- 1Centre of Excellence PLECO (Plant and Ecosystems), Department of Biology, University of Antwerp, Wilrijk, Belgium
- 2Laboratoire Ecologie, Evolution, Interactions des Systèmes Amazoniens (LEEISA), Université de Guyane, CNRS, IFREMER, Cayenne, French Guiana
- 3INRA, UMR Ecology of Guiana Forests (EcoFoG), AgroParisTech, Cirad, CNRS, Université des Antilles, Université de Guyane, Kourou, French Guiana
- 4The Centre for Research on Ecology and Forestry Applications, Cerdanyola del Vallès, Catalonia, Spain
- 5Lawrence Berkeley National Laboratory, Climate and Ecosystem Science Division, Berkeley, CA, United States
Tropical soils are a major contributor to the balance of greenhouse gas (GHG) fluxes in the atmosphere. Models of tropical GHG fluxes predict that both the frequency of drought events and changes in atmospheric deposition of nitrogen (N) will significantly affect dynamics of soil carbon dioxide (CO2) and methane (CH4) production and consumption. In this study, we examined the combined effect of a reduction in precipitation and an increase in nutrient availability on soil CO2 and CH4 fluxes in a primary French Guiana tropical forest. Drought conditions were simulated by intercepting precipitation falling through the forest canopy with tarpaulin roofs. Nutrient availability was manipulated through application of granular N and/or phosphorus (P) fertilizer to the soil. Soil water content (SWC) below the roofs decreased rapidly and stayed at continuously low values until roof removal, which as a consequence roughly doubled the duration of the dry season. After roof removal, SWC slowly increased but remained lower than in the control soils even after 2.5 months of wet-season precipitation. We showed that drought-imposed reduction in SWC decreased the CO2 emissions (i.e., CO2 efflux), but strongly increased the CH4 emissions. N, P, and N × P (i.e., NP) additions all significantly increased CO2 emission but had no effect on CH4 fluxes. In treatments where both fertilization and drought were applied, the positive effect of N, P, and NP fertilization on CO2 efflux was reduced. After roof removal, soil CO2 efflux was more resilient in the control plots than in the fertilized plots while there was only a modest effect of roof removal on soil CH4 fluxes. Our results suggest that a combined increase in drought and nutrient availability in soil can locally increase the emissions of both CO2 and CH4 from tropical soils, for a long term.
Introduction
Climate models predict a range of changes in weather patterns in tropical forest regions (Feng et al., 2013). Across Amazonia, a drier and warmer climate is expected for the coming century, which is predicted to result in an increased frequency of drought events, and can put a strain on the Amazon's ecological functioning (Duffy et al., 2015). In addition, atmospheric deposition of nitrogen (N) can result in a stoichiometric imbalance of carbon (C) and N relative to phosphorus (P) in tropical biomes (Peñuelas et al., 2013). These global changes can have a broad impact on tropical ecosystem functioning and are predicted to lead to a severe disturbance of the Amazonian forest biome (Huntingford et al., 2004). Even though the exact magnitude of this projection is still debated (Cook et al., 2012), modeling studies report that mature tropical forests are highly vulnerable to extreme drought events (Phillips et al., 2009) and to changes in precipitation regimes in combination with modifications in nutrient stoichiometry (Malhi et al., 2008). However, soil biological processes, which are an important determinant of forest functioning, remain poorly described in models focusing on forest ecosystems.
Soils are an important source and sink of radiatively active trace-gases, i.e., carbon dioxide (CO2) and methane (CH4). Soil trace gas production and consumption are highly sensitive to soil moisture (Davidson et al., 2004, 2008) through its effect on soil redox state, diffusion, nutrient pools, and microbial activity (Conrad, 1996; Davidson et al., 2004, 2008). Several studies have explored the effect of precipitation regimes on soil trace-gas emissions either through experimental throughfall exclusion (Davidson et al., 2004; Cleveland et al., 2010; Wood and Silver, 2012; Meir et al., 2015) or comparisons of annual emissions of years differing in throughfall (Bonal et al., 2008; Rowland et al., 2014; Doughty et al., 2015; O'Connell et al., 2018). These studies report contrasting results with either a reduction (Bonal et al., 2008; Wood and Silver, 2012; Rowland et al., 2014), an increase (Cleveland et al., 2010; O'Connell et al., 2018), or no change (Davidson et al., 2008) in soil CO2 emissions (i.e., soil CO2 efflux; soil respiration) with drought. Discrepancies in the direction, and especially extent, of fluxes are related to differences in forest or soil types (Meir et al., 2015) or the structure of microbial communities (Schimel et al., 2007). The few studies that have investigated the effect of drought on soil CH4 fluxes report a net reduction in CH4 emissions (Davidson et al., 2004, 2008; Wood and Silver, 2012; O'Connell et al., 2018).
Apart from drought, changes in nutrient availability also impact soil gas fluxes in tropical forests. It is generally acknowledged that P rather than N is limiting in lowland tropical forests (Martinelli et al., 1999; Camenzind et al., 2018; Wright et al., 2018; Wright, 2019), and therefore we might expect stronger effects of P addition rather than N addition. In agreement with this, in several tropical forests around the world it has been shown that P addition tends to result in a strong increase in microbial biomass (Cleveland et al., 2002; Kaspari et al., 2008; Liu et al., 2013; Fanin et al., 2015). Fewer data are available on the effect of P addition on fluxes of trace gases, but P addition tended to increase soil CO2 efflux (Cleveland and Townsend, 2006) and CH4 consumption (Zhang et al., 2011). Nitrogen addition also tended to increase soil CO2 efflux in a field experiment in Costa Rica (Cleveland and Townsend, 2006) while N had no effect or induced a decrease in soil CO2 efflux in China (Mo et al., 2008). Such discrepancies are likely related to multiple factors including microbial community composition, soil properties, precipitation, and differences in initial N availability among tropical forests. Nitrogen addition alone tends to have an inhibitive effect on methanotrophs and thus lower the consumption of CH4 by soils (Zhang et al., 2011). Several studies have shown that simultaneous additions of both N and P did not impact soil microbial structure or functioning (Liu et al., 2013; Fanin et al., 2015), possibly because increased P availability mitigates the inhibitive effect of N addition on soil CH4 consumption. Together, these studies indicate that we are far from predicting the combined effect of nutrient addition and drought on trace-gas fluxes in soils.
In a temperate ecosystem, Aronson et al. (2012) showed positive effects of soil water content (SWC) and N addition on soil CH4 fluxes, but it is unsure whether such results will extrapolate to tropical forest ecosystems. Moreover, little is known about the capacity of soils to recover from one or multiple stressors, such as drought and changes in nutrient availability. Previous results in mature tropical forests suggest that during post-drought periods, soil CO2 efflux (as a result of both root/autotrophic respiration and microbial/heterotrophic respiration) were stimulated by the return of water leading to both renewed root growth and a large increase of microbial activity (Bonal et al., 2008; O'Connell et al., 2018). O'Connell et al. (2018) showed a large peak of CH4 emissions after drought, higher than the pre-drought period, a decrease of soil aeration, and a change of organic and inorganic P content. Prolonged dry periods can be associated with soil nutrient accumulation which is then made available for microorganisms during soil rewetting (Fierer and Schimel, 2002). Moreover, increased root exudation (Preece and Penuelas, 2016), litterfall (Wagner et al., 2013), or microbial mortality during or after drought can also contribute to an increase in heterotrophic respiration once SWC is restored (Bengough et al., 2011).
To our knowledge, no study to date has addressed the combined effect of nutrient availability and drought on soil GHG fluxes in tropical forests. Field-based throughfall exclusion experiments combined with fertilization are therefore urgently needed to improve model-based simulations, which are currently not accounting for these effects.
In the current experiment, we asked the following question: how quickly and to what extent do soil GHG fluxes (CO2 and CH4) respond to changes in nutrient content and water content in a tropical forest soil? To answer this question, we conducted a full factorial drought and NP fertilization experiment during 1 year in a mature tropical forest of the Guiana shield. We hypothesized a strong positive effect of SWC on soil CO2 effluxes and a negative effect on CH4 fluxes. We hypothesized a positive effect of nutrients on CO2 production, but we had no a priori expectation on the effect on soil CH4 fluxes as both CH4 production and CH4 consumption could be stimulated with unknown resulting flux. Furthermore, we expected any effect of nutrients to be conditional on SWC such that its effect was less pronounced when water is limiting.
