- 1Laboratoire Mer, Molécules, Santé (MMS EA2160), Université Catholique de l'Ouest, Angers, France
- 2Institut des Molécules et des Matériaux du Mans, UMR CNRS 6283, Le Mans Université, Le Mans, France
- 3Ifremer, Laboratoire d'Ecotoxicologie, Nantes, France
- 4UMR BOREA, MNHN, UPMC, UCBN, CNRS-7208, IRD-207, SFR ICORE, Université de Caen Normandie, Caen, France
- 5FR CNRS 3473 IUML, Laboratoire Mer, Molécules, Santé (MMS EA2160), Université de Nantes, Nantes, France
The impact of a microplastic (MP) mixture composed of polyethylene (PE) and polypropylene (PP) plastic particles, prepared from commercially available products, was evaluated in blue mussels Mytilus spp. exposed to three environmentally relevant concentrations: 0.008 μg L−1 (low), 10 μg L−1 (medium), and 100 μg L−1 (high). Organisms were exposed for 10 days followed by 10 days of depuration in clean seawater under controlled laboratory conditions. The evaluation of MP effects on mussel clearance rate, tissue structure, antioxidant defenses, immune and digestive parameters, and DNA integrity were investigated while the identification of plastic particles in mussel tissues (gills, digestive gland, and remaining tissues), and biodeposits (feces and pseudofaeces) was performed using infrared microscopy (μFT-IR). Results showed the presence of MPs only in the digestive gland of mussels exposed to the highest tested concentration of MPs with a mean of 0.75 particle/mussel (after the 10 days of exposure). In biodeposits, PE and PP particles were detected following exposure to all tested concentrations confirming the ingestion of MPs by the organisms. A differential response of antioxidant enzyme activities between digestive gland and gills was observed. Significant increases in superoxide dismutase (SOD) and catalase (CAT) activities were measured in the digestive gland of mussels exposed to the low (0.008 μg L−1) and medium (10 μg L−1) concentrations of MPs and in the gills from mussels exposed to the highest concentration (100 μg L−1) of MPs that could be indicative of a change in the redox balance. Moreover, an increase in acid phosphatase activity was measured in hemolymph of mussels exposed to 0.008 and 10 μg L−1 concentrations. No significant difference was observed in the clearance rate, and histopathological parameters between control and exposed mussels. This study brings new insights on the potential sublethal impacts of MPs at environmentally relevant concentrations in marine bivalves.
Introduction
In the last decades, global production of plastic has increased by 500% (over 322 million tons in 2016) and almost 10% of the annual production is estimated to end up into the oceans (Plastics Europe, 2016). Since the early 1970s, the presence of floating plastic has been reported in every marine water mass with great accumulation in the Northern Hemisphere subtropical gyres in the North Atlantic (Colton et al., 1974; Moore, 2008). In recent years, the presence of plastic debris called microplastics (MPs) mainly originating from macroplastic's fragmentation has been reported in aquatic ecosystems even in the most remote places such as the Artic (Cózar et al., 2014). Since the size of MPs is comprised between 1 μm and 5 mm (NOOA, 2008), they are likely to interact with marine organisms. Effects of MPs have been previously studied in phytoplancton (Cole et al., 2013), lugworms (Wright et al., 2013), and bivalves (Paul-Pont et al., 2016; Sussarellu et al., 2016; Ribeiro et al., 2017).
