- 1Department of Forest Resources Management, Faculty of Forestry, Nong Lam University, Ho Chi Minh City, Vietnam
- 2ISEA, University of New-Caledonia (UNC), Noumea, France
- 3IMPMC, Institut de Recherche pour le Développement (IRD), UPMC, CNRS, MNHN, Paris, France
Mangroves are highly productive ecosystems, being key components of trophic food chains along tropical coastlines. In Southern Vietnam, planted Rhizophora apiculata develops in the upper intertidal zone, while Avicennia alba naturally colonizes areas at lower elevation along tidal creeks. The main objective of this study was to quantify the seasonal variability of leaf litter decomposition rates considering that in previous studies we observed a strong influence of the monsoon on C and nutrients cycling within the mangrove and in adjacent creeks. We were also interested in changes in the quality of the leaf litter during its decomposition, focusing on nutrients, δ13C, and trace metals. To reach our goals, we used the litterbag technique. During the monsoon, leaf litter decay rates were increased in the Avicennia stand, most probably because of enhanced leaching resulting from a higher level of the river due to heavy rainfall. In addition, the enhanced decay rates measured in the Avicennia stand during the rainy season resulted in a strong 13C depletion, while 13C enrichment was measured in the other conditions. Elemental concentrations changes during decay were not clearly influenced by season, but depended on the element considered. K, Mg, Na, and Ca had t50 values lower than the one of the bulk material. P and S contents also rapidly decreased during the first decomposition stages, but these elements had longer t50 values than the bulk leaves. Conversely, an enrichment in most trace metals was observed during leaf litter decomposition whatever the season. We suggest that these metals were either trapped from the water column and the forest floor by organic molecules composing the litter, or subjected to litter decomposers uptake. Consequently, this study shows that the release or the uptake of elements during mangrove leaf litter decomposition varied with different parameters, which may have ecological implications on the nature of the elements exported toward adjacent ecosystems, and on their primary productivity.
Introduction
Mangrove forests area was estimated at 150,790 km2, representing <1% of tropical forests and 0.4% of global forest surfaces (Spalding, 2010). However, despite this limited extension, they are a valuable sink for atmospheric CO2 and play a key role in energy flow, nutrient, and carbon cycling in the coastal ocean (Alongi, 2002). Unfortunately because of anthropogenic pressure, mangroves are being lost globally at a mean rate close to 1% per year (Alongi, 2002; Duke et al., 2007; Spalding, 2010); and in some countries, this rate can be as high as 8% (Polidoro et al., 2010). Mangroves destruction may strongly influence carbon and nutrient dynamics along tropical coastlines.
Mangrove plants can fix and store elevated amount of carbon in their biomass (Saenger, 2002). Part of their productivity can be exported to adjacent estuarine ecosystems and to the ocean by the tidal action (Bouillon et al., 2008; Maher et al., 2013; Stieglitz et al., 2013), but some mangrove-derived organic compounds can accumulate in their soils during several 100 or 1000 years (Lallier-Vergès et al., 2008; Adame et al., 2015; Jacotot et al., 2018). Processes of litterfall decomposition on mangrove forest floors are influenced by several parameters, including mangrove species, season, and position of the stand in the intertidal zone (Duke et al., 1981; Saenger and Snedaker, 1993; Feller et al., 1999; Mfilinge et al., 2002; Kristensen et al., 2017). The most important components of all litter materials are the leaves; they can represent 40–85% of the litterfall (Mackey and Smail, 1996; Ochieng and Erftemeijer, 2002). They are, therefore, the main source of nutrients for the forest floor or the adjacent ecosystems. The initial chemical composition of the leaves, and specifically their C/N ratios, were describe to have a key influence on decay rates (Mfilinge et al., 2002). Sherman et al. (1998) showed that low N concentrations in the senescent leaves reduced the rate of microbial decomposition and macrofaunal consumption of the leaf litter. Additionally, nutrient release from litter during decay processes depends on climate, elevated temperatures increase decomposition rates (Sánchez-Andrés et al., 2010).
In mangrove ecosystems, sedimentary organic matter is a blend of allochthonous and autochthonous sources, deriving from algae to higher plants. Kristensen et al. (2008) compiled available data, and showed that, on the one hand, 58% of δ13C values measured in mangrove sediments were lower than −25‰, suggesting an important input of mangrove litter, and on the other hand that the significant number of relatively high δ13C values indicate large inputs of imported phytoplankton, seagrasses and microphytobenthos. In terrestrial ecosystems, 13C enrichment, depletion, or negligible shifts in the residual litter were reported during OM decomposition due to physical, chemical, and biological processes (Gioacchini et al., 2006; Gautam et al., 2016). These changes depend on several parameters, including litter quality, environmental conditions, and microbial biomass (Connin et al., 2001; Zhang et al., 2018). Feng (2002) suggested that for a system containing multiple organic components, like cellulose and lignin, which have different quality and different isotopic compositions, the temporal evolution of isotopic ratios reflected the competition between isotopic fractionation and preferential preservation of organic components. Considering that δ13C is commonly used to trace OM sources in tropical coastal ecosystem, we consider relevant to understand changes in δ13C during mangrove litter decomposition.
Organic-rich mangrove soils can act as long-term sinks for most trace metals, because of their precipitation with sulfide minerals during sulfato-reduction processes (Harbison, 1986). However, trace metal speciation in mangrove soils is highly variable, depending on tide, season, and mangrove species (Marchand et al., 2006, 2012). When bioavailable, trace metals can be subject to an uptake by mangrove plants. The latter can store high amount of trace metals in their tissues (Marchand et al., 2012, 2016), which can return to the soil through litterfall during decay processes (Silva et al., 1998; Abohassan, 2013).
The Can Gio mangrove forest is the largest contiguous mangrove forest in Vietnam. It develops between a densely populated megacity (Ho Chi Minh City, the biggest industrial city in Vietnam) and the East Sea (the Vietnamese name is Bien Dong). Can Gio is known as the “green lungs and kidney of Ho Chi Minh City.” Can Gio mangrove forest covered an area of about 40,000 ha in 1964 (Hong and San, 1993), and had a high biodiversity with more than 200 species of fauna and 52 species of flora. Unfortunately, the forest was almost completely destroyed by the spraying of a mixture of herbicides and defoliants during the Vietnam War (1964–1971) (Ross, 1975), with only Avicennia and Nypa able to survive and naturally regenerate. Fortunately, since 1978, a vast reforestation program, mainly using Rhizophora apiculata, has been implemented by the Ho Chi Minh City Forest Department. When Rhizophora stands established, the mangrove area extended through natural regeneration and rapid colonization of mudflats along riverbanks notably by A. alba. In January 2000, Can Gio became the first mangrove forest in Vietnam to be included in the World Network of Biosphere Reserves by the United Nations Educational, Scientific and Cultural Organization (UNESCO). The importance of nutrients recycling via mangrove-derived organic matter mineralization in sustaining coastal food webs in the Can Gio estuary was demonstrated in previous studies using fatty acid biomarkers and dual stable isotopes (δ13C and δ15N) (David et al., 2018a, 2019). Additionally, higher NH4 concentrations (Taillardat et al., 2019), pCO2 and CO2 emissions (David et al., 2018b; Taillardat et al., 2018; Vinh et al., 2019) were measured during the monsoon due to enhanced microbial activity. The monsoon also increased metal loads in the estuary (Thanh-Nho et al., 2018), and it was demonstrated that their transfer to the biota differed between mangrove stands (Thanh-Nho et al., 2019). Consequently in previous studies concerning Can Gio mangrove, we were able to show: (i) the importance of mangrove-derived organic matter in the functioning of this estuary, (ii) the seasonal variability of OM quality and mineralization, (iii) the influence of OM on trace metals cycling; however up to now, the first step of mangrove leaf litter decomposition was not studied. Consequently, the main objectives of the present study were: (i) to determine the influence of the monsoon on leaf litter decomposition rates, and (ii) to assess nutrients and trace metals dynamics, as well the changes in δ13C for the mangrove leaves during litter decay processes. We hypothesize that that the monsoon season would increase decay rates because of enhanced temperature and rainfall. We also hypothesized that changes in elemental and isotopic compositions of the leaves will depend on the element considered.
Materials and Methods
Study Area
Can Gio Estuary is a deltaic confluence of the Sai Gon, Dong Nai, and Vam Co Rivers. It is located between 10° 22′ and 10° 40′ N and 106° 46′ and 107° 00′ E, and between the biggest industrial city in Vietnam (Ho Chi Minh City) and the East Sea (Figure 1). This estuary is subject to semi-diurnal tides, and the tidal amplitude ranges from 2 to 4 m. The climate is monsoonal, with a dry season lasting from December to April and a wet season from May to November (Figure 2). The total annual rainfall is 1,816 mm yr−1, with most of the rain occurring during the rainy season (1,599 mm yr−1) over a total of 154 days per year. The average annual temperature is 27.4°C, with the highest (37.6°C) occurring in October and the lowest (21.7°C) in February (Figure 2). The mean annual humidity is 86%, ranging from 80.8 to 91.4%. During the rainy season, representing ~80% of the annual rainfall, the rivers bring much more silt, accreting the plain with an annual particles flux estimated at around 160 million tons (Milliman and Meade, 1983; Schwarzer et al., 2016). During the dry season, the fresh water flow is strongly reduced, and the coastal areas are severely affected by saline intrusion. The water salinity of the Long Tau River is higher (26.06‰) during the dry season than during the wet season (16.52‰), as observed by Thanh-Nho et al. (2018). Currently, the Can Gio mangrove forest area covers ~32,125 ha of mangrove forests, including 23,508 ha of interior forest and 8,617 ha of mixed forest (Vinh, 2018). The A. alba stands develop between +20 and +30 above MSL, while the Rhizophora stand develops between +40 and +75 cm above MSL (Vinh, 2018). A transitional forest develops between these two stands, mainly composed of R. apiculata, A. alba, and Althaea officinalis, as well as, sparse Excoecaria agallocha and Sonneratia alba.