Materials and Methods
Study Site
The study was conducted in French Guiana, South America, at the Paracou research station (Figure 1A, 05°16′54″N, 52°54′44″W). Decadal average annual rainfall at the study site was 3,102 mm ± 70 mm and average annual air temperature was 25.7°C ± 0.1°C (from 2004 to 2014, Aguilos et al., 2019). The tropical wet climate of French Guiana is highly seasonal due to the north/south movement of the Inter-Tropical Convergence Zone. This zone brings heavy rains from December to July (wet season, with precipitation between 300 and 500 mm mo−1) and a long dry season from mid-August to mid-November. Precipitation during the dry period is typically <100 mm mo−1 (Aguilos et al., 2019). Soils at Paracou are predominantly schist soils with veins of pegmatite along a Precambrian metamorphic formation called the Bonidoro series (Sabatier et al., 1997). The soils are mostly nutrient-poor acrisols (FAO et al., 1998).
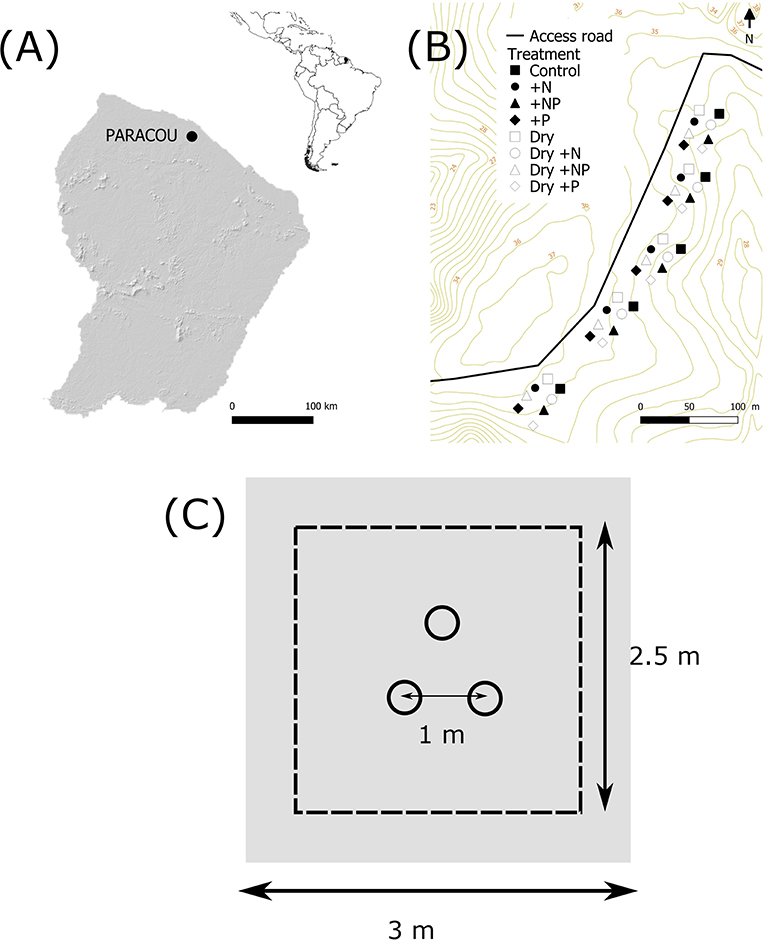
Figure 1. Site locations and experimental design. (A) Location of the Paracou Research Station, French Guiana. The insert shows the location of French Guiana in South America. (B) Location of the 40 plots in the top hill, close to the trail of the station. (C) Experimental set up of the plot: three collars spaced by 1 m, the area of fertilization was 2.5 × 2.5 m plot and the throughfall exclusion was 3 × 3 m.
Experimental Design
The study zone covered ~2.4 ha (400 m by 60 m, Figure 1B). A full factorial experimental design was applied with drought (two levels: ambient or “Dry”) and fertilization (four levels: “Control,” “+N,” “+P,” or “+NP”) treatments crossed. Forty plots (2.5 × 2.5 m) were represented by three PVC soil collars, each at least 1.0 m apart (i.e., 120 collars in total, Figure 1C). The collars (20 cm in diameter, 7 cm in height) were installed to a depth of ~4 cm into the soil 3 months prior to the first measurement and were left in the field during the full study period.
Drought Treatment
In order to simulate a prolonged drought period, 20 plots (“Dry”) were covered by translucent plastic tarpaulins, creating a roof 1.50 m above the soil surface. Edge effects were avoided by using tarpaulins (i.e., 3 × 3 m) larger than the plot surface area. The tarpaulins were installed in October 2017 (dry season) before the return of the rainy season and removed in April 2018 during the rainy season. Plots were not trenched to minimize soil disturbance and allow natural nutrient cycling and water movements between the roots and microorganisms responsible for CO2 and CH4 fluxes, following the design of Wood and Silver (2012). Nearby the plots, litter traps of the same size as the tarpaulins were set up at 1.50 m above the soil surface. Once every 2 weeks, the litter was collected from the litter traps and homogeneously spread on the soil surface below the tarpaulin of the corresponding plot. No trees were cut or damaged during the treatment application.
Fertilization Treatments
In January 2018 (3 months after starting the drought treatment), a fertilization treatment was applied to 30 different 2.5 × 2.5 m plots either with N (125 kg N ha−1 as coated urea [(NH2)2CO]), P (50 kg P ha−1 as triple superphosphate [Ca(H2PO4)2 H2O]) or with a mixture of these two elements. In total, five replicate plots with 15 sample points (three collars per plots) per combination of treatments (drought/fertilization) were therefore available. Previous studies conducted at the same site (Paracou research station) showed an average natural annual inputs of N and P via leaf litterfall of 65 kg N ha−1 y−1 and 1.4 kg P ha−1 y−1, respectively. The amount of nutrients added in this experiment therefore correspond to twice the annual N input and 35 times the annual P input from leaf litter decomposition (Bonal et al., 2008; Hättenschwiler et al., 2008; Barantal et al., 2012). Our nutrient addition is similar to the amount of nutrients applied in other fertilization experiments in tropical forests (Wright et al., 2018; Wright, 2019).
Soil Characteristics
Soil samples for chemical and physical characterization were collected at the start of the experiment (September 2017, dry season). A composite soil sample of three topsoil cores of 8 cm by 15 cm (width/depth; Bi-partite root auger, Eijkelkamp, The Netherlands) in each plot was analyzed to determine the amount of clay, silt, sand, organic C, total N, aluminum (Al), iron (Fe), total P, and cation exchange capacity (CEC). Analyses were carried out by the soil analysis laboratory of INRA (Arras, France). Soil bulk density (BD) to a depth of 5 cm was measured with 100-cm3 cylinders. Soil pH (KCl) was measured by mixing 10 g of moist soil with 1 M KCl in a 1:2.5 ratio. The resulting slurry was stirred for 1 h, then allowed to sit for another hour before measuring with a pH probe. After fertilization (January 2018), available P (ppm) was measured by Bray-P acid fluoride extraction (Bray and Kurtz, 1945) of soil dried at 60°C for 48 h; the resulting solution was analyzed on an iCAP 6300 Duo ICP optical emission spectrometer (Thermo Fisher Scientific, USA). Available N (ppm), defined as the sum of the and concentrations, was measured by extracting moist soil with 1 M KCl, after which the concentrations of and were determined colorimetrically (San++ continuous flow analyzer, Skalar Inc., Breda, The Netherlands).
Soil Gas Measurements
Soil gas flux measurement campaigns were carried out once a month from September to December 2017, every week from January to March 2018 (following fertilization), and every 2 weeks from March to July 2018.
Soil CO2 and CH4 fluxes were measured between 10:00 and 15:00 local time with two Ultraportable Greenhouse Gas Analyzers (UGGA, Los Gatos Research Inc., Mountain View, USA) connected to two home-made PVC chambers of 2.564 L operating in closed circuit and including an internal fan for mixing air inside each chamber. The volumes of each collar were summed, corresponding to a total volume of 3.882 ± 0.193 L for the UGGA/chamber system. The fluxes were measured during 5 min measurement periods (CO2 and CH4 concentration logged every 20 s), this period was sufficient to detect fluxes of each gas (Courtois et al., 2019). Two consecutive days (hereafter referred to as a sampling campaign) were necessary to measure the soil gas fluxes, because of the restricted time period of measurement. Fluxes were computed with the HMR package (Pedersen, 2011) for the two gases using linear regression (LR), or revised Hutchinson/Mosier (HMR) methods following recommendations from Pedersen (2011). SWC and soil surface temperature were recorded next to each collar during each sampling campaign using a dielectric soil moisture sensor, with general mineral soil calibration, at a depth of 5 cm (SM150T, Delta-T Devices, England) and a thermometer at a depth of 10 cm (HI 98501, Hanna instruments, USA), respectively.