As they are filter-feeding organisms, bivalves are well-known bioindicators for environmental pollution and are closely studied to investigate the presence and toxicity of MPs toward aquatic organisms. These organisms filter large volumes of water and could ingest great quantities of plastic particles (Cole et al., 2013). Several studies have reported the presence of MPs in bivalves (Mathalon and Hill, 2014; Van Cauwenberghe and Janssen, 2014; Van Cauwenberghe et al., 2015) and their potential toxicity toward these organisms (von Moos et al., 2012; Paul-Pont et al., 2016; Sussarellu et al., 2016; Ribeiro et al., 2017). Several effects have been shown such as reproduction alterations (Sussarellu et al., 2016), inflammatory responses (von Moos et al., 2012), oxidative and DNA damages (Ribeiro et al., 2017), or translocation of particles into the circulatory system (Browne et al., 2008). However, in these studies, bivalves were exposed to mono-dispersed plastic microbeads at concentrations ranging from 23 μg L−1 to 2.5 g L−1 and exposures conducted at environmentally relevant concentrations are scarce. By contrast to concentrations higher than 1 mg L−1, concentrations between 10 and 100 μg L−1 can be considered as representative of polluted sites located off the coast (Goldstein et al., 2012; Sul et al., 2014). In addition, many of these studies used MPs smaller than those reported from the field and of regular shape and size (mostly often microbeads) which are less representative of fragmented plastic particles identified in marine environment (Lenz et al., 2016). Very recently, few authors started to test MPs made from fragmented plastics which can be considered as more representative of MPs found in aquatic ecosystems (Rainieri et al., 2018; Weber et al., 2018).
The aim of our study was to evaluate the accumulation and ecotoxicity of polypropylene (PP) and polyethylene (PE) MPs, in the blue mussel Mytilus spp. Particles of PP and PE were milled in the laboratory from commercially available products and the types of polymers were chosen according to a previous study conducted in situ in the French Region Pays de la Loire (Phuong et al., 2017). Mussels were exposed to environmentally relevant concentrations: a low concentration of 0.008 μg L−1 for the coast regions (Desforges et al., 2014), a medium concentration of 10 μg L−1 for coastal region/gyres (Sul et al., 2014), and a higher concentration of 100 μg L−1 for gyres (Goldstein et al., 2012). A multi-biomarker approach was chosen to evaluate the effects of MPs on mussels. The impact of MP exposure on mussel physiology was evaluated by measuring the clearance rate and tissue alterations by histological observations. In addition, detoxification and oxidative stress mechanisms were assessed. The measurement of Reactive Oxygen Species (ROS) was performed in the hemocytes. Antioxydant enzyme activities of glutathione-S-transferase (GST), catalase (CAT), and superoxide dismutase (SOD) were measured in gills and in the digestive gland, such as the gene expression level of glutathione peroxydase (GPx), CAT, and SOD. In hemocytes, the alteration of the immune system was investigated through the measurement of acid phosphatase (AcP) activity, which can provide early warning signals of the sublethal effects of contaminants and the susceptibility of animals to infectious diseases (Gagnaire et al., 2007; Luna-Acosta et al., 2010). Genotoxic effects in the hemocytes were evaluated by the comet assay (Gomes et al., 2013; Barranger et al., 2014; Lacaze et al., 2015). In parallel, the quantification of MPs content in mussel tissues (gills, digestive glands, and other tissues), and biodeposits (feces and pseudofaeces) was conducted after tissue digestion, filtration, and observation in microscopy coupled with infrared spectroscopy.
Materials and Methods
Preparation of Microplastic Powders and Suspensions
Preparation and Characterization of Microplastics
Microplastics were prepared by cryo-milling a few grams of commercial PE and PP samples, chosen for their bright colors that may help for their microscopic observation.
A few grams of each plastic (PE and PP) were put into a sample container containing a magnetic bar or impactor and both sides of the container were closed with two stainless steel caps. The container was inserted into a cryogenic mechanical miller (SPEX, 6770 Freezer-mill) filled with liquid nitrogen. During the milling process, an oscillating magnetic field was generated, which drove the magnetic bar forward and backward inside the container and make the impacts with the caps. Milling consisted in 2 min of pre-cooling followed by 2 cycles of milling. Each cycle lasted 4 min with a 1-min pause between the 2 cycles. The so-obtained powders of PE and PP particles were then sieved during 60 min, respectively on a stain sieve of 400 μm. All the fraction under 400 μm was kept and a granulometry analysis was done using a particle laser diffractometer Beckman Coulter LS 130 to measure the size distribution of the particles.
