- 1Faculty of Agricultural and Environmental Sciences, University of Rostock, Rostock, Germany
- 2Ecohydrology Research Group, Department of Earth and Environmental Sciences, University of Waterloo, Waterloo, ON, Canada
- 3Leibniz Institute for Baltic Sea Research (LG), Warnemünde, Germany
- 4Ecohydrology Research Group, Water Institute and Department of Earth and Environmental Sciences, University of Waterloo, Waterloo, ON, Canada
Sea-level rise coupled with land subsidence from wetland drainage exposes increasingly large areas of coastal peatlands to seawater intrusion. Seawater contains high concentrations of sulfate (SO42−), which can alter the decomposition of organic matter thereby releasing organic and inorganic solutes from peat. In this study, a flow-through reactor system was used in order to examine the transport of SO42− through peat as well as its effect on solute release. Moderately-decomposed fen peat samples received input solutions with SO42− concentrations of 0, 100, 700, and 2,700 mg L−1; sample effluent was analyzed for a variety of geochemical parameters including dissolved organic carbon (DOC), dissolved inorganic carbon (DIC) and total dissolved nitrogen (TDN) as well as the concentrations of major cations and anions. The input solution remained anoxic throughout the experiment; however, no signs of a pronounced SO42− reduction were detected in the effluent. SO42− transport in the fen peat resembled non-reactive bromide (Br−) transport, indicating that in the absence of SO42− reduction the anion may be considered a conservative tracer. However, slightly elevated concentrations of DOC and TDN, associated with raised SO42− levels, suggest the minor desorption of organic acids through anion exchange. An increased solute release due to stimulated decomposition processes, including SO42− reduction, was observed for samples with acetate as an additional marine carbon source included in their input solution. The solute release of peats with different degrees of decomposition differed greatly under SO42−-enriched conditions where strongly-decomposed fen peat samples released the highest concentrations of DOC, DIC and TDN.
Introduction
Over the millennia, large quantities of partly-decomposed organic matter have accumulated in waterlogged peatlands. The hydrological and biogeochemical conditions in a peatland determine whether it may serve as a sink or a source for carbon- and nitrogen-containing compounds. It is well known that the drainage of peatlands for agricultural purposes initiates the aerobic decomposition of organic matter in the top layer, resulting in the enhanced emission of greenhouse gases, including carbon dioxide (CO2) and nitrous oxide (N2O) (Kasimir-Klemedtsson et al., 1997) as well as a mobilization of dissolved organic matter (generally measured as dissolved organic carbon, DOC) and other nutrients such as ammonium () (Zak and Gelbrecht, 2007). Hence, the drainage of peatlands has ramifications in the exacerbation of global warming, land subsidence and nutrient loads for adjacent aquatic ecosystems.
Under anoxic conditions, the decomposition of organic matter is controlled by the presence of electron acceptors such as sulfate (SO42−), an inorganic and highly mobile form of sulfur, which is used in the metabolism of sulfate-reducing bacteria (SRB). While SO42− concentrations in terrestrial ecosystems are generally low, marine ecosystems are SO42−-enriched as seawater contains approximately 2700 mg L−1 (~29 mM, Algeo et al., 2015). Infiltration of seawater into peatlands can therefore drastically increase SO42− concentrations, altering the biogeochemistry of the peat thereby implicating the mineralization of organic matter. Due to climate change and predicted sea level rise, sea water intrusion and the impact on the quality of groundwater resources will be relevant for larger areas of peatlands in the future (Sherif and Singh, 1999; Ardón et al., 2016).
However, when formerly drained peatlands are rewetted (i.e., the water level in the peatland is raised), increased SO42− concentrations may aid climate protection by reducing emissions of the greenhouse gas methane (CH4). The rewetting often results in water levels higher than in the initial state, as the land has subsided, which may result in an emission of a large quantity of CH4 (Wen et al., 2018). When SO42− is present, the SRB, although tending to be low-abundant even under favorable redox conditions, generally outcompete the methanogens in the peat and therefore impede the formation of CH4 (Hausmann et al., 2016). Coastal peatlands are therefore considered well suited for rewetting projects associated with dyke removal (Koebsch et al., 2019), although under certain circumstances (e.g., abundance of suitable electron donors, Weston et al., 2011) methanogenesis and SO42− reduction can occur at the same time (Oremland et al., 1982; Hahn et al., 2015).
While the impact of water salinity and SO42− on greenhouse gas emissions in peatlands has aroused scientific interest, there are hardly any studies focusing on the mechanistic understanding of SO42− mobility in peat and its impact on the release of solutes from peat. Solute release from peat is known to be affected by a wide range of environmental variables, with water table fluctuations and the associated change of redox conditions being the most prominent one (Borch et al., 2010). In particular, factors prompting the DOC release from peatlands have been studied previously, since DOC concentrations in surface water in Europe have increased in the last decades (Roulet and Moore, 2006), which is for example problematic for drinking water treatment (Ritson et al., 2014). Factors influencing DOC release from peat include ionic strength [and its proxy, electrical conductivity (EC)] and pH of the pore water. When EC increases and pH decreases, amounts of DOC released typically decline as a result of decreased charge density, limiting the solubility of the DOC (Münch et al., 2002; Clark et al., 2011; Ardón et al., 2016; Tiemeyer et al., 2017). Increases in EC have also been observed to impact other geochemical processes in peat, including the desorption of by salt ions (Ardón et al., 2013), an increase of hydraulic conductivity due to a pore dilation (Ours et al., 1997) and a decrease in plant uptake of nutrients as a result of salt stress (Hanin et al., 2016). The explicit impact of changing SO42− concentrations on solute release has been studied mostly for moderately increased concentrations simulating atmospheric deposits (Blodau et al., 2007; up to 10 mg L−1), discharge of river water to a wetland (Lamers et al., 1998; up to 400 mg L−1) or intrusion of highly diluted seawater into a wetland (Ardón et al., 2016; up to 300 mg L−1). In an extensive mesocosm experiment, Lamers et al. (1998) observed an increase in alkalinity due to the consumption of hydrogen ions during SO42− reduction, a reduced uptake of nutrients, such as potassium (K+), due to the toxicity of sulfide (HS−) and an increased release of due to the increase of organic matter decomposition. They also observed that increased SO42− concentrations can turn peat into a source for phosphate, similar as observed for other aquatic sediments (Caraco et al., 1989). The underlying process is the formation of iron sulfide, which reduces the availability of iron (Fe) as binding partner for P both in the reduced form of vivianite or as oxidized Fe(III)-P binding forms (Zak et al., 2010). However, the sulfur cycle in peatlands is complex as sulfur can occur in various organic and inorganic forms and can be recycled multiple times (i.e., reduced and reoxidized) as stated for bogs (Wieder and Lang, 1988; Blodau et al., 2007), forested wetlands (Mandernack et al., 2000) and salt marshes (Gardner, 1990). To the author's knowledge, there has been no comprehensive study on SO42− dynamics and mobility within fen peat with respect to release of solutes from peat induced by SO42−-related processes. The main objective of this study was to gain an improved understanding of the processes determining the mobility of SO42− of varying concentrations in fen peat samples and their short-term impact on the solute release from the peat.
Materials and Methods
Field Sampling and Peat Properties
Fifteen undisturbed core samples of moderately-decomposed reed-sedge fen peat (referred to as “MD-Peat”) were collected in May 2018 horizontally at a depth of 50 cm below surface from a drained fen in northwestern Germany (“Pölchow”, 54°00′20.3′′N, 12°06′58.8′′E). The acrylic glass sampling tubes (Ø = 4.2 cm, L = 10 cm, V = 139 cm3) were incorporated into a sharpened shuttle corer which was pushed manually into the peat. The groundwater level was 75 cm below surface so that the taken peat samples were wet but not completely water-saturated. The peatland has not been affected by Baltic seawater intrusion [SO42− concentrations in the groundwater accumulated in the profile pit were low (3.7 mg L−1)]. For comparison reasons additional samples (n = 3) of two fen peats with a different degree of decomposition were collected: firstly a degraded highly-decomposed peat (referred to as “HD-Peat”) taken horizontally from the dried top soil in Pölchow in a depth of 20 cm, secondly a SO42−-affected and water-saturated slightly-decomposed peat (referred to as “SD-Peat”) taken vertically in a depth of 10 cm in the shallow water of the Baltic Sea adjacent to the coastal fen “Hütelmoor” (54°13′19.38′′N, 12°10′7.02′′E). The coastline on the latter site has transformed over time to constantly expose parts of the peat layer to seawater (Kreuzburg et al., 2018). Additional disturbed samples of each peat were collected to determine the organic matter content as well as the solid-phase total carbon, nitrogen and sulfur (C/N/S) contents. After collection, all peat samples were refrigerated and transported to the Ecohydrology Research Group at the University of Waterloo (Canada) for the laboratory experiments. The basic physicochemical properties of the peat material used in this study are shown in Table 1. The C/N/S contents were measured for homogenized and freeze-dried samples on a CHNS Carbo Erba analyzer (method detection limit: 0.1% dwt) without any pre-treatment of the freeze-dried samples. The Fe content was determined by Inductively-Coupled Plasma Optical Emission Spectrometry (ICP-OES) after an aqua regia digestion according to HFA (2014). The bulk density (ρb) was determined gravimetrically based on the sample volume and the oven-dried (3 days at 80°C) sample mass. Subsequently, the peat samples were ignited at 550°C to determine the loss on ignition (LOI) as a proxy for the organic matter content (DIN EN 15935, 2012), which was used to calculate the particle density (ρs) of the peat samples with standard values for organic (1.4 g cm−3) and mineral (2.65 g cm−3) components [ρs = (1.4 × LOI + 2.65 × (100–LOI))/100]. The total porosity was then calculated from bulk and particle densities (Φ = 1 − ρb / ρs).