Data Analysis
We used mean values per plot for all variables and data were first evaluated for assumptions of normality and homoscedasticity prior to further statistical analyses. To meet these assumptions, the soil CH4 flux data were log transformed with a base of 10 (gas flux + most negative gas flux in dataset + 1). A Tukey-Kramer test was used to compare the changes in nutrient availabilities in the soil after fertilization. In addition, a principal component analysis (PCA, Figure S1) was performed to identify main relationships between the different soil properties and plots later assigned to treatments.
To assess how the drought and fertilization treatments affected CO2 and CH4 fluxes in the soil before and after roof removal, linear mixed-effects models were run, where fertilization treatment (+N, +P, +NP, Control), drought treatment (Dry, Control) and sampling campaign were treated as fixed factors and plot as random factor. Models were selected minimizing AIC.
Linear regression models were used to investigate the relationships between soil fluxes, i.e., CO2 and CH4, and SWC. For CO2 and CH4 fluxes in the dry plots, the relationships were best described by:
And, for CH4 fluxes in the Control plots by:
where SWC is the soil water content (m3 m−3) and a, b, and c the constants fitted in each regression model.
Resilience indices, from Orwin and Wardle (2004), were calculated to assess the recovery of soil CO2 and CH4 fluxes after removing the roof, characterizing the end of the drought treatment. This resilience index, which compares the absolute difference that exists between post-drought and control treatment relative to the initial absolute effect of the drought, ranges from −1 to +1, with +1 indicating maximum resilience (being complete recovery after rewetting).
All statistical analyses were conducted and figures produced using R statistical software (R Core Team, 2018) using the packages dplyr (Wickham et al., 2015), nlme (Lindstrom and Bates, 1990), multcomp (Bretz et al., 2016), MuMIn (Barton, 2009), and FactoMineR (Husson et al., 2013).
Results
Soil samples had a high sand content (77.84% of sand), a low nutrient concentration (1.71% of C, 0.12% of N, and 0.022% of P), a low nutrient availability (18.87 mg of N and 1.06 mg of P) and a low pH (3.88). Soil characteristics were very similar between plots (Table S1, Figure S1).
One month after initiation of the throughfall exclusion treatment (i.e., prolonged drought initiated with roof installation), SWC of dry plots was roughly half that of control plots, and this difference remained over the course of the experiment (Figure 2A). As a consequence, the throughfall exclusion treatment extended the duration of the dry season by at least a factor 2 maintaining a SWC of ~10%, whereas control plots followed precipitation events resulted in SWC between 15 and 35% (Figure 2A). After roof removal, differences in SWC decreased but control plots remained wetter until the end of the experiment. The respective fertilization treatments significantly increased N and P availability in soil (Figures 2B,C; 3 fold for N under N addition and 10 fold for P under P addition).
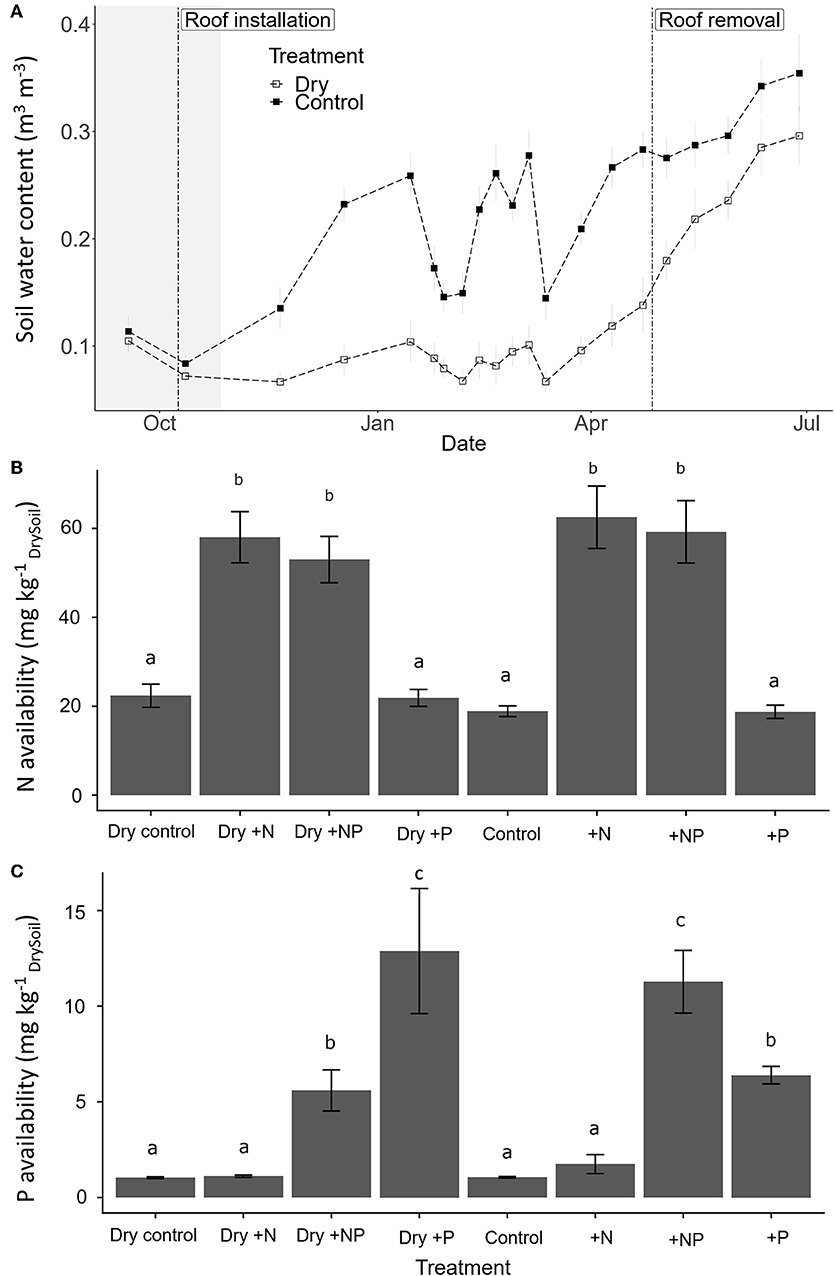
Figure 2. (A) Temporal change in mean soil water content (SWC; ± SE; 0–5 cm; n = 20) in the controls (close square) and “dry-control” for the throughfall exclusion plots (open square) over the study period, French Guiana. Vertical dashed line indicate the dates of the roof installation (09/10/2017) and roof removal (27/04/2018) in the throughfall exclusion plots. The shaded area represents the dry season 2017. (B) Effect of fertilization on available N and (C) on available P in soils 2 weeks after fertilization. Different lowercase letters indicate significant differences among treatments; error bars indicate standard error of the mean (n = 5).
Both drought, fertilization and the combination of both had a significant impact on CO2 efflux (Table 1, Figure 3A). The effect of fertilization with N only and P only on CO2 fluxes was transient where no throughfall exclusion was applied; CO2 efflux started to increase 19 days (i.e., sampling campaign #3) after the fertilization, reached a peak (62% increased as compared to control plots) at 34 days (i.e., sampling campaign #5) and returned to the same range of magnitude of the control plots 55 days after fertilization (Figures 4A,B). In the combined drought and fertilization (Dry +N, Dry +P, and Dry +NP) however, Dry +N had no effect on CO2 efflux (Figure 4A), while Dry +P steadily increased CO2 efflux from the first measurement until about day 82 (i.e., sampling campaign #10) after fertilization (Figure 4B). Addition of both N and P (+NP) on all plots immediately increased CO2 efflux (86% increased as compared to control), which kept increasing until 26 and 82 days (for no throughfall exclusion and throughfall exclusion, respectively) and remained steady until the end of the experiment (Figure 4C). This increase in CO2 efflux was stronger and earlier in +NP plots than in +N or +P plots. The increase 26 days after the fertilization was stronger in plots with no throughfall exclusion than in plots where rain was excluded (Figure 4C).
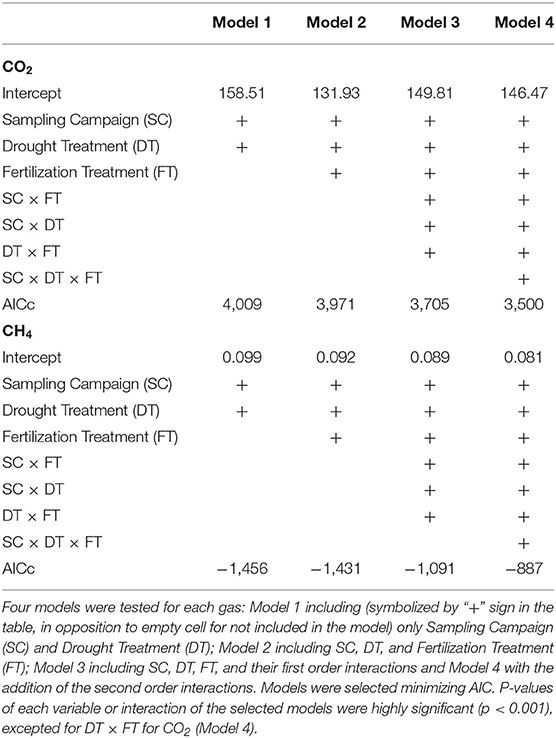
Table 1. Results from the linear mixed models for CO2 and CH4 fluxes of the soil, in a tropical forest, French Guiana.