Microplastic Suspensions
Stock suspensions of each polymer were prepared in milliQ water at concentrations of 1 and 0.1 mg mL−1. One aliquot was sampled from the suspension at 0.1 mg mL−1 and three suspensions were successively prepared by serial dilutions in milliQ water to obtain the last suspension at 0.1 μg mL−1. To achieve the desired final concentration of both polymers in the aquariums, a volume of either the suspension at 1 mg mL−1, 0.1 mg mL−1, or 0.1 μg mL−1 was sampled and spilled in each aquarium to obtain 50% PE and 50% PP at 0.008, 10, and 100 μg L−1 (methodology summarized in Supplementary Material S1). In this experiment, no surfactant was used to avoid any potential toxic effect of the substance used and potential change in the behavior of MPs. A manual vigorous mixing was thus applied to each suspension before adding the final aliquot to the aquariums. Moreover, in order to confirm our method of dispersion, an aliquot of the stock and diluted suspensions, which corresponds to the volume added to aquariums to obtain 100, 10, and 0.008 μg L−1, was observed under the microscope to confirm the presence of PE and PP particles. Every suspension was prepared and added daily, before each water renewal.
Maintenance of Organisms and Exposure Conditions
Mussels
Adult mussels (Mytilus spp.) were collected from a mussel farming located at Saint-Cast-le-Guildo, Northern French coast, and transferred to the laboratory tanks filled with artificial seawater (ASW) at a salinity of 30 PSU (Practical Salinity Units) which was prepared with commercial salt (Tropic Marin®, France), and under continuous aeration. Mussels were selected on the basis of size (5–6 cm in length); regarding their reproductive cycle, histological observations revealed that the mussels' batch showed 3 gametogenetic stages: late spawning/post spawning (cycle n), sexual resting, early beginning of gonial mitosis (cycle n+1). Mussels were kept for 8 days for acclimatization in tanks (70 individuals per tank) containing 35 L of ASW. The acclimatization and the following experimental treatments were conducted under the same controlled laboratory conditions, consisting of a stable temperature of 15 ± 1°C and a photoperiod (12:12 h). Daily, half of the water was renewed and mussels were fed with an algal solution of Tetraselmis suecica (Teramer, France) according to the product specifications for the feeding of bivalves (40 μL L−1 of water, 108 cells mL−1, 4.3 x 1010 cells mussel−1).
Experimental Setup and Sampling
At the end of the acclimatization period, 15 mussels were sampled to establish the physiological conditions of mussels at T0. The other mussels were then randomly subdivided into groups of 4 individuals and transferred into 3.5 L glass aquariums containing 3 L of ASW. For each experimental condition, eight aquariums containing each 4 individuals (32 mussels per condition) were used. Mussels were exposed for 10 days to 0.008, 10, or 100 μg L−1 of a mixture of PE and PP (as described in section Preparation of Microplastic Powders and Suspensions). A group of unexposed mussels (negative control) was maintained in the same condition as the treatment groups. Organisms were daily fed for an hour before water renewal, to avoid interactions between algae and plastic particles. Biodeposits (feces and peudofaeces) were sampled each day with glass pipettes just before water renewal and plastic contamination.
From the 15 mussels sampled just before the beginning of the exposure (T0): 5 mussels were used for histopathology and AcP activity; 5 for genotoxicity (comet assay) and oxidative stress analysis, and 5 for condition index and analysis of MPs content in mussel tissues. At the end of the exposure period (10 days = T10), 20 mussels (from 5 replicate aquariums) per condition were sampled: 5 mussels (1 per replicate aquarium) for analysis of MPs content in mussel tissues; 5 mussels for histopathology and immune markers; 5 mussels for genotoxicity (comet assay) and oxidative stress analysis; and finally, 5 mussels for the evaluation of the clearance rate. The remaining mussels (from the last 3 replicate aquariums) were cleaned by gently rinsing the shell to avoid any transfer of MPs, and transferred to clean glass aquariums for 10 days of depuration with similar ASW, maintenance, and food conditions as those used during the exposure. After the 10 days of depuration (T20), 5 mussels per condition (1–2 per replicate aquarium) were used for the analysis of MPs content in mussel tissues and the measurement of the clearance rate.
