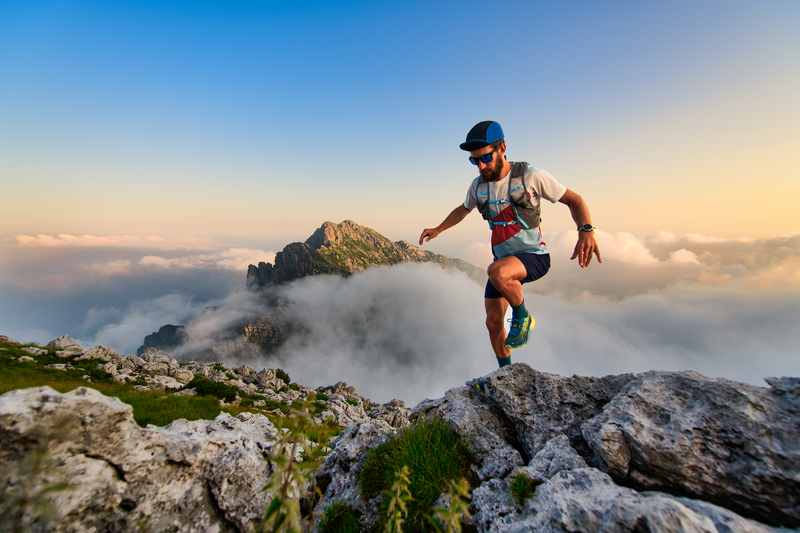
94% of researchers rate our articles as excellent or good
Learn more about the work of our research integrity team to safeguard the quality of each article we publish.
Find out more
OPINION article
Front. Environ. Sci. , 20 June 2019
Sec. Water and Wastewater Management
Volume 7 - 2019 | https://doi.org/10.3389/fenvs.2019.00095
Source control consists of strategies and programs to prevent the discharge of harmful substances, typically at the point or source of waste generation, into wastewater collection systems. Source control is not a new topic as some form of source control has been practiced well before it was mandated in the Clean Water Act (CWA) in 1972. Pretreatment programs for industrial discharges are mandated under sections 212 and 502(4) of the CWA for most, but not all, publicly owned treatment works (POTWs) that discharge to waters of the U.S. (e.g., surface waters). The principal objectives of the national pretreatment program are to prevent the introduction of pollutants into a POTW that:
• Interfere with treatment operations (liquid and solids)
• Pass through the treatment process and are discharged to receiving waters
• Limit opportunities to recycle and reclaim municipal and industrial wastewaters and biosolids
Based on these objectives, the primary focus of the national pretreatment program is on meeting discharge limits and non-potable reuse criteria. When considering potable reuse, however, where municipal wastewater effluent is used as a source of potable water supply, conventional source control strategies are not sufficient as the focus of source control for potable reuse must involve an integrated approach to the development of a water supply program. The objectives of this paper are to examine the limits of conventional source control programs, to suggest a more encompassing definition of source control for potable reuse, and to discuss measures that can be taken to enhance the performance of wastewater treatment facilities (WWTFs) in a more encompassing definition of source control.
Source control programs have evolved since the passage of the CWA. Most conventional source control programs are focused typically on the contributing service area to a treatment facility (see Figure 1). As interest in potable reuse has increased, enhanced source control programs have been developed that include a greater range of compounds, such as precursor compounds, and other specific constituents that may have health implications or are difficult to treat in advanced water treatment (AWT) facilities (Rimer et al., 2017a,b,c). Technological developments, such as sensor networks for wastewater collection system monitoring, have also made it possible to implement more stringent source control measures (SWAN, 2017). The key elements of an enhanced source control program for potable reuse are identified in Tchobanoglous et al. (2015). Two excellent operational examples of enhanced source control programs for potable reuse include those developed by the Orange County Sanitation District (OCSD, 2018) and The City of San Diego (SDPUD, 2018).
Figure 1. Definition sketch for the boundaries of conventional and enhanced source control programs for effluent dispersal and reuse, and a comprehensive source control program for potable reuse (Adapted from Tchobanoglous et al., 2014).
While source control measures can be used to control concentrated sources, some constituents found in domestic wastewater at trace levels, such as nanoparticles and organic constituents, cannot be managed effectively using source control. The U.S. EPA refers to these groups or classes of compounds, typically categorized by consumer end use, as contaminants of emerging concern (CEC) (U.S. EPA, 2019). The end use categories of CECs include household chemicals, pharmaceuticals, food additives, plasticizers, personal care products, and flame retardants. Given the challenges in controlling the formulation and limiting the use of CECs in consumer products, they must be removed or reduced in concentration using biological and/or advanced treatment processes.
The purpose here is to suggest that to optimize the effluent quality for AWT, a new definition of source control is needed. Comprehensive source control which encompasses conventional and enhanced source control but also includes the entire WWTF, as shown on Figure 1, is suggested. It has been demonstrated repeatedly that enhancements to the WWTF can have significant beneficial effects on the performance of advanced water treatment facilities (AWTFs). Factors that affect the performance of WWTFs and measures that can be taken to enhance their performance are considered in the following two sections.
Important design and operational factors known to impact the performance of WWTF are reported in Table 1. In what follows, each of these factors is examined with respect to its identified impact on wastewater treatment. The impact each of the factors will have on existing WWTF effluent quality will vary, depending on the characteristics of the wastewater, and the design and operation of existing facilities. The reason it is important to consider these factors is that when planning for potable reuse, the objective of wastewater treatment should be to produce the highest quality effluent possible, consistent with operational and economic constraints.
Table 1. Factors, measures, and operational strategies that must be considered in optimizing the performance of wastewater treatment facilities producing an effluent to be treated further in an advanced water treatment facility for potable reusea.
Since the early 1990s when conservation measures began to be implemented in earnest in the United States, indoor water use has decreased from 240 to 265 L/capita·d (65 to 70 gal/capita·d) to less than 132 L/capita·d (35 gal/capita·d) in some cities (Tchobanoglous et al., 2014). Perhaps the most dramatic change has been the wastewater flowrates to the City of Los Angeles Hyperion Wastewater Treatment Plant, where the average daily flow has deceased from a flow rate of about 1552 million liter per day (ML/d) [410 million gallon/day (Mgal/d)] in 2004 to a flowrate of 1233 ML/d (326 Mgal/d) in 2014.
