactide (PLA) and poly(butylene adipate-co-terephthalate) (PBAT) are common components of biodegradable plastic films which can be substituted for low-density polyethylene (LDPE) films (Künkel et al., 2016). However, there is some evidence that even nominally biodegradable plastics tend to disintegrate instead of being mineralized (de Souza Machado et al., 2018). A recent study demonstrated that even after 3 years, large quantities of commercially available, biodegradable plastic carrier bags were still present in soils and other environmental compartments (Napper and Thompson, 2019).
Our study aimed to compare possible effects of irregularly shaped MP particles of the conventional plastic type LDPE and a biodegradable blend of PLA/PBAT on nematodes. We evaluated the biological endpoints of reproduction and body length in the model organism C. elegans in response to MP exposure at different concentrations. We hypothesized that (i) C. elegans can ingest MP and (ii) MP adversely affect reproduction and body length of C. elegans, with stronger negative impacts at higher MP concentrations. Furthermore, we expected to observe comparable effects of both conventional and biodegradable MP.
Materials and Methods
Microplastic Preparation and Characteristics
Two types of plastics were used in the experiment: (1) low-density polyethylene (LDPE) in the form of granules (Lupolen 2420 H, LyondellBasell Industries N.V., Rotterdam, Netherlands) and (2) a blend consisting of the polymers polylactide (PLA, IngeoTM Biopolymer 7001D, NatureWorks LLC, Minnetonka, MN, United States) and poly(butylene adipate-co-terephthalate) (PBAT, Ecoflex F Blend C1200, BASF SE, Ludwigshafen, Germany) with a mixing ratio of 80/20% w/w compounded at the “Institut für Kunststofftechnik” (University of Stuttgart, Stuttgart, Germany).
We used irregularly shaped MP particles because a non-spherical shape can be expected due to input of fragmented MP from mulch film and plastic bag residues in compost into soils. The particle size ingestible by C. elegans is smaller than 3 μm (Fang-Yen et al., 2009). However, it is very challenging, to produce irregularly shaped MP particles with defined size distribution <50 μm. For our experiments, plastic granules were first ground to MP particles <5 mm with a speed rotor mill (Pulverisette, Fritsch GmbH, Idar-Oberstein, Germany) and later milled to smaller fragments using a cryomill with liquid nitrogen (Cryomill, Retsch, Haan, Germany) at the Fraunhofer Institute for Chemical Technology (Pfinztal, Germany). This procedure yielded particle sizes of 57 ± 40 μm (LDPE) and 40 ± 31 μm (PLA/PBAT) (Figure 1). Due to light microscopy detection limits at ≤3 μm (see Supplementary Material 1), we could not differentiate particles ≤3 μm. The proportions of particles ≤3 μm (ingestible for C. elegans) were 8.0% (LDPE) and 7.4% (PLA/PBAT). Particles of both plastic types were similarly shaped, as shown by their form factors (Supplementary Figure 1). A detailed description of particle characteristics is given in Supplementary Material 1.
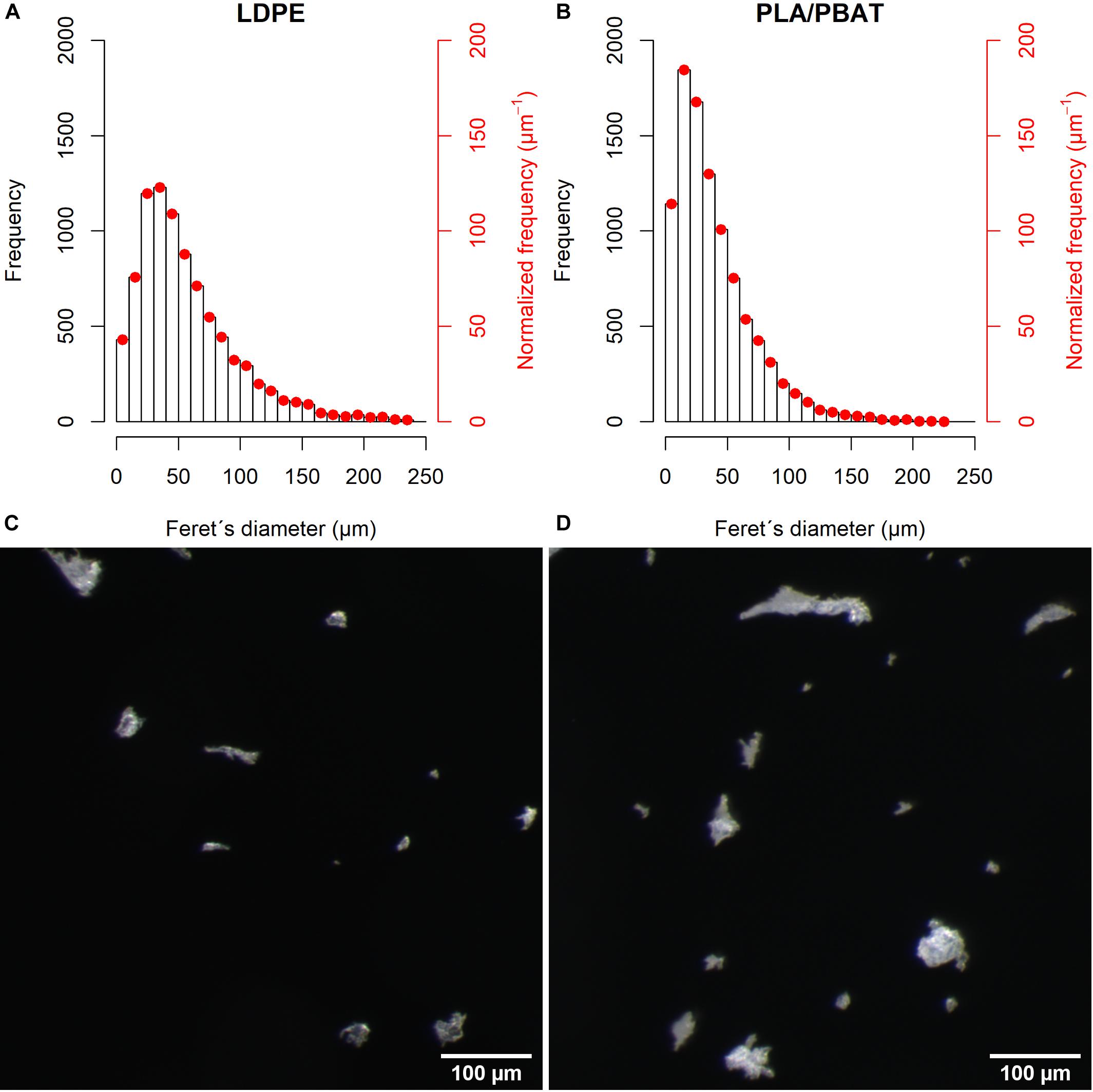
Figure 1. The particle size distribution of (A) LDPE and (B) PLA/PBAT. The second ordinate shows the normalized frequency according to Filella (2015). Micrograph of (C) LDPE and (D) PLA/PBAT.