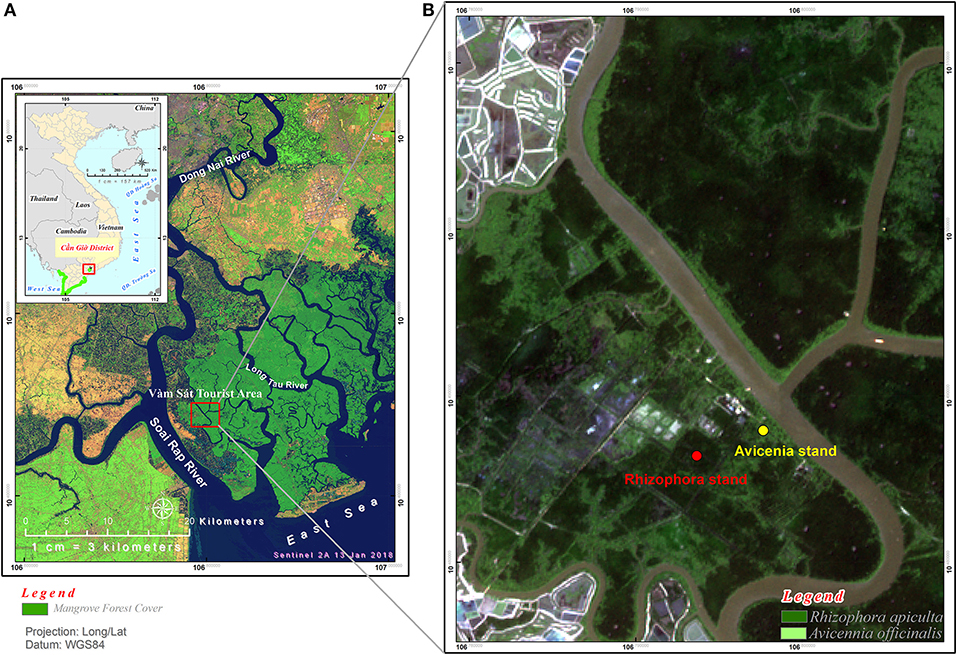
Figure 1. Study sites location: (A) Can Gio mangrove forest (in dark green color) in Southern Vietnam, (B) positions of the Rhizophora stand and the Avicennia stand studied.
Data Collection and Methods
Litterbags Experiment
The decomposition of leaf litter was determined using the litterbag technique. This method may underestimate actual decomposition, because of a slightly different microhabitat and microclimate. In addition, the mesh size of the bags excluded breakdown by large invertebrates, such as crabs, which play a role in leaf degradation. However, this method reflects trends and allows comparison among species and sites (Ashton et al., 1999). These experiments were conducted in the field during the two seasons: from December 2016 to February 2017 during the dry season, and from June 2017 to August 2017 during the rainy season. R. apiculata and A. alba yellow leaves were picked on several trees at each season. Leaves were stored in a frozen box in the field and then taken back to the laboratory, where they were rinsed with purified water to remove any excess salt, and then air dried until reaching a constant mass. For each season, the leaves were split into 90 litterbags (15 * 20 cm and 1.0 mm mesh size). Forty five litterbags containing Rhizophora leaves were installed in the R. apiculata stand, and 45 litterbags containing Avicennia leaves were installed in the A. alba stand. Each bag contained 8 g dry weight (DW) of R. apiculata leaves or 6 gDW of A. alba leaves. In each mangrove stand, three random points were chosen, and at each point, three bags were installed. Bags were deposited on forest floor and tightly attached to Avicennia trunk or Rhizophora prop roots. Consequently, for each collection period, nine bags were removed from the field, representing three samples; one sample was composed of tree bags. Collection occurred 7, 14, 28, 56, and 84 days after the beginning of the experiment and during the two seasons. After collection, the leaf samples were rinsed carefully with purified water, placed in zipper bags, sealed in aluminum foil in order to minimize gas exchange, stored in a frozen box in the field, and then taken back to the laboratory. Samples were dried by Freeze Drying Vacuum at −52°C until reaching a constant mass at the LAMA Lab IRD—Noumea, New Caledonia.
Analytical Methods and Calculations
For each site and each collection date, three leaf litter samples (composed of the detritus from three bags) were analyzed. Each sample was ground to 250 μm with a cutting mill (Fritsch, Pulverisette 15, Germany) and divided into two sub-samples. One subsample was used to determine the total concentrations of Ca, Mg, K, S, Fe, Al, Ni, Cr, Co, Cu, and Zn by inductively coupled plasma optical emission spectrometry (ICP-OES) after extraction in a solution of 1% w/v solution of FNH4 and nitric acid (70%) (Bourgeois et al., 2019). The second sub-sample was used to determine the total C and N concentrations, as well as δ13C values, using a Flash EA1112 elemental analyzer coupled to a Thermo Finnigan DELTA plus XP isotope ratio mass spectrometer using a ConFlo III interface. The analytical precisions of the analyzer were checked using the IAEA-600 caffeine standard (IAEA Nucleus) and were <1% for TOC, 0.15% for N, and 0.3% for δ13C. All the analyses were performed at the LAMA Lab IRD—Noumea, New Caledonia. Carbon isotope ratios are expressed as δ13C values in ‰ (per million) using the following equation:
Rsample and Rstandard refer to the 13C/12C ratio in the sample and the standard, respectively. The C/N ratios were determined for each sample.
The percent of mass, nutrients, and trace metals remaining in the litter were calculated using a single negative exponential equation:
where Wt was the remaining mass at time (t), Wo was the initial leaf mass, and k was the decay constant. The half-time (t50) was calculated using the formula proposed by Olson (1963):
Because of the rapid initial loss due to leaching, a double exponential model may be more suitable for some elements. However, the single exponential model, which was used in this study, is an easily applicable and robust method. Decay constants and half-lives could be compared within this study and with other experiments conducted elsewhere (Ashton et al., 1999). In addition, the rapid initial loss measured may have been enhanced by the two different methods we used to dry the samples; freeze-drying tends to be more effective than air-drying (used for the T0 leave samples) in removing water content even if we wait until obtaining constant mass for both methods.
A parametric two-way analysis of variance (ANOVA) was used to test the effect of seasons and sites on decomposition rates. This method was also applied to assess the difference in δ13C values and C/N ratios among mangrove forests (Table 1). Data analyses were performed using XLSTAT software version 2017.4 for Mac OS.
Results
Leaf Litter Decomposition in the Rhizophora Stand
In the Rhizophora stand, leaf litter was degraded rapidly during the first week, with decay rates of 4.65 ± 0.39% d−1 and 3.62 ± 0.35% d−1 during the dry and the rainy season, respectively; and then decomposition rates decreased with time. After 84 days, the leaf litter lost 77.5 ± 10.9 and 74.8 ± 7.1% of its original DW during the dry and the rainy season, respectively (Figure 3). Mean t50 values were similar between seasons (43.3 days). OC mean t50 values were 36.5 and 40.8 days during the dry and rainy seasons, respectively, with a steady decrease with time irrespective of the season (Figure 4A). The N content in the leaf litter increased during the first week irrespective of the season, reaching 107.5 and 112.9% of the original DW during the dry and the rainy season, respectively (Figure 4). After the first week, N contents decreased slowly, and t50 were higher than 69 days irrespective of the season. The initial C/N ratios of the Rhizophora leaves ranged from 61.2 to 68.2 (Figure 5). During decay, C/N ratios decreased to 28.5 and to 27.5, during the dry and the rainy season, respectively. The δ13C values of the original yellow leaves ranged from −29.43 to −28.60‰. Whatever the season, an enrichment was observed during the first week, followed by a stabilization and a depletion after 2 months. The enrichments during the first week were ~1‰ during the dry season and ~0.5‰ during the rainy season, while the depletions at the end of the experiment ranged between 0.2 and 0.7‰.
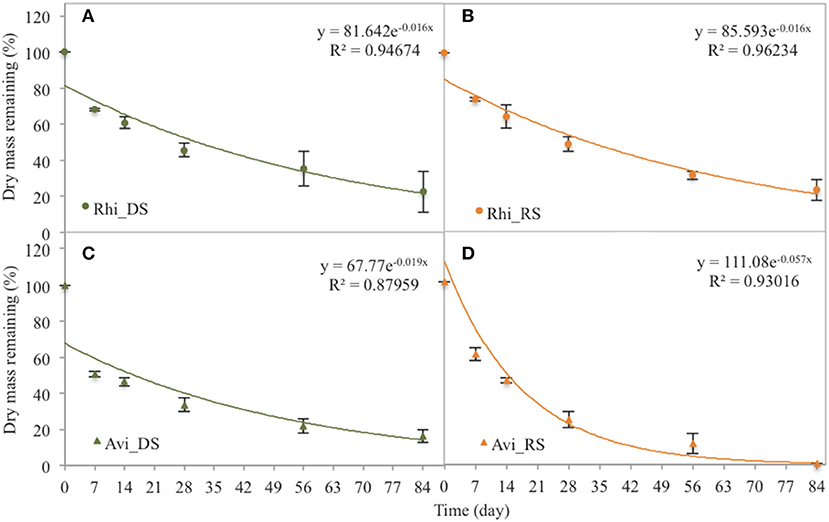
Figure 3. Dry mass remaining of Rhizophora (Rhi) and Avicennia (Avi) leaves during the 84 days of the decomposition experiment. DS is the dry season, and RS is the rainy season. (A) Rhizophora dry season. (B) Rhizophora rainy season. (C) Avicennia dry season. (D) Avicennia rainy season.