The observed decrease in indoor water use has a number of consequences, including (1) reduced quantities of wastewater available for reuse after suitable processing; (2) the deposition of solids in stagnant or low velocity zones in collection sewers which undergo anaerobic decomposition with the concomitant release of hydrogen sulfide, leading to increased corrosion in downstream concrete sewers; (3) the development of anaerobic conditions leads to changes in the characteristics of the wastewater to be treated; (4) low flow conditions which exacerbate the flow rate variations observed at WWTFs, especially in smaller plants; and (5) wastewater constituent concentrations have increased, although the mass of constituents [expressed in kilograms per day (kg/d)] that must be treated has remained the same or has increased consistent with population growth. On a positive note, reduced wastewater flow rates make flow equalization, as discussed subsequently, more feasible, especially during dry weather periods. The consequences of water conservation are addressed further in the following discussion.
As a result of climate change, areas that are wet are getting wetter while areas that are dry are getting dryer. But of far greater consequence is the intensity of the rainfall when it occurs. Unfortunately, most WWTFs are designed like bathtubs - water in equals water out. In numerous WWTFs, the biological process has been washed out due to the very rapid increase in wastewater flowrates resulting from sudden intense rainfall events. In combined wastewater collection systems (i.e., collection systems used to transport both wastewater and stormwater) increased flowrates occur primarily as a result of direct inflow from street and other drains. In separated wastewater collection systems increased flowrates occur primarily as a result of inflow from illicit drains, inflow from surface access ports, and infiltration due to high ground water levels. In some cases, peak flowrates have exceeded the average flowrate by a factor of five or more in less than 30 min. Clearly, WWTFs were never designed to deal with such flowrate fluctuations. In almost all cases, effective treatment of wastewater that is to serve as an influent to an AWTF is reduced (Wilén et al., 2006; Plósz et al., 2009). With the new reality of intense rainfall events, new operating strategies, including flow equalization, will have to be developed to deal with the excess flows in WWTFs which are subject to rapid increases in wastewater flowrates.
Preliminary treatment refers generally to the removal of oils, fats, waxes, fatty acids, and grease (referred to as O/G) and grit following coarse screening of raw domestic wastewater. The term fats, oils, and grease (FOG), used previously, has been replaced by O/G. As a result of water conservation, the concentration of O/G in wastewater has also increased, along with other constituent concentrations. Higher O/G concentrations are of concern in both collection systems and within WWTFs.
In wastewater collection systems, higher concentrations of O/G have led to: (1) blockages due to accumulations at irregular points within the collection system, (2) formation of hydrogen sulfide in O/G accumulations, where the water supply contains higher concentrations of sulfate, and (3) the enhanced coating of grit particles, reducing the effective specific gravity and the corresponding settling rates of the grit.
In wastewater treatment plants, the principal problems with O/G, if not removed, are that it is slowly biodegradable, can reduce bioactivity by coating biological forms and interfering with oxygen transfer, can lead to formation of filamentous microorganisms, and can cause foaming (Tseng, 1979; Nelson and Lauer, 1994; Serrano and Salgado, 2016). The presence of O/G can also lead to the formation of grease balls which can accumulate on the surface of the activated sludge process and, if present in the treated effluent, can lead to the formation of grease and mudballs in granular media filters (Tchobanoglous et al., 2014). Another concern with increased O/G concentrations is that a much higher percentage of the total O/G derived from oils now used commonly, including canola, corn, olive, vegetable oils, and other cooking oils, is soluble at ambient wastewater temperatures. When the soluble concentrations of O/G were lower, surface skimming in primary sedimentation tanks was adequate to control the O/G entering biological treatment reactors. With the mixing that occurs in collection systems, especially with low flows, many of the soluble oils become emulsified, which further complicates their removal.
The issue with O/G coated grit particles is that they are not removed effectively with conventional grit removal facilities now in use at most WWTFs. Specific gravities of O/G coated grit particles are now on the order of 1.3–1.4, as opposed to a value of 2.65 used in most textbooks and for the conventional design of grit removal facilities. Because of their low specific gravity, grit particles are carried over into the biological treatment facilities. As the O/G surrounding grit particles is reduced through microbial degradation, the specific gravity of the grit increases, and grit accumulations can occur in the aeration reactor. Recognizing that the specific gravity of grit in wastewater is not 2.65, new grit removal technologies have been developed. Perhaps the most effective being the multi-tray vortex grit separator (Wilson et al., 2007; Tchobanoglous et al., 2014). In any case, if the O/G concentration in the wastewater is high, every effort should be made to remove it, along with grit coated with O/G, to enhance the performance of the biological treatment process.
Typical flowrate, biochemical oxygen demand (BOD) concentrations, and BOD mass loadings as measured at a WWTF following coarse screening and grit removal are shown on Figure 2A. Under normal dry-weather conditions, peak influent flowrates and BOD and total suspended solids (TSS) concentrations can reach two or more times the average values. Similarly, low flowrates and BOD and TSS concentrations can be two or more times less than the average values. In general, the observed variations are more pronounced in small wastewater systems where storage capacity in the collection system does not provide damping. In these systems, as a result of water conservation and exfiltration, the flowrates in the early morning hours may approach zero. Without flow equalization, these variations in flowrate and constituent concentrations have been shown to deteriorate plant performance, especially in plants with limited (or excessive) volumetric capacity. Reduced WWTF performance is characterized by greater variability in the constituent concentrations in the treated effluent and reduced removal of trace organic constituents (Gujer and Erni, 1978; Niku et al., 1981a,b; Plósz et al., 2009). As wastewater flowrates are reduced and excess treatment tankage becomes available, the tankage could be repurposed for flow equalization during dry weather periods; however, WWTFs must still develop a strategy for dealing with the excessive flow resulting from sudden peak rainfall events.
Figure 2. Typical hourly variations observed in flow, BOD concentrations, and BOD mass-loadings in influent after screening and grit removal (A) unequalized, measured values and (B) flow equalized values based on the data in (A). The required flow equalization volume is 4.1 ML (1.1 Mgal) (adapted from Tchobanoglous et al., 2014).