Hemolymph was individually collected by punction from the posterior adductor muscle sinus using a sterile hypodermic needle (23 G) in a 2 mL syringe pre-rinsed with the anticoagulant Alsever's solution (113.7 mM glucose, 27.2 mM sodium citrate, 58.44 mM sodium chloride, pH 6.1). For immune markers, hemolymph was immediately frozen in liquid nitrogen and stored at −80°C. For flow cytometry analyses and slide preparation for the comet assay, hemolymph was kept on ice until use (N = 5 individuals, each from a different replicate aquarium).
For histological observations, flesh was removed from the shell and the whole mussels were individually fixed in Davidson's solution (10% glycerol, 20% formaldehyde, 30% ethanol at 95%, 30% sterile seawater, 10% acetic acid) for 48 h, then in 70% ethanol up to analysis (N = 5 individuals, each from a different replicate aquarium).
Gills and digestive glands were sampled (from 5 mussels, each from a different replicate aquarium) and either stored at −20°C for determination of MPs content, or immediately frozen in liquid nitrogen for gene expression and enzymatic activity measurements. Just before molecular and biochemical analysis, frozen tissues were grinded into a fine powder at −196°C with a mixer mill MM400 (Retsch) and directly stored at −80°C for enzymatic analyses or in trizol for RNA extraction. After grinding, half of each samples (under powder form) were used for gene expression to obtain 5 individual samples per condition. For enzymatic activity measurements, the other half of each powder were pooled in three lots, corresponding approximatively to 1.5 mussels per tube (mussels from each tube coming from a different replicate aquarium), to get enough tissue quantity for the analysis (N = 3).
Microplastic Analyses in Tissues
Sample Digestion and μFT-IR Analysis
Detection of MPs was performed on individual organs of each mussel at the beginning of the exposure (T0), the end of the 10 days exposure (T10), and at the end of the 10 days depuration period (T20): gills, digestive gland and mussel's remaining tissue following the first step of the protocol published by Phuong et al. (2017). The tissue sample was placed into a 10 mL beaker with 5 mL of 10% KOH (m/v). The mix was then heated at 60°C with a 2 h agitation for gills and digestive glands, but 24 h for mussel “remaining tissue.” For biodeposits, samples from each day and each tank were pooled together, dried and weighted, and finally placed into a 50 mL beaker with 20 mL of 10% KOH (m/v). The mix was then heated at 60°C with agitation for 24 h. The solutions were then filtered on a 12 μm cellulose nitrate filter (Whatman®, Germany), and the filter was dried at room temperature in a glass closed Petri dish until analysis.
The analysis of PE and PP particles on the filters was performed by using Fourier transform infrared microscopy system (μFT-IR; Spotlight 200i FT-IR microscopy system, PerkinElmer®) in reflection mode. Each spectrum was recorded after 8 accumulations ranging from 600 to 4,000 cm−1 and the whole surface filter was inspected, each detected particle being individually analyzed. The obtained spectra were then compared to the polymer database (PerkinElmer Polymer database) to confirm the type of plastic.
Quality Control
To prevent any cross-contamination, all equipments used in the laboratory were previously rinsed with milliQ water, dried at room temperature in a hood and kept under aluminum foil to avoid contact with the ambient air. During the experiments, laboratory coats made of cotton and nitrile gloves were worn, dissection of mussels was operated under a hood, and tissue samples were kept in aluminum foil to avoid contamination. During digestion, beakers were covered with aluminum foil. Once the filtration was performed, the filters were kept in glass Petri dishes until μFT-IR analysis. Because of a remaining risk of cross-contamination of the samples, blanks were performed. The procedure of a sample digestion and μFT-IR analysis for blanks without mussel tissues was performed each time a series of digestion was performed (6 replicates in total).