Cultivation of C. elegans and Preparation of MP Feed Suspensions
We used the C. elegans wild-type strain N2 in our assays, which was obtained from the Caenorhabditis Genetics Center (University of Minnesota). C. elegans was fed with Escherichia coli OP50 and cultivated on Nematode Growth Medium agar plates. For both assays, L1 nematodes were used. L1 refers to nematodes from the first of four larval stages in the life cycle of C. elegans before they become fertile adults (Gonzalez-Moragas et al., 2015).
For the treatments, MP feed suspensions were prepared at concentrations of 1, 10, and 100 mg MP L–1. The MP feed suspensions consisted of M9 buffer, a common worm buffer for handling C. elegans (He, 2011), freshly harvested E. coli OP50 from overnight cultures and MP. For the control group, feed suspensions of M9 buffer and freshly harvested E. coli OP50 without MP were prepared. All feed suspensions (with and without MP) were then shaken for 30 min and placed in an ultrasonic bath for 15 min to prevent agglomeration of the particles in the treatments. Before an aliquot of 100 μl of the feed suspensions were added to the agar plates, the suspensions were vortexed for 10 s. Following the addition of the feed suspensions to the agar plates, they were dried under the laboratory hood and incubated at 19.5°C for 12 h. The entire procedure was performed under sterile conditions.
Ingestion Assay
Nematodes were exposed to Nile red (NR) stained PLA/PBAT particles at a concentration of 100 mg L–1. For this purpose, PLA/PBAT particles were colored with the fluorescent dye NR (72485, Sigma-Aldrich, St. Louis, MO, United States) that was recently used for the detection of MP in environmental samples (e.g., Shim et al., 2016; Maes et al., 2017). For staining of the MP particles, a NR stock solution of 1 mg L–1 methanol was first prepared. MP particles were stained with a NR working solution of 10 μg ml–1 by shaking the suspensions at 35 rpm for 1 h on a laboratory shaker (Roto-Shake Genie, Scientific Industries, Inc., New York, NY, United States). After incubation, the suspensions were transferred to glass Petri dishes where the solvents evaporated under the laboratory hood. The stained MP particles were used for the preparation of MP feed suspensions. Five nematodes were taken from a pre-culture and added to Nematode Growth Medium agar plates prepared with MP feed suspensions. The adult nematodes were transferred to new plates after 3 and 6 days, respectively. After 9 days of incubation, images were taken with a fluorescence microscope using an excitation wavelength of 510–560 nm and emission wavelength >590 nm (Axiophot with filter set 487914/analogous to current filter set 14, Carl Zeiss Microscopy GmbH, Jena, Germany). The Feret’s diameter of MP particles incorporated by C. elegans was determined using Fiji 1.52p (Schindelin et al., 2012; Schneider et al., 2012). For better visualization, the particles were pseudo-colored on the images.
Reproduction and Body Length Assay
To exclude potential side effects of NR, here we only used non-stained MP particles. The experimental design consisted of two plastic types (LDPE or PLA/PBAT) at three different concentrations (1, 10, and 100 mg L–1) and a control without MP addition (each n = 8).
L1 nematodes were individually picked from a pre-culture with a smoothed platinum picker and placed on the agar plates (one nematode per plate) prepared with feed suspensions with MP (treatments) or without (control). Subsequently, the nematodes were exposed to these feed suspensions on the agar plates at 19.5°C until oviposition (∼3 days). At intervals of 24 h the nematodes were transferred to new agar plates prepared with the nutrient suspensions with MP (treatments) or without (control) until the end of the reproduction phase (∼3 days). In total, nematodes of the treatment groups were exposed to MP for 6 days.
Nematode offspring per 24 h were counted optically with a stereomicroscope (Nikon SMZ1000, Nikon, Tokyo, Japan). The body length of the adult nematodes that survived the reproduction phase was determined using a stereomicroscope with camera (Zeiss Axio Scope.A1 & AxioCam ICc 5, Carl Zeiss Microscopy GmbH, Jena, Germany) and Fiji 1.52p. Before taking images, the nematodes were anesthetized with 20 mM tetramisole hydrochloride (L9756, Sigma-Aldrich, St. Louis, MO, United States).
Statistics
For data analysis, we used the statistical software R (R Core Team, 2018). To examine our hypotheses, we fitted a one-way analysis of variance model to our data and specified linear contrasts of interest as proposed by Mangiafico (2015) using the “glht” command from the R package “multcomp” (Hothorn et al., 2008). To test for an effect of MP on reproduction and body length, we compared each treatment to the control group. To clarify differences between the plastic types, we compared LDPE and PLA/PBAT per concentration level. To test the assumption that higher concentrations would lead to stronger effects, we compared the higher to the lower concentrations for each plastic type. We adjusted the p-values according to Benjamini and Hochberg (1995), to correct for several comparisons. Following the recommendation of Wasserstein et al. (2019), we did not define a significance level and deliberately omitted the term “statistically significant.” The results of the statistical tests are given in Supplementary Table 2. We confirmed the model assumptions of the ANOVA visually by residual diagnostics plots (Kozak and Piepho, 2018).
We only considered nematodes that remained alive until the completion of the reproductive phase. Underdeveloped worms and worms that died before completing the reproductive phase from unexplained causes of death (e.g., mechanical damage) were excluded from the analysis. This resulted in an unbalanced design with at least n = 5 (Supplementary Table 1).
Results
Ingestion Assay
We confirmed the uptake of NR stained PLA/PBAT MP particles in the pharynx and posterior intestinal lumen of C. elegans by fluorescence microscopy (Figures 2A,B). The particles ingested by C. elegans displayed in Figure 2, in the pharynx and intestine had a Feret’s diameter of 2.3–5.1 μm and 1.3–2.5 μm, respectively.