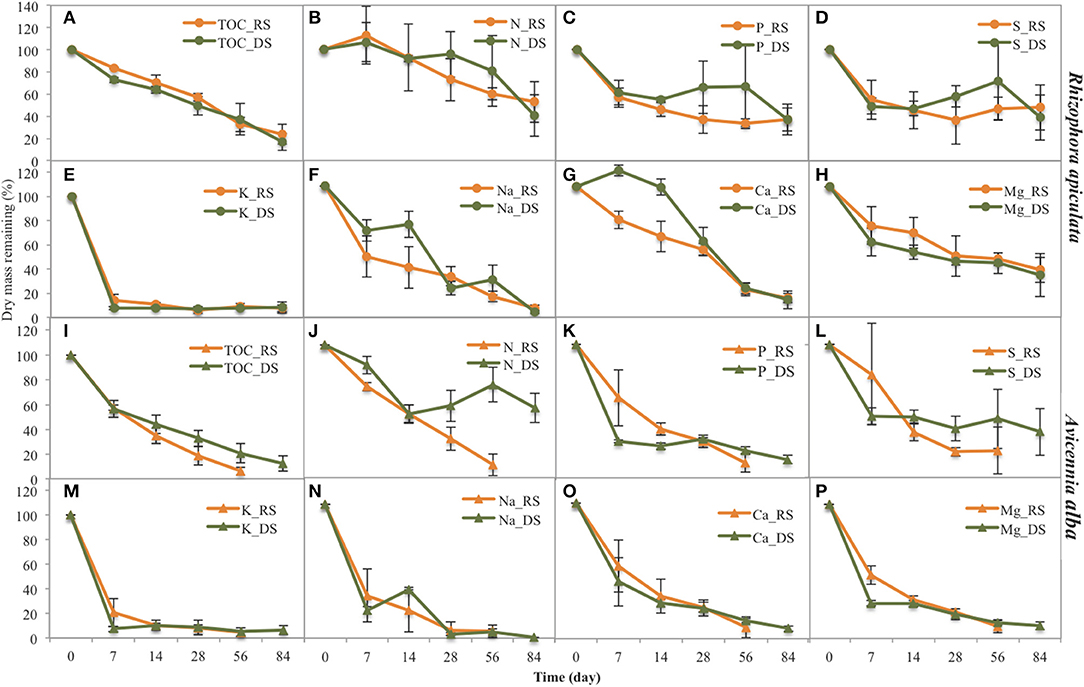
Figure 4. Remaining dry mass of several elements during leaf litter decomposition of the two species studied (Rhizophora and Avicennia) and at the two seasons (RS: rainy season, DS: dry season). (A) TOC for Rhizophora, (B) N for Rhizophora, (C) P for Rhizophora, (D) S for Rhizophora, E K for Rhizophora, (F) Na for Rhizophora, (G) Ca for Rhizophora, (H) Mg for Rhizophora, (I) TOC for Avicennia, (J) N for Avicennia, (K) P for Avicennia a, (L) S for Avicennia a, (M) K for Avicennia a, (N) Na for Avicennia, (O) Ca for Avicennia a, (P) Mg for Avicennia.
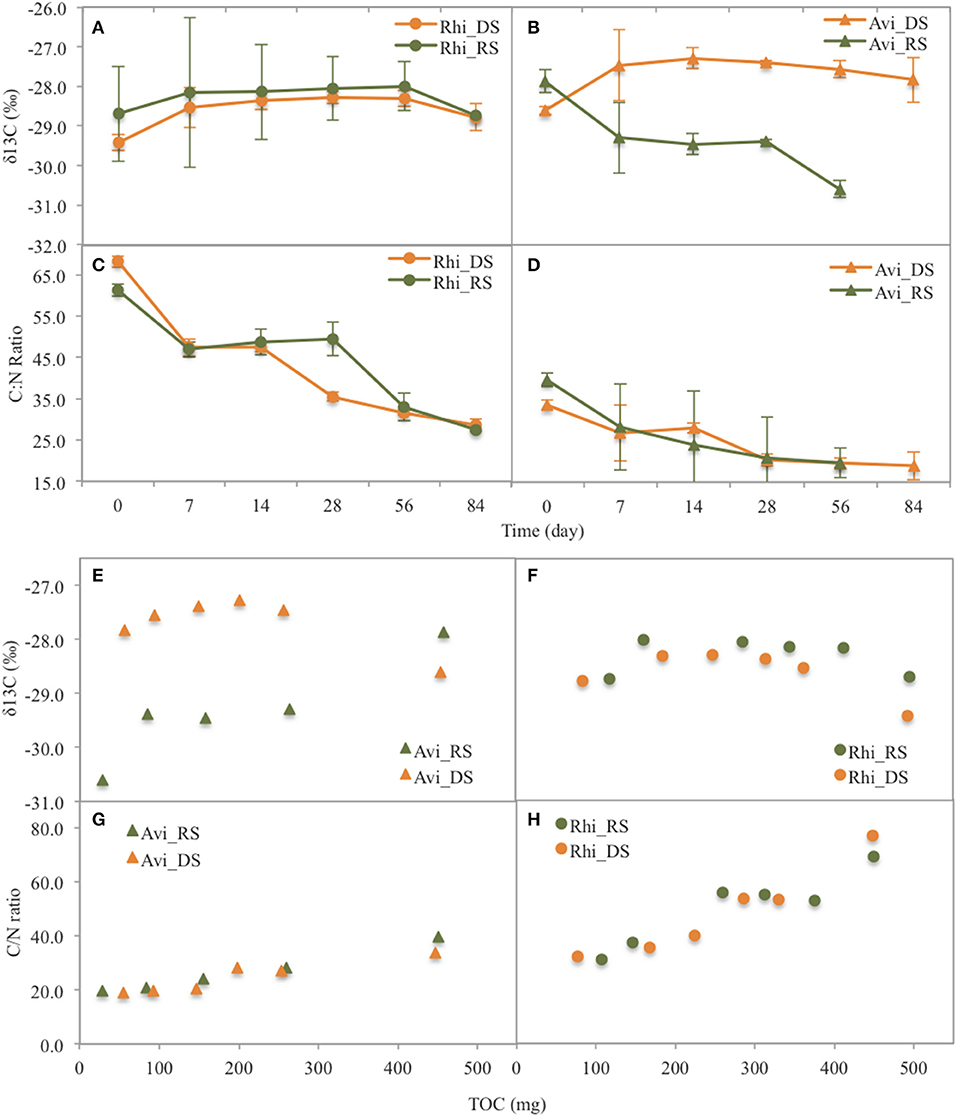
Figure 5. Changes (with time and with TOC) of δ13C values and C/N ratios of the leaf litter of the two species Rhizophora (Rhi) and Avicennia (Avi) during leaf litter decomposition at the two seasons (DS: dry season, RS: rainy season). (A) δ13C with time for Rhizophora, (B) δ13C with time for Avicennia, (C) C/N with time for Rhizophora, (D) C/N with time for Avicennia, (E) δ13C with TOC for Avicennia, (F) δ13C with TOC for Rhizophora, (G) C/N with TOC for Avicennia, (H) C/N with TOC for Rhizophora.
K decomposition rates were the highest of all the elements during the first week, with only 7.6 and 14.1% of original DW remaining during the dry and the rainy season, respectively (Figure 4). However, Na presented the shortest mean t50 values, with 19.8 and 24.8 days during dry and rainy season, respectively; while K t50 values of 36.5 and 46.2 days during the rainy and dry seasons, respectively (Table 2). Mg releases were rapid during the first week as 70.4 and 57.4% of the original DW remained after the first week during the dry and the wet season, respectively. This rapid decrease was followed by a slow immobilization, with quite elevated t50 values, ~69 days irrespective of the season. P releases during the first week were similar between seasons, with ~60% of the original DW remaining. However, after this first week, P evolution differed between seasons: P contents slightly decreased during the rainy season, but during the dry season they increased to day 56 and decreased after (Figure 4C). P mean t50 values were 77.0 and 90.0 days during rainy and dry seasons, respectively. Like N, Ca content increased during the first week during the dry season, reaching 111.5% of the original DW (Figure 4G); and after the first week, Ca dynamics showed a slow decrease. During the rainy season, Ca content steadily decreased during the decomposition process. S showed a very specific trend; after a rapid release during the first week (50% of the original DW remained), S contents increased during both seasons.
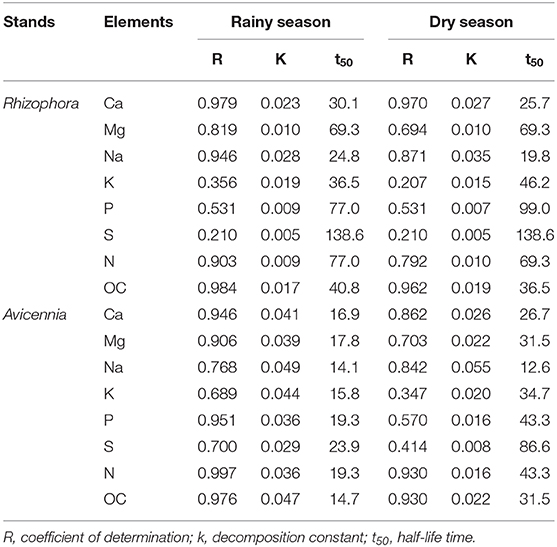
Table 2. Simple negative exponential regression equations (X = ae−kt), % of litter mass remaining in the litterbags (X) with time (t) during decomposition of leaf litter in the studied Rhizophora and Avicennia stands.