Flow-Through Reactor Experiment
A one-month SO42− displacement experiment was carried out using a flow-through reactor (FTR) set-up (Figure 1). These reactors are designed to measure biogeochemical reaction rates on undisturbed soils or sediments and yield kinetic parameters and information on reaction pathways that can be extrapolated to natural conditions (Pallud et al., 2007). They have been used to study solute transport in peat (Kleimeier et al., 2017) as well as SO42− and nitrate (NO3−) reduction in sediments (Pallud and Van Cappellen, 2006; Stam et al., 2010; Laverman et al., 2012). In addition to information on SO42− transport, the 1-month monitoring of this experiment also provided insight into the short-term effects of increased SO42− concentrations on the release of solutes. The FTRs (21 reactors in total) consisted of the peat core contained within an acrylic glass tube attached to two PVC plate end-caps with an opening for tubing connection. Between each plate end-cap and the soil column an O-ring, a glass fiber filter (pore size 1 μm) and a hydrophilic polypropylene membrane filter (pore size 0.2 μm) were inserted to avoid leakage, uniformize inflow and reduce the filtration effort for the effluent sampling. The bottom end-cap of the FTRs was connected to a peristaltic pump (Gilson MINIPULSE® 3) and served as an inflow channel while the top end-cap connected to the outflow channel allowed the effluent to collect in polyethylene sampling bottles (125 mL). Inflow and outflow channel consisted of viton tubing (ID = 1.59 mm). During the entire experiment duration of 1 month, the pump rate (PR) was set to 4 mL h−1 (corresponding to a Darcy flux of 7 cm d−1) with slight differences between the pump channels (range of 3.4 to 4.5 mL h−1).
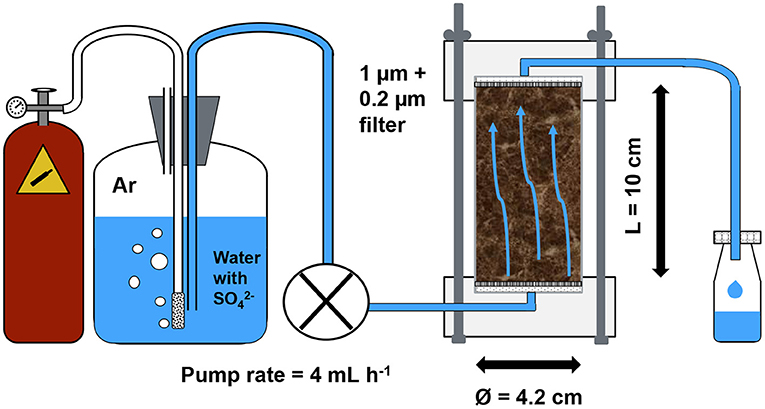
Figure 1. Schematic diagram of the experimental set-up. The reactors were placed in an environmental chamber at constant temperature of 15°C. The sulfate-enriched water was depleted in oxygen via sparging with argon (Ar), flowed with a pump rate of 4 mL h−1 through the peat samples and was subsequently collected and sampled.
Triplicates of MD-peat samples were assigned to different SO42− treatments corresponding to SO42− milieus in terrestrial (0 and 100 mg L−1), brackish (700 mg L−1) and marine (2,700 mg L−1) ecosystems (see Table 2). The latter treatment was also applied to the SD-peat and HD-peat samples. Furthermore, another treatment of the MD-peat containing 2,700 mg SO42− L−1 and 590 mg acetate (CH3COO−) L−1 (concentration inspired by Schmaljohann, 1996) simulating a potential input of marine dissolved organic matter (DOM) was added to guarantee an excess of electron donors, as the peat consists of rather persistent plant residues. Acetate is generally considered as the most relevant electron donor for anaerobic decomposition of organic matter by SRB in the marine environment (Boschker et al., 2001). In the following, the different treatments are referred to with the labels shown in Table 2 (e.g., SULF0), the addition of acetate to a treatment is indicated within the label with a “+A.”
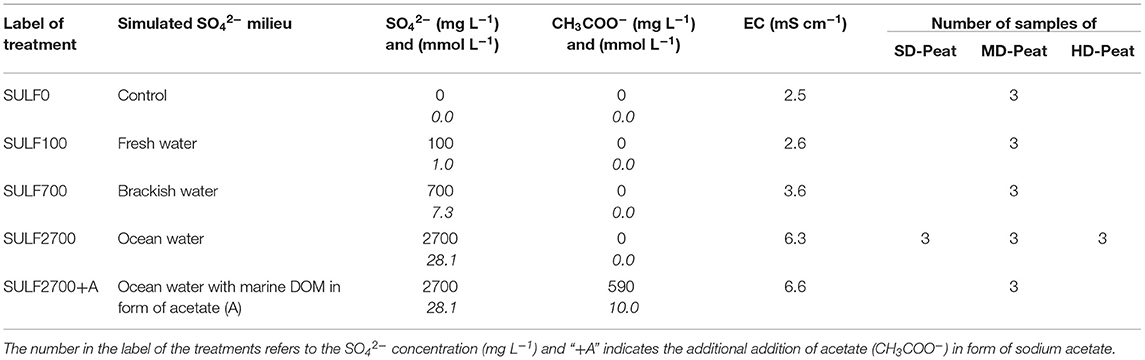
Table 2. Overview of the different applied SO42− concentrations and associated number of peat samples.
The artificial brackish input solution was prepared by diluting the quantitatively most important seawater salts according to Kester et al. (1967) (NaCl, KCl, MgCl2 · 6H2O, CaCl2 · 2H2O, NaHCO3) in 20-fold dilution in ultra-pure water (Milli-Q) and then adding Na2SO4 to adjust the different SO42− treatments and NaCH3COO for the treatment SULF2700+A. The EC of the five different input solutions was between 2.5 and 6.6 mS cm−1 (measured with a Horiba LAQUA B-213 Twin EC meter), representing seawater diluted by freshwater in the mixing zone (observed in the Hütelmoor field site, but see also e.g., Jørgensen et al., 2008). To induce the development of anoxic conditions the input solutions to the FTRs were continuously sparged with argon gas reducing oxygen concentrations to levels between 0.2 and 0.9 mg L−1 (measured with a Thermo Scientific Orion 5 Star multifunction meter). The continuous sparging with argon also led to an increase in the pH of the input solution (up to 8.8, measured with a Horiba LAQUA B-213 Twin pH meter) due to degassing of carbon dioxide, which was adjusted to values between 7 and 7.5 through addition of minor amount of hydrochlorid acid. However, as the pH of seawater (~8.1) is generally higher than freshwater, variations of pH may also occur under natural field conditions. As the SO42− reduction rate is sensitive to the temperature (Stam et al., 2010), the FTR experiment was conducted in an environmental chamber (Percival Scientific CTH-118) at a constant temperature of 15°C representing common summer temperatures in peatland groundwater at the Hütelmoor field site (M. Ibenthal, University of Rostock, personal communication). The placement of the experimental set-up in the chamber also ensured dark conditions to prevent algae growth in the acrylic glass tubes.