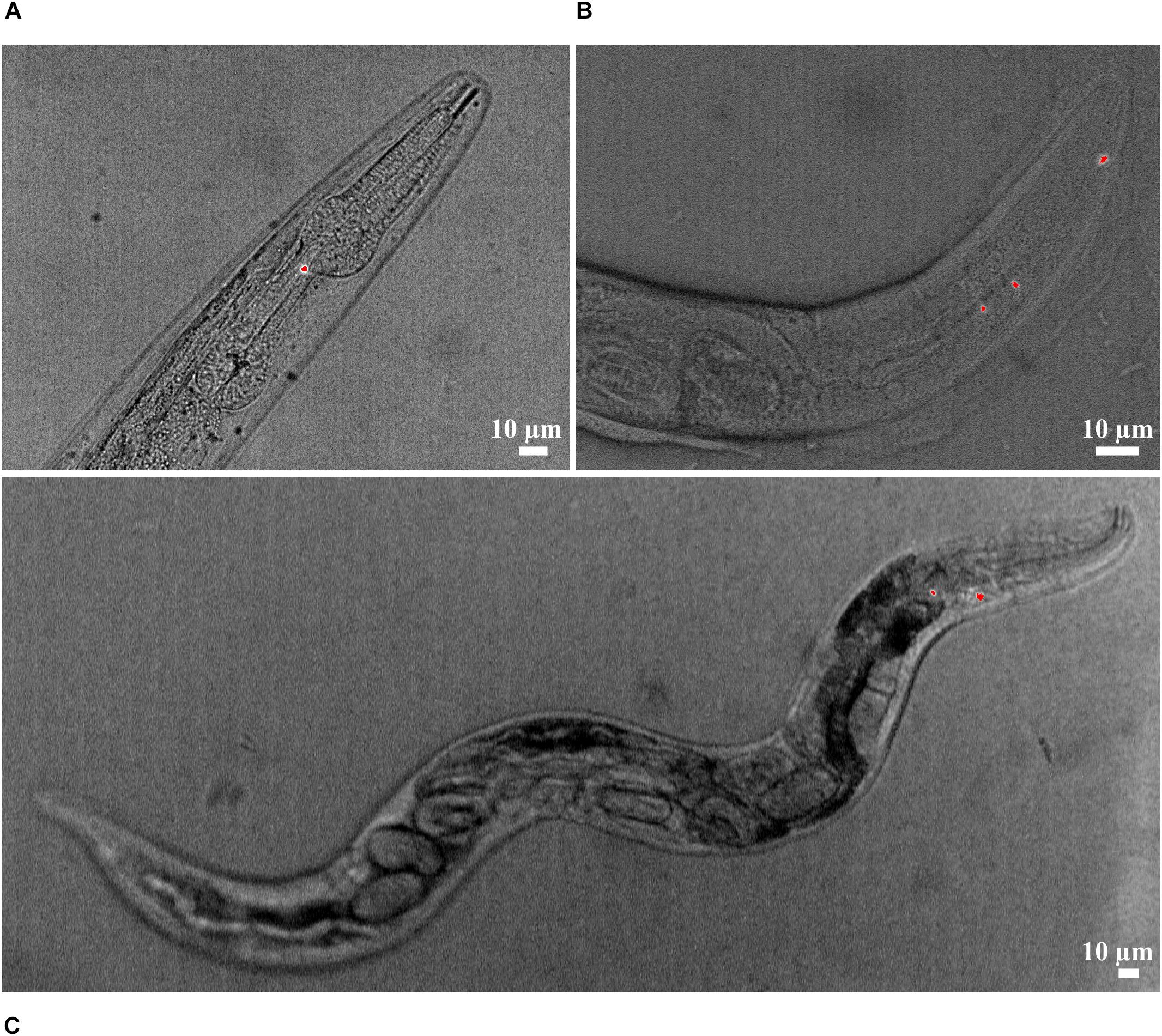
Figure 2. Ingestion of NR-stained PLA/PBAT particles (red) in the (A) pharynx and (B) intestine lumen of C. elegans. (C) Nematode with NR-stained PLA/PBAT particles in the pharynx and internal hatching.
Surprisingly, internal hatching of larvae was observed in a nematode with particles in the pharynx (Figure 2C). This phenomenon could also be observed in four nematodes from the reproduction assay exposed to 10 (n = 1) and 100 mg L–1 LDPE (n = 2) and 100 mg L–1 PLA/PBAT (n = 1). These individuals were not considered in the statistical analysis because they died before the completion of the reproductive phase. In the control group without MP, internal hatching did not occur.
Reproduction and Body Length Assay
Number of nematode offspring in the control group was 267 ± 6 (mean ± SE) (Figure 3A). Under MP exposure, nematodes produced 4.6–22.9% fewer offspring than nematodes in the control group. The strongest reduction in comparison to the control group was found at 10 mg L–1 LDPE (p = 0.03). For both plastic types, we observed a tendency toward stronger declines at higher concentrations. Under exposure to 10 and 100 mg L–1 compared to 1 mg L–1 LDPE, offspring declined by 18.4% (p = 0.08) and 9.9% (p = 0.37) stronger relative to the control. Exposure to 10 and 100 mg L–1 compared to 1 mg L–1 PLA/PBAT, resulted in declines relative to the control which were by 4.7% (p = 0.63) and 6.5% (p = 0.49) stronger. We found only marginal differences in offspring between LDPE and PLA/PBAT at all concentration levels (Supplementary Table 2). The coefficient of variation (CV) of the treatment groups (9.2–17.5%) was higher than the CV of the control group (5.5%).