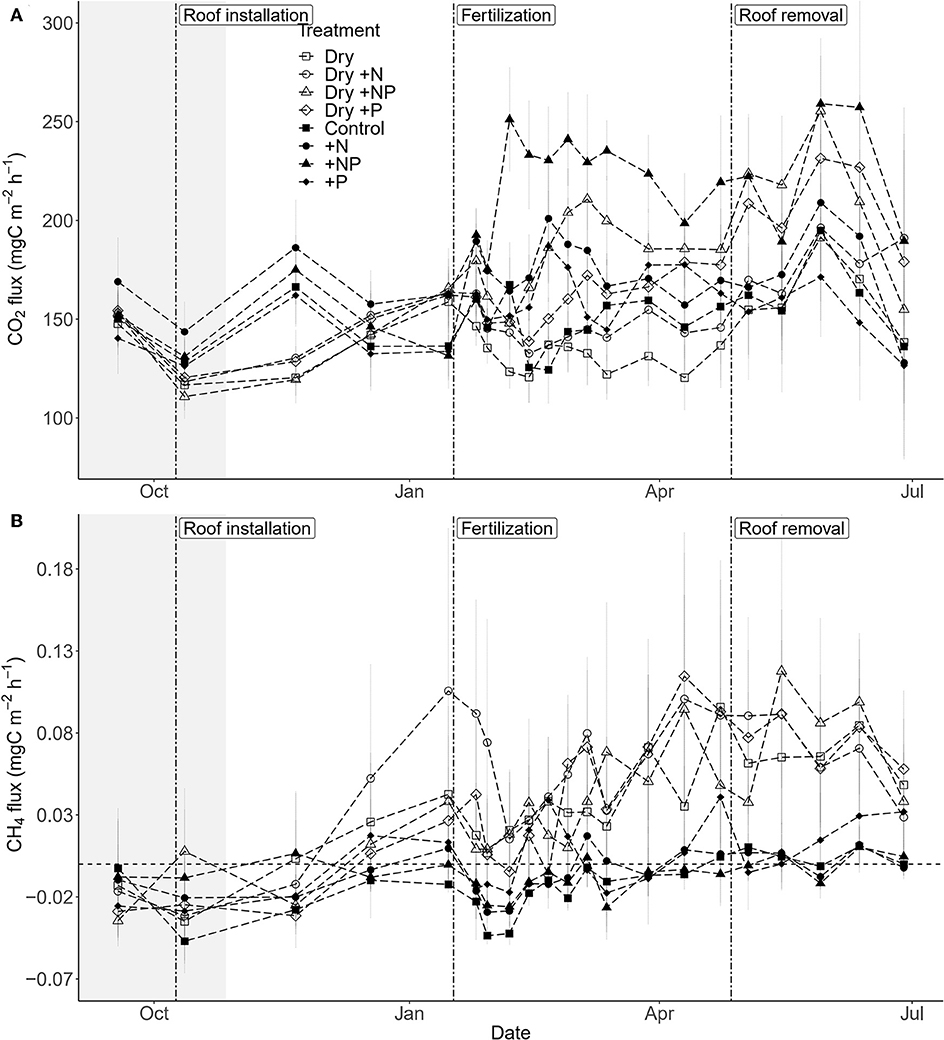
Figure 3. Temporal change in (A) mean soil CO2 efflux and (B) mean soil CH4 flux over the study period (± SE; n = 5 plots per treatment) in a tropical forest, French Guiana. Vertical dashed lines indicate the dates of the roof installation (09/10/2017), roof removal (27/04/2018), and fertilization (17/01/2018). The shaded area represents the dry season 2017. Drought treatment is symbolized by open symbols, fertilization by circles for +N, triangles for +NP, and diamonds for +P and squares for controls.
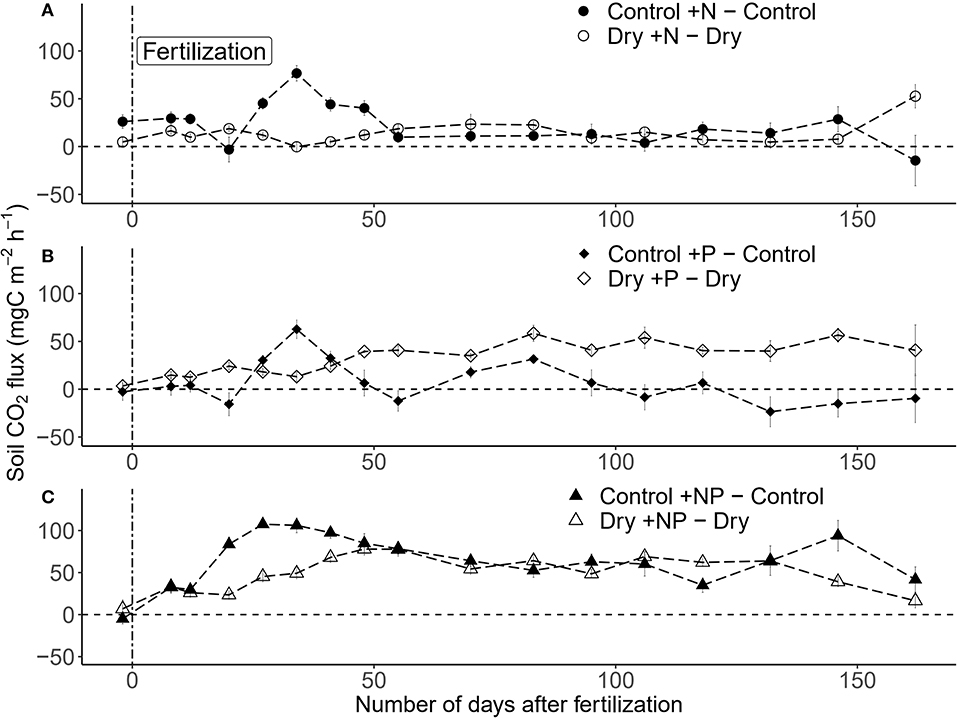
Figure 4. Differences among treatments for (A) +N, (B) +P, and (C) +NP over the study period (± SE; n = 5 plots per treatment) in a tropical forest, French Guiana. The vertical dashed line indicates the date of the fertilization (17/01/2018).
Overall, resilience of soil CO2 efflux to drought was greater in unfertilized than in fertilized plots (Figure 5A). Over a two-month period after roof removal, resilience of CO2 efflux was high and steady in the control plots, low and steady in +P plots or strongly decreased in +N and +NP plots.
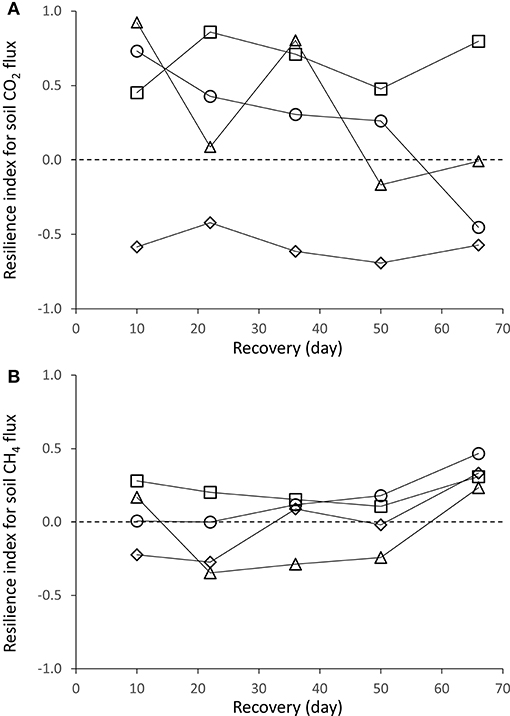
Figure 5. Change in the resilience index for (A) CO2 and (B) CH4 fluxes of the soil, in a tropical forest, French Guiana; where control plots are symbolized by squares, +N by circles, +NP by triangles, and +P by diamonds.