Ecophysiology Measurement
Condition Index
The total weight, and the flesh weight of 10 mussels were individually determined for each condition at the beginning (T0) and end of the 10 days exposure period (T10). The condition index (CI) was determined as follows:
CI = [(wet weight of soft tissues/total wet weight) * 100] (AFNOR, 1985)
Clearance Rate
Clearance rate (CR), defined as the volume of water cleared of suspended particles per unit time, was estimated for mussels previously exposed for 10 days to clean seawater (negative control) or PE+PP (0.008, 10, and 100 μg L−1) and after 10 days of depuration (in clean ASW), using a static system (Pernet et al., 2008). Mussels were placed in 1.3 L metabolic chambers for 1-h before measurements started, then provided with Tisochrysis lutea at an initial concentration of 345,000 cells mL−1. Mixing was promoted by fine bubble aeration around the wall of the metabolic chamber to minimize the re-suspension of feces. The clearance rate was measured by an indirect method of assessing the concentration of the algal solution every 15 min for 1 h according to classical algal cell counting technique (Utermöhl, 1958). Briefly, a fixation of the algal cells was realized adding a drop of Lugol's solution (I2, 5%; KI 10%, seawater) to 10 ml of the sampled. Cells were counted under a light microscope using a Malassez counting chamber at 250X (Duchemin et al., 2008).
Histological Observations
After fixation in Davidson's solution, cross sections of mussels (5 mm-thick) were cut and then routinely processed for histology. The 3-μm paraffin-embedded sections were stained according to the trichrome protocol of Prenant Gabe (Gabe, 1968). Each specimen was sexed and classified into distinct stages of gonadal maturation corresponding to sexual resting, early developing (gonial mitosis), developing, sexual maturity, spawning, and post-spawning (Berthelin et al., 2001). The histological slides were also examined to determine the 9 following tissue alterations: (1–2) diffuse hemocytic infiltrations and granulocytomas (aggregated hemocytes) in the connective tissue; (3–4) hemocytic infiltrations in the digestive tract (stomach and intestine) and necrosis; (5–6) atrophies of the wall of the digestive tubules (with lumen enlargement) and necrosis; (7) presence of copepods Mytilicola sp. in the lumen of digestive tract; (8) infiltrations/degeneration of gills and; (9) myodegeneration of the anterior pedal-byssal retractor muscles and foot when it was present on the cross section. For each mussel, the tissue disturbances were semi-quantified according to a scale with 4 levels from slight (score of 1) to severe (score of 4) (Buisson et al., 2008). An average index was then calculated for each condition and tissue alteration.
Biomarkers
Biochemical Measurements
For the assessment of GST, SOD, and CAT activities, each sample was homogenized in TRIS buffer (TRIS 50 mM, NaCl 150 mM, DTT 1 mM), antiprotease mixture (Sigma P8340, diluted in 1/1,000) adjusted to pH 7.4 in a 1:3 ratio (w:v) at 4°C to prevent enzyme or tissue degradation. The homogenates were then centrifuged for 25 min at 9,000 g. GST activity was determined spectrophotometrically at 340 nm (e = 9.6 mM−1 cm−1) by monitoring the formation of 1-glutathion-2,4-dinitrobenzene, resulting from the conjugation of the substrate, 1-chloro-2,4-dinitrobenzene (CDNB), with glutathione reduced form (GSH) (Habig et al., 1974). SOD activity was determined as the degree of inhibition of cytochrome C reduction by superoxide anion radicals generated by xanthine oxydase/xanthine reaction at 550 nm (McCord and Fridovich, 1969). Results were expressed as SOD Unit per mg protein (SOD Unit mg−1 protein) with one unit of SOD activity defined as the amount of sample producing 50% inhibition in 1 mL reaction system per mg of protein. The activity of CAT was spectrophotometrically estimated as the decrease in absorbance at 240 nm (e = 0.04 mM−1 cm−1) due to dismutation of hydrogen peroxide (H2O2) (Claiborne, 1985). Results were expressed as μmoles of H2O2 transformed per min and per mg of protein (μmoles min−1 mg−1 protein).