Two types of flow equalization can be used: in-line and off-line storage. The choice of equalization type depends on local conditions and the amount of flow and load equalization required (Tchobanoglous et al., 2014). The corresponding values obtained when the flowrate given in Figure 2A is equalized using in-line storage are shown on Figure 2B. As shown on Figure 2B, the peak BOD concentration and mass loading are reduced from 300 to 240 mg/L and 440 to about 280 kg/h, respectively. In this case, the required in-line storage volume to equalize the flowrate was 4.1 ML (1.1 Mgal). In many WWTFs, the required volumetric storage capacity for full-flow equalization may not be available. With less than full-flow equalization, greater variation in constituent mass loadings will occur, unless flow is diverted to an off-line storage facility based on influent constituent concentrations. The principal benefits of flow equalization in biological wastewater treatment include the reduction or elimination of shock loadings, dilution of potentially toxic constituents, and load equalization, all of which are important in maintaining the stability of the process including the secondary settling facilities (Foess et al., 1977; Gujer and Erni, 1978; Niku et al., 1981a,b; Giokas et al., 2002; Tchobanoglous et al., 2014). Flow equalization facilities can also be used to equalize return flows, as discussed below.
Flow equalization is equally important in the operation of AWTFs. To overcome the operational difficulties resulting from daily effluent flowrate variations from the Orange County Sanitation District (OCSD), including stress cracks in microfiltration membrane manifolds, the Orange County Water District incorporated 57 ML (15 Mgal) of flow equalization storage in front of the AWTF in the 2017-2018 expansion of the Groundwater Replenishment System AWTF (OCWD, 2017). Operationally, it would have been more beneficial to install flow equalization at the OCSD WWTF, but it was not possible due to space limitations, which is often the situation with older treatment plant sites.
A number of new filtration technologies are available that can be used to provide enhanced primary treatment (i.e., reduced BOD, TSS, and particle size). Two of the most effective approaches are, as illustrated in Figure 3, primary effluent filtration (PEF) and primary filtration (PF). In PEF, effluent from primary sedimentation is filtered before being applied to the biological treatment process (Caliskaner et al., 2016). In PF, a cloth disk filter contained in a small reactor is used to replace conventional primary sedimentation (Caliskaner et al., 2018, 2019). Before discussing these technologies, and presenting some performance data, it is important to comment on why both of these technologies work. The filtration processes work because only marginally neutrally buoyant suspended particles are being filtered (see Figure 3). In both cases floatable and readily settleable material are removed separately and not passed through the filter, thus avoiding conventional grease and solids loading problems which have plagued other direct filtration systems. Another benefit of either PEF or PF filtration systems is the removal of emulsified oils, due to the small nominal pore size of the filter mediums.
Figure 3. Definition sketch for (A) primary effluent filtration (PEF) and (B) primary filtration (PF) with cloth disc filter. In both cases, floatable material and heavier settleable solids are removed separately.
As PEF has been discussed extensively in the literature (Matsumoto and Tchobanoglous, 1982; Matsumoto et al., 1982; ERM Inc., 1986; Brown, 1987; Jimenez et al., 1999), the following remarks are focused on PF with the cloth disk filter. Comparative data are presented following the primary filter discussion.
The cloth disk filter employees a cloth pile filter material with a total depth in the filtering mode of 10 mm. The nominal pore size of the filter medium is 5 μm. The impact of replacing primary sedimentation facilities with PF is illustrated on Figure 4. As shown, the mass loadings to the biological treatment process for both TSS and BOD are reduced significantly. The difference in TSS and BOD mass loadings between primary sedimentation and PF effluent is significant. For TSS, the difference between the two means is 185 kg/d (260–75 kg/d). For BOD the difference between the two means is 180 kg/d (380–200 kg/d). If full or partial flow equalization is employed, the difference in BOD and TSS loadings would be more striking.
Figure 4. Average daily mass loadings from a primary clarifier compared to primary filtration (A) TSS and (B) BOD [Adapted from Caliskaner et al. (2019)].
Other process benefits from using the cloth disc filter for PF are (1) with a nominal filter cloth pore size of 5 μm, the effluent wastewater particle size distribution is altered and the variability is reduced, as compared to the particles found in settled primary effluent; (2) the filter effluent is relatively immune to influent spikes in TSS; (3) emulsified O/G, as noted previously, is removed effectively, and (4) the disc filter only occupies one fifth of the space needed for a conventional primary sedimentation process. The energy and economic benefits of PF are reported in Caliskaner et al. (2019).
Typical TSS performance data for both PEF and PF are presented in Table 2. As shown, different amounts of TSS are removed by sedimentation and filtration in the two processes. However, what is most significant is that the overall removal efficiencies are essentially the same for both processes.
Table 2. TSS removal performance using cloth disk filter for primary effluent and primary filtration.
Given the many advantages of filtration using modern technologies, it seems that PEF should be considered in plants with existing clarifiers, while PF may be applicable to new designs or existing plants that either do not have primary clarifiers or are considering replacement of clarifiers.
In wastewater treatment plants, return flows are generated from sludge thickeners, sludge stabilization (e.g., aerobic digestion, anaerobic digestion, and lagoon supernatant), sludge dewatering, sludge drying, and effluent filtration. Typically, return flows are returned as-produced to the head end of the WWTFs or introduced directly to the secondary process for treatment, increasing the organic, nutrient, and colloidal solids loading to the primary and secondary treatment processes, and, in turn, deteriorating treatment performance.
Return flows from small plants are typically from thickening of primary and secondary waste sludges before aerobic digestion or lagoon stabilization, backwash water from effluent filtration, and supernatant flows from aerobic digestion and or stabilization lagoons. Methods for dealing with the return flow from small WWTFs are essentially the same as those from large plants, as discussed below, but are seldom implemented as potable reuse from small WWTFs is generally not economical due to the restrictive nature of regulations, the cost of the source control program, the cost of the required compliance sampling program, the expensive instrumentation, and the need for highly qualified operators.
Return flows from effluent filtration and solids processing facilities in larger plants results in the generation of multiple recycle streams each with a different composition, flowrate, and potential impact on the treatment plant (Gujer and Erni, 1978; Tchobanoglous et al., 2014; Rimer et al., 2017b). Both anaerobic and aerobic digestion result in the release of humic and fulvic acids, soluble organic nitrogen-containing compounds, ammonium, and ortho-phosphate into the bulk liquid. The presence of Mannich polymers, found in recycle streams from sludge thickening and dewatering operations, are especially troublesome as they have been implicated in the formation of N-nitrosodimethylamine (NDMA), at AWTFs. Similarly, some of the polymers used to improve effluent filter performance, which are present in filter backwash water can also be problematic. In many plants these return flows occur during the daytime hours, further taxing the capacity of the treatment facilities. Every effort should be made to reduce or eliminate the impacts of return sidestreams.