Only throughfall exclusion had an impact on CH4 fluxes (Table 1, Figure 3B). All plots where rain was excluded, regardless of the fertilization treatments, displayed higher CH4 fluxes than plots where rain was not excluded. This effect resulted in a change of the relationships between CH4 fluxes and SWC (Figure 6). In control plots (without roof), soils shifted from a sink under dryer conditions to a source under wetter conditions (Figure 6). In plots where rain was excluded, there was a strong increase in CH4 fluxes, even under dryer conditions (SWC <15%, Figure 6).
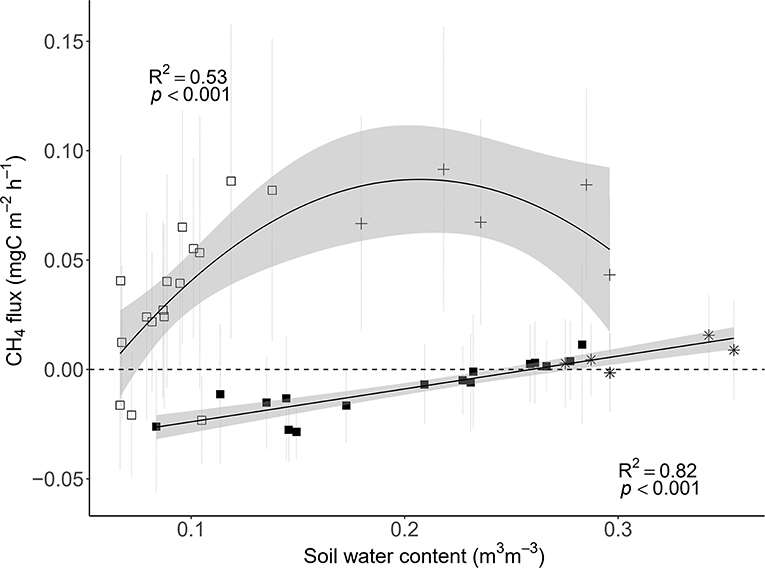
Figure 6. Relationships between soil CH4 fluxes and soil water content (SWC) in the control (closed square and black star; SoilFluxes = SWC−0.04) and dry plots (open square and black cross; SoilFluxes = SWC2+0.02 SWC−0.09) for the entire study period (n = 21 sampling campaigns; 18/09/2017–28/06/2018) in a tropical forest, French Guiana. The roof removal period is symbolized by the black stars and crosses. For both functions, the values of the slopes are very closed to 0.
Resilience of soil CH4 fluxes to drought was in the same order of magnitude for unfertilized and fertilized plots. Fifty days after roof removal, resilience of soil CH4 fluxes slightly increased in all plots (Figure 5B). As SWC gradually increased in plots after roof removal, the shape of the relationship between SWC and CH4 displayed a negative trend toward lower CH4 fluxes with higher SWC.
Discussion
In this study, we report on an in situ manipulation of N and P fertilization combined with a drought treatment on two soil GHG fluxes (CO2 and CH4), in a tropical rainforest. These treatments had complex but marked effects on both soil GHG fluxes. Soil CO2 efflux consistently increased with fertilization but was reduced by drought-imposed reductions in SWC. In contrast to CO2 fluxes, none of the nutrient additions (i.e., +N, +P, and +NP) had an effect on soil CH4 flux, but the drought treatment led to an increase in CH4 emission. Below we will further discuss each of these main findings.
Combined Effects of Fertilization and Drought on Soil CO2 Efflux
Our experiment showed a positive effect of fertilization on soil CO2 efflux, which was modulated by drought. A low soil CO2 efflux, but not lower than the pretreatment period, was maintained during the artificial drought period, which lasted for at least two times the regular duration of the dry season.
The response to the fertilization treatments was rapid (≈1 month; Figures 3A, 4) but relatively transient for +N and +P, compared to +NP. Although soils in French Guiana have very low concentrations in available P (Grau et al., 2017; Van Langenhove et al., 2019) compared with many southern and western Amazonian soils [i.e., 10 times lower for Acrisols (Quesada et al., 2010)], our results showed a higher CO2 efflux in +NP fertilized soils than in +N or +P alone, suggesting co-limitation of N and P. In concordance, previous studies on the same tropical French Guianese soils have demonstrated the positive impact of N and P additions on faunal decomposers and litter decomposition rates (Barantal et al., 2012; Fanin et al., 2015, 2016) but no effects when N or P were added alone. This co-limitation of soil respiration contrasts with findings from fertilization experiments in tropical lowland forests in Panama (Kaspari et al., 2008), Costa Rica (Cleveland and Townsend, 2006), and Hawaii (Hobbie and Vitousek, 2000), which showed a significant and positive effect of N or P addition on soil organic matter decomposition. One possible explanation for the different soil responses is the difference between soils across these tropical sites, where all study sites mentioned above had soils that were richer in N and P compared to our site in French Guiana [i.e., 0.02% vs. 0.10% P content and 0.12% vs. 0.50% N content for this study and a soil in Costa Rica (Cleveland and Townsend, 2006), respectively]. The strong and rapid effects of NP fertilization and to a lesser extent N and P fertilization alone on CO2 efflux observed here may in part be attributed to the extremely nutrient-poor soil at our site. This is further corroborated by results from Soong et al. (2018) who showed that CO2 efflux was most increased with a combined application of NPK in an incubation experiment with soil from the same site. The modest effects of the singly added N on CO2 efflux could however be due to acidification, a known side-effect of adding Urea (Chen et al., 2016; Riggs and Hobbie, 2016); a decrease in pH can negatively affect microbial activities and mask microbial N limitation. If this were the case, it was however overcome when P was added along with N as evidenced by the high CO2 efflux in the +NP treatment.
A comparison between fertilized plots and dry fertilized plots showed that a decrease in SWC tended to mitigate the fertilization effects on CO2 efflux. The effect of our throughfall exclusion experiment on soil CO2 efflux was in the same order of magnitude as a synthesis of eight throughfall exclusion experiments in humid tropical forests (Wood and Silver, 2012). That study showed a reduction of CO2 of ~20% (in our study ~16% for the February–April period and ~25% in November). A strong relationship between soil CO2 efflux and natural changes in SWC evidences the predominant soil water control on temporal variations in C fluxes, especially during the dry to wet-season transition (October 2017–January 2018), compared with low variation in CO2 efflux in the dry plots. Precipitation, driving SWC, can modify biophysical and biogeochemical soil conditions and thereby soil CO2 efflux in complex ways, to which root and microbial respiration contribute approximately equally in tropical forests (Hanson et al., 2000). In our study, the highest SWC for CO2 efflux was ~28% in the control plots (Figure S2), regardless of fertilization regime. Above or below this optimum, soil CO2 efflux decreased by a maximum factor of 1.4. This finding is in agreement with previous studies performed in the same site (Rowland et al., 2014; Courtois et al., 2019). On the one hand, excess SWC can create a physical barrier for gas diffusion between the soil and the atmosphere, and a biological inhibitor for root and microbial activities because of the decrease, or even absence, of O2 (Wood et al., 2013). On the other hand, low SWC in dry soil can strongly reduce nutrient availability and impose water limitation and thereby decrease soil CO2 efflux. Therefore, CO2 efflux rates remained low in the dry plots, where SWC was rarely above 10%. In contrast to bio-physical expectations, O'Connell et al. (2018) observed an increase in soil CO2 effluxes under natural drought conditions, which can be explained by different soil conditions such as higher nutrient content, lower bulk density, and texture. In our study, soil CO2 dynamics were driven by SWC and soil nutrient contents, necessary for nutrient exchanges and nutrient uptake by roots and micro-organisms. However, additional measurements on soil and plant properties would be necessary to better understand the mechanism behind the interactions of fertilization and drought and partitioning between microbial and root CO2 efflux.
Our results show that soil CO2 effluxes in the control plots were more resilient to drought than those in the fertilized plots. We explain the increase to initial levels of soil CO2 efflux (heterotrophic and autotrophic respiration) after roof removal by both renewed root growth and a pulse of microbial activity as described in previous studies (Fierer and Schimel, 2002; O'Connell et al., 2018). However, this resilience of soil CO2 effluxes to drought was particularly low and more variable in the fertilized plots, suggesting a long-lasting effects of the N, NP, and P addition treatments (over a 105-day period) on soil C dynamics. The possible rapid reversible effect of a long drought, and its interaction with nutrient levels and limitation, should be of interest to further improve C cycling models of tropical forests.