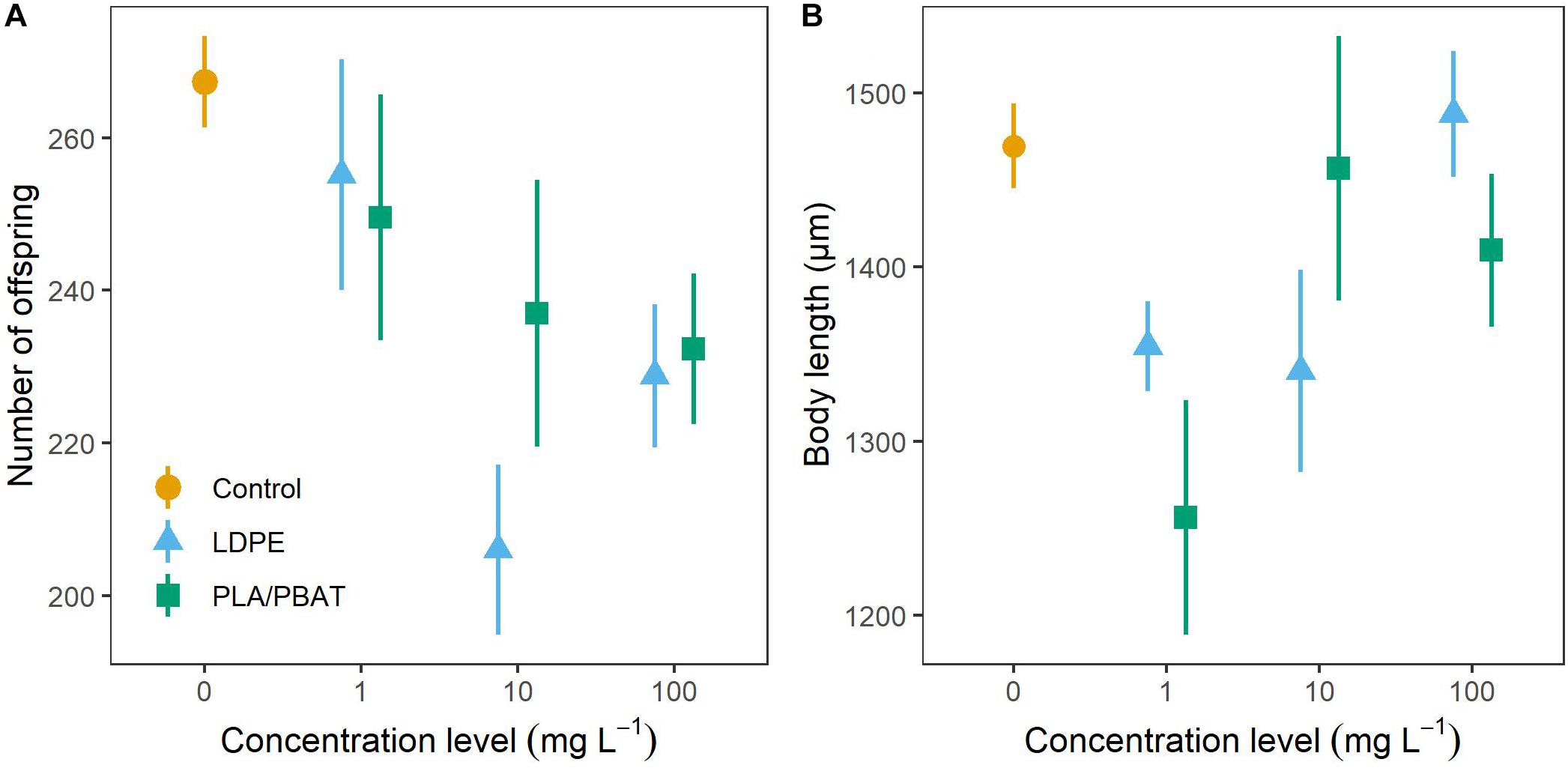
Figure 3. (A) Number of offspring and (B) body length of C. elegans as a function of concentration and plastic type compared to the control group. Data are presented as means ± SE.
In the control group, body length of the nematodes was 1,470 ± 24 μm (Figure 3B). We could not observe a clear pattern for the body length of nematodes exposed to MP. Body length decreased most strongly relative to the control at exposure level of 1 mg L–1 PLA/PBAT (14.5%, p = 0.06) and at both 1 mg L–1 (7.8%, p = 0.25), and 10 mg L–1 (8.8%, p = 0.21) LDPE. Observed body lengths in all other treatments were close to the body length of the control group. Body length was not influenced by plastic type. With a CV of 4.1%, the control group exhibited lesser variance than the treatment groups, the CVs of which ranged from 4.7 to 14.2%.
Discussion
By demonstrating that C. elegans can and does ingest MP particles, we established one prerequisite for the potential development of toxic effects (Horton et al., 2017; Kim and An, 2019) in C. elegans. Particle ingestion is generally controlled by a species-specific particle-to-mouth size ratio that defines the size of the particles which are ingestible by a particular species (Horton et al., 2017). Recently, Mueller et al. (2020) provided evidence for earlier findings from Fang-Yen et al. (2009) that the ingestible particle size of C. elegans is limited by the size of the buccal cavity, which in their study developed to 4.4 ± 0.5 μm. While polystyrene (PS) beads <3 μm entered the entire intestinal system of C. elegans, particles >6 μm did not enter the body of the nematodes at all (Mueller et al., 2020). In agreement with these findings, we detected in our study MP particles with sizes of 2.3–5.1 μm and 1.3–2.5 μm in the pharynges and intestines of C. elegans, respectively.
We found that MP reduced offspring of C. elegans by 4.5–22.9%, with a tendency toward greater declines in offspring at higher MP concentrations. The strong decline in offspring at 10 mg L–1 compared to the control might indicate the existence of a critical effect concentration of MP. Contra-intuitively, the decrease was more pronounced at 10 mg L–1 LDPE than at 100 mg L–1 LDPE which we attribute to the statistical uncertainties of the comparison between these treatments (p = 0.44, see Supplementary Table 2). The existence of a critical effect concentration is supported by observations in Lei et al. (2018a), who found clear lethal effects of PS beads in C. elegans at concentrations higher than 5 mg L–1. Mueller et al. (2020) established clear dose-response curves for the reproduction of nematodes exposed to PS beads from 0.1 to 10 μm, with smaller particles exerting a stronger toxicity. Only 0.1 μm-sized particles caused effects on nematode reproduction with an EC50 at 77 mg L–1 (all units converted) below the highest concentration level of 100 mg L–1 used in our study, while larger particles caused inhibitions in reproduction at considerably higher concentrations (Mueller et al., 2020). Lei et al. (2018b) compared the toxic effects of five different MP types of irregular shape (mean diameter: ∼70 μm) on body length, embryo number, brood size, calcium levels in the intestine, and the expression of stress-indicating enzyme activity of C. elegans. Additionally, they compared the ingestion and toxic effects of PS beads of different sizes (0.1, 1, and 5 μm). Lei et al. (2018b) found offspring reductions of 2.4–28.0%, which were similar to the offspring reductions observed in our study. They found that body length was reduced by 4.9–11.4% when nematodes were exposed to MP, while we could not observe a clear MP effect pattern, as some MP treatments showed reductions compared to the control, while others did not.