Two important ways to mitigate the effects of return flows is through flow equalization and treatment before reintroducing them into the treatment process. As noted previously, flowrates observed at WWTFs are declining, resulting in the availability of excess treatment capacity which can be used for flow equalization of return flows. Flow equalized return flows can be applied to the treatment process when excess treatment capacity is available in the evening and early morning hours. Depending on the nature of the constituents in the sidestream, it may be necessary to reduce TSS and colloidal matter. For potable reuse and where stringent discharge requirements must be met, if may be necessary to remove nutrients and residual organic matter. Because many dewatering operations are conducted as batch processes it may also be necessary to flow equalize the return sidestream before treatment. Sidestream treatment of one form or another has been conducted at the Washington D.C. Blue Plains Advanced Wastewater Treatment Plant since 2011 (Figdore et al., 2010, 2011). Where satellite treatment plants are used, as discussed in the following section, all return flow sidestreams are discharged to the collection system for treatment at a downstream regional WWTF. Additional details on sidestream constituent concentrations and treatment options and processes may be found in Tchobanoglous et al. (2014).
The benefits of effective preliminary treatment, flow equalization, enhanced primary treatment, and the flow equalization and or treatment of recycle sidestreams have already been discussed. There are, however, a number of operational and process changes that can be made to improve the operation of the biological treatment process, which, in turn, improve the performance of AWTFs. Ultimately, what is important is to optimize the wastewater treatment process with respect to the performance requirements of the AWTF. Although the list of potential modifications is almost endless, four operational changes are highlighted below.
With increasing constituent concentrations, the need to process return flows during the daytime hours, and where it is not possible to flow equalize, it may be necessary to modify the treatment process flow configuration and aeration system to avoid a deterioration in the quality of the effluent. In some plug-flow activated sludge configurations, it may not be possible to provide sufficient oxygen at the front end of the treatment process. To overcome aeration system limitations, plug flow reactors have been reconfigured to operate in a step-feed mode, in which wastewater enters the biological reactor at several points along its length thus reducing the oxygen demand (Buhr et al., 1984; Bhattarai, 2015). The benefits of the step feed mode of operation include operational flexibility, ability to optimize the use of existing facilities, improved handling of peak flows, and stable operation (Bhattarai, 2015). The addition of pure oxygen, using a Speece Cone (ECO Oxygen Technologies, LLC, Indianapolis, IN), to supplement the oxygen resources of both plug-flow and complete-mix biological reactors is also feasible. In most applications, pure oxygen would be added to a small side stream until it is supersaturated with oxygen and then returned to the process where it is mixed with the main flow (Barreto et al., 2018).
One of the most significant modifications that can be made to a biological treatment process is changing the operation to a nitrification or nitrification/denitrification mode. The impact of operation in a nitrification mode at the upstream WWTF on microfiltration transmembrane pressure at an ATWF is illustrated on Figure 5. As shown when the biological treatment process was converted to a nitrification process, the variability of the transmembrane microfiltration pressure became less erratic, the operating pressure was reduced, the degree of fouling was reduced, and run time between cleanings was increased, all of critical importance with respect to the operation of the AWTF (Tchobanoglous et al., 2015). In switching to a nitrification or a nitrification/denitrification (NdN) mode it is important to note that diurnal variations in flow and ammonia loading as well as other process variables can have a significant impact on the performance of a nitrifying activated sludge process (Gujer and Erni, 1978). A variety of different nitrogen removal process configurations are available, depending on the design of the existing treatment facilities (Tchobanoglous et al., 2014). The specific NdN process employed will depend on the degree of nitrogen removal required for the AWTF.
Figure 5. Reduced membrane operating pressures observed at the Groundwater Replenishment System operated by the Orange County Water District after the Orange County Sanitation District implemented biological nitrification in March 2010. Source: Graphic courtesy of OCWD.
Most activated sludge processes are operated and controlled based on the solids retention time (SRT), the food to microorganism ratio (F/M), or the organic volumetric loading rate. Of these control methods, SRT is used most commonly. To save energy, some plants are operated at low SRT values (e.g., 0.75–1 day). Operation at such low SRT values will not allow nitrification to occur and the sludge is difficult to settle. While most conventional plants are operated with SRT values in the range from 5 to 8 days, this operating range may not be optimal with respect to potable reuse. The benefits for operating at a longer SRT to improve the microbial environment, improve process stability, improve nutrient removal, and to enhance the degradation of CECs has been demonstrated and reported in the literature (Greenwood et al., 2002; Chan et al., 2011; Leu et al., 2012; Tchobanoglous et al., 2014). With respect to CECs and nanoparticles, numerous studies have been conducted on their occurrence and removal in existing WWTFs (Clara et al., 2005; Oulton et al., 2010; Leu et al., 2012; Margot et al., 2015) and considerable research is ongoing to find methods of optimizing their removal during biological treatment (Fatta-Kassinos et al., 2016; Ahuja, 2019). However more work is needed to optimize their removal and to balance what should be treated in the WWTF and what should be treated in the AWTF. What is important is that the operation of the biological treatment process should be coordinated with the operation of the AWTF.
Bioaugmentation involves the addition of specialized bacterial strains to the activated sludge process to enhance the overall performance and/or for the removal of specific constituents in the wastewater. There are two general forms of bioaugmentation. In the first form, microorganisms, cultured in separate specialized facilities, are added to the wastewater to be treated (Limbergen and Verstraete, 1998). In the second form, another smaller separate bioreactor is used to grow cells especially selected or engineered to degrade specific compounds. (Cardinal and Stenstrom, 1991; Babcock et al., 1992; Figdore et al., 2018). Sidestream granular activated sludge to enhance nitrification and prompt phosphorus removal is another approach that can be used to produce a high-quality effluent (Figdore et al., 2018). The effective removal of specific constituents through bioaugmentation has been demonstrated repeatedly (Cardinal and Stenstrom, 1991; Babcock et al., 1992; Nzila et al., 2016). When bioaugmentation was introduced in the early 1990s there was an initial interest, but no compelling reason to adopt bioaugmentation. Today, with growing water scarcity and the need to use and recycle water, anything that can be done to enhance wastewater treatment including bioaugmentation should be considered carefully.