Acid phosphatase (AcP) activity was measured in pooled hemolymph samples and expressed as enzyme unit (1 unit being equal to the quantity of enzyme required for the formation of 1 μmol of p-nitrophenol min−1 mg of protein) following instructions of Sigma kit (CS0740).
Amylase activity was measured in individual digestive gland with Sigma® kit (MAK009). Amylase activity was determined using a coupled enzymatic assay resulting in a colorimetric (405 nm) product, proportional to the amount of substrate ethylidene-pNP-G7 cleaved by the amylase (1 unit being the amount of amylase that cleaves ethylidene-pNP-G7 to generate 1.0 mmole of p-nitrophenol per minute).
Gene Expression of GPx, SOD, and CAT
Total RNA was isolated from frozen tissues powder using TRI Reagent according to the manufacturer's instructions. RNA concentrations were determined using a ND-1000 spectrophotometer (ThermoScientific®, Waltham MA, USA). RNA was treated with DNase I (Ambion, Life Technologies®, 0.1 U per μg of RNA) following the manufacturer's instructions, and precipitated with isopropanol (v/v) and 0.3 M sodium acetate. RNA integrity was assessed on an Agilent bioanalyzer using RNA 6000 Nano kits (Agilent Technologies®), according to the manufacturer's instructions. Reverse transcription was carried out with the High- Capacity cDNA Reverse Transcription Kit (Applied Biosystems®), according to the manufacturer's instructions on 1 μg of total RNA. The levels of SOD, CAT and GPx transcripts were measured by real-time PCR using Elongation Factor 1 alpha (EF1α) and ribosomal protein 7 (rpl7) as reference genes. Specific primers were designed using Primer 3V 4.0.0 (Untergasser et al., 2012). The PCR efficiency (E) was assessed for each primer and tissue on serial dilutions of a pool sample containing cDNA from all experimental conditions and was comprised between 95% < E < 104%. The primer sequences and Genbank accession numbers for the genes considered are in Supplementary Material S2. Real-time PCR reactions (20 μL final volume containing: Agilent Brilliant III Ultra-Fast SYBR® Green QPCR Master Mix, 0.05 μM of each primer and cDNA diluted to 1/40 for digestive gland and to 1/20 for gills) were carried out in triplicate in 96-wells microplates in a Mx3000P thermalcycler (Agilent®). Runs started with 3 min at 95°C, followed by 40 cycles of 20 s at 95°C and 20 s at 60°C. Accurate amplification of the target amplicon was checked by performing a melting curve. Each run included a cDNA inter-run control (composed of a pool sample containing cDNA from all experimental conditions), a no-template control and a water control. The geometric mean of the two reference genes was used to normalize gene expression using the Pfaffl formula (Pfaffl, 2001) and specific amplification efficiencies.
ROS Production Measurement by Flow Cytometry
Potential induction of ROS production in hemolymph from mussels exposed to MPs was evaluated with the fluorescent probe 2,7 -dichlorodihydrofluorescein diacetate (H2DCF-DA). Protocol was adapted from Haberkorn et al. (2014). A stock solution of H2DCF-DA at 10 mM in DMSO was used to prepare a 1 mM working solution in filtered sterile seawater (FSSW) prior to use. Briefly, 100 μL of each hemolymph sample were diluted to ¼ with FSSW. Samples were incubated at 18°C in the dark for 120 min with 10 μM H2DFC-DA (Haberkorn et al., 2014). After the incubation period, DCF fluorescence, quantitatively related to the ROS production of hemocytes, was measured at 515–545 nm with an Accuri C6 flow cytometer (Becton Dickinson Accuri™) flow cytometry.
Comet Assay
The comet assay was conducted as previously described (Akcha et al., 2003; Barranger et al., 2014). Following sampling, individual hemocytes were recovered by centrifugation (1,500 rpm, 5 min) of the hemolymph samples. The hemocyte pellet was then resuspended in 160 μL of 0.5% low melting point agarose for the preparation of 2 comet slides. Following lysis and denaturation in the electrophoresis buffer, DNA migration was performed for 15 min at 23 V (390 mA, E = 0.