Given the estimated fraction of MP particles <3 μm used in our experiments of 8.0 (LDPE) and 7.4% (PLA/PBAT), the concentration levels of 1, 10, and 100 mg L–1 in our study translate into 0.08, 0.8, 8.0 mg L–1 and 0.074, 0.74, to 7.4 mg L–1 in the ingestible range for C. elegans. Thus, in Mueller et al. (2020) the likelihood of an ingestion of MP by C. elegans was much higher than in our study, since they used spherical particles in the size range of 0.1–3 μm at concentrations of 40–12,500 mg L–1. The authors found effects on reproduction at considerably higher concentrations than >100 mg L–1 (exception: 0.1 μm –sized particles with an EC50 of 77 mg L–1), which indicates that the observed toxicity of MP in our study at much lower concentrations of ingestible particles was not solely attributed to the ingestion of particles. While direct toxicity of ingested MP particles was shown for 1 μm particles that were preferably ingested and accumulated in the intestines of C. elegans (Lei et al., 2018b), there is some experimental evidence that negative effects on reproduction of C. elegans are regulated by the ratio of the total surface area of MP particles to the volume of the medium, where the MP is contained (Mueller et al., 2020). Yet, the underlying mechanism of such a surface-related toxicity still needs to be clarified. Mueller et al. (2020) proposed as possible mechanisms of the surface-related toxicity the binding of food bacteria to MP and a dilution of food bacteria by MP, which could result in a limitation of food availability for the nematodes. In our study, surface-related toxicity could explain, why the MP particles reduced offspring although most of the particles were not directly ingestible by nematodes. Due to their higher specific surface area, irregularly shaped particles (used in our study) presumably lead to higher surface-related toxicity at lower concentrations compared to spherical MP particles (used in Mueller et al., 2020). Hence, toxic effects of MP are also likely to be controlled by particle shape.
In line with Lei et al. (2018b), negative effects on the reproduction of C. elegans under MP exposure occurred irrespective of the plastic type. Since LDPE and PLA/PBAT particles in our study had a similar shape and size, the observed reductions in offspring were probably attributable to physical effects, such as intestinal damage (Lei et al., 2018b) or indirect nutritional effects due to interaction of particles with the food bacteria (Mueller et al., 2020). Natural particles such as mesoporous SiO2 particles, which can be found in soils, can also be ingested by C. elegans (Acosta et al., 2018). However, only exposure to nano-sized particles led to reductions in lifespan, mobility, and reproduction, while micro-sized particles showed no effect (Acosta et al., 2018). Mueller et al. (2020) compared the effects of SiO2 particles to PS beads of equal size and showed that SiO2 particles exhibited a clearly lower toxicity, which was indicated by toxic effects at significantly higher particle to bacteria ratio. The authors claimed that the specific density of a material played an important role for the toxicity, because particles with specific density in the range of the bacteria like plastics would be taken up more readily.
We found a greater variance within our MP treatments than in the control treatment for both offspring and body length. One possible explanation is that not all nematodes exposed to MP had ingested them, such that only worms that ingested MP were affected. This was not possible to check, however, as we used non-stained MP in the reproduction and body length assay, and this was not detectable under the microscope inside the nematode bodies. Furthermore, it is possible that the total surface of the particles in the MP feed suspensions differed between the replications within and among the groups due to discrepancies in particle compositions present in the respective suspensions.
Studies of MP effects on other soil-dwelling animals have also reported negative effects of MP on some biological functions, with other functions unaffected. For instance, under high MP exposure, mortality level of the earthworm Lumbricus terrestris increased, growth inhibited, and biomass reduced, whereas even at higher concentrations no effect on reproduction was found (Huerta Lwanga et al., 2016). In comparison, Rodriguez-Seijo et al. (2017) observed no adverse effects on survival, reproduction, or body weight of the earthworm Eisenia andrei, but did find gut damage and histological alterations including congestion and fibrosis. Under MP exposure, the earthworm Eisenia fetida showed only marginal effects, with the anti-oxidase system negatively affected only at artificially high concentrations (Wang et al., 2019), while the isopod Porcellio scaber was not affected by MP at all (Jemec Kokalj et al., 2018). In view of our results and those from these studies, it appears that not all species or their biological endpoints are sensitive to MP, as our findings indicate that body length is a non-sensitive metric for MP toxicity in C. elegans. The fact that some biological endpoints were affected by MP and others were not may be explained by the disposable soma theory (Kirkwood, 1977). According to this theory, an organism can try to compensate for stress, in our case induced by MP. This stress and its compensation may lead to an imbalance of biological functions, as one function decreases at the expense of maintenance of another.
Remarkably, in the ingestion assay, we observed that several nematodes that ingested MP (not quantified, however) exhibited internal hatching (Figure 2C). In the reproduction assay, four nematodes which had been exposed to MP and died during the exposure also showed internal hatching. Generally, the phenomenon of internal hatching, also referred to as matricide, can occur under conditions of stress, e.g., starvation, exposure to toxins, or presence of bacteria (Mosser et al., 2011; Pestov et al., 2011). Nematodes, supposedly in response to stress, lay their eggs internally to ensure survival of their offspring, as the offspring receive sufficient nutrients in the body of the adult nematode. However, in our study, it was not clear whether internal hatching was induced directly by MP. Kiyama et al. (2012) observed that in the presence of food, uptake of MP was strongly reduced. Conversely, under the condition of food deficiency, more particles were taken up. A possible scenario in our study could have been that a combined effect of MP particle consumption and starvation would have led to internal hatching. The potential link of MP uptake and internal hatching should be investigated quantitatively in future studies.
We are aware that in our approach exposure of C. elegans to MP was rather artificial (MP feed suspensions on agar plates). We chose this exposure, though, because we aimed at understanding the general potential of MP to develop a toxicity in C. elegans. In soils, it would not have been possible to achieve a general process understanding. The design of more realistic experimental setups with soils is challenging because currently there are only few data on MP contamination in soils and it is not clear whether the MP concentrations found to date in urban (0.3–67.5 g kg–1), riparian (0–0.055 g kg–1), and agricultural soils (0–42,960 particles kg–1) (Helmberger et al., 2019) lead to negative effects on soil biota. Due to detection limits, even less data is available in environmental samples for MP particles <10 μm (Haegerbaeumer et al., 2019) and thus for the ingestible size range of C. elegans. Given the aliquot of 100 μl that we gave to each nematode in our experiments, one nematode was effectively exposed to 0.1, 1, and 10 μg of MP. Based on a global median abundance of 12,030 individuals per 1 kg dry soil (van den Hoogen et al., 2019) and the mass-based MP concentrations reported in soil (see above), this translates into an exposure to 0–4.6 μg per nematode for riparian and 25–5,600 μg per nematode for soils in industrial areas. Thus, the applied MP amounts in our study are in a range typically found in natural soils.