In the Rhizophora stand, most trace elements contents increased during the decay process for either season (Figure 6). During the rainy season, Co, Al, and Fe contents sharply increased during the first week, to ~80, 21, and 12 times that in the original DW, respectively. Cr, Ni, Cu, and Zn concentrations also increased during the first week, but not sharply. After the first week, the remaining Zn and Ni contents increased; whereas, Cr and Cu contents decreased before a rapid increase. During the dry season, most metal contents steadily increased during the decay process, with contents after 84 days being ~92, 29, 21, 8, 1, 3, and 3 times higher in the original DW for Co, Al, Fe, Cr, Ni, Cu, and Zn, respectively.
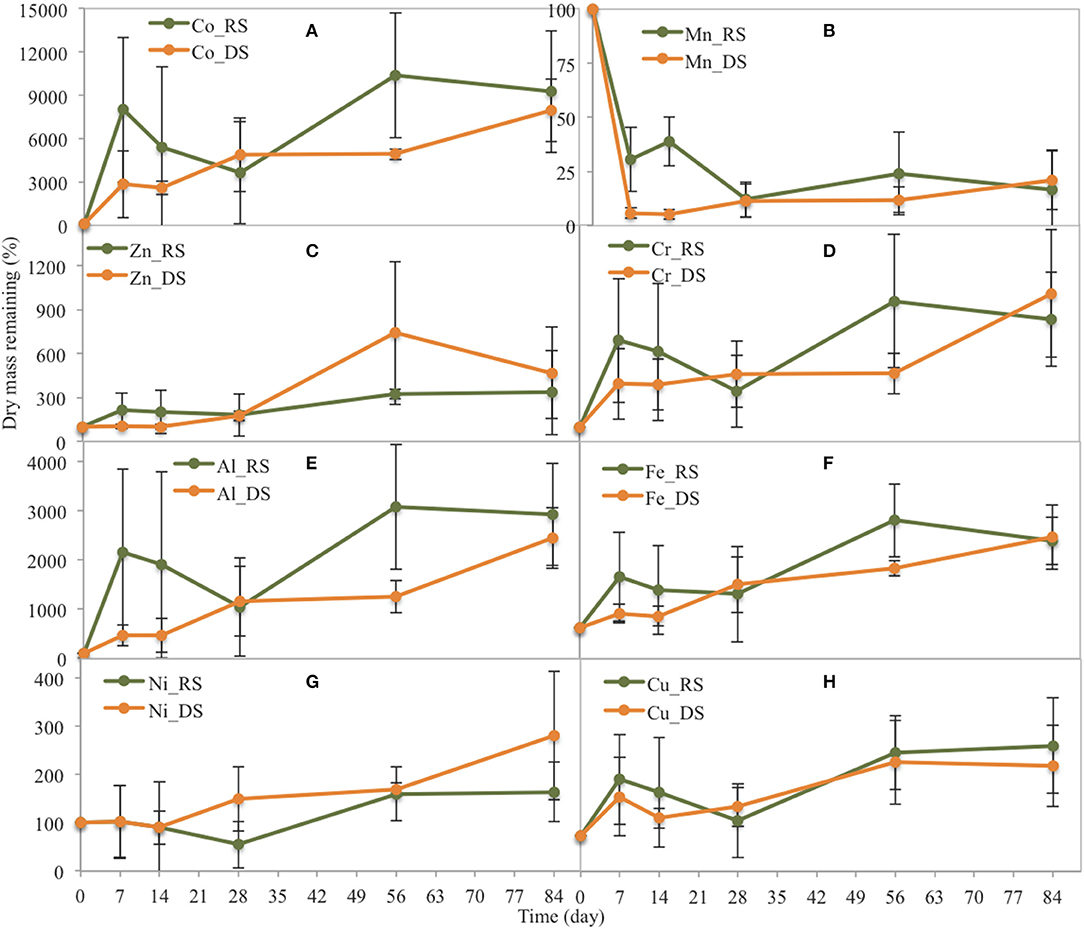
Figure 6. Changes in the dry mass of some trace metals related to their original dry mass in yellow leaves during leaf litter decomposition in the Rhizophora stand at the two seasons (DS: dry season, RS: rainy season). (A) Co, (B) Mn, (C) Zn, (D) Cr, (E) Al, (F) Fe, (G) Ni, (H) Cu.
Leaf Litter Decomposition in the Avicennia Stand
In the Avicennia stand, a higher decomposition rate was measured during the rainy season (with a t50 value of 12.2 days) compared to that measured during the dry season (with a t50 value of 36.5 days). At the end of the experiment, the leaf litter lost 83.6 ± 5.5% during the dry season, and was completely decomposed during the rainy season (Figure 3). Decomposition rates decreased with time all along the experiment. OC mean t50 values were 31.5 and 14.7 days during the dry and rainy seasons, respectively. OC was released rapidly during the first week, decreasing of more than 40% DW irrespective of the season (Figure 4I). N was released throughout the experiment and at a faster rate during the rainy season, with mean t50 of 19.3 and 43.3 days during the rainy and the dry season, respectively. The initial C/N ratios ranged from 33.5 to 39.6 for the Avicennia leaves (Figure 5). C/N ratios decreased to 18.9 and to 19.6, during the dry and the rainy season, respectively. The δ13C values of the original yellow leaves ranged from −28.61 to −27.86‰. Two different trends of δ13C shifts were observed during leaf litter decomposition (Figure 5). During the dry season, an enrichment was observed during the first week, followed by a stabilization and a depletion after 2 months. During the rainy season, we observed a δ13C depletion during the entire experiment, with the final value being 2.7‰ more negative than the original value (Figure 5).
K decomposition rates were the fastest of all the elements during the first week, with only 7.5 and 20.6% remained during the dry and the rainy season, respectively (Figures 4E,M). Na showed a rapid release during the first week, with 20.8 and 31.8% of the original DW remained during the dry and rainy seasons, respectively. Na mean t50 values were 12.6 days and 14.1 during the dry and the rainy season, respectively (Table 2). K t50 values were 15.8 and 34.7 days during the rainy and dry seasons, respectively. Mg releases were rapid during the first week, and this decay was even more rapid during the rainy season (Figures 4H,P), as 47.1 and 26.1% of the original DW remained after the first week during the dry and the rainy season, respectively. Mg mean t50 were 31.5 and 14.7 days during dry and rainy seasons, respectively. P contents also showed a rapid decrease during the first week, but this decrease was higher during the dry season than during the rainy, with 27.7 and 60.0% of the original DW remaining (Figure 4K). P mean t50 were 43.3 and 19.3 days during dry and rainy seasons, respectively. Ca was rapidly released during the first week irrespective of the season, with 42.3 and 53.4% of the original DW remaining during the dry and the rainy season, respectively. Considering the entire length of the experiment, Ca decomposition rates were higher during the rainy season than the dry season, with mean t50 of 19.3 and 43.3 days (Figure 4O), respectively. S contents in the leaf litter also showed a rapid decrease during the first week and a slow immobilization afterward irrespective of the season (Figures 4D,L). S releases were higher during the rainy than during the dry season, with mean t50 of 23.9 and 86.6, respectively.
In the Avicennia stand during the rainy season, Co, Cr, Ni, Fe, Cu, and Al contents sharply increased during the first week, reaching up to 20 times that in the original DW (Figure 7). Their contents rapidly decreased during the second week and then slightly decreased until the end of the experiment. During the dry season, most metal content in the leaf litter of Avicennia (Co, Cr, Ni, Fe, Cu, and Al) steadily increased during the decomposition process. The contents in the leaf litter after 84 days were ~2, 2, 2, 4, 1, and 5 times that in the original DW for Co, Cr, Ni, Fe, Cu, and Al, respectively. Only the Zn content slightly decreased during the first week and then showed a rapid increase at the end of decomposition process during the rainy season, reaching 224% of that in the original DW. However, during the dry season, the Zn content slightly decreased during the first week before increasing to reach ~90% of that in the original DW at the end of the experiment. Conversely, the Mn content decreased during leaf litter decay. After the first week, 5.8 and 30.7% of that in the original DW remained in the Rhizophora stand, during the dry and the rainy season, respectively. In the Avicennia stand, 11.7 and 28.1% of that in the original DW remained after the first week during the dry and the rainy season, respectively. After the first week, the remaining Mn content ranged between ~20 and 40% irrespective of the season.