Prior to the SO42− treatments, a control solution (SULF0) containing 0 mg SO42− L−1 was pumped (4 mL h−1) into the FTRs for 3 days to purge the cores of gas bubbles that may block water flow as well as to flush out the pre-existing SO42− and equilibrate the peat cores with the salt solution. Only for the marine SD-peat a solution with 700 mg SO42− L−1 was used to maintain the natural SO42− milieu in those three samples. The outflow of the first and the last 50 mL of the flushing phase was analyzed to determine the initial and stabilized chemical concentrations. During the SO42− treatment phase (1 month), the outflow samples were collected every 24 h for the first 3 days and afterwards every 48 to 72 h. During the first 5 days of the SO42− treatment, an additional bromide (Br−) concentration of 100 mg Br− L−1 was added into the input solutions in form of 149 mg KBr L−1 as a step input to obtain a non-reactive tracer breakthrough curve (BTC). During the Br− injection outflow water samples were collected every 2 h on the first day, every 4 h on the second day, every 8 h on the third day, every 12 h on the fourth day and every 24 h on the fifth day and the samples were analyzed for Br− concentration with ion chromatography (see below).
Pore Water Geochemistry Analyses
During the SO42− treatments, water samples were collected from the outflow of the FTRs and were sub-sampled into separate vials. One milliliter of pore water was refiltered (to protect the measurement device) through a 0.2 μm membrane filter (Thermo Scientific Polysulfone filter) for analysis of major anions including Cl−, NO3− and SO42− using ion chromatography (IC, Dionex ICS-5000 with a capillary IonPac® AS18 column). A volume of 7 ml of pore water sample was acidified with 3 drops of 1M HCl and was analyzed for DOC and total dissolved nitrogen (TDN) using the non-purgeable organic carbon method on a total organic carbon analyzer (Shimadzu TOC-LCPH/CPN). Another volume of 7 mL pore water sample was subsampled for concentrations of dissolved inorganic carbon (DIC) that was measured using the same TOC analyzer. For the SULF2700+A treatment, an additional 1 mL sample was treated with 20 μL of a 500 ppm CrO42− solution and analyzed for organic acids using IC to assess acetate concentrations (method detection limit: 0.017 mg L−1) and subtract them from the measured DOC concentrations to get the “acetate-free” DOC release, accepting inaccuracies due to a potential acetogenesis in the peat. For one FTR per treatment, 10 mL of the water samples were filtered through a 0.45 μm membrane filter (Thermo Scientific Polysulfone filter) and were acidified with 2% ultrapure HNO3 for analysis of major cations and trace metals including Fe and manganese (Mn) using Inductively-Coupled Plasma Optical Emission Spectrometry (Thermo iCAP 6200 Duo ICP-OES). Once or twice a week a volume of 1.5 mL was collected into vials containing 50 μL of a 5% zinc acetate solution and the concentration of HS− was measured colorimetrically according to Cline (1969) and using an UV-Visible spectrophotometer (Thermo Scientific Evolution 260 Bio) measuring the absorbance at 670 nm.
Modeling of Breakthrough Curves
For the evaluation of the BTCs the software “STANMOD” (available for download from www.pc-progress.com) with the incorporated model CTXFIT (Toride et al., 1999) was used to determine solute transport parameters by fitting a modeled BTC to the measured data with a least-square fitting procedure. As previous studies have shown that peat acts as a dual porosity medium (e.g., Rezanezhad et al., 2012, 2016), a physical non-equilibrium model was used. The mobile-immobile model (MIM) solves the convection-dispersion equation for a mobile pore region, which exchanges solutes via diffusion with an immobile pore region. The dispersion coefficient D (cm2 h−1) and the two dimensionless parameters β (equivalent to the mobile water content for non-sorbing solutes) and ω (mass transfer coefficient) served as calibration parameters. The retardation factor R was set to 1 (meaning no adsorption occurs), as a pronounced anion adsorption to organic compounds is generally only expected under acidic conditions (Ottow, 2011) and high organic matter contents adversely affect SO42− adsorption (Johnson and Todd, 1983). The average pore water velocity v (cm h−1) was also set to a fixed calculated value [v = PR/(sample cross section × Φ)].
Results and Discussion
Sulfate Transport
The SO42− BTCs for the different treatments showed slightly different shapes and reached a similar relative concentration (C/C0) after approximately three pore volumes, as shown in Figure 2A. However, the differences in their shape are not due to differences in the SO42− input concentration, they are instead a result of differences in the soil pore distribution for the individual peat samples. This effect is verified by non-reactive Br− BTCs (which were always performed for the same Br− concentration) which show a very similar shape to the SO42− BTCs, as exemplified in Figure 2B for a breakthrough of 100 mg Br− L−1 and 100 mg SO42− L−1. These results suggest that no SO42− reduction occurred and SO42− behaved as a conservative anion (such as Br−) during solute transport in the studied fen peat samples. The Br− and SO42− BTCs for all tested peats (Figure 2C) showed an early breakthrough characterized by a C/C0 > 0.5 at one pore volume (Rezanezhad et al., 2016). This is indicative of non-equilibrium flow, meaning that the MIM serves as an appropriate simulation model in this scenario. The obtained solute transport parameters for the different peats contrasted for Br− and SO42− (Table 3) are similar and in the same range as in comparable studies on fen peat (Kleimeier et al., 2017; Liu et al., 2017; McCarter et al., 2018). Generally, the parameters D, β and ω are considered soil sample-specific and should not depend on the applied solute, which also was supported in this study with Wilcoxon signed-rank tests (α = 0.05), that did not reveal any significant differences between Br− and SO42− parameters. Yet, the variations between the parameters of the different peats were also minor suggesting that the effect of degree of decomposition and organic matter content on the shape of the BTC is less pronounced than what has been shown in previous studies (Liu et al., 2017).
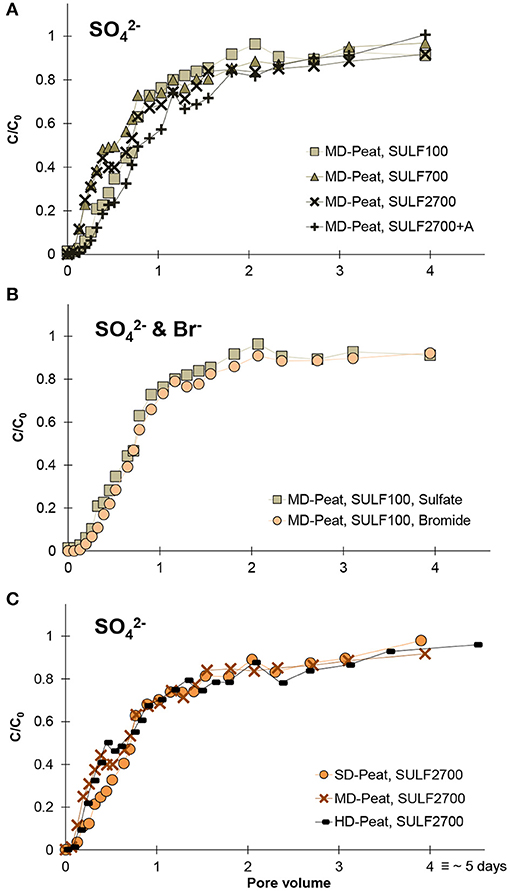
Figure 2. Average (n = 3) breakthrough curves for (A) all applied SO42− concentrations for the moderately-decomposed peat, (B) SO42− and Br− for the treatment SULF100 for the moderately-decomposed peat and (C) SO42− for the treatment SULF2700 for slightly, moderately, and highly-decomposed peat.
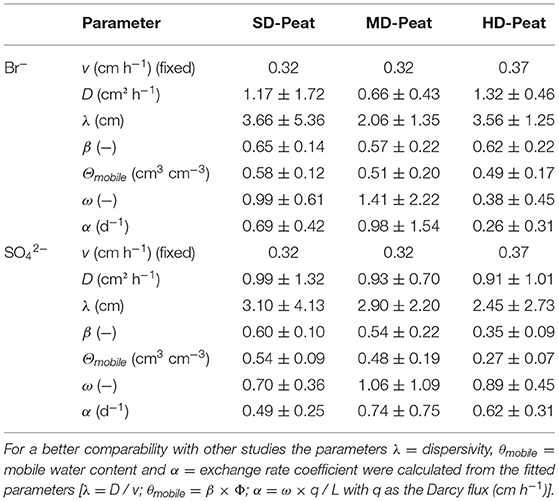
Table 3. Average solute transport parameters (± standard deviation) (v = average pore water velocity, D = dispersion coefficient, β = mobile water content, ω = exchange coefficient between mobile and immobile regions) determined with CTXFIT for the slightly, moderately and highly-decomposed peat for SO42− and Br− breakthrough.