In some situations, it may not be cost-effective to upgrade an existing biological treatment process and that switching treatment processes may warranted. At the present time, numerous options are available. Three examples are offered. One of the most common options involves conversion of a conventional plug-flow activated sludge process to staged activated sludge process capable of removing nitrogen and phosphorus (Tchobanoglous et al., 2014). Where excess tankage capacity is available, such a conversion can be accomplished readily. The benefits with respect to effluent quality are well documented.
Another conversion, currently in favor, is to replace an existing activated sludge process and effluent filtration, where used, with a membrane bioreactor (MBR) process. In a recent study it was reported that switching to an MBR resulted in improved removal of solids, nutrients, and micropollutants (Kitanou et al., 2018). In considering a switch to an MBR process, it is important to consider the entire plant and not just the MBR process. For example, for optimal performance, the flowrate variation to the MBR process should not be excessive, with flow equalization recommended if optimal effluent quality is to be achieved.
A third option is to convert an existing activated sludge process to a granular activated sludge process, a relatively new process which is carried out in a single tank. Operationally, the objective is to select microorganisms that will form granules of microorganisms instead of the flocculant microbial suspensions, common in conventional activate sludge. The process involves three steps (1) simultaneous fill (from the bottom) and draw (from the surface) without aeration, (2) react in which the influent is stopped, and air is introduced intermittently, and (3) settle where the granules are separated from the liquid. The process is simple to operate as there are no moving parts. The principal advantages are excellent removals of TSS, BOD, nitrogen, phosphorus, and other constituents; smaller footprint; secondary clarifiers not required; and energy savings due to reduced aeration requirements (de Kreuk and Loosdrecht, 2006; Figdore et al., 2017, 2018; Pronk et al., 2017).
Wastewater treatment processes can be operated under manual or automatic control. In general, it has been found that treatment process performance can be improved under automatic control. In larger plants, automatic process control is used to manage process parameters such as SRT, dissolved oxygen, and clarifier sludge depth. The increased use of process control sensors and instrumentation will allow for the expanded use of cloud based data processing, real time process monitoring, and automated process operations for improved performance.
Effluent filtration is used to improve the quality of the effluent from WWTFs by reducing the residual TSS concentration and the TSS variability. Some BOD, phosphorus, and microorganism removal is also accomplished, depending on the type and operation of the biological treatment process (Zanetti et al., 2006; Asano et al., 2007; Tchobanoglous et al., 2014, 2015) Another significant benefit of effluent filtration is that more effective and consistent disinfection can be achieved, regardless of the disinfectant employed (e.g., chlorine, ozone, or UV irradiation). Effluent filtration is required for most reuse applications (Asano et al., 2007). In general, removal of residual effluent TSS has been shown to improve the performance and stability of AWTFs and is included in most potable reuse treatment process flow diagrams (Asano et al., 2007; Rimer et al., 2017c). For example, in AWTFs that use ozone as a pretreatment step, effluent filtration is important in reducing the residual organics in TSS that exert an ozone demand. Similarly, residual effluent TSS can reduce the run time of microfiltration or ultrafiltration membranes, resulting in more frequent backwash intervals, reducing the amount of water that can be captured (Citulski et al., 2006). However, it should be noted that in some situations, depending on the design and operating characteristics of the WWTF and the AWTF, effluent filtration may not be necessary or feasible. If effluent filtration is employed, great care should be taken in the selection of the type of organic polymer, if used, to avoid the downstream formation of NDMA and other constituents of health concern.
Historically, some form of chlorine disinfection has been used most commonly for effluent dispersal to the environment. In some cases, two-stage disinfection with free and combined chlorine has been adopted to reduce the formation of disinfection byproducts (Hua et al., 2012; LACSD, 2013). To reduce the formations of potentially toxic disinfection byproducts, UV disinfection has been adopted in many locations (Palen, 2015). The need for effluent disinfection and the disinfectant to be used will depend on the operational requirements of the technologies employed in the AWTF.
Where implementation of the modifications to treatment processes discussed above are not feasible or cost effective, some alternative treatment configurations may be attractive. Two of these are (1) the use of divided treatment trains and (2) the use of satellite wastewater treatment facilities. Each of these options is considered in the following discussion.
At regional facilities, where excess capacity is available as a result of water conservation or over-design, the plant treatment train may be divided such that one part of the plant can be isolated for operation at constant loading prior to the AWTF (see Figure 6). Because primary facilities are often difficult to isolate, PF with a cloth disk filter could be used for the isolated portion of the treatment plant. The freed up primary sedimentation capacity could be used for flow equalization or sidestream flow equalization and treatment, thus optimizing the performance of the non-flow equalized portion of the plant. In some locations where the treatment plant facilities have been expanded, such as the City of San Jose in California, the expanded portion of the plant is operated at a more-or-less constant flowrate.
Figure 6. Definition sketch for divided treatment trains in an existing or new WWTF to optimize effluent quality for advanced water treatment for potable reuse and for dispersal in the environment.
Historically, centralized wastewater collection systems are arranged to route wastewater to remote locations for treatment near a discharge location. As a result, both non-potable and potable reuse in urban areas is often inhibited by infrastructure costs for transporting and storing reclaimed water at or near the points of use (i.e., typically far from treatment plant location). Among the most feasible alternatives to centralized wastewater treatment to achieve increased water reuse is an integrated wastewater management strategy employing satellite wastewater treatment facilities (SWWTFs), where wastewater treatment can be optimized. Satellite WWTFs are located typically within the sewer service area (i.e., sewershed), at or near the point of waste generation or potential reuse applications (Asano et al., 2007; Tchobanoglous, 2018). Satellite WWTFs, as illustrated on Figure 7, can take a variety of forms, including: (1) treatment facilities for subdivisions, portions of a community, or an entire community in a regional system; (2) extraction type treatment facilities where varying amounts of wastewater are extracted from a wastewater collection system, treated, and used for specific local applications; and (3) interception type treatment facilities, used commonly for in-building recycling, where the wastewater to be treated and reused is intercepted before reaching a collection system (Asano et al., 2007).