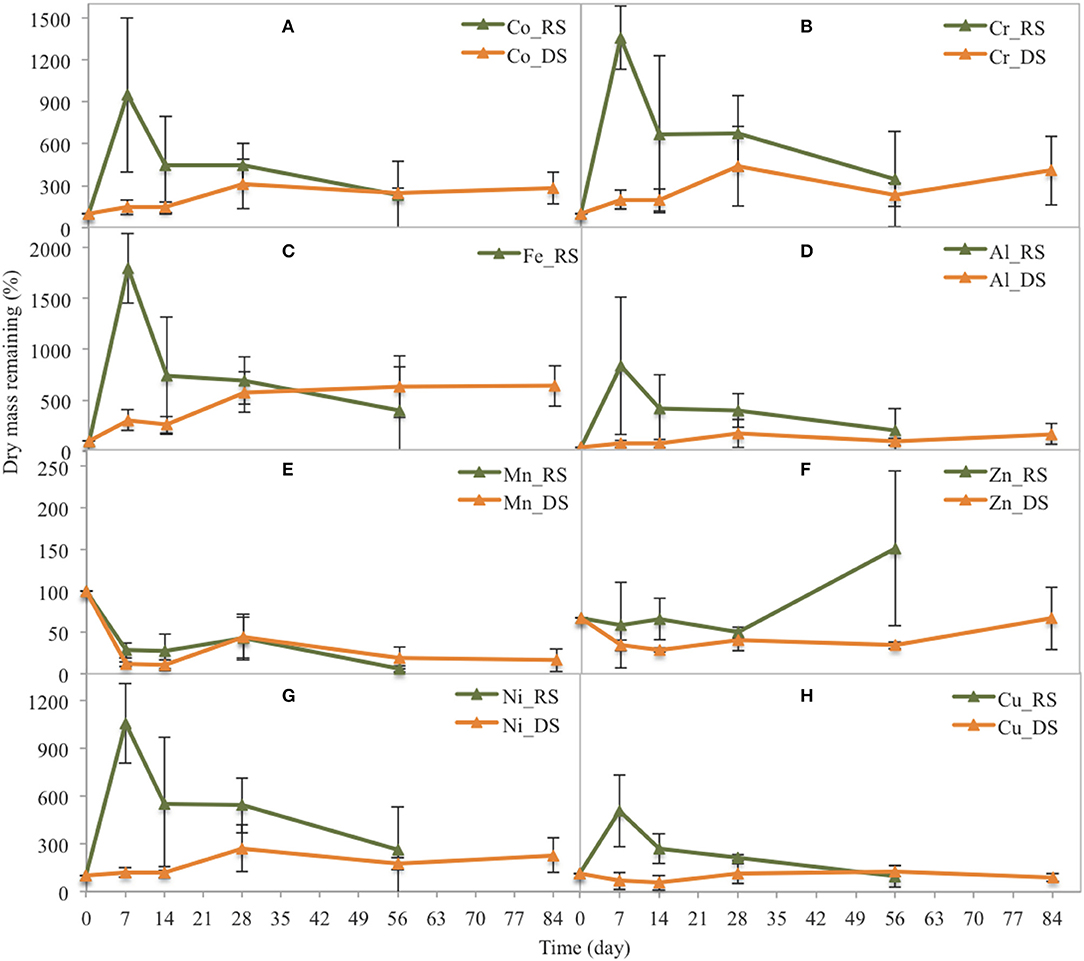
Figure 7. Changes in the dry mass of some trace metals related to their original dry mass in yellow leaves during leaf litter decomposition in the Avicennia stand at the two seasons (DS: dry season, RS: rainy season). (A) Co, (B) Cr, (C) Fe, (D) Al, (E) Mn, (F) Zn, (G) Ni, (H) Cu.
Statistical Analysis
Decomposition rates were significantly different between mangrove species (p < 0.001) and between seasons (p < 0.001). The interaction between season and species also significantly influenced decomposition rates (p < 0.001; Table 1). Decomposition rates were significantly higher in the Avicennia stand than in the Rhizophora stand in both seasons (Table 1 and Figure 3). Eventually, leaf litter decomposition rates of both species significantly varied during the 3 months of the experiments (p < 0.001; Table 1), and were faster during the first week. The organic carbon (OC) decay rates of the leaf litter were significantly different between the Rhizophora and Avicennia stands (p < 0.001). C/N ratios evolution during the decay processes were significantly different between species (p < 0.001) but were not affected by season (p > 0.05), and the interaction between species and season was also not significant (p > 0.05). The δ13C values of the leaf litter during decay processes were significantly different between seasons (p < 0.001) and were not affected by mangrove species (p > 0.05). However, the interaction between season and species significantly influenced the δ13C values (p < 0.001; Table 1).
Discussion
Mangrove Leaf Litter Decay Rates
Decay rates vary with temperature, and thus decrease with latitude (Tam et al., 1998). In the present study, t50 for Rhizophora leaves was ~43 days whatever the season, which was similar to previous studies for the same species at the same latitude in Malaysia or in Thailand (Boonruang, 1978; Ashton et al., 1999). For Avicennia leaves, t50 reported in a Chinese mangrove was similar to the one we measured during the rainy season (<20 days) (Zhou et al., 2010), while in Pakistan, Shafique et al. (2015) reported a t50 close to 50 days, little bit higher than what we measured during the dry season. During the rainy season, the river level was really higher, as observed in other studies (Schwarzer et al., 2016), and thus the Avicennia stand, which develops at a lower elevation that the Rhizophora one, was subject to an increased frequency of tidal inundation that is probably responsible for the fast decay rates measured. Ashton et al. (1999) reported that leaf litter immerged in seawater decomposed five times more quickly than one exposed to air. When leaves are wetted, there is initially a very rapid leaching of the most labile organic matter, increasing decomposition rates (Battle and Golladay, 2001). In addition, the tide's physical impact on the leaves may cause fragmentation and increased weight loss. Consequently, the position of the stand in the tidal zone and the related length of tidal inundation may have an influence on the residence time of mangrove leaf litter, as observed by Ong et al. (1982) and then Twilley et al. (1986). Since the seasonal variation of leaf litter decay rates was almost negligible in the Rhizophora stand, while in the Avicennia stand the decay rate was almost twice as fast during the rainy season as compared to the dry season, we suggest that the intense rainfall occurring during the monsoon in Southern Vietnam strongly influenced decay rates by increasing the level of the river, while the role of temperature was limited.
In addition, the composition of the leaves of the two species differed, with the Avicennia leaves being less refractory to decomposition, with lower C/N values. This result is consistent with previous studies that demonstrated that litter decomposition was faster with higher N concentrations in the leaves because of a higher nutritional value for decomposers (Pelegraí et al., 1997; Wafar et al., 1997). Conversely, Rhizophora leaves are rich in tannins (Kristensen et al., 2008), which are known to be aversive to detritivores and inhibit microbial activity. The rapid initial lost during the first week was already reported in previous studies that showed that mangrove leaf litter could lose half of its original DW during the first week (Twilley et al., 1986; Imgraben and Dittmann, 2008; Sánchez-Andrés et al., 2010). This initial loss represented the leaching of the most labile organic matter by the tidal action. The remaining leaf biomass, being more refractory, was decomposed more slowly and depended on the action of decomposers, bacterial, and fungal communities.
Changes in TOC, C/N, and Major Elements During Mangrove Leaf Litter Decomposition
Through mangrove leaf litter degradation, nutrients are released to adjacent environments sustaining their primary productivity (Mfilinge et al., 2002). In the present study, during the decomposition process, we observed that the nutrient contents in the leaf litter of Avicennia and Rhizophora varied with time, mangrove species, and season. Nutrient releases were significantly faster in the Avicennia stand than in the Rhizophora stand irrespective of the season (Table 2). In addition, in the Avicennia stand, nutrient discharges were also significantly higher during the rainy season due to enhanced leaching as explained earlier, and all elements showed similar decay rates than the bulk material. However, some differences between elements appeared during leaf litter decomposition in the Rhizophora stand during both seasons and in the Avicennia stand during the dry season. During mangrove leaf litter decomposition, C releases were very similar to the DW losses, while N releases were slower. Additionally, during the first week, Rhizophora leaves, with a lower initial N content than Avicennia leaves, gained N from external sources, reaching almost 120% of that in the original DW. This N gain most probably reflected the colonization of leaf litter by diverse microbial communities (Gautam et al., 2016). Nordhaus et al. (2017) also suggested an increasing portion of labile nitrogenous OM and total N in decomposing leaves over time were partly related to the activity of leaf-colonizing bacteria. Twilley et al. (1986) observed that during litter decomposition, the leaves of Rhizophora species sequestered N from the environment, while Avicennia leaves released N to the environment. Consequently, C/N ratios decreased during leaf litter decomposition; and despite lower bulk decays rates in the Rhizophora stand, the C/N ratio decrease was more pronounced in this stand due to the original composition of the leaves. Bonanomi et al. (2010) suggested that litter C/N ratio progressively decreases until a threshold value is attained, which then allows a direct N release into the soil.
If OC decay rates were similar to the bulk material, some elements were characterized by faster rates of decomposition. K release was the fastest of all elements, losing more than 80 % of its original DW value during the first week regardless of the season in both the Avicennia and the Rhizophora stands (Figures 4E,M). Previous studies observed that K was the most mobile cation during leaf litter decay and that its leaching in the early stages of organic matter decomposition was very efficient (Alvarez et al., 2008; Bonanomi et al., 2010; Gautam et al., 2016). Bonanomi et al. (2010) suggested that the rapid K leaching reflected the presence of this element in plant tissues as a water-soluble salt not bound to complex organic molecules. This hypothesis may also be applied to Ca, Mg, and Na, which were characterized by higher decay rates than the bulk leaf litter. All these elements were characterized by a rapid leaching during the first week of the experiment, followed by a phase of immobilization or a slower release of nutrients possibly governed by the changes in refractory components. The P dynamic in the decomposing leaf litter was specific, characterized by both a rapid leaching during the first week, and decay rates during the length of the experiment lower than that of the bulk material. This result suggests that part of the initial P content was associated with non-structural components rapidly leached by the tidal action, but that another part was associated with refractory molecules. Another hypothesis was that P evolution during the later phase might be related to the P transfer from the forest floor to the decomposing leaf litter through fungal hyphae and/or bacterial immobilization (Lindahl et al., 2007). S showed the lowest decay rates. Similar to the other elements, S was characterized by a rapid leaching during the first week, and its mass stabilized very rapidly in the Avicennia stand but increased in the Rhizophora stand. Schroth et al. (2007) indicated that the highest fraction of S in the leaf litter was organic sulfide, which was the most reactive fraction, associated with a highly labile pool of organic matter. They also showed that the sulfonate fractional abundance increased during humification. We suggest that the soil surface in the Rhizophora stand may be more favorable to sulfato-reduction processes (Kristensen et al., 2017), with low Eh values (Vinh et al., 2019); and that consequently, sulfur compounds can be formed and be incorporated in the decomposing organic leaf material. It is generally accepted that inorganic S species react with functionalized molecules under very mild conditions (Damste and De Leeuw, 1990).