No Combined Effects of Fertilization and Drought on Soil CH4 Flux
Soils shifted from sinks of CH4 during the dry season (beginning of the experiment, shaded area in Figure 3B) to sources of CH4 during the wet season in the control plots, which is in agreement with other studies in tropical forests (Keller and Reiners, 1994; Keller et al., 2005; Davidson et al., 2008; Teh et al., 2014; Courtois et al., 2018). Increased precipitation is likely to decrease rates of O2 diffusion into the soil (Silver et al., 1999; Teh et al., 2005; Liptzin et al., 2011), which potentially increases CH4 production. Furthermore, soil water saturation can create a physical barrier for gas diffusion, and potentially stop the root and microbial activities because of a lack of available O2. Studies on drought effects on soil CH4 flux however remain sparse, although all showed an increase in CH4 consumption (Davidson et al., 2004, 2008) with extremely high spatial heterogeneity (Wood and Silver, 2012; Courtois et al., 2019). In our study however, soils are mainly a source under drought treatment, with highest CH4 emissions when SWC was about 20% in the dry plots.
In contrast to our hypothesis, relationships between SWC and CH4 flux were complex in that its relationship differed between dry and control plots, where dry plot soils were consistent sources of CH4 even when where SWC levels overlapped between treatments. Importantly, this positive effect of drought persisted during the entire study period of throughfall exclusion, with no clear reason. A possible explanation can be distinct responses of the CH4 producers and consumers to soil water conditions. In their study, Benstead and King (1997) reported that methanotrophs are particularly sensitive to water stress, creating larger emissions of CH4 in dry soils due to lower consumption of CH4. Also, it is possible that belowground substrate availability to methanogens is reduced in the dry season but not in the wet season, which could cause variation in topsoil SWC to differentially affect methane fluxes between natural and imposed drought conditions. Therefore, more information about methanogen behavior to soil water limitation is necessary. Soil water conditions can control the gas diffusion as well as the distribution of CH4 producers and consumers along the soil profile as shown by Koehler et al. (2012) in a tropical lowland forest in Panama. Finally, soil fauna (e.g., termites) can also significantly contribute to the CH4 emissions but no particular changes in the presence of the soil macro-fauna (e.g., termites ant ants) was observed in our plots during the study period.
Fertilization with N and P alone or combined did not change the CH4 flux. These results did not corroborate with findings by Hanson and Hanson (1996) who reported a decrease in CH4 uptake due to a shift from CH4 oxidation to ammonia oxidation by methanotrophs with added ammonium resulting from urea hydrolysis. Our results also did not support a previous study by Zhang et al. (2011) that reported an increase in CH4 consumption after P fertilization most likely due to an increase in methanotrophic abundance. Phosphorus supply in soils can increase pH, water and nutrient uptake by plant roots, which can lead to higher O2 diffusivity and O2 availability in soil pore spaces for methanotrophs (Zhang et al., 2011). Also, P addition can induce a significant increase in microbial biomass (Cleveland et al., 2002; Kaspari et al., 2008; Liu et al., 2012, 2013; Fanin et al., 2015) even if these effects were transient and disappeared over longer periods. However, several studies showed that simultaneous addition of both N and P did not impact soil microbial structure and functioning (Liu et al., 2013; Fanin et al., 2015), but an increase in P availability might mitigate the inhibitive effect of N addition on soil CH4 consumption. These studies highlight that the relationships between bio-physical processes behind CH4 fluxes and soil nutrient contents are still unknown and possibly site-specific.
During 50 days after roof removal, natural precipitation had a modest effect on soil CH4 fluxes, which was corroborated by low resilience indices (Figure 5B). However, differences in soil CH4 fluxes between dry and control plots tended to disappear once rain was allowed back into the dry plots (Figure 6), suggesting a possible reversible effect in the longer term, regardless of fertilization regime. Davidson et al. (2008) also reported a recovery capacity of the soils for the N2O and CH4 fluxes after a drought treatment. They suggested that changes in the balance of the gaseous production and consumption via nitrification, denitrification, methanogenesis, and methanotrophy recovered by the return of the precipitation where a change in the soil aeration was instrumental in this recovery.
Conclusion
In this in situ experimental study, we show marked differences in the responses of soil C dynamics depending on whether nutrients and water were applied or withheld, respectively, as single or combined treatments. Differences were (i) a positive effect of N and P additions, mitigated by SWC via imposed drought conditions, for CO2 efflux, and (ii) a positive and strong effect of SWC for CH4 flux. Surprisingly, fertilization only affected soil CO2 efflux, and drought caused soil to become sources of CH4 instead of sinks. These results suggested that changes in nutrients and water contents in soils most likely influence the complex processes of CO2 and CH4 exchanges, which are controlled by multiple biophysical and biogeochemical conditions, e.g., methanotroph activities. Studies of soil microbial properties are now needed to disentangle the effects of the fertilization and drought treatments on CO2 and CH4 fluxes. In this study, we also find that resilience of soil CO2 efflux after a long dry period, equal to at least twice the duration of a regular dry season, is high for unfertilized plots. While, although slow, the resilience for soil CH4 flux is consistent for all fertilization treatments. For both soil fluxes, we observe that resilience is slow, and should thus be examined over a long period of time. Finally, we highlight here that in tropical forest ecosystems nutrient and water availabilities, both expected to be affected by climate change, can dramatically affect soil GHG fluxes.
Data Availability Statement
Publicly available datasets were analyzed in this study. This data can be found here: https://zenodo.org/record/3372722.
Author Contributions
CS and EC conceived and designed the experiments. TS-G, CS, EC, LB, and LV carried out the field and lab-work. TS-G, CS, EC, and LB analyzed the data. LB, EC, and CS wrote the paper. All authors commented on the analysis, interpretation, and presentation of the data.
Funding
This work had benefited from an Investissements d'Avenir grant managed by Agence Nationale de la Recherche (CEBA, ref. ANR-10-LABX-25-01) and was supported by the European Research Council Synergy grant ERC-2013-SyG-610028-IMBALANCE-P and European Commission (Marie Curie Excellence Grant RainForest-GHG, H2020-MSCA-IF-2017-796438).
Conflict of Interest
The authors declare that the research was conducted in the absence of any commercial or financial relationships that could be construed as a potential conflict of interest.
Acknowledgments
The authors would like to thank Fred Kockelbergh and Joke Van den Berge for building the chambers. We are grateful to Jocelyn Cazal, Maricar Aguillos, Benoit Burban, Jean-Yves Goret, Baptiste Rabineau and the master students of the Module FTH for their help in the field. Finally, we also wish to thank the reviewers who provided incisive, constructive, and informed criticism of the manuscript.
Supplementary Material
The Supplementary Material for this article can be found online at: https://www.frontiersin.org/articles/10.3389/fenvs.2019.00180/full#supplementary-material
References
Aguilos, M., Stahl, C., Burban, B., Hérault, B., Courtois, E., Coste, S., et al. (2019). Interannual and seasonal variations in ecosystem transpiration and water use efficiency in a tropical rainforest. Forests 10, 1–14. doi: 10.3390/f10010014
Aronson, E. L., Vann, D. R., and Helliker, B. R. (2012). Methane flux response to nitrogen amendment in an upland pine forest soil and riparian zone. J. Geophys. Res. Biogeosciences. 117:G03012. doi: 10.1029/2012JG001962
Barantal, S., Schimann, H., Fromin, N., and Hättenschwiler, S. (2012). Nutrient and carbon limitation on decomposition in an Amazonian moist forest. Ecosystems 15, 1039–1052. doi: 10.1007/s10021-012-9564-9
Barton, K. (2009). MuMIn: Multi-Model Inference. R package version 0.12. 0. Available online at: http://r-forge.r-project.org/projects/mumin/ (accessed January 30, 2009).