Conclusion
We found that nematodes can ingest MP particles which might negatively affect their reproduction. Toxic effects of MP on nematode reproduction in soils cannot be ruled out. The toxicity risk for conventional and biodegradable MP particles is likely to be the same, as MP toxicity is rather attributable to physical and indirect nutritional effects rather than to chemical effects. Although we have hints of negative effects of MP on the body length of nematodes, our results are not conclusive. Since nematodes, as key members of the soil food web, may be at risk under MP exposure, our results suggest potentially negative implications for important soil functions, e.g., the regulation of biogeochemical cycles. Further studies are needed to estimate critical effect concentrations and to elucidate the influence of particle shape for nematodes under realistic exposure scenarios in soils.
Data Availability Statement
The datasets generated for this study are available on request to the corresponding author.
Author Contributions
LS, SM, EK, RM, HP, and LR contributed conception and design of the study. LS performed the ingestion assay as well as the reproduction and body length assay supervised by RM and LR. LS conducted the data evaluation and statistics supported by HP. US determined the size distribution and characteristics of the microplastic particles supervised by FB. LS wrote the first draft of the manuscript. All authors contributed to manuscript revision, read and approved the submitted version.
Funding
The research is part of the project MiKoBo (Mikrokunststoffe in Komposten und Gärprodukten aus Bioabfallverwertungsanlagen und deren Eintrag in Böden – Erfassen, Bewerten, Vermeiden) which is funded by the Ministry of Environment, Climate and Energy Baden-Württemberg in the framework of BWPLUS – Baden-Württemberg Programm Lebensgrundlage Umwelt und ihre Sicherung (reference number: BWMK18003). HP was financially supported by the Ellrichshausen Foundation.
Conflict of Interest
The authors declare that the research was conducted in the absence of any commercial or financial relationships that could be construed as a potential conflict of interest.
Acknowledgments
We thank Julia Resch (“Institut für Kunststofftechnik,” Stuttgart, Germany) and Ansilla Bayha (Fraunhofer Institute for Chemical Technology, Pfinztal, Germany) for the grinding and provision of the microplastic particles.
Supplementary Material
The Supplementary Material for this article can be found online at: https://www.frontiersin.org/articles/10.3389/fenvs.2020.00041/full#supplementary-material
References
Acosta, C., Barat, J. M., Martínez-Máñez, R., Sancenón, F., Llopis, S., González, N., et al. (2018). Toxicological assessment of mesoporous silica particles in the nematode Caenorhabditis elegans. Environ. Res. 166, 61–70. doi: 10.1016/j.envres.2018.05.018
Bandopadhyay, S., Martin-Closas, L., Pelacho, A. M., and DeBruyn, J. M. (2018). Biodegradable plastic mulch films: impacts on soil microbial communities and ecosystem functions. Front. Microbiol. 9:819. doi: 10.3389/fmicb.2018.00819
Bardgett, R. D., Cook, R., Yeates, G. W., and Denton, C. S. (1999). The influence of nematodes on below-ground processes in grassland ecosystems. Plant Soil 212, 23–33. doi: 10.1023/A:1004642218792
Benjamini, Y., and Hochberg, Y. (1995). Controlling the false discovery rate: a practical and powerful approach to multiple testing. J. R. Stat. Soc. Ser. B 57, 289–300. doi: 10.1111/j.2517-6161.1995.tb02031.x
Bläsing, M., and Amelung, W. (2018). Plastics in soil: analytical methods and possible sources. Sci. Total Environ. 612, 422–435. doi: 10.1016/j.scitotenv.2017.08.086
Cao, D., Wang, X., Luo, X., Liu, G., and Zheng, H. (2017). Effects of polystyrene microplastics on the fitness of earthworms in an agricultural soil. IOP Conf. Ser. Earth Environ. Sci. 61, 012148. doi: 10.1088/1755-1315/61/1/012148
Chae, Y., and An, Y.-J. (2018). Current research trends on plastic pollution and ecological impacts on the soil ecosystem: a review. Environ. Pollut. 240, 387–395. doi: 10.1016/j.envpol.2018.05.008
Chen, H., Chen, W., Hui, L., Ruixue, M., Ziling, Y., Liangzhong, L., et al. (2019). A review of toxicity induced by persistent organic pollutants (POPs) and endocrine-disrupting chemicals (EDCs) in the nematode Caenorhabditis elegans). J. Environ. Manag. 237, 519–525. doi: 10.1016/j.jenvman.2019.02.102
de Souza Machado, A. A., Kloas, W., Zarfl, C., Hempel, S., and Rillig, M. C. (2018). Microplastics as an emerging threat to terrestrial ecosystems. Global Change Biol. 24, 1405–1416. doi: 10.1111/gcb.14020
Fang-Yen, C., Avery, L., and Samuel, A. D. T. (2009). Two size-selective mechanisms specifically trap bacteria-sized food particles in Caenorhabditis elegans. Proc. Natl. Acad. Sci. U.S.A. 106, 20093–20096. doi: 10.1073/pnas.0904036106
Ferris, H. (2010). Contribution of nematodes to the structure and function of the soil food web. J. Nematol. 42, 63–67.