Changes in Natural 13C Abundance During Mangrove Leaf Litter Decomposition
OM sources in mangrove ecosystems have historically been identified using isotopic tools, notably δ13C (Bouillon et al., 2008). However, to our knowledge, little attention has been paid to isotopic shift during mangrove leaf litter decomposition processes. In the Avicennia stand during the rainy season, 13C depletion was observed throughout the experiment. We suggest that fast organic matter decay rates, with t50 of the bulk material lower than 15 days, was responsible for this depletion. Gioacchini et al. (2006) observed that the decrease in δ13C during OM decomposition corresponded to the period of maximum mass loss, which would fit well with our observations. They explained this as the preferential microbial breakdown of the more easily degradable C sources, such as carbohydrates and cellulose, which are commonly 13C enriched compared to the more resistant C components, such as lignin (Benner et al., 1987). Consequently, enhanced preferential decomposition processes due to intense leaching are suggested to be responsible for the 13C negative shift. Conversely, during the first week of decomposition in the Rhizophora stand during both seasons and in the Avicennia stand during the dry season, 13C enrichment was measured. This may result from the incorporation of C originated from microbial biomass, characterized by higher δ13C values, into the decomposed litter. This hypothesis may be supported by the N gain of Rhizophora leaf litter during the first week of the experiment (Figures 4B). Recently, Yang et al. (2018) suggested that 13C enrichment during mangrove leaf litter decomposition was related to fungal and bacterial respiration. However, Preston et al. (2009) suggested that microbial biomass constituted only a small fraction of decomposing litter and may not have any major influence on δ13C dynamics, which may limit our hypothesis. In these stands at those seasons, 13C depletion was measured after 2 months of decomposition, which may reflect the preferential preservation of lignin compounds characterized by lower δ13C values, as observed for the Avicennia stand during the rainy season. This process may occur later as lower decomposition rates were measured in these conditions. Consequently, in our experiment, we measured both 13C depletion and enrichment during leaf litter decomposition that were possibly related either to microbial fractionation or to selective preservation of lignin compounds. The main driver of these δ13C shifts in our study seemed to be the decomposition rates.
Trace Metals Enrichment During Leaf Litter Decomposition
An enrichment of trace metals (Fe, Al, Co, Cr, Ni, Zn, and Cu) in the decomposing leaf litter of A. alba and R. apiculata was observed irrespective of the season. Only Mn had the same evolution as the major elements described earlier with rapid leaching during the first week and <10% of the value in the original DW remaining, followed by an immobilization. Silva et al. (1998) observed that Mn was more susceptible to leaching than other metals, which were frequently associated with refractory structural compounds. Manganese is an essential micronutrient, participating in the structure of photosynthetic proteins and enzymes (Mukhopadhyay and Sharma, 1991). Foliar tissue contains predominantly Mn2+, which can be solubilized during leaf litter decomposition, explaining its fast release. However, in a terrestrial forest, it was also observed than Mn can be rapidly immobilized as Mn3+/4+ oxides, resulting in an enrichment of the remaining litter (Keiluweit et al., 2015). The specific conditions of the mangrove forest floor, the tidal immersion, and its richness in organic matter that lead to anoxic conditions (Vinh et al., 2019) may prevent Mn2+ oxidation and its subsequent immobilization.
Regarding the other trace metals, we suggest that the main cause for metal enrichment in the litter was the trapping of dissolved metals that were present in the water column at high tide and at the soil surface, which contains trace metals in different forms depending on the redox conditions. Previous studies observed enrichment of trace metals of the remaining litter during its decomposition, up to several hundred times (Zawislanski et al., 2001; Scheid et al., 2009). Mangrove soils are known to be a sink for trace metals due to their richness in organic matter and possible sulfide precipitation (Noel et al., 2014; Marchand et al., 2016). Dissolved metals can be complexed by organic molecules present in the litter or by the uptake by bacteria decomposing the litter. Thanh-Nho et al. (2018) described the distribution of trace metals in the Can Gio estuary, demonstrating that during the monsoon season enhanced amounts of trace metals were delivered to the estuary, both in particulate and dissolved phases. In addition, we observed that trace metal enrichment during mangrove leaf litter decomposition was higher in the Rhizophora stand than in the Avicennia stand. Taking into account that the Rhizophora stand was less frequently inundated than the Avicennia stand, we would suggest that the forest floor was the main source of trace metals, or that the organic molecules composing the Rhizophora leaves and their decomposers are more efficient in trapping trace metals. In the Can Gio mangrove forest beneath the Rhizophora stand, Thanh-Nho et al. (2018) measured lower trace metal concentrations in the solid phase compared to a higher level in the dissolved phase, which may explain the results obtained in the present study, dissolved metals being more bioavailable. In addition, as described in a previous section, the N content increase in the Rhizophora litter during the first week may translate the rapid development of bacteria, inducing enhanced metal uptake by decomposers. In the Avicennia stand during the rainy season, when decomposition processes were the most efficient, trace metal releases were measured after the first week of the experiment. We suggest that part of the organic molecules that had trapped trace metals during the first week may have been decomposed, leading to metals releases.
Conclusions
The tropical planted mangrove studied (Can Gio, Vietnam) is ~40 years old, and is characterized by a specific zonation of the ecosystem, with planted Rhizophora trees developing at the highest elevation of the intertidal zone and Avicennia trees naturally colonizing the lower intertidal zone along rivers and creeks. The litterbags experiments conducted during this study provide evidence that changes in leaf litter quality during decomposition processes on forest floor depended on the mangrove species, its position in the intertidal zone, and the season. The intense rainfall occurring during the monsoon strongly increased the frequency of tidal inundation of the Avicennia stand, which possibly resulted in an enhanced leaching and explaining the faster decay rates measured. We also suggest that Avicennia leaves characterized by higher N content and lower C/N ratios, were more labile that the Rhizophora leaves. Whatever the season or the species, some elements (K, Ca, Mg, and Na) were subject to a rapid leaching during the first week of the experiment, reflecting their probable presence in plant tissues as a water-soluble salt not bound to complex organic molecules. These elements can thus be rapidly available for different organisms or adjacent ecosystems. Conversely, S mass increased in the Rhizophora leaf litter, most probably because sulfur compounds were formed in anoxic conditions in the forest floor and then incorporated in the decomposing organic leaf material. All trace metals studied, except Mn, also showed enrichment in the leaf litter during their decomposition irrespective of the stand or the season, possibly trapped by organic molecules and/or the decomposers. Eventually, both 13C depletion and enrichment during mangrove leaf litter decay processes were observed. Depletion may be related to the enhanced decay rates in the Avicennia stand during the rainy season, and reflects the fast decomposition of the most labile compounds and the preferential preservation of lignin compounds. The enrichment observed in the other conditions may possibly result from the development of bacteria, but more studies must be performed to confirm this hypothesis. The seasonal and species differences in leaf litter decay processes may have impacts on the ecological functioning of the Can Gio estuary. Due to the position of the Avicennia stand, close to tidal creeks, and the lability of their leaves, their decomposition products may be useful in sustaining heterotrophic activity in adjacent waters and may be the basis of the trophic food chain. Conversely, Rhizophora leaf litter may accumulate in soils, and their decomposition products may be more available for mangrove plants and detritus consumers within the soil.
Data Availability Statement
All datasets generated for this study are included in the article/supplementary material.
Author Contributions
CM, TV, and MA performed the experimental design. TV and KL performed data collection and statistical analyses. CM and TV performed the data interpretation. TV, CM, KL, and MA wrote the paper.
Funding
The project was supported by IRD (Institut de Recherche pour le Développement) through an ARTS Ph.D. Grant to TV, and by the Air Liquide Foundation.
Conflict of Interest
The authors declare that the research was conducted in the absence of any commercial or financial relationships that could be construed as a potential conflict of interest.
Acknowledgments
We would like to thank the authorities of Can Gio District People Committee, the CGMBR, and the Vam Sat Ecological Tourist Zone of Ho Chi Minh city who facilitated the fieldwork. We also thank the LAMA lab in Noumea (New Caledonia) for elemental and isotopic analyses.
References
Abohassan, R. A. (2013). Heavy metal pollution in Avicennia marina mangrove systems on the Red Sea Coast of Saudi Arabia. J. King Abdulaziz Univer. 24:35. doi: 10.4197/Met.24-1.3
Adame, M. F., Santini, N. S., Tovilla, C., Vázquez-Lule, A., Castro, L., and Guevara, M. (2015). Carbon stocks and soil sequestration rates of tropical riverine wetlands. Biogeosciences 12, 3805–3818. doi: 10.5194/bg-12-3805-2015
Alongi, D. M. (2002). Present state and future of the world's mangrove forests. Environ. Conserv. 29, 331–349. doi: 10.1017/S03768929020002317
Alvarez, E. M., Marcos, L. F., Torrado, V. M., and Sanjurjo, J. F. (2008). Dynamics of macronutrients during the first stages of litter decomposition from forest species in a temperate area (Galicia, NW Spain). Nutr. Cycl. Agroecosyst. 80, 243–256. doi: 10.1007/s10705-007-9140-4
Ashton, E., Hogarth, P., and Ormond, R. (1999). Breakdown of mangrove leaf litter in a managed mangrove forest in Peninsular Malaysia. Hydrobiologia. 413, 77–88. doi: 10.1023/A:1003842910811
Battle, J. M., and Golladay, S. W. (2001). Hydroperiod influence on breakdown of leaf litter in cypress-gum wetlands. Am. Midl. Nat. 146, 128–145. doi: 10.1674/0003-0031(2001)1460128:HIOBOL2.0.CO;2
Benner, R., Fogel, M. L., Sprague, E. K., and Hodson, R. E. (1987). Depletion of 13C in lignin and its implications for stable carbon isotope studies. Nature 329, 708–710. doi: 10.1038/329708a0
Bonanomi, G., Incerti, G., Antignani, V., Capodilupo, M., and Mazzoleni, S. (2010). Decomposition and nutrient dynamics in mixed litter of Mediterranean species. Plant Soil. 331, 481–496. doi: 10.1007/s11104-009-0269-6
Boonruang, P. (1978). The degradation rates of mangrove leaves of Rhizophora apiculata (Bl.) and Avicennia marina (Forsk.) Vierh. at Phuket Island, Thailand. Phuket Mar. Biol. Center Res. Bull. 26, 1–7.