SO42− and sodium (Na+) concentrations in the outflow of the peat samples reached the input concentration after 5 days for SO42− and 10 days for Na+ and remained stable throughout the experiment. Only during the final phase of the experiment a slight difference in SO42− concentration was observed between the input and output concentrations of the SULF2700+A treatment implying that SO42− reduction occurred only in the latter case. This is consistent with the results of the measurement of HS−, which was only detected in higher concentrations (up to 2.4 mg L−1, other treatments of MD-peat: 0.03 to 0.13 mg L−1) for the SULF2700+A treatment in the later phase of the experiment—although a non-detection of HS− can also be related to reactions of HS− with other compounds such as ferrous iron (Fe2+) (see effect of acetate). The SD- and HD-peat samples produced HS− concentrations between 0.06 and 0.2 mg L−1 with only a slight increase in effluent concentration occurring over time, indicating a very weak background SO42− reduction. Results from all treatments imply that more SO42− reduction could have been detected if the experiment was run for a longer duration. For the Fe-rich peat samples, MD-peat and HD-peat, the delayed stimulation of SO42− reduction can be attributed to an initial period of Fe(III) reduction. The predominance of Fe(III) reduction over SO42− reduction has been observed by Küsel et al. (2008), who found that in an upper peat horizon in a lowland fen with a similar Fe content (33 g kg−1), the Fe(III) reduction made up 72% of the anaerobic organic carbon mineralization. Conversely, for the SO42−-affected SD-peat with lower Fe content, it can be postulated that the microbially available carbon pool was depleted due to previous pronounced SO42− reduction occurring under field conditions.
Solute Release
Initial Flushing
Containing 40% organic carbon and 3% nitrogen (Table 1), the MD-peat represents a potential source for nutrients. During the equilibration period with SO42−-free water (3 days = 2.3 PV), the substances which had accumulated in the pore water in the peat were flushed and the concentrations of DOC, DIC and TDN decreased, on average, from 9 to 4 mg DOC L−1, from 16 to 3 mg DIC L−1 and from 0.7 to 0.4 mg TDN L−1. The initial values measured for DOC are lower than in previous studies using flow-through experiments with peat [32 mg DOC L−1 in Gosch et al. (2018), 11 mg DOC L−1 in Tiemeyer et al. (2017)], which can be explained by a larger sample volume of 50 mL in this study causing a dilution of the peak concentration as well as a sample filtration using 0.2 μm filters rather than the commonly used 0.45 μm filters. However, in the cases of this experiment as well as the aforementioned previous studies, the DOC concentrations stabilized in a range of 30 to 40% of the initial concentration. Field concentrations of DOC and TDN from the sampling site were measured to be 16 mg DOC L−1 and 3.6 mg TDN L−1. The apparent discrepancy between the field values and laboratory column experiments has already been observed in other studies (Stutter et al., 2007; Tiemeyer et al., 2017), and is generally explained by longer residence times and limited dilution in the field. The initial values for the HD-peat were higher than for the MD-peat with a decrease from 13 to 6 mg DOC L−1, 17 to 6 DIC mg L−1 and 6.6 to 0.5 mg TDN L−1. Formerly drained and degraded peat is known to release more solutes than less decomposed peat, due to the formation of more mobile compounds through aerobic mineralization (Zak et al., 2010). Therefore for rewetting purposes the degraded top soil of formerly drained peatlands is sometimes removed to reduce the leaching of nutrients and emission of greenhouse gases (Zak et al., 2018). In this study, the initial release of 22 mg NO3− L−1 from the HD-peat was 440 times higher than that of 0.05 mg NO3− L−1 release occurring from the underlying MD-peat. For the marine SD-peat, which was initially flushed with a 700 mg L−1 SO42− solution, the concentrations changed from 28 to 25 mg DOC L−1, 12 to 1 mg DIC L−1 and 3 to 1 mg TDN L−1, and no NO3− was detected in the effluent. The relatively lower decrease in DOC concentrations observed for the MD-peat sample group might be related to the effects of changes in EC wherein the antecedent EC from the marine field conditions was closer to the artificial seawater than in the terrestrial peat.
Effect of Sulfate Concentration
During the SO42− application in the FTRs, the solute release evolved differently for the various SO42− treatments of the MD-peat (Figures 3A–C). For most of the samples DOC and TDN concentrations decreased continuously. However, the higher the SO42− concentration in the input solution the lower the average slope of the linear trend for DOC and the higher the average DOC concentration at which the values seemed to stabilize (for SULF0 and SULF100 ~ 2 mg L−1, for SULF700 ~3 mg L−1 and for SULF2700 ~5 mg L−1). These results imply a positive correlation between EC and DOC and do not confirm observations by previous studies on DOC fluctuations, who observed a negative correlation between EC and DOC release (Münch et al., 2002; Clark et al., 2011; Tiemeyer et al., 2017). For example, in Tiemeyer et al. (2017) an increase in EC from ~100 to 1,000 μS cm−1 caused a decrease of DOC from ~10 to 1 mg DOC L−1. However, the results in this study are consistent with a previous study by Gosch et al. (2018), who observed a positive relation between EC and DOC for peat material from the same field site from which samples were collected for this study. The non-validity of the negative correlation between EC and DOC relation for the MD-peat might be attributed to the peat (pore water) chemistry (see effect of peat decomposition degree). The existence of interfering impact factors is also indicated by contradicting effects comparing laboratory and field data (Tiemeyer et al., 2017) or surface water and pore water geochemistry (Knorr, 2013).
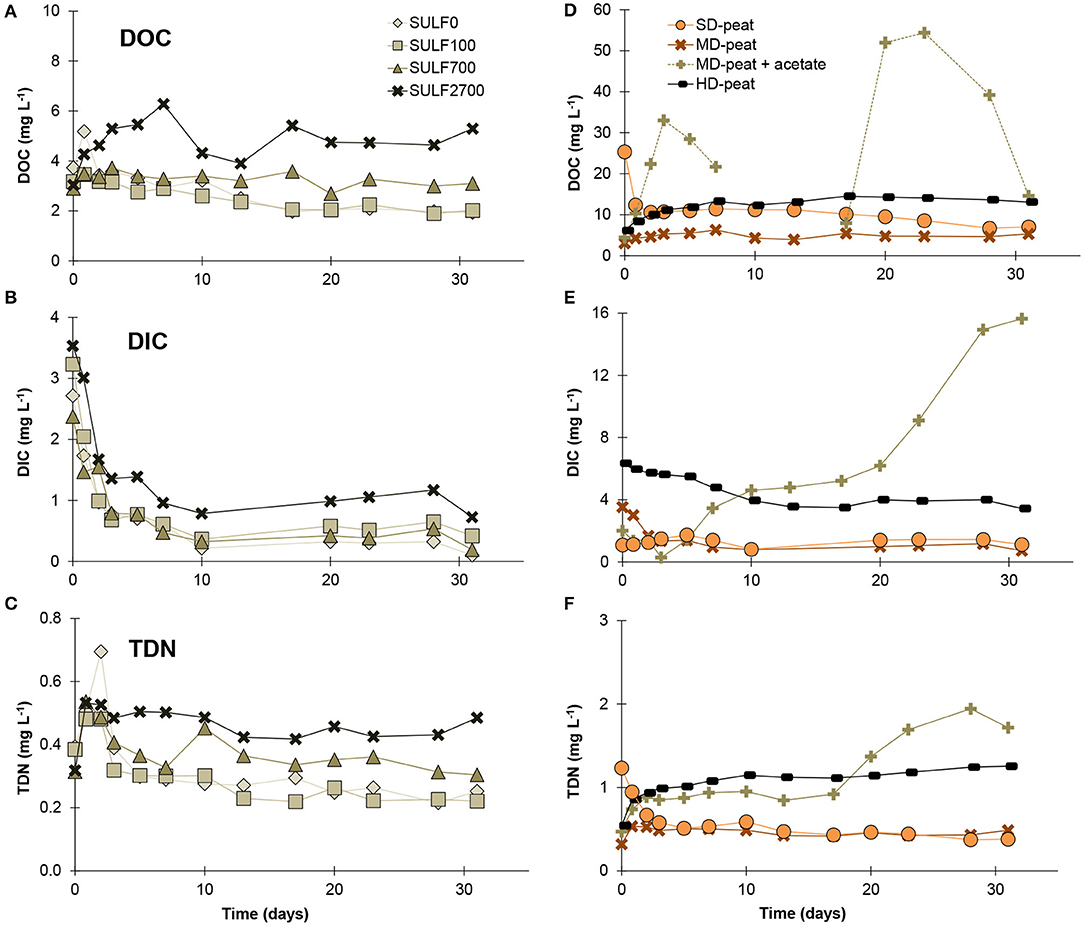
Figure 3. Average concentrations (n = 3) for DOC, DIC, and TDN in the effluent of the flow-through reactors during the SO42− applications (A–C) for the different SO42− treatments and (D–F) for the different degrees of decomposition for the SULF2700 treatment and for the MD-peat for the SULF2700+A treatment. Note the scaling differences.