Filella, M. (2015). Questions of size and numbers in environmental research on microplastics: methodological and conceptual aspects. Environ. Chem. 12:527. doi: 10.1071/EN15012
Foley, C. J., Feiner, Z. S., Malinich, T. D., and Höök, T. O. (2018). A meta-analysis of the effects of exposure to microplastics on fish and aquatic invertebrates. Sci. Total Environ. 63, 550–559. doi: 10.1016/j.scitotenv.2018.03.046
Franzellitti, S., Canesi, L., Auguste, M., Wathsala, R. H. G. R., and Fabbri, E. (2019). Microplastic exposure and effects in aquatic organisms: a physiological perspective. Environ. Toxicol. Pharmacol. 68, 37–51. doi: 10.1016/j.etap.2019.03.009
Galloway, T. S., Cole, M., and Lewis, C. (2017). Interactions of microplastic debris throughout the marine ecosystem. Nat. Ecol. Evol. 1:116. doi: 10.1038/s41559-017-0116
Gonzalez-Moragas, L., Roig, A., and Laromaine, A. (2015). C. elegans as a tool for in vivo nanoparticle assessment. Adv. Colloid Interface Sci. 219, 10–26. doi: 10.1016/j.cis.2015.02.001
Griffiths, B., Ritz, K., Ebblewhite, N., and Dobson, G. (1998). Soil microbial community structure: effects of substrate loading rates. Soil Biol. Biochem. 31, 145–153. doi: 10.1016/S0038-0717(98)00117-5
Haegerbaeumer, A., Mueller, M.-T., Fueser, H., and Traunspurger, W. (2019). Impacts of micro- and nano-sized plastic particles on benthic invertebrates: a literature review and gap analysis. Front. Environ. Sci. 7:1596.
Helmberger, M. S., Tiemann, L. K., and Grieshop, M. J. (2019). Towards an ecology of soil microplastics. Funct. Ecol. 7, 550–560.
Horton, A. A., Svendsen, C., Williams, R. J., Spurgeon, D. J., and Lahive, E. (2017). Large microplastic particles in sediments of tributaries of the river thames, uk - abundance, sources and methods for effective quantification. Mar. Pollut. Bull. 114, 218–226. doi: 10.1016/j.marpolbul.2016.09.004
Hothorn, T., Bretz, F., and Westfall, P. (2008). Simultaneous inference in general parametric models. Biometr. J.. Biometr. Z. 50, 346–363. doi: 10.1002/bimj.200810425
Huerta Lwanga, E., Gertsen, H., Gooren, H., Peters, P., Salánki, T., van der Ploeg, M., et al. (2016). Microplastics in the terrestrial ecosystem: implications for Lumbricus terrestris (oligochaeta, lumbricidae). Environ. Sci. Technol. 50, 2685–2691. doi: 10.1021/acs.est.5b05478
Huerta Lwanga, E., Gertsen, H., Gooren, H., Peters, P., Salánki, T., van der Ploeg, M., et al. (2017a). Incorporation of microplastics from litter into burrows of Lumbricus terrestris. Environ. Pollut. 220, 523–531. doi: 10.1016/j.envpol.2016.09.096
Huerta Lwanga, E., Mendoza Vega, J., Ku Quej, V., Chi, J., de, L. A., Sanchez Del Cid, L., et al. (2017b). Field evidence for transfer of plastic debris along a terrestrial food chain. Sci. Rep. 7, 14071. doi: 10.1038/s41598-017-14588-2
Hurley, R. R., and Nizzetto, L. (2018). Fate and occurrence of micro(nano)plastics in soils: knowledge gaps and possible risks. Curr. Opin. Environ. Sci. Health 1, 6–11. doi: 10.1016/j.coesh.2017.10.006
Jemec Kokalj, A., Horvat, P., Skalar, T., and Kržan, A. (2018). Plastic bag and facial cleanser derived microplastic do not affect feeding behaviour and energy reserves of terrestrial isopods. Sci. Total Environ. 615, 761–766. doi: 10.1016/j.scitotenv.2017.10.020
Keller, J., Antje, B., Hajo, H., Ralph, M., Liliane, R., and Matthias, K. (2018). Toxicity Assay for Citrinin, Zearalenone and Zearalenone-14-Sulfate using the nematode Caenorhabditis elegans as model organism. Toxins 10:284. doi: 10.3390/toxins10070284
Kim, S. W., and An, Y.-J. (2019). Soil microplastics inhibit the movement of springtail species. Environ. Int. 126, 699–706. doi: 10.1016/j.envint.2019.02.067
Kiyama, Y., Miyahara, K., and Ohshima, Y. (2012). Active uptake of artificial particles in the nematode Caenorhabditis elegans. J, Exp. Biol. 215, 1178–1183. doi: 10.1242/jeb.067199
Kozak, M., and Piepho, H.-P. (2018). What’s normal anyway? Residual plots are more telling than significance tests when checking anova assumptions. J. Agron. Crop Sci. 204, 86–98. doi: 10.1111/jac.12220
Künkel, A., Becker, J., Börger, L., Hamprecht, J., Koltzenburg, S., Loos, R., et al. (2016). “Polymers, Biodegradable,” in Ullmann’s Encyclopedia of Industrial Chemistry (American Cancer Society) (Wiley-VCH Verlag GmbH & Co. KGaA: Weinheim), 1–29.
Lei, L., Liu, M., Song, Y., Lu, S., Hu, J., Cao, C., et al. (2018a). Polystyrene (nano)microplastics cause size-dependent neurotoxicity, oxidative damage and other adverse effects in Caenorhabditis elegans. Environ. Sci. Nano 5, 2009–2020. doi: 10.1039/C8EN00412A
Lei, L., Wu, S., Lu, S., Liu, M., Song, Y., Fu, Z., et al. (2018b). Microplastic particles cause intestinal damage and other adverse effects in zebrafish Danio rerio and nematode Caenorhabditis elegans. Sci. Total Environ. 61, 1–8. doi: 10.1016/j.scitotenv.2017.11.103
Leung, M. C. K., Williams, P. L., Benedetto, A., Au, C., Helmcke, K. J., Aschner, M., et al. (2008). Caenorhabditis elegans: an emerging model in biomedical and environmental toxicology. Toxicol. Sci. 106, 5–28. doi: 10.1093/toxsci/kfn121
Maaß, S., Daphi, D., Lehmann, A., and Rillig, M. C. (2017). Transport of microplastics by two collembolan species. Environ. Pollut. 225, 456–459. doi: 10.1016/j.envpol.2017.03.009
Maes, T., Jessop, R., Wellner, N., Haupt, K., and Mayes, A. G. (2017). A rapid-screening approach to detect and quantify microplastics based on fluorescent tagging with nile red. Sci. Rep. 7, 44501. doi: 10.1038/srep44501
Mangiafico, S. S. (2015). An r Companion for the Handbook of Biological Statistics, Version 1.3.2. Available online at: rcompanion.org/documents/RCompanionBioStatistics.pdf (accessed August 06, 2019).