Figure 7. Schematic view of an integrated wastewater management system employing satellite wastewater treatment facilities for local and potable reuse applications with residuals processing at a centralized WWTF [adapted from Gikas and Tchobanoglous (2009)].
An important and unique feature of SWWTFs, as shown on Figure 7, is the fact that they are all connected to the centralized wastewater collection system, where excess wastewater and the solids resulting from treatment are processed. Without the need to treat return flows, and the fact that SWWTFs can be operated at a constant flowrate, their performance can be optimized, producing a higher quality effluent with less variability for both non-potable and potable reuse applications.
The use of upstream satellite WWTFs is a well-established practice in California in the City of Los Angeles, the County Sanitation Districts of Los Angeles County (CSDLAC), and the City of San Diego. The Donald C. Tillman Water Reclamation Plant in the City of Los Angeles, put into operation in 1985, is used to irrigate a world famous 2.6-hectare Japanese garden, to fill the 1.11-hectare lake located within the garden, and to maintain flow in the Los Angeles River (City of Los Angeles, 2018). Groundwater replenishment with imported river water, local stormwater runoff, and reclaimed water from one of the satellite plants has been used by CSDLAC since 1962 (Sanitation Districts of Los Angeles County, 2019). In San Diego, The North City Reclamation Plant (NCRP) in San Diego, also a satellite plant, was built to enhance local reuse of the treated effluent, primarily golf courses (City of San Diego, 2018). In all cases, the effluent from these plants, operated at a constant flowrate without any return sidestreams, is significantly better, as measured by constituent concentrations and reduced effluent variability, than that of similarly sized plants operated as conventional WWTFs.
Where treated wastewater effluent is to be treated further for potable reuse, the objective of wastewater treatment should be to produce the highest quality effluent possible on a continuous basis. Source control, focused on the service area, is of critical importance in achieving a high level of effluent water quality where the treated effluent is to be dispersed into the environment or used for non-potable reuse applications. Enhanced source control for potable reuse, also focused on the service area, expands conventional programs to include additional compounds that are not processed effectively in AWTFs. The proposition put forth in this paper is that to optimize the performance of WWTFs, source control for potable reuse should not be limited to the service area, but should encompass the design and operation of the wastewater treatment facilities and is referred to as comprehensive source control. Based on past experience, there are a number of proven measures and strategies to improve and optimize WWTF performance that should be considered when planning for potable reuse. The implementation of comprehensive source control is expected to produce the consistent, high quality effluent needed for the optimal performance of AWTFs, making potable reuse safer and a feasible option for more communities.
All authors listed have made a substantial, direct and intellectual contribution to the work, and approved it for publication.
The authors declare that the research was conducted in the absence of any commercial or financial relationships that could be construed as a potential conflict of interest.
Ahuja, S. (2019). Advances in Water Purificatιon Techniques: Meeting the Needs of Developed and Developing Countries. Cambridge, MA: Elsevier.
Asano, T., Burton, F. L, Leverenz, H., Tsuchihashi, R., and Tchobanoglous, G. (2007). Water Reuse: Issues, Technologies, and Applications. New York, NY: McGraw-Hill Book Company.
Babcock, R. W., Ro, K. S. Jr., Hsieh, C., and Stenstrom, M. K. (1992). Development of an off-line enricher-reactor process for activated sludge degradation of hazardous wastes. Water Environ Res. 64, 782–790.
Barreto, C. M., Ochoa, I. M., Garcia, H. A., Hooijmans, C. M., Livingston, D., Herrera, A., et al. (2018). Sidestream superoxygenation for wastewater treatment: oxygen transfer in clean water and mixed liquor. J. Environ. Manage 219, 125–137. doi: 10.1016/j.jenνman.2018.04.035
Bhattarai, R. P. (2015). “Lessons from austin's full-scale step-feed BNR demonstration,” in Presented at the Fifth Annual W. Wesley Eckenfelder Lecture Design into Practice: Leveraging Best Design Applications (Arlington, TX: The University of Texas at Arlington).
Brown, D. S. (1987). Evaluation of a pulsed bed filter for filtration of municipal primary effluent. J. WPCF. 59, 72–78.
Buhr, H. O., Goddard, M. F., Wilson, T. E., and Ambrose, W. A. (1984). Making full use of step feed capability. J. WPCF. 56, 325–330.
Caliskaner, O., Tchobanoglous, G., Reid, T., Young, R., Downey, M., and Kunzmans, B. (2016). “Advanced primary treatment via filtration to increase energy savings and plant capacity,” in Proceedings of the 2016 Water Environment Federation Technical Exhibition and Conference (New Orleans, LA).
Caliskaner, O., Tchobanoglous, G., Wu, Z., Davis, B., Reid, T., and Dyson, J. (2018). Performance Evaluation of First Full Scale and Demonstration Primary Filtration Projects. Washington, DC: Water Environment Federation.
Caliskaner, O., Wu, Z., and Lund, J. (2019). Raw Wastewater Filtration to Reduce Secondary Treatment Electrical Energy Demand: Draft Final Project Report. Report prepared for the Energy Research and Development Division, California Energy Commission, Kennedy Jenks Consultants, Rancho Cordova, CA.
Cardinal, L. J., and Stenstrom, M. K. (1991). Enhanced biodegradation of polyaromatic hydrocarbons in the activated sludge process. Res. J. Water Pollut. Control Feder. 63, 950–957. Available online at: www.jstor.org/stable/i25044086
Chan, L., Leu, S. Y., Rosso, D., and Stenstrom, M. K. (2011). The relationship between mixed-liquor particle size and solids retention time in the activated sludge process. Water Environ Res. 83, 2178–2186.
Citulski, J. A., Farahbakhsh, K., and Kent, F. C. (2006). Effects of total suspended solids loading on short-term fouling in the treatment of secondary effluent by an immersed ultrafiltration pilot system. Water Environ Res. 81, 2427–2436.
City of Los Angeles (2018). Donald C. Tillman Water Reclamation Plant. Available online at: https://eng.lacity.org/dc_water_reclamation (cited October 9, 2018).
City of San Diego (2018). Water and Wastewater Facilities. Available online at: https://www.sandiego.gov/mwwd/facilities/northcity (cited May 6, 2019).