This result also does not confirm previous findings by Ardón et al. (2016), who observed a decreasing DOC release with higher SO42− concentrations (range of 100 to 300 mg SO42− L−1). Ardón et al. (2016) concluded that the consumption of DOC via SO42− reduction was responsible for this decline. However, peat samples undergoing the SULF0, SULF100, SULF700, and SULF2700 treatment exhibited no pronounced SO42− reduction, hence Ardón et al. (2016) conclusion cannot be considered as the cause for the observed differences in DOC/DIC/TDN between the treatments. Instead, minor differences observed in DOC release from the different SO42− treatments may be attributed to anion exchange of DOM anions by SO42− (Brouns et al., 2014). An increased dispersion of organic matter caused by a replacement of calcium (Ca2+) by Na+ (e.g., Edelstein et al., 2010) is considered unlikely as increased Ca2+ concentrations were observed in effluent immediately after the start of the SO42− treatments (see segment about cation-related processes below). In contrast, DOC release in the different treatments differed more toward the end of the experiment.
The release of TDN over time resembled the release of DOC, which is reflected by a correlation coefficient calculated between 0.5 and 0.9, indicating a strong relationship between these two parameters. This result suggests that the majority of the nitrogen released derived from DOM rather than from . This is consistent with observations made in a shrub-dominated peatland by Wang et al. (2016) who found that 68% of nitrogen compounds released from peat monoliths were dissolved organic nitrogen (DON), while and NO3−/ made up only 8 and 24%, respectively. In this study, NO3− (method detection limit: 0.05 mg L−1) was detected only irregularly and in a low concentration between 0 to 0.13 mg L−1 in the effluent of MD-peat samples.
The release of DIC as an indicator for decomposition of organic matter showed less treatment-dependent tendencies than DOC and TDN. The SULF0, SULF100, and SULF700 treatments caused similar releases of DIC. Only peat samples treated with SULF2700 led to slightly higher DIC concentrations. However, DIC concentrations in the effluent of all samples first decreased before stabilizing at values between 0 and 1 mg L−1. This supports the assumption that none of the SO42− concentrations led to a pronounced SO42− reduction in the time frame of this experiment. As the decrease also occurred for the SULF0 treatment, it can be assumed that the decrease is the continuation of the initial leaching of DIC.
Increased Na+ concentrations from the input solution resulted in cation exchange leading to variable increases in different cation concentrations throughout the experiment (data not shown). For the MD-peat, the concentrations of Ca2+ and K+ increased shortly after the treatment commenced with the highest Ca2+/K+ peak occurring in samples treated with the greatest Na+ concentrations (SULF2700 and SULF2700+A) and subsequently decreased toward the value of the input solution. It stands to reason that Ca2+ and K+ were exchanged by Na+, although Ca2+ is generally bound more strongly to the peat than Na+ as a result of its bivalence (Succow and Joosten, 2001). Magnesium (Mg2+) concentrations increased very slowly over time and had not reached the input concentration value after 31 days, indicating that the displacement of other cations by Mg2+ took longer than the experimental duration. Total dissolved manganese (TDMn) (showing a peak right after the start of the treatment and then stabilizing at a value between 0.12 and 0.28 mg L−1) and total dissolved aluminum (TDAl) (stable value, but graduated from SULF0 with 0.01 mg L−1 to SULF2700+A with 0.05 mg L−1) concentrations were lowest for the SULF0 treatment and highest for the SULF2700+A treatment suggesting that, for the corresponding ions (Mn2+, Al3+), the Na+ concentrations determined the release of these solutes. However, the evaluation is less clear for Fe and Mn as they may potentially be involved in the anaerobic decomposition processes and are present in different oxidation states that ICP measurements cannot distinguish. Increased concentrations of total dissolved iron (TDFe) were observed in all treatments compared to SULF0. Although, the order of magnitude of the measured peaks varied greatly (between 0.8 and 17 mg L−1) and did not correlate with the SO42− treatments.
Effect of Acetate
In contrast to the SO42−-only treatments, a pronounced increase of DIC release accompanied by an increase in HS− was observed for the acetate-enriched SULF2700+A treatment (Figure 4), providing evidence for SO42− reduction (with hydrogen carbonate and sulfide as end-products). The duration of the experiment covered only the initial phase of SO42− reduction as the concentrations of HS− and DIC were still increasing after 31 days. A pronounced SO42− reduction appeared to begin after ~ 17 days (= ~ 15 pore volumes). This time period represents a lag time during which redox conditions favorable for SO42− reduction established in the samples. The SRB are generally outcompeted by microorganisms active at higher redox potentials that get energy via aerobic respiration, denitrification or Fe(III) and Mn(IV) reduction. As major HS− concentrations and DIC increase were only observed in acetate addition treatment (SULF2700+A), it can be assumed that the peat in this study did not represent a suitable carbon source for the SRB under the prevailing laboratory conditions, to which also the non-increase of SO42− reduction rate for SO42−-treated bog peat has been attributed (Vile et al., 2003). These findings confirm recent results of isotopic measurement of DIC in the pore water of sea-exposed fen peat of the Hütelmoor field site, which indicate that the detected DIC concentrations do not originate from the submerged peat itself, but from easily degradable marine DOM (personal communication with J. Westphal, Leibniz Institute for Baltic Sea Research Warnemünde). The measured HS− concentrations were considerably lower than the measured DIC concentrations (comparison in molar concentrations see Figure 5). This means that either not all HS− was detected due to its chemical reactivity or other processes than SO42− reduction co-drove the DIC production. It is possible that a part of the released HS− was bound to the DOM (Heitmann and Blodau, 2006) or precipitated as iron sulfide (van der Welle et al., 2007) and was, therefore, not detected with the applied method in this study. Other mineralization processes such as Fe(III) reduction could have driven the DIC production as an increase of DOC and TDN in the SULF2700+A treatment was observed indicating a general stimulation of decomposition processes. Concentrations of TDFe were highly elevated in the SULF2700+A treatment (peak concentration of 28 mg L−1) compared to the acetate-free SULF2700 treatment (peak concentration of 2 mg L−1). These results imply that Fe(III) reduction had occurred, during which Fe2+ is released; yet, the time curve of HS− indicates that DIC production was mainly controlled by SO42− reduction (Figure 4A). This also applies to other parameters—overall, the release of DOC, DIC, TDN and total dissolved phosphorus (TDP) was stimulated by SO42− reduction. Conversely, the release of TDMn in the SULF2700+A treatment resembled its counterpart (SULF2700) and experienced only a slightly lower peak concentration. This suggests that TDMn-release was mainly affected by cation exchange and not Mn(IV) reduction, which is consistent with the gradations in TDMn-concentrations for the acetate-free treatments following Na+ input concentrations (see effect of sulfate concentration).
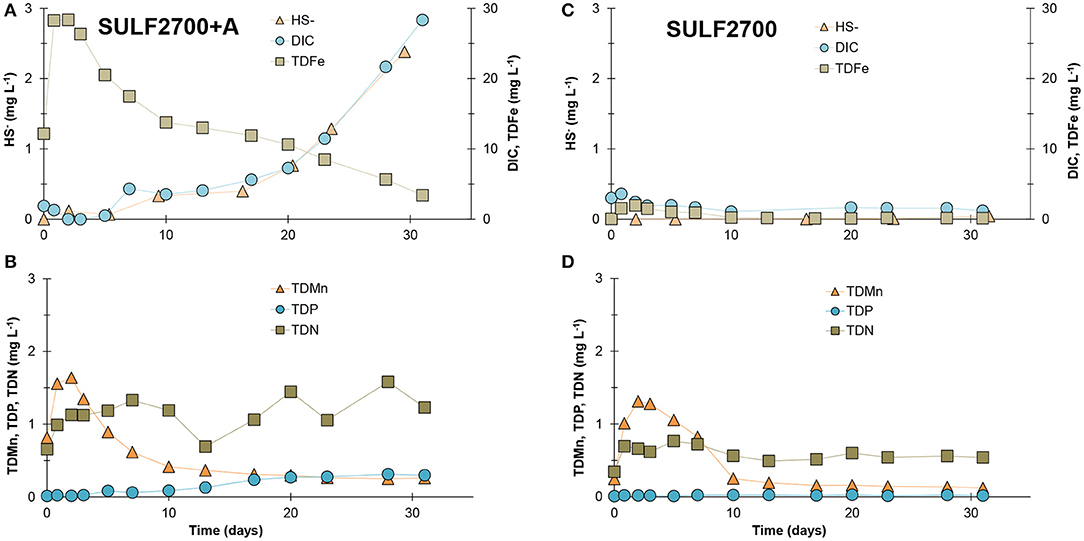
Figure 4. Release of HS−, DIC, and TDFe as well as TDMn, total dissolved phosphorus (TDP) and TDN measured in the effluent of the flow-through reactor from an individual peat sample during (A,B) SULF2700+A and (C,D) SULF2700 treatment.