Bouillon, S., Borges, A. V. E., Castañeda-Moya Diele, K., Dittmar, T., and Duke, N. C. (2008). Mangrove production and carbon sinks: a revision of global budget estimates. Global Biogeochem. Cycles. 22, 1–12. doi: 10.1029/2007GB003052
Bourgeois, C., Alfaro, A. C., Dencer-Brown, A., Duprey, J. L., Desnues, A., and Marchand, C. (2019). Stocks and soil-plant transfer of macro-nutrients and trace metals in temperate New Zealand estuarine mangroves. Plant Soil. 436, 565–586. doi: 10.1007/s11104-019-03945-x
Connin, S., Feng, X., and Virginia, R. (2001). Isotopic discrimination during long-term decomposition in an arid land ecosystem. Soil Biol. Biochem. 33, 41–51. doi: 10.1016/S0038-0717(00)00113-9
Damste, J. S. S., and De Leeuw, J. W. (1990). Analysis, structure and geochemical significance of organically-bound sulphur in the geosphere: state of the art and future research. Org. Geochem. 16, 1077–1101. doi: 10.1016/0146-6380(90)90145-P
David, F., Marchand, C., Taillardat, P., Thanh Nho, N., and Meziane, T. (2018a). Nutritional composition of suspended particulate matter in a tropical mangrove creek during a tidal cycle (Can Gio, Vietnam). Estuar. Coastal Shelf Sci. 200, 126–130. doi: 10.1016/j.ecss.2017.10.017
David, F., Marchand, C., Thanh-Nho, N., Taillardat, P., and Meziane, T. (2019). Trophic relationships and basal resources utilization in the Can Gio Mangrove Biosphere Reserve (Southern Vietnam). J. Sea Res. 145, 35–43. doi: 10.1016/j.seares.2018.12.006
David, F., Meziane, T., Nhu-Trang, T. T., Truong, V. V., Thanh-Nho, N., Taillardat, P., et al. (2018b). Carbon biogeochemistry and CO2 emissions in a human impacted and mangrove dominated tropical estuary (Can Gio, Vietnam). Biogeochemistry 138, 261–275. doi: 10.1007/s10533-018-0444-z
Duke, N. C., Bunt, J., and Williams, W. (1981). Mangrove litter fall in north-eastern Australia. I. Annual totals by component in selected species. Austr. J. Botany 29, 547–553. doi: 10.1071/BT9810547
Duke, N. C. J., Meynecke, O., Dittmann, S., Ellison, A. M., and Anger, K. (2007). A world without mangroves? Science 317, 41–42. doi: 10.1126/science.317.5834.41b
Feller, I. C., Whigham, D. F., O'Neill, J. P., and McKee, K. L. (1999). Effects of nutrient enrichment on within-stand cycling in a mangrove forest. Ecology 80, 2193–2205. doi: 10.1890/0012-9658(1999)0802193:EONEOW2.0.CO;2
Feng, X. (2002). A theoretical analysis of carbon isotope evolution of decomposing plant litters and soil organic matter. Glob. Biogeochem. Cycles 16:66-1-66-11. doi: 10.1029/2002GB001867
Gautam, M. K., Lee, K. S., Song, Y. B, Lee, D., and Bong, Y.-S. (2016). Early-stage changes in natural 13C and 15N abundance and nutrient dynamics during different litter decomposition. J. Plant Res. 129, 463–476. doi: 10.1007/s10265-016-0798-z
Gioacchini, P., Masia, A., Canaccini, F., Boldreghini, P., and Tonon, G. (2006). Isotopic discrimination during litter decomposition and δ13C and δ15N soil profiles in a young artificial stand and in an old floodplain forest. Isotopes Environ. Health Stud. 42, 135–149. doi: 10.1080/10256010600671357
Harbison, P. (1986). Mangrove muds: a sink and a source for trace metals. Mar. Pollut. Bull. 17, 246–250. doi: 10.1016/0025-326X(86)90057-3
Imgraben, S., and Dittmann, S. (2008). Leaf litter dynamics and litter consumption in two temperate South Australian mangrove forests. J. Sea Res. 59, 83–93. doi: 10.1016/j.seares.2007.06.004
Jacotot, A., Marchand, C., Rosenheim, B. E., Domack, E., and Allenbach, M. (2018). Mangrove soil carbon stocks along an elevation gradient: influence of the late Holocene marine regression (New Caledonia). Mar. Geol. 404:60670. doi: 10.1016/j.margeo.2018.07.005
Keiluweit, M., Nico, P., Harmon, M. E., Mao, J., Pett-Ridge, J., and Kleber, M. (2015). Long-term litter decomposition controlled by manganese redox cycling. Proc. Natl. Acad. Sci. U.S.A. 112, E5253–E5260. doi: 10.1073/pnas.1508945112
Kristensen, E., Bouillon, S., Dittmar, T., and Marchand, C. (2008). Organic carbon dynamics in mangrove ecosystems: a review. Aquat. Bot. 89, 201–219. doi: 10.1016/j.aquabot.2007.12.005
Kristensen, E., Connolly, R. M., Otero, X. L., Marchand, C., Ferreira, T. O., and Rivera-Monroy, V. H. (2017). Biogeochemical cycles: global approaches and perspectives. In: Rivera-Monroy V, Lee S, Kristensen E, Twilley R, editors. Mangrove Ecosystems: A Global Biogeographic Perspective. Cham: Springer (2017). doi: 10.1007/978-3-319-62206-4_6
Lallier-Vergès, E., Marchand, C., Disnar, J. R., and Lottier, N. (2008). Origin and diagenesis of lignin and carbohydrates in mangrove sediments of Guadeloupe (French West Indies): evidence for a two-step evolution of organic deposits. Chem. Geol. 255, 388–398. doi: 10.1016/j.chemgeo.2008.07.009
Lindahl, B. D., Ihrmark, K., Boberg, J., Trumbore, S. E., Högberg, P., Stenlid, J., et al. (2007). Spatial separation of litter decomposition and mycorrhizal nitrogen uptake in a boreal forest. N. Phytol. 173, 611–620. doi: 10.1111/j.1469-8137.2006.01936.x
Mackey, A., and Smail, G. (1996). The decomposition of mangrove litter in a subtropical mangrove forest. Hydrobiologia 332, 93–98. doi: 10.1007/BF00016688
Maher, D. T., Santos, I. R., Golsby-Smith, L., Gleeson, J., and Eyre, B. D. (2013). Groundwater-derived dissolved inorganic and organic carbon exports from a mangrove tidal creek: the missing mangrove carbon sink? Limnol. Oceanogr. 58, 475–488. doi: 10.4319/lo.2013.58.2.0475
Marchand, C., Fernandez, J. M., and Moreton, B. (2016). Trace metal geochemistry in mangrove sediments and their transfer to mangrove plants (New Caledonia). Sci. Total Environ. 562, 216–227. doi: 10.1016/j.scitotenv.2016.03.206
Marchand, C., Fernandez, J. M., Moreton, B., Landi, L., Lallier-Vergès, E., and Baltzer, F. (2012). The partitioning of transitional metals (Fe, Mn, Ni, Cr) in mangrove sediments downstream of a ferralitized ultramafic watershed (New Caledonia). Chem. Geol. 300, 70–80. doi: 10.1016/j.chemgeo.2012.01.018
Marchand, C., Lallier-Vergès, E., Baltzer, F., Albéric, P., Cossa, D., and Baillif, P. (2006). Heavy metals distribution in mangrove sediments along the mobile coastline of French Guiana. Mar. Chem. 98, 1–17. doi: 10.1016/j.marchem.2005.06.001
Mfilinge, P., Atta, N., and Tsuchiya, M. (2002). Nutrient dynamics and leaf litter decomposition in a subtropical mangrove forest at Oura Bay, Okinawa, Japan. Trees 16, 172–180. doi: 10.1007/s00468-001-0156-0
Milliman, J. D., and Meade, R. H. (1983). World-wide delivery of river sediment to the oceans. J. Geol. 91, 1–21. doi: 10.1086/628741
Mukhopadhyay, M. J., and Sharma, A. (1991). Manganese in cell metabolism of higher plants. Botanical Rev. 57, 117–149. doi: 10.1007/BF02858767
Noel, V., Marchand, C., Juillot, F., Ona-Nguema, G., Viollier, E., Marakovic, G., et al. (2014). EXAFS analysis of iron cycling in mangrove sediments downstream a lateritized ultramafic watershed (Vavouto Bay, New Caledonia). Geochim. Cosmochim. Acta 136, 211–228. doi: 10.1016/j.gca.2014.03.019
Nordhaus, I., Salewski, T., and Jennerjahn, T. C. (2017). Interspecific variations in mangrove leaf litter decomposition are related to labile nitrogenous compounds. Estuar. Coast. Shelf Sci. 192, 137–148. doi: 10.1016/j.ecss.2017.04.029
Ochieng, C. A., and Erftemeijer, P. L. (2002). Phenology, litterfall and nutrient resorption in Avicennia marina (Forssk.) Vierh in Gazi Bay, Kenya. Trees 16, 167–171. doi: 10.1007/s00468-001-0146-2
Olson, J. S. (1963). Energy storage and the balance of producers and decomposers in ecological systems. Ecology 44, 322–331. doi: 10.2307/1932179
Ong, J.-E., Malaysia, U. S., Gong, W. K., and, Wong, C. H. (1982). Studies on Nutrient Levels in Standing Biomass, Litter and Slash in a Mangrove Forest. School of Biological Sciences, Universiti Sains, Malaysia.