Clara, M., Strenn, B., Gans, O., Martinez, E., Kreuzinger, N., and Kroiss, H. (2005). Removal of selected pharmaceuticals, fragrances and endocrine disruptíng compounds in a membrane bíoreactor and conventional wastewater treatment plants. Water Res. 39, 4797–5807. doi: 10.1016/j.watres.2005.09.015
de Kreuk, M., and Loosdrecht, M. V. (2006). Formation of aerobic granules with domestic sewage. J. Environ. Eng. 132, 694–697. doi: 10.1061/(ASCE)0733-9372(2006)132:6(694)
ERM Inc. (1986). Primary Effluent Filtration With A Pulsed Bed Filter: Technical Note. Prepared for: U. S. Environmental Protection Agency, Office of Municipal Pollution Control, Municipal Facilities Division. Washington, DC: ERM Inc.
Fatta-Kassinos, D., Dionysiou, D. D., and Kümmerer, K. (2016). Advanced Treatment Technologies for Urban Wastewater Reuse. Cham; Heidelberg: Springer.
Figdore, B., Bowden, G., Bodniewicz, B., Bailey, W., Derminassian, R., Kharkar, S., et al. (2010). Impact of Thermal Hydrolysis Solids Pretreatment on Sidestream Treatment Process Selection at the DCWASA Blue Plains AWTP. WEFTEC 2010 October 2010, New Orleans, LA.
Figdore, B., Bowden, G., Stinson, B., Wett, B., Hell, M., Bailey, W., et al. (2011). “Treatment of dewatering sidestream from a thermal hydrolysis-mesophilic anaerobic digestion process with a single-sludge deammonification process,” in Proceedings of the Water Environment Federation (Alexandria, VA). doi: 10.2175/193864711802639192
Figdore, B. A., Stensel, H. D., and Winkler, M. H. (2017). Comparison of different aerobic granular sludge types for activated sludge nitrification bioaugmentation potential. Bioresour. Technol. 251, 189–196. doi: 10.1016/j.biortech.2017.11.004
Figdore, B. A., Stensel, H. D., and Winkler, M. H. (2018). Bioaugmentation of sidestream nitrifying-denitrifying phosphorus-accumulating granules in a low-SRT activated sludge system at low temperature. Water Res. 135, 241–250. doi: 10.1016/j.watres.2018.02.035
Foess, G. W., Meenahan, J. G., and Blough, D. (1977). Evaluation of in-line and side-line flow equalization systems. J. WPCF 49, 120–130.
Gikas, P., and Tchobanoglous, G. (2009). The role of satellite and decentralized strategies in water resources management. J. Environ. Manage. 90, 144–152. doi: 10.1016/j.jenvman.2007.08.016
Giokas, D., Vlessidis, A., Angelídis, M., Tsímarakis, G., and Karayannis, M. (2002). Systematic analysis of the operational response of activated sludge process to variable wastewater flows. α case study. Clean Technol. Envir. 4, 183–190. doi: 10.1007/s10098-002-0145-z
Greenwood, S., Anderson, C., Rieth, M., and Stein, T. (2002). A Constant SRT Calculated from Liquid Flows Improves BNR Activated Sludge Performance. Metropolitan Council, St. Paul, MN. Available online at: http://web.deu.edu.tr/atiksu/ana58/bionutre.pdf
Gujer, W., and Erni, P. (1978). The effect of diurnal ammonium load variation on the performance of nitrifying activated sludge processes. Prog. Wat. Tech. 10, 391–407.
Hua, G. B., Davis, P., Cunningham, A., Yeats, S., and Sealey, K. (2012). Sequential chlorination to control disinfection byproducts and meet stringent disinfection requirements at the κanapaha water reclamation facility. Florida Water Resour J. 64, 46–51.
Jimenez, B., Chavez, A., Leyva, A., and Tchobanoglous, G. (1999). Sand and synthetic medium filtration of primary treatment effluent from mexico city. Water Res. 34, 473–480.
Kitanou, S., Tahri, M., Sachiri, B., Mahi, M., Hafsi, M., Taky, A., et al. (2018). Comparative Study of Membrane Bioreactor (MBR). And Activated Sludge Processes in the Treatment of Moroccan Domestic Wastewater. Water Sci Technol. 78, 1129–1136. doi: 10.2166/wst.2018.384
LACSD (2013). Demonstration of Sequential Chlorination for Tertiary Recycled Water Disinfection At The San Jose Creek East Water Reclamation Plant: Final Report, Report prepared by the Sanitation Districts of Los Angeles County, Submitted to The California Department of Public Health. Whittier CA: LACSD.
Leu, S. Y., Chan, L., and Stenstrom, M. K. (2012). Toward long solids retention time of activated sludge processes: benefits in energy saving, effluent quality, and stability. Water Environ Res. 84, 42–52.
Limbergen, H. V., and Verstraete, W. (1998). Bioaυgmentation in activated sludge: current features and future perspectives. Appl. Microboil. Bíotechnol. 50, 16–23.
Margot, J., Rossi, L., Barry, D. A., and Holliger, C. (2015). A review of the fate of micropollutants in wastewater treatment plants. Wiley Interdiscipl. Rev. Water 2, 457–487. doi: 10.1002/wat2.1090
Matsumoto, M. R., Galeziewski, T. M., Tchobanoglous, G., and Ross, D. S. (1982). Filtration of primary effluent. J. WPCF 54, 1581–1591.
Matsumoto, M. R., and Tchobanoglous, G. (1982). “Feasibility and applicability of primary effluent filtration,” in Proceedings Utah Water Pollution Control Association Conference, Salt Lake City, UT, 159–159.
Nelson, F. G., and Lauer, W. N. (1994). Oils and grease as they affect sewage treatment. Sewage Works J. 16, 1105–1111.
Niku, S., Schroeder, E. D., Tchobanoglous, G., and Samaniego, F. J. (1981a). Performance of Activated Sludge Processes: Reliability, Stability and Variability, 1-124, R805097-01. Washington, DC: U.S. Environmental Protection Agency.
Niku, S., Schroeder, E. D., Tchobanoglous, G., and Samaniego, F. J. (1981b). Performance of Activated Sludge Processes: Reliability, Stability and Variability, 1- 11, EPA-600/S2-81-227. Cincinnati, OH: Project Summary U.S. Environmental Protection Agency.