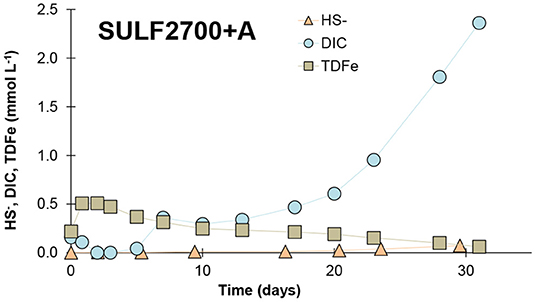
Figure 5. Release of end products of sulfate reduction (HS−, DIC) and of TDFe as interfering substance in molar concentration measured in the effluent of the flow-through reactor from an individual peat sample during SULF2700+A treatment.
Effect of Peat Decomposition Degree
The observed differences of solute release between the peats of varying degrees of decomposition were more pronounced and clearer than between the different SO42− concentrations applied to one peat material (Figure 3 for DOC/DIC/TDN, Figure 6 for TDFe). The substance release of the SD-peat matched the expectations that the DOC would decrease abruptly after an EC increase (see effect of sulfate concentration). Following an increase in EC from 3.6 mS cm−1 (SULF700) to 6.3 mS cm−1 (SULF2700), DOC and TDN concentrations decreased on average by 51 and 22%, respectively. In contrast, DOC and TDN concentrations varied only slightly for the MD-peat and responded immediately with an increase to the SULF2700 treatment for the HD-peat (Figure 3D). The observed differences in the time curve of DOC and TDN between the three peats could be related to the large differences in Fe content (Table 1), where the HD-peat contains 50 times more Fe than the SD-peat. As previously discussed (see initial flushing), the long-term drained and degraded peat generally has a different composition than less decomposed peat. While the percentage of organic carbon decreases with decomposition, the percentage of phosphorus (P), Fe and Al increases (Zeitz and Velty, 2002; Litaor et al., 2004). Knorr (2013) observed that DOC and TDFe concentrations had a clear positive correlation in pore and surface water while the impact of water salinity was not consistent for the two types of water. Iron salts such as ferric sulfate are well known to induce flocculation of fine organic and inorganic particles from the production of cationic hydrolysis products and are generally used to clean drinking water from DOM (Sharp et al., 2006). However, during Fe(III) reduction Fe2+ is released thereby co-mobilizing the previously flocculated DOM with other formerly bound solutes like phosphate. The correlation coefficient R2 between TDFe and DOC concentrations in the effluent of this study ranged between 0.36 (SULF2700) and 0.95 (SULF100) for the pure SO42− treatments of the MD-peat. The Fe-rich HD-peat samples have a calculated R2 value of 0.58, demonstrating Fe-related interference is an important impact factor for DOC.
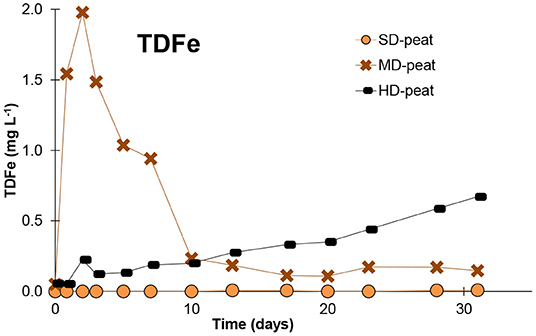
Figure 6. Release of TDFe from SD-, MD-, and HD-peat measured in the effluent of the flow-through reactors.
The observed differences between the SD-, MD-, and HD-peat in solute release could also be related to their pore-related parameters, such as porosity and proportion of the immobile pore regions detected via BTC analysis. Immobile zones provide an opposing effect on DOC concentrations where stagnant pore water zones may serve as hot spots for DOM production, as they represent the preferred habitat for microorganisms (Nunes et al., 2015; Rezanezhad et al., 2016). Immobile pore regions also increase the average pore water velocity and preferential flow thereby reducing the residence time of the water in the mobile pore regions and the potential for solute exchange between liquid and solid phase (Tiemeyer et al., 2017). However, as the release of DOC/TDN from SD-peat and MD-peat reached comparable values during the experiment, physical differences—whose effect should be long-lasting—may only be relevant to the HD-peat. Generally, the greater the degree of decomposition in peat the more pronounced the preferential flow occurs (Liu et al., 2017) and the immobile pore region fraction increases. In case of the HD-peat the greater proportion of immobile pore water might have contributed to the higher constant release of DOC/TDN.
Conclusions
In this flow-through experiment peat samples treated with sulfate (SO42−)-enriched water did not produce pronounced SO42− reduction within 1 month's time. SO42− and bromide (Br−) breakthrough curves were similar indicating that SO42− behaved as a conservative tracer within the experiment and that the SO42− penetration was dependent on the physical properties of the peat samples. The addition of acetate, a major electron donor in marine sediments, initiated SO42− reduction after ~17 days and led to a strong increase of release of solutes such as dissolved organic carbon (DOC) and inorganic carbon (DIC). This suggests that under these experimental conditions the composition and decomposability of the organic matter was more important to enabling decomposing processes than the presence of terminal electron acceptors. This was also demonstrated by the increased solute concentrations for peat with a higher degree of decomposition. However, increased SO42− concentrations did result in slightly elevated levels of DOC and total dissolved nitrogen (TDN). These results are attributed to the potential desorption of negatively charged organic molecules via anion exchange with SO42−. For the different tested peats, the short-term release of DOC was presumably affected not only by the ionic strength of the inflowing water but also by the iron (Fe) content of the peat, as DOC bound to Fe compounds can get mobilized during Fe(III) reduction. Overall, the main short-term effects of SO42−-rich seawater inflow in peatlands seem to be desorption processes, whose characteristics are controlled by the ionic composition and strength of the seawater, and peat chemistry, particularly Fe content and degree of decomposition. Decomposition-related solute release may develop in the long-term, when anoxic, stagnant conditions prevail. This may be accelerated if marine, easily degradable organic matter is flushed in the peatland during seawater flooding even though concentrations of bioavailable DOC might be lower than the applied acetate concentrations in this study. The onset of SO42− reduction may reduce the gaseous emissions of carbon-containing methane (CH4) from the peatland, but may also increase the load of dissolved carbon compounds in the peatland's outflow.
Data Availability Statement
The datasets generated for this study are available on request to the corresponding author.
Author Contributions
LG: design of experiment, data acquisition, data analysis, data interpretation, and drafting of manuscript. HT: data acquisition and revision of draft. MK: data interpretation and revision of draft. MJ: design of experiment, data interpretation, and revision of draft. FR: design of experiment, data analysis, data interpretation, and revision of draft. BL: design of experiment and revision of draft.
Funding
This study was conducted within the framework of the Research Training Group Baltic TRANSCOAST funded by the DFG (Deutsche Forschungsgemeinschaft) under grant number GRK 2000 (www.baltic-transcoast.uni-rostock.de). This is Baltic TRANSCOAST publication no. GRK2000/0026.
We acknowledge financial support by the DFG and the University of Rostock within the funding programme Open Access Publishing.
Conflict of Interest
The authors declare that the research was conducted in the absence of any commercial or financial relationships that could be construed as a potential conflict of interest.
Acknowledgments
We also would like to acknowledge the lab facilities provided by the Canada Excellence Research Chair program in Ecohydrology. We thank Marianne Vandergriendt (Ecohydrology Research Group, University of Waterloo, Canada) for water sample analysis and all members from the Ecohydrology Research Group who helped with the experiment and analysis.