Pelegraí, S. P., Rivera-Monroy, V. H., and Twilley, R. R. (1997). A comparison of nitrogen fixation (acetylene reduction) among three species of mangrove litter, sediments, and pneumatophores in south Florida, USA. Hydrobiologia 356, 73–79. doi: 10.1023/A:1003124316042
Polidoro, B. A., Carpenter, K. E., Collins, L., Duke, N. C., Ellison, A. M., Ellison, J. C., et al. (2010). The loss of species: mangrove extinction risk and geographic areas of global concern. PLoS ONE 5:e10095. doi: 10.1371/journal.pone.0010095
Preston, C. M., Nault, J. R., and Trofymow, J. (2009). Chemical changes during 6 years of decomposition of 11 litters in some Canadian forest sites. Part 2. 13C abundance, solid-state 13C NMR spectroscopy and the meaning of “lignin”. Ecosystems 12, 1078–1102. doi: 10.1007/s10021-009-9267-z
Ross, P. (1975). “The mangroves of south vietnam: the impact of military use of herbicides,” in Proceedings of the International Symposium on Biology and Management of Mangroves. Gainesville, FL: Institute of Food and Agricultural Sciences, University of Florida.
Saenger, P. (2002). Mangrove Ecology, Silviculture and Conservation. Dordrecht: Kluwer Academic Publishers (2002). doi: 10.1007/978-94-015-9962-7
Saenger, P., and Snedaker, S. C. (1993). Pantropical trends in mangrove above-ground biomass and annual litterfall. Oecologia 96, 293–299. doi: 10.1007/BF00317496
Sánchez-Andrés, R., Sánchez-Carrillo, S., Alatorre, L., Cirujano, S., and Álvarez-Cobelas, M. (2010). Litterfall dynamics and nutrient decomposition of arid mangroves in the Gulf of California: their role sustaining ecosystem heterotrophy. Estuar. Coast. Shelf Sci. 89, 191–199. doi: 10.1016/j.ecss.2010.07.005
Scheid, S. M. S., Günthardt-Goerg Schulin, R., and Nowack, B. (2009). Accumulation and solubility of metals during leaf litter decomposition in non-polluted and polluted soil. Eur. J. Soil Sci. 60, 613–621. doi: 10.1111/j.1365-2389.2009.01153.x
Schroth, A. W., Bostick, B. C., Graham, M., Kaste, J. M., Mitchell, M. J., and Friedland, A. J. (2007). Sulfur species behavior in soil organic matter during decomposition. J. Geophys. Res. 112, 1–10. doi: 10.1029/2007JG000538
Schwarzer, K., Nguyen Cong, T., and Ricklefs, K. (2016). “Sediment re-deposition in the mangrove environment of Can Gio, Saigon River estuary (Vietnam),” in Proceedings of the 14th International Coastal Symposium, eds A. Vila-Concejo, E. Bruce, D. M. Kennedy, and R. J. McCarroll (Sydney, Journal of Coastal Research, Special Issue, No. 75), 138–142.
Shafique, S., Siddiqui, P. J., Aziz, R., Shaukat, S., and Farooqui, Z. (2015). Decomposition of Avicennia marina (Forsk.) Vierh. Foliage under field and laboratory conditions in the backwaters of Karachi, Pakistan. Banglad. J. Botany 44, 1–7. doi: 10.3329/bjb.v44i1.22716
Sherman, R. E., Fahey, T. J., and Howarth, R. W. (1998). Soil-plant interactions in a neotropical mangrove forest: iron, phosphorus and sulfur dynamics. Oecologia 115, 553–563. doi: 10.1007/s004420050553
Silva, C., Lacerda, L., Ovalle, A., and Rezende, C. (1998). The dynamics of heavy metals through litterfall and decomposition in a red mangrove forest. Mangrov. Salt Marshes 2, 149–157. doi: 10.1023/A:1009923223882
Stieglitz, T. C., Clark, J. F., and Hancock, G. J. (2013). The mangrove pump: the tidal flushing of animal burrows in a tropical mangrove forest determined from radionuclide budgets. Geochim. Cosmochim. Acta 102, 12–22. doi: 10.1016/j.gca.2012.10.033
Taillardat, P., Ziegler, A. D., Friess, D., Widory, D., David, F., Ohte, N., et al. (2019). Nutrient dynamics in mangrove porewater and adjacent tidal creek using nitrate dual-stable isotopes: a new approach to challenge the Outwelling Hypothesis? Mar. Chem. 214:103662. doi: 10.1016/j.marchem.2019.103662
Taillardat, P., Ziegler, A. D., Friess, D., Widory, D., Van, V. T., David, F., et al. (2018). Surface and porewater export from a mangrove forest during contrasting tidal cycles and seasons: implications for tidal creek carbon dynamics. Geochim. Cosmochim. Acta 237, 32–48. doi: 10.1016/j.gca.2018.06.012
Tam, N., Wong, Y., Lan, C., and Wang, L. (1998). Litter production and decomposition in a subtropical mangrove swamp receiving wastewater. J. Exp. Mar. Biol. Ecol. 226, 1–18. doi: 10.1016/S0022-0981(97)00233-5
Thanh-Nho, N., Marchand, C., Strady, E., Huu-Phat, N., and Nhu-Trang, T. T. (2019). Bioaccumulation of some trace elements in tropical mangrove plants and snails (Can Gio, Vietnam). Environ. Pollut. 248, 635–645. doi: 10.1016/j.envpol.2019.02.041
Thanh-Nho, N., Strady, E., Nhu-Trang, T. T., David, F., and Marchand, C. (2018). Trace metals partitioning between particulate and dissolved phases along a tropical mangrove estuary (Can Gio, Vietnam). Chemosphere 196, 311–322. doi: 10.1016/j.chemosphere.2017.12.189
Twilley, R. W., Lugo, A. E., and Patterson-Zucca, C. (1986). Litter production and turnover in basin mangrove forests in southwest Florida. Ecology 67, 670–683. doi: 10.2307/1937691
Vinh, T. V. (2018). Carbon Stocks and Fluxes in a Tropical Mangrove Forest (Can Gio, Vietnam). Ph.D. thesis of the University of New Caledonia. p. 163.
Vinh, T. V., Allenbach, M., Aimée, J., and Marchand, C. (2019). Seasonal variability of CO2 fluxes at different interfaces and vertical CO2 concentrations profiles in a Rhizophora mangrove stand (Can Gio, Viet Nam). Atmos. Environ. 201, 301–309. doi: 10.1016/j.atmosenv.2018.12.049
Wafar, S., Untawale, A., and Wafar, M. (1997). Litter fall and energy flux in a mangrove ecosystem. Estuar. Coast. Shelf Sci. 44, 111–124. doi: 10.1006/ecss.1996.0152
Yang, Z., Songa, W., Zhaoa, Y., Zhoua, J., Wang, Z., Luoa, Y., et al. (2018). Differential responses of litter decomposition to regional excessive nitrogen input and global warming between two mangrove species. Estuar. Coast. Shelf Sci. 214, 141–148. doi: 10.1016/j.ecss.2018.09.018
Zawislanski, P., Chau, S., Mountford, H., Wong, H., and Sears, T. (2001). Accumulation of selenium and trace metals on plant litter in a tidal marsh. Estuar. Coast. Shelf Sci. 52, 589–603. doi: 10.1006/ecss.2001.0772
Zhang, G., Yu, X., Xu, J., Duan, H., Rafay, L., Zhang, Q., et al. (2018). Effects of environmental variation on stable isotope abundances during typical seasonal floodplain dry season litter decomposition. Sci. Total Environ. 630, 1205–1215. doi: 10.1016/j.scitotenv.2018.02.298
Keywords: mangrove, leaf litter, decomposition, monsoon, Vietnam
Citation: Vinh TV, Allenbach M, Linh KTV and Marchand C (2020) Changes in Leaf Litter Quality During Its Decomposition in a Tropical Planted Mangrove Forest (Can Gio, Vietnam). Front. Environ. Sci. 8:10. doi: 10.3389/fenvs.2020.00010
Received: 28 April 2019; Accepted: 16 January 2020;
Published: 13 February 2020.
Edited by:
Luiz Drude Lacerda, Federal University of Ceara, BrazilReviewed by:
Kam W. Tang, Swansea University, United KingdomNguyen Tai Tue, Hanoi University of Science, Vietnam
Copyright © 2020 Vinh, Allenbach, Linh and Marchand. This is an open-access article distributed under the terms of the Creative Commons Attribution License (CC BY). The use, distribution or reproduction in other forums is permitted, provided the original author(s) and the copyright owner(s) are credited and that the original publication in this journal is cited, in accordance with accepted academic practice. No use, distribution or reproduction is permitted which does not comply with these terms.
*Correspondence: Cyril Marchand, Y3lyaWwubWFyY2hhbmRAdW5jLm5j