Nzila, A., Razzak, S. A., and Zhu, J. (2016). Bioaugmentation: an emerging strategy of industrial wastewater treatment for reuse and discharge. Int. J. Environ. Res. Public Health 13, 846–856. doi: 10.3390/ijerph13090846
OCSD (2018). 2017-18 Annual Report, Resource Protection Division, Pretreatment Program, Orange County Sanitation District. Available onlie at: https://www.ocsd.com/Home/ShowDocument?id=26330 (cited May 2019).
OCWD (2017). Groundwater Replenishment System 2017 Annual Report, Prepared for The California Regional Water Quality Control Board, Santa Ana Region, Orange County Water District. Prepared by D. L. Burris ed Irvine, CA: DDB Engineering, Inc.
Oulton, R. L., Kohn, T., and Cwiertny, D. M. (2010). Pharmaceuticals and personal care products in effluent matrices: α survey of transformationand removal during wastewater treatment and implications for wastewater management. J. Environ Monit. 12, 1956–1978. doi: 10.1039/cOem00068j
Palen, G. (2015). How UV Disinfection Can Reduce Disinfection Byproducts While Enhancing Microbial Inactivation. Norfolk, VA: Virginia American Water Works Association.
Plósz, B. G., Liltved, H., and Ratnaweera, H. (2009). Climate change impacts on activated sludge wastewater treatment: a case study from norway. Water Sci. Technol. 60, 533–541. doi: 10.2166/wst.2009.386
Pronk, M., Giesen, A., Thompson, A., Robertson, S., and Loosdrecht, M. V. (2017). Aerobic granular biomass technology: advancements in design, applications and further developments. Water Pract. Technol. 12, 987–996. doi: 10.2166/wpt.2017.101
Rimer, A., DeCarolis, J., and Sathyamoorthy, S. (2017a). Guidelines for Source Water Control Options and the Impact of Selected Strategies on Direct Potable Reυse. Washington, DC: Water Environment & Research Foundation.
Rimer, A., DeCarolis, J., and Sathyamoorthy, S. (2017b). Guidelines for Source Water Control Options and the Impact of Selected Strategies on Direct Potable Reυse: Literature Compendium. Washington, DC: Water Environment & Research Foundation.
Rimer, A., DeCarolis, J., and Sathyamoorthy, S. (2017c). Guidelines for Source Water Control Options and the Impact of Selected Strategies on Direct Potable Reυse: Case Studies. Washington, DC: Water Environment & Research Foundation.
Sanitation Districts of Los Angeles County (2019). 2018.Recycled Water. Available online at: https://lacsd.org/waterreuse/AboutRecycledWater.asp (cited May 6, 2019).
SDPUD (2018). Draft Title 22 Engineering Report. North City Pure Water Project, San Diego Public Utilities Department. Available online at: https://www.sandiego.gov/sites/default/files/ncpw_project_draft_title_22_engineering_report_0.pdf
Serrano, E., and Salgado, E. R. (2016). Analysis of the Impact of the Industrial Fat, Oil and Grease (FOG) on a Municipal Wastewater Treatment Plant by Means Respirometry and Microscopic. Available online at: http://www.surcis.com/en/analysis-of-fog-effect-in-activated-sludge-process_12639.pdf (accessed May, 2019).
SWAN (2017). Sewershed Monitoring Survey, The Survey was Conducted for CH2M and Water Environment & Research Foundation. Smart Water Networks Forum.
Tchobanoglous, G. (2018). Integrated wastewater management: the future of water reuse in large metropolitan areas. Integr. Environ. Assess. Manag. 15, 160–163. doi: 10.1002/ieam.4103
Tchobanoglous, G., Cotruvo, J., Crook, J., McDonald, E., Olivieri, A., Salveson, A., and Trussell, R. S. (2015). Framework for Direct Potable Reuse, WateReuse Research Foundation, American Water Works Association, Water Environment Federation and National Water Research Institute, Washington, DC.
Tchobanoglous, G., Stensel, H. D., Tsuchihashi, R., and Burton, F. L. (2014). Wastewater Engineering: Treatment and Resource Recovery, 5th Edn. Metcalf and Eddy I AECOM. New York, NY: McGraw-Hill Book Company.
Tseng, S. K. (1979). The effect of grease on the activated sludge process in wastewater treatment. J Chinese Inst Eng. 2, 143–149.
U.S. EPA (2019). Reviewing New Chemicals under the Toxic Substances Control Act (TSCA). U.S. Environmental Protection Agency. https://www.epa.gov/reviewing-new-chemicals-under-toxic-substances-control-act-tsca/statistics-new-chemicals-review (cited May 20, 2019).
Wilén, B. M., Lumley, D., Mattsson, A., and Mino, T. (2006). Rain events and their effect on effluent quality studied at a full scale activated sludge treatment plant. Water Sci. Technol. 54, 201–208.
Wilson, G., Tchobnoglous, G., and Grittiyhs, J. (2007). The Grit Book: Understanding Wastewater Grit. Hillsboro, OR: Eutek Systems Inc.
Keywords: potable reuse, source control, enhanced wastewater treatment, satellite wastewater treatment facility, flow equalization, climate change
Citation: Tchobanoglous G and Leverenz H (2019) Comprehensive Source Control for Potable Reuse. Front. Environ. Sci. 7:95. doi: 10.3389/fenvs.2019.00095
Received: 16 January 2019; Accepted: 04 June 2019;
Published: 20 June 2019.
Edited by:
Björn Vinnerås, Swedish University of Agricultural Sciences, SwedenReviewed by:
Kara L. Nelson, University of California, Berkeley, United StatesCopyright © 2019 Tchobanoglous and Leverenz. This is an open-access article distributed under the terms of the Creative Commons Attribution License (CC BY). The use, distribution or reproduction in other forums is permitted, provided the original author(s) and the copyright owner(s) are credited and that the original publication in this journal is cited, in accordance with accepted academic practice. No use, distribution or reproduction is permitted which does not comply with these terms.
*Correspondence: George Tchobanoglous, Z3RjaG9iYW5vZ2xvdXNAdWNkYXZpcy5lZHU=
Disclaimer: All claims expressed in this article are solely those of the authors and do not necessarily represent those of their affiliated organizations, or those of the publisher, the editors and the reviewers. Any product that may be evaluated in this article or claim that may be made by its manufacturer is not guaranteed or endorsed by the publisher.
Research integrity at Frontiers
Learn more about the work of our research integrity team to safeguard the quality of each article we publish.