References
Algeo, T. J., Luo, G. M., Song, H. Y., Lyons, T. W., and Canfield, D. E. (2015). Reconstruction of secular variation in seawater sulfate concentrations. Biogeosciences 12, 2131–2151. doi: 10.5194/bg-12-2131-2015
Ardón, M., Helton, A. M., and Bernhardt, E. S. (2016). Drought and saltwater incursion synergistically reduce dissolved organic carbon export from coastal freshwater wetlands. Biogeochemistry 127, 411–426. doi: 10.1007/s10533-016-0189-5
Ardón, M., Morse, J. L., Colman, B. P., and Bernhardt, E. S. (2013). Drought-induced saltwater incursion leads to increased wetland nitrogen export. Glob. Chang. Biol. 19, 2976–2985. doi: 10.1111/gcb.12287
Blodau, C., Mayer, B., Peiffer, S., and Moore, T. R. (2007). Support for an anaerobic sulfur cycle in two Canadian peatland soils. J. Geophys. Res. Biogeosciences 112, 1–10. doi: 10.1029/2006JG000364
Borch, T., Kretzschmar, R., and Kappler, A. (2010). Biogeochemical redox processes and their impact on contaminant dynamics - environmental science & technology (ACS Publications). Environ. Sci. Technol. 44, 15–23. doi: 10.1021/es9026248
Boschker, H. T., De Graaf, W., Köster, M., Meyer-Reil, L. A., and Cappenberg, T. E. (2001). Bacterial populations and processes involved in acetate and propionate consumption in anoxic brackish sediment. FEMS Microbiol. Ecol. 35, 97–103. doi: 10.1111/j.1574-6941.2001.tb00792.x
Brouns, K., Verhoeven, J. T. A., and Hefting, M. M. (2014). The effects of salinization on aerobic and anaerobic decomposition and mineralization in peat meadows: the roles of peat type and land use. J. Environ. Manage. 143, 44–53. doi: 10.1016/j.jenvman.2014.04.009
Caraco, N. F., Cole, J. J., and Likens, G. E. (1989). Evidence for sulphate-controlled phosphorus release from sediments of aquatic systems. Nature 341, 316–318. doi: 10.1038/341316a0
Clark, J. M., Van Der Heijden, G. M. F., Palmer, S. M., Chapman, P. J., and Bottrell, S. H. (2011). Variation in the sensitivity of DOC release between different organic soils following H2SO4 and sea-salt additions. Eur. J. Soil Sci. 62, 267–284. doi: 10.1111/j.1365-2389.2010.01344.x
Cline, J. D. (1969). Spectrophotometric determination of hydrogen sulfide in natural waters. Limnol. Oceanogr. 14, 454–458. doi: 10.4319/lo.1969.14.3.0454
DIN EN 15935 (2012). (Deutsches Institut für Normung e.V.) Sludge, Treated Biowaste, Soil and Waste - Determination of Loss on Ignition. Berlin: Beuth Verlag.
Edelstein, M., Ben-Hur, M., and Plaut, Z. (2010). Water salinity and sodicity effects on soil structure and hydraulic properties. Adv. Hortic. Sci. 24, 154–160.
Gardner, L. R. (1990). Simulation of the diagenesis of carbon, sulfur, and dissolved oxygen in salt marsh sediments. Ecol. Monogr. 60, 91–111. doi: 10.2307/1943027
Gosch, L., Janssen, M., and Lennartz, B. (2018). Impact of the water salinity on the hydraulic conductivity of fen peat. Hydrol. Process. 32, 1214–1222. doi: 10.1002/hyp.11478
Hahn, J., Köhler, S., Glatzel, S., and Jurasinski, G. (2015). Methane exchange in a coastal fen in the first year after flooding - A systems shift. PLoS ONE 10:e140657. doi: 10.1371/journal.pone.0140657
Hanin, M., Ebel, C., Ngom, M., Laplaze, L., and Masmoudi, K. (2016). New insights on plant salt tolerance mechanisms and their potential use for breeding. Front. Plant Sci. 7:1787. doi: 10.3389/fpls.2016.01787
Hausmann, B., Knorr, K., Schreck, K., Tringe, S. G., Glavina, T., Loy, A., et al. (2016). Consortia of low-abundance bacteria drive sulfate reduction-dependent degradation of fermentation products in peat soil microcosms. ISME J. 10, 2365–2375. doi: 10.1038/ismej.2016.42
Heitmann, T., and Blodau, C. (2006). Oxidation and incorporation of hydrogen sulfide by dissolved organic matter. Chem. Geol. 235, 12–20. doi: 10.1016/j.chemgeo.2006.05.011
HFA (2014). (Gutachterausschuss Forstliche Analytik) Handbuch Forstliche Analytik (HFA): Eine Loseblatt-Sammlung der Analysemethoden im Forstbereich. Bundesministerium fr Ernung und Landwirtschaft (BMEL), 5th ed. Bonn.
Johnson, D. W., and Todd, D. E. (1983). Relationships among iron, aluminum, carbon, and sulfate in a variety of forest soils. Soil Sci. Soc. Am. J. 47:792–800. doi: 10.2136/sssaj1983.03615995004700040035x
Jørgensen, N. O., Andersen, M. S., and Engesgaard, P. (2008). Investigation of a dynamic seawater intrusion event using strontium isotopes (87Sr/86Sr). J. Hydrol. 348, 257–269. doi: 10.1016/j.jhydrol.2007.10.001
Kasimir-Klemedtsson, Å., Klemedtsson, L., Berglund, K., Martikainen, P., Silvola, J., and Oenema, O. (1997). Greenhouse gas emissions from farmed organic soils: a review. Soil Use Manag. 13, 245–250. doi: 10.1111/j.1475-2743.1997.tb00595.x
Kester, D. R., Duedall, I. W., Connors, D. N., and Pytkowicz, R. M. (1967). Preparation of artificial seawater. Limnol. Oceanogr. 12, 176–179. doi: 10.4319/lo.1967.12.1.0176
Kleimeier, C., Rezanezhad, F., Cappellen, P., and Van, L.ennartz, B. (2017). Influence of pore structure on solute transport in degraded and undegraded fen peat soils. Mires Peat 19, 1–9. doi: 10.19189/MaP.2017.OMB.282
Knorr, K. H. (2013). DOC-dynamics in a small headwater catchment as driven by redox fluctuations and hydrological flow paths - are DOC exports mediated by iron reduction/oxidation cycles? Biogeosciences 10, 891–904. doi: 10.5194/bg-10-891-2013
Koebsch, F., Winkel, M., Liebner, S., Liu, B., Westphal, J., Spitzy, A., et al. (2019). Sulfate deprivation triggers high methane production in a disturbed and rewetted coastal peatland. Biogeosciences 16, 1937–1953. doi: 10.5194/bg-16-1937-2019
Kreuzburg, M., Ibenthal, M., Janssen, M., Rehder, G., Voss, M., Naumann, M., et al. (2018). Sub-marine continuation of peat deposits from a coastal peatland in the southern baltic sea and its holocene development. Front. Earth Sci. 6:103. doi: 10.3389/feart.2018.00103
Küsel, K., Blöthe, M., Schulz, D., Reiche, M., and Drake, H. L. (2008). Microbial reduction of iron and porewater biogeochemistry in acidic peatlands. Biogeosciences 5, 1537–1549. doi: 10.5194/bg-5-1537-2008
Lamers, L. P. M., Tomassen, H. B. M., and Roelofs, J. G. M. (1998). Sulfate-induced eutrophication and phytotoxicity in freshwater wetlands. Environ. Sci. Technol. 32, 199–205. doi: 10.1021/es970362f
Laverman, A. M., Pallud, C., Abell, J., Cappellen, P., and Van (2012). Comparative survey of potential nitrate and sulfate reduction rates in aquatic sediments. Geochim. Cosmochim. Acta 77, 474–488. doi: 10.1016/j.gca.2011.10.033
Litaor, M. I., Reichmann, O., Auerswald, K., Haim, A., and Shenker, M. (2004). The geochemistry of phosphorus in peat soils of a semiarid altered wetland. Soil Sci. Soc. Am. J. 68, 2078–2085. doi: 10.2136/sssaj2004.2078
Liu, H., Forsmann, D. M., Kjærgaard, C., Saki, H., and Lennartz, B. (2017). Solute transport properties of fen peat differing in organic matter content. J. Environ. Qual. 46, 1106–1113. doi: 10.2134/jeq2017.01.0031
Mandernack, K. W., Lynch, L., Krouse, H. R., and Morgan, M. D. (2000). Sulfur cycling in wetland peat of the New Jersey Pinelands and its effect on stream water chemistry. Geochim. Cosmochim. Acta 64, 3949–3964. doi: 10.1016/S0016-7037(00)00491-9
McCarter, C. P. R., Weber, T. K. D., and Price, J. S. (2018). Competitive transport processes of chloride, sodium, potassium, and ammonium in fen peat. J. Contam. Hydrol. 217, 17–31. doi: 10.1016/j.jconhyd.2018.08.004