Bengough, A. G., McKenzie, B., Hallett, P., and Valentine, T. (2011). Root elongation, water stress, and mechanical impedance: a review of limiting stresses and beneficial root tip traits. J. Exp. Bot. 62, 59–68. doi: 10.1093/jxb/erq350
Benstead, J., and King, G. M. (1997). Response of methanotrophic activity in forest soil to methane availability. FEMS Microbiol. Ecol. 23, 333–340. doi: 10.1016/S0168-6496(97)00041-X
Bonal, D., Bosc, A., Ponton, S., Goret, J. Y., Burban, B., Gross, P., et al. (2008). Impact of severe dry season on net ecosystem exchange in the Neotropical rainforest of French Guiana. Global Change Biol. 14, 1917–1933. doi: 10.1111/j.1365-2486.2008.01610.x
Bray, R. H., and Kurtz, L. (1945). Determination of total, organic, and available forms of phosphorus in soils. Soil Sci. 59, 39–46. doi: 10.1097/00010694-194501000-00006
Bretz, F., Hothorn, T., and Westfall, P. (2016). Multiple Comparisons Using R. New York, NY: Chapman and Hall/CRC. doi: 10.1201/9781420010909
Camenzind, T., Hättenschwiler, S., Treseder, K. K., Lehmann, A., and Rillig, M. C. (2018). Nutrient limitation of soil microbial processes in tropical forests. Ecol. Monogr. 88, 4–21. doi: 10.1002/ecm.1279
Chen, H., Gurmesa, G. A., Zhang, W., Zhu, X., Zheng, M., Mao, Q., et al. (2016). Nitrogen saturation in humid tropical forests after 6 years of nitrogen and phosphorus addition: hypothesis testing. Funct. Ecol. 30, 305–313. doi: 10.1111/1365-2435.12475
Cleveland, C. C., and Townsend, A. R. (2006). Nutrient additions to a tropical rain forest drive substantial soil carbon dioxide losses to the atmosphere. Proc. Natl. Acad. Sci. U.S.A. 103, 10316–10321. doi: 10.1073/pnas.0600989103
Cleveland, C. C., Townsend, A. R., and Schmidt, S. K. (2002). Phosphorus limitation of microbial processes in moist tropical forests: evidence from short-term laboratory incubations and field studies. Ecosystems 5, 0680–0691. doi: 10.1007/s10021-002-0202-9
Cleveland, C. C., Wieder, W. R., Reed, S. C., and Townsend, A. R. (2010). Experimental drought in a tropical rain forest increases soil carbon dioxide losses to the atmosphere. Ecology 91, 2313–2323. doi: 10.1890/09-1582.1
Conrad, R. (1996). Soil microorganisms as controllers of atmospheric trace gases (H2, CO, CH4, OCS, N2O, and NO). Microbiol. Mol. Biol. Rev. 60, 609–640.
Cook, B., Zeng, N., and Yoon, J. H. (2012). Will Amazonia dry out? Magnitude and causes of change from IPCC climate model projections. Earth Interact. 16, 1–27. doi: 10.1175/2011EI398.1
Courtois, E. A., Stahl, C., Burban, B., den Berge, J. V., Berveiller, D., et al. (2019). Automatic high-frequency measurements of full soil greenhouse gas fluxes in a tropical forest. Biogeosciences 16, 785–796. doi: 10.5194/bg-16-785-2019
Courtois, E. A., Stahl, C., Van den Berge, J., Bréchet, L., Van Langenhove, L., Richter, A., et al. (2018). Spatial variation of soil CO2, CH4 and N2O fluxes across topographical positions in tropical forests of the Guiana shield. Ecosystems 21, 1445–1458. doi: 10.1007/s10021-018-0232-6
Davidson, E. A., Ishida, F. Y., and Nepstad, D. C. (2004). Effects of an experimental drought on soil emissions of carbon dioxide, methane, nitrous oxide, and nitric oxide in a moist tropical forest. Global Change Biol. 10, 718–730. doi: 10.1111/j.1365-2486.2004.00762.x
Davidson, E. A., Nepstad, D. C., Ishida, F. Y., and Brando, P. M. (2008). Effects of an experimental drought and recovery on soil emissions of carbon dioxide, methane, nitrous oxide, and nitric oxide in a moist tropical forest. Global Change Biol. 14, 2582–2590. doi: 10.1111/j.1365-2486.2008.01694.x
Doughty, C. E., Metcalfe, D., Girardin, C., Amézquita, F. F., Cabrera, D. G., Huasco, W. H., et al. (2015). Drought impact on forest carbon dynamics and fluxes in Amazonia. Nature 519, 78–82. doi: 10.1038/nature14213
Duffy, P. B., Brando, P., Asner, G. P., and Field, C. B. (2015). Projections of future meteorological drought and wet periods in the Amazon. Proc. Natl. Acad. Sci. U.S.A. 112, 13172–13177. doi: 10.1073/pnas.1421010112
Fanin, N., Hättenschwiler, S., Chavez Soria, P. F., and Fromin, N. (2016). (A)synchronous availabilities of N and P regulate the activity and structure of the microbial decomposer community. Front. Microbiol. 6:1507. doi: 10.3389/fmicb.2015.01507
Fanin, N., Hättenschwiler, S., Schimann, H., and Fromin, N. (2015). Interactive effects of C, N and P fertilization on soil microbial community structure and function in an A mazonian rain forest. Funct. Ecol. 29, 140–150. doi: 10.1111/1365-2435.12329
FAO ISRIC, and ISSS. (1998). World Reference Base for Soil Resources. World Soil Resources Reports 84. FAO: Rome.
Feng, X., Porporato, A., and Rodriguez-Iturbe, I. (2013). Changes in rainfall seasonality in the tropics. Nat. Clim. Change 3, 811–815. doi: 10.1038/nclimate1907
Fierer, N., and Schimel, J. P. (2002). Effects of drying–rewetting frequency on soil carbon and nitrogen transformations. Soil Biol. Biochem. 34, 777–787. doi: 10.1016/S0038-0717(02)00007-X
Grau, O., Peñuelas, J., Ferry, B., Freycon, V., Blanc, L., Desprez, M., et al. (2017). Nutrient-cycling mechanisms other than the direct absorption from soil may control forest structure and dynamics in poor Amazonian soils. Sci. Rep. 7:45017. doi: 10.1038/srep45017
Hanson, P. J., Edwards, N. T., Garten, C. T., and Andrews, J. A. (2000). Separating root and soil microbial contributions to soil respiration: a review of methods and observations. Biogeochemistry 48, 115–146. doi: 10.1023/A:1006244819642
Hanson, R. S., and Hanson, T. E. (1996). Methanotrophic bacteria. Microbiol. Mol. Biol. Rev. 60, 439–471.
Hättenschwiler, S., Aeschlimann, B., Coûteaux, M. M., Roy, J., and Bonal, D. (2008). High variation in foliage and leaf litter chemistry among 45 tree species of a neotropical rainforest community. N. Phytol. 179, 165–175. doi: 10.1111/j.1469-8137.2008.02438.x
Hobbie, S. E., and Vitousek, P. M. (2000). Nutrient limitation of decomposition in Hawaiian forests. Ecology 81, 1867–1877. doi: 10.1890/0012-9658(2000)081[1867:NLODIH]2.0.CO;2
Huntingford, C., Harris, P., Gedney, N., Cox, P., Betts, R., Marengo, J., et al. (2004). Using a GCM analogue model to investigate the potential for Amazonian forest dieback. Theor. Appl. Climatol. 78, 177–185. doi: 10.1007/s00704-004-0051-x
Husson, F., Josse, J., Le, S., and Mazet, J. (2013). FactoMineR: Multivariate Exploratory Data Analysis and Data Mining With R. R package version 1. Available online at: http://CRAN.R-project.org/package=FactoMineR
Kaspari, M., Garcia, M. N., Harms, K. E., Santana, M., Wright, S. J., and Yavitt, J. B. (2008). Multiple nutrients limit litterfall and decomposition in a tropical forest. Ecol. Lett. 11, 35–43. doi: 10.1111/j.1461-0248.2007.01124.x
Keller, M., and Reiners, W. A. (1994). Soil-atmosphere exchange of nitrous oxide, nitric oxide, and methane under secondary succession of pasture to forest in the Atlantic lowlands of Costa Rica. Global Biogeochem. Cycles 8, 399–409. doi: 10.1029/94GB01660
Keller, M., Varner, R., Dias, J. D., Silva, H., Crill, P., de Oliveira, R. C. Jr., et al. (2005). Soil–atmosphere exchange of nitrous oxide, nitric oxide, methane, and carbon dioxide in logged and undisturbed forest in the Tapajos National Forest, Brazil. Earth Interact. 9, 1–28. doi: 10.1175/EI125.1
Koehler, B., Corre, M. D., Steger, K., Well, R., Zehe, E., Sueta, J. P., et al. (2012). An in-depth look into a tropical lowland forest soil: nitrogen-addition effects on the contents of N2O, CO2 and CH4 and N2O isotopic signatures down to 2-m depth. Biogeochemistry 111, 695–713. doi: 10.1007/s10533-012-9711-6
Lindstrom, M. L., and Bates, D. M. (1990). Nonlinear mixed effects models for repeated measures data. Biometrics 46, 673–687. doi: 10.2307/2532087
Liptzin, D., Silver, W. L., and Detto, M. (2011). Temporal dynamics in soil oxygen and greenhouse gases in two humid tropical forests. Ecosystems 14, 171–182. doi: 10.1007/s10021-010-9402-x