Mosser, T., Matic, I., and Leroy, M. (2011). Bacterium-induced internal egg hatching frequency is predictive of life span in Caenorhabditis elegans populations. Appl. Environ. Microbiol. 77, 8189–8192. doi: 10.1128/AEM.06357-11
Mueller, M.-T., Fueser, H., Trac, L. N., Mayer, P., Traunspurger, W., and Höss, S. (2020). Surface-related toxicity of polystyrene beads to nematodes and the role of food availability. Environ. Sci. Technol. 54, 1790–1798. doi: 10.1021/acs.est.9b06583
Napper, I. E., and Thompson, R. C. (2019). Environmental deterioration of biodegradable, oxo-biodegradable, compostable, and conventional plastic carrier bags in the sea, soil, and open-air over a 3-year period. Environ. Sci. Technol. 53, 4775–4783. doi: 10.1021/acs.est.8b06984
Orgiazzi, A., Bardgett, R. D., and Barrios, E. (2016). Global Soil Biodiversity Atlas: Supporting the eu Biodiversity Strategy and the Global soil Biodiversity Initiative: Preserving Soil Organism Through Sustainable Land Management Practices and Environmental Policies for the Protection and Enhancement of Ecosystem Services. Global Soil Biodiversity Initiative. Luxembourg: Publications Office of the European Union.
Pestov, N. B., Shakhparonov, M. I., and Korneenko, T. V. (2011). Matricide in Caenorhabditis elegans as an example of programmed death of an animal organism: the role of mitochondrial oxidative stress. Russian J. Bioorganic Chem. 37, 634–639. doi: 10.1134/S106816201105013X
Rillig, M. C., Ingraffia, R., and de Souza Machado, A. A. (2017a). Microplastic incorporation into soil in agroecosystems. Front. Plant Sci. 8:1805. doi: 10.3389/fpls.2017.01805
Rillig, M. C., Ziersch, L., and Hempel, S. (2017b). Microplastic transport in soil by earthworms. Sci. Rep. 7:1362. doi: 10.1038/s41598-017-01594-7
Rillig, M. C., Lehmann, A., Ryo, M., and Bergmann, J. (2019). Shaping up: toward considering the shape and form of pollutants. Environ. Sci. Technol. 53, 7925–7926. doi: 10.1021/acs.est.9b03520
Rodriguez-Seijo, A., Lourenço, J., Rocha-Santos, T. A. P., da Costa, J., Duarte, A. C., Vala, H., et al. (2017). Histopathological and molecular effects of microplastics in Eisenia andrei bouché. Environ. Pollut. 220, 495–503. doi: 10.1016/j.envpol.2016.09.092
Sander, M. (2019). Biodegradation of polymeric mulch films in agricultural soils: concepts, knowledge gaps, and future research directions. Environ. Sci. Technol. 53, 2304–2315. doi: 10.1021/acs.est.8b05208
Schindelin, J., Arganda-Carreras, I., Frise, E., Kaynig, V., Longair, M., Pietzsch, T., et al. (2012). Fiji: an open-source platform for biological-image analysis. Nat. Methods 9, 676–682. doi: 10.1038/nmeth.2019
Schneider, C. A., Rasband, W. S., and Eliceiri, K. W. (2012). NIH image to ImageJ: 25 years of image analysis. Nat. Methods 9, 671–675. doi: 10.1038/nmeth.2089
Shim, W. J., Song, Y. K., Hong, S. H., and Jang, M. (2016). Identification and quantification of microplastics using Nile Red staining. Mar. Pollut. Bull. 113, 469–476. doi: 10.1016/j.marpolbul.2016.10.049
van den Hoogen, J., Geisen, S., Routh, D., Ferris, H., Traunspurger, W., Wardle, D. A., et al. (2019). Soil nematode abundance and functional group composition at a global scale. Nature 572, 194–198.
Wang, J., Coffin, S., Sun, C., Schlenk, D., and Gan, J. (2019). Negligible effects of microplastics on animal fitness and hoc bioaccumulation in earthworm Eisenia fetida in soil. Environ. Pollut. 249, 776–784. doi: 10.1016/j.envpol.2019.03.102
Wasserstein, R. L., Schirm, A. L., and Lazar, N. A. (2019). Moving to a world beyond “p < 0.05”. Am. Stat. 73, 1–19.
Watteau, F., Dignac, M.-F., Bouchard, A., Revallier, A., and Houot, S. (2018). Microplastic detection in soil amended with municipal solid waste composts as revealed by transmission electronic microscopy and pyrolysis/gc/ms. Front. Sustain. Food Syst. 2:253. doi: 10.3389/fsufs.2018.00081
Wu, T., Xu, H., Liang, X., and Tang, M. (2019). Caenorhabditis elegans as a complete model organism for biosafety assessments of nanoparticles. Chemosphere 221, 708–726. doi: 10.1016/j.chemosphere.2019.01.021
Yeates, G. W., and Bongers, T. (1999). Nematode diversity in agroecosystems. Agricu. Ecosyst. Environ. 74, 113–135. doi: 10.1016/S0167-8809(99)00033-X
Keywords: plastic residues, Nematoda, ingestion, low-density polyethylene, polylactide, poly(butylene adipate-co-terephthalate)
Citation: Schöpfer L, Menzel R, Schnepf U, Ruess L, Marhan S, Brümmer F, Pagel H and Kandeler E (2020) Microplastics Effects on Reproduction and Body Length of the Soil-Dwelling Nematode Caenorhabditis elegans. Front. Environ. Sci. 8:41. doi: 10.3389/fenvs.2020.00041
Received: 16 January 2020; Accepted: 20 March 2020;
Published: 09 April 2020.
Edited by:
Vera I. Slaveykova, Université de Genève, SwitzerlandReviewed by:
Moritz Bigalke, University of Bern, SwitzerlandDefu He, East China Normal University, China
Copyright © 2020 Schöpfer, Menzel, Schnepf, Ruess, Marhan, Brümmer, Pagel and Kandeler. This is an open-access article distributed under the terms of the Creative Commons Attribution License (CC BY). The use, distribution or reproduction in other forums is permitted, provided the original author(s) and the copyright owner(s) are credited and that the original publication in this journal is cited, in accordance with accepted academic practice. No use, distribution or reproduction is permitted which does not comply with these terms.
*Correspondence: Lion Schöpfer, bC5zY2hvZXBmZXJAdW5pLWhvaGVuaGVpbS5kZQ==