Münch, J. M., Totsche, K. U., and Kaiser, K. (2002). Physicochemical factors controlling the release of dissolved organic carbon from columns of forest subsoils. Eur. J. Soil Sci. 53, 311–320. doi: 10.1046/j.1365-2389.2002.00439.x
Nunes, F. L. D., Aquilina, L., de Ridder, J., Francez, A.-J., Quaiser, A., Caudal, J.-P., et al. (2015). Time-scales of hydrological forcing on the geochemistry and bacterial community structure of temperate peat soils. Sci. Rep. 5:14612. doi: 10.1038/srep14612
Oremland, R. S., Marsh, L. M., and Polcin, S. (1982). Methane production and simultaneous sulphate reduction in anoxic, salt marsh sediments. Nature 296, 143–145. doi: 10.1038/296143a0
Ottow, J. C. G. (2011). Mikrobiologie von Böden - Biodiversität, Ökophysiologie und Metagenomik. Heidelberg: Springer-Verlag. doi: 10.1007/978-3-642-00824-5
Ours, D. P., Siegel, D. I., and Glaser, P. H. (1997). Chemical dilation and the dual porosity of humified bog peat. J. Hydrol. 196, 348–360. doi: 10.1016/S0022-1694(96)03247-7
Pallud, C., Meile, C., Laverman, A. M., Abell, J., Cappellen, P., and Van (2007). The use of flow-through sediment reactors in biogeochemical kinetics: methodology and examples of applications. Mar. Chem. 106, 256–271. doi: 10.1016/j.marchem.2006.12.011
Pallud, C., and Van Cappellen, P. (2006). Kinetics of microbial sulfate reduction in estuarine sediments. Geochim. Cosmochim. Acta 70, 1148–1162. doi: 10.1016/j.gca.2005.11.002
Rezanezhad, F., Price, J. S., and Craig, J. R. (2012). The effects of dual porosity on transport and retardation in peat: a laboratory experiment. Can. J. Soil Sci. 92, 723–732. doi: 10.4141/cjss2011-050
Rezanezhad, F., Price, J. S., Quinton, W. L., Lennartz, B., Milojevic, T., and Van Cappellen, P. (2016). Structure of peat soils and implications for water storage, flow and solute transport: a review update for geochemists. Chem. Geol. 429, 75–84. doi: 10.1016/j.chemgeo.2016.03.010
Ritson, J. P., Bell, M., Graham, N. J. D., Templeton, M. R., Brazier, R. E., Verhoef, A., et al. (2014). Simulated climate change impact on summer dissolved organic carbon release from peat and surface vegetation: Implications for drinking water treatment. Water Res. 67, 66–76. doi: 10.1016/j.watres.2014.09.015
Schmaljohann, R. (1996). Methane dynamics in the sediment and water column of Kiel Harbour (Baltic Sea). Mar. Ecol. Prog. Ser. 131, 263–273. doi: 10.3354/meps131263
Sharp, E. L., Parsons, S. A., and Jefferson, B. (2006). The impact of seasonal variations in DOC arising from a moorland peat catchment on coagulation with iron and aluminium salts. Environ. Pollut. 140, 436–443. doi: 10.1016/j.envpol.2005.08.001
Sherif, M. M., and Singh, V. P. (1999). Effect of climate change on sea water intrusion in coastal aquifers. Hydrol. Process. 13, 1277–1287.
Stam, M. C., Mason, P. R. D., Pallud, C., and Van Cappellen, P. (2010). Sulfate reducing activity and sulfur isotope fractionation by natural microbial communities in sediments of a hypersaline soda lake (Mono Lake, California). Chem. Geol. 278, 23–30. doi: 10.1016/j.chemgeo.2010.08.006
Stutter, M. I., Lumsdon, D. G., and Cooper, R. J. (2007). Temperature and soil moisture effects on dissolved organic matter release from a moorland Podzol O horizon under field and controlled laboratory conditions. Eur. J. Soil Sci. 58, 1007–1016. doi: 10.1111/j.1365-2389.2006.00880.x
Succow, M., and Joosten, H. (2001). Landschaftsökologische Moorkunde, 2nd ed. Stuttgart: E. Schweizerbart'sche Verlagsbuchhandlung.
Tiemeyer, B., Pfaffner, N., Frank, S., Kaiser, K., and Fiedler, S. (2017). Pore water velocity and ionic strength effects on DOC release from peat-sand mixtures: results from laboratory and field experiments. Geoderma 296, 86–97. doi: 10.1016/j.geoderma.2017.02.024
Toride, N., Leij, F. J., and van Genuchten, M. T. (1999). The CXTFIT Code for Estimating Transport Parameters from Laboratory or Field Tracer Experiments. Version 2.1. Riverside, CA: Research Report 137, US Salinity Laboratory, Agricultural Research Service, US Department of Agriculture.
van der Welle, M. E. W., Smolders, A. J. P., Op Den Camp, H. J. M., Roelofs, J. G. M., and Lamers, L. P. M. (2007). Biogeochemical interactions between iron and sulphate in freshwater wetlands and their implications for interspecific competition between aquatic macrophytes. Freshw. Biol. 52, 434–447. doi: 10.1111/j.1365-2427.2006.01683.x
Vile, M. A., Bridgham, S. D., and Wieder, R. K. (2003). Response of anaerobic carbon mineralization rates to sulfate amendments in a boreal peatland. Ecol. Appl. 13, 720–734. doi: 10.1890/1051-0761(2003)013[0720:ROACMR]2.0.CO;2
Wang, H., Richardson, C. J., Ho, M., and Flanagan, N. (2016). Drained coastal peatlands: A potential nitrogen source to marine ecosystems under prolonged drought and heavy storm events—A microcosm experiment. Sci. Total Environ. 566–567, 621–626. doi: 10.1016/j.scitotenv.2016.04.211
Wen, X., Unger, V., Jurasinski, G., Koebsch, F., Horn, F., Rehder, G., et al. (2018). Predominance of methanogens over methanotrophs contributes to high methane emissions in rewetted fens. Biogeosci. Discuss. 15, 6519–6536. doi: 10.5194/bg-15-6519-2018
Weston, N. B., Vile, M. A., Neubauer, S. C., and Velinsky, D. J. (2011). Accelerated microbial organic matter mineralization following salt-water intrusion into tidal freshwater marsh soils. Biogeochemistry 102, 135–151. doi: 10.1007/s10533-010-9427-4
Wieder, R. K., and Lang, G. E. (1988). Cycling of inorganic and organic sulfur in peat from Big Run Bog, West Virginia. Biogeochemistry 5, 221–242. doi: 10.1007/BF02180229
Zak, D., and Gelbrecht, J. (2007). The mobilisation of phosphorus, organic carbon and ammonium in the initial stage of fen rewetting (a case study from NE Germany). Biogeochemistry 85, 141–151. doi: 10.1007/s10533-007-9122-2
Zak, D., Goldhammer, T., Cabezas, A., Gelbrecht, J., Gurke, R., Wagner, C., et al. (2018). Top soil removal reduces water pollution from phosphorus and dissolved organic matter and lowers methane emissions from rewetted peatlands. J. Appl. Ecol. 55, 311–320. doi: 10.1111/1365-2664.12931
Zak, D., Wagner, C., Payer, B., Augustin, J., and Gelbrecht, J. (2010). Phosphorus mobilization in rewetted fens: the effect of altered peat properties and implications for their restoration. Ecol. Appl. 20, 1336–1349. doi: 10.1890/08-2053.1
Keywords: fen peat, sulfate, solute transport, solute release, coastal zone
Citation: Gosch L, Townsend H, Kreuzburg M, Janssen M, Rezanezhad F and Lennartz B (2019) Sulfate Mobility in Fen Peat and Its Impact on the Release of Solutes. Front. Environ. Sci. 7:189. doi: 10.3389/fenvs.2019.00189
Received: 18 June 2019; Accepted: 13 November 2019;
Published: 27 November 2019.
Edited by:
Laodong Guo, University of Wisconsin–Milwaukee, United StatesReviewed by:
Dominik Zak, Aarhus University, DenmarkJohn Crusius, Alaska Science Center (United States Geological Survey), United States
Copyright © 2019 Gosch, Townsend, Kreuzburg, Janssen, Rezanezhad and Lennartz. This is an open-access article distributed under the terms of the Creative Commons Attribution License (CC BY). The use, distribution or reproduction in other forums is permitted, provided the original author(s) and the copyright owner(s) are credited and that the original publication in this journal is cited, in accordance with accepted academic practice. No use, distribution or reproduction is permitted which does not comply with these terms.
*Correspondence: Lennart Gosch, lennart.gosch@uni-rostock.de