Liu, L., Gundersen, P., Zhang, T., and Mo, J. (2012). Effects of phosphorus addition on soil microbial biomass and community composition in three forest types in tropical China. Soil Biol. Biochem. 44, 31–38. doi: 10.1016/j.soilbio.2011.08.017
Liu, L., Zhang, T., Gilliam, F. S., Gundersen, P., Zhang, W., Chen, H., et al. (2013). Interactive effects of nitrogen and phosphorus on soil microbial communities in a tropical forest. PLoS ONE 8:e61188. doi: 10.1371/journal.pone.0061188
Malhi, Y., Roberts, J. T., Betts, R. A., Killeen, T. J., Li, W., and Nobre, C. A. (2008). Climate change, deforestation, and the fate of the Amazon. Science 319, 169–172. doi: 10.1126/science.1146961
Martinelli, L., Piccolo, M., Townsend, A., Vitousek, P., Cuevas, E., McDowell, W., et al. (1999). Nitrogen stable isotopic composition of leaves and soil: tropical versus temperate forests. Biogeochemistry 46, 45–65. doi: 10.1007/BF01007573
Meir, P., Wood, T. E., Galbraith, D. R., Brando, P. M., Da Costa, A. C., Rowland, L., et al. (2015). Threshold responses to soil moisture deficit by trees and soil in tropical rain forests: insights from field experiments. Bioscience 65, 882–892. doi: 10.1093/biosci/biv107
Mo, J., Zhang, W., Zhu, W., Gundersen, P., Fang, Y., Li, D., et al. (2008). Nitrogen addition reduces soil respiration in a mature tropical forest in southern China. Global Change Biol. 14, 403–412. doi: 10.1111/j.1365-2486.2007.01503.x
O'Connell, C. S., Ruan, L., and Silver, W. L. (2018). Drought drives rapid shifts in tropical rainforest soil biogeochemistry and greenhouse gas emissions. Nat. Commun. 9:1348. doi: 10.1038/s41467-018-03352-3
Orwin, K. H., and Wardle, D. A. (2004). New indices for quantifying the resistance and resilience of soil biota to exogenous disturbances. Soil Biol. Biochem. 36, 1907–1912. doi: 10.1016/j.soilbio.2004.04.036
Pedersen, A. (2011). HMR: Flux Estimation With Static Chamber Data. R package version 0.31. Available online at: https://CRAN.R-project.org/package=FactoMineR
Peñuelas, J., Poulter, B., Sardans, J., Ciais, P., Van Der Velde, M., Bopp, L., et al. (2013). Human-induced nitrogen–phosphorus imbalances alter natural and managed ecosystems across the globe. Nat. Commun. 4:2934. doi: 10.1038/ncomms3934
Phillips, O. L., Aragão, L. E., Lewis, S. L., Fisher, J. B., Lloyd, J., López-González, G., et al. (2009). Drought sensitivity of the Amazon rainforest. Science 323, 1344–1347. doi: 10.1126/science.1164033
Preece, C., and Penuelas, J. (2016). Rhizodeposition under drought and consequences for soil communities and ecosystem resilience. Plant Soil 409, 1–17. doi: 10.1007/s11104-016-3090-z
Quesada, C. A., Lloyd, J., Schwarz, M., Patiño, S., Baker, T. R., Czimczik, C., et al. (2010). Variations in chemical and physical properties of Amazon forest soils in relation to their genesis. Biogeosciences 7, 1515–1541. doi: 10.5194/bg-7-1515-2010
R Core Team (2018). R: A Language and Environment for Statistical Computing. Open Source Software, Version 3.5.1. Vienna. Available online at: https://www.r-project.org
Riggs, C. E., and Hobbie, S. E. (2016). Mechanisms driving the soil organic matter decomposition response to nitrogen enrichment in grassland soils. Soil Biol. Biochem. 99, 54–65. doi: 10.1016/j.soilbio.2016.04.023
Rowland, L., Hill, T. C., Stahl, C., Siebicke, L., Burban, B., Zaragoza-Castells, J., et al. (2014). Evidence for strong seasonality in the carbon storage and carbon use efficiency of an Amazonian forest. Global Change Biol. 20, 979–991. doi: 10.1111/gcb.12375
Sabatier, D., Grimaldi, M., Prévost, M.-F., Guillaume, J., Godron, M., Dosso, M., et al. (1997). The influence of soil cover organization on the floristic and structural heterogeneity of a Guianan rain forest. Plant Ecol. 131, 81–108. doi: 10.1023/A:1009775025850
Schimel, J., Balser, T. C., and Wallenstein, M. (2007). Microbial stress-response physiology and its implications for ecosystem function. Ecology 88, 1386–1394. doi: 10.1890/06-0219
Silver, W. L., Lugo, A., and Keller, M. (1999). Soil oxygen availability and biogeochemistry along rainfall and topographic gradients in upland wet tropical forest soils. Biogeochemistry 44, 301–328. doi: 10.1007/BF00996995
Soong, J. L., Marañon-Jimenez, S., Cotrufo, M. F., Boeckx, P., Bodé, S., Guenet, B., et al. (2018). Soil microbial CNP and respiration responses to organic matter and nutrient additions: evidence from a tropical soil incubation. Soil Biol. Biochem. 122, 141–149. doi: 10.1016/j.soilbio.2018.04.011
Teh, Y., Diem, T., Jones, S., Huaraca Quispe, L. P., Baggs, E., Morley, N., et al. (2014). Methane and nitrous oxide fluxes across an elevation gradient in the tropical Peruvian Andes. Biogeosciences 11, 2325–2339. doi: 10.5194/bg-11-2325-2014
Teh, Y. A., Silver, W. L., and Conrad, M. E. (2005). Oxygen effects on methane production and oxidation in humid tropical forest soils. Global Change Biol. 11, 1283–1297. doi: 10.1111/j.1365-2486.2005.00983.x
Van Langenhove, L., Depaepe, T., Vicca, S., van den Berge, J., Stahl, C., Courtois, E., et al. (2019). Regulation of nitrogen fixation from free-living organisms in soil and leaf litter of two tropical forests of the Guiana shield. Plant Soil. doi: 10.1007/s11104-019-04012-1. [Epub ahead of print].
Wagner, F., Rossi, V., Stahl, C., Bonal, D., and Hérault, B. (2013). Asynchronism in leaf and wood production in tropical forests: a study combining satellite and ground-based measurements. Biogeosciences 10, 7307–7321. doi: 10.5194/bg-10-7307-2013
Wickham, H., Francois, R., Henry, L., and Müller, K. (2015). dplyr: A Grammar of Data Manipulation. R package version 0.43. Available online at: https://CRAN.R-project.org/package=dplyr
Wood, T. E., Detto, M., and Silver, W. L. (2013). Sensitivity of soil respiration to variability in soil moisture and temperature in a humid tropical forest. PLoS ONE 8:e80965. doi: 10.1371/journal.pone.0080965
Wood, T. E., and Silver, W. L. (2012). Strong spatial variability in trace gasdynamics following experimental drought in a humid tropical forest. Global Biogeochem. Cycles 26, 1–12. doi: 10.1029/2010GB004014
Wright, S. J. (2019). Plant responses to nutrient addition experiments conducted in tropical forests. Ecol. Monogr. 89:e01382. doi: 10.1002/ecm.1382
Wright, S. J., Turner, B. L., Yavitt, J. B., Harms, K. E., Kaspari, M., Tanner, E. V. J., et al. (2018). Plant responses to fertilization experiments in lowland, species-rich, tropical forests. Ecology 99, 1129–1138. doi: 10.1002/ecy.2193
Keywords: carbon dioxide, drought, fertilization, methane, nitrogen, phosphorus, soil GHG fluxes, tropical forest
Citation: Bréchet L, Courtois EA, Saint-Germain T, Janssens IA, Asensio D, Ramirez-Rojas I, Soong JL, Van Langenhove L, Verbruggen E and Stahl C (2019) Disentangling Drought and Nutrient Effects on Soil Carbon Dioxide and Methane Fluxes in a Tropical Forest. Front. Environ. Sci. 7:180. doi: 10.3389/fenvs.2019.00180
Received: 26 April 2019; Accepted: 28 October 2019;
Published: 13 November 2019.
Edited by:
Sasha C. Reed, United States Geological Survey, United StatesReviewed by:
Tessa Camenzind, Freie Universität Berlin, GermanyBenjamin W. Sullivan, University of Nevada, Reno, United States
Copyright © 2019 Bréchet, Courtois, Saint-Germain, Janssens, Asensio, Ramirez-Rojas, Soong, Van Langenhove, Verbruggen and Stahl. This is an open-access article distributed under the terms of the Creative Commons Attribution License (CC BY). The use, distribution or reproduction in other forums is permitted, provided the original author(s) and the copyright owner(s) are credited and that the original publication in this journal is cited, in accordance with accepted academic practice. No use, distribution or reproduction is permitted which does not comply with these terms.
*Correspondence: Laëtitia Bréchet, bGFldGkuYnJlY2hldEBnbWFpbC5jb20=
†These authors have contributed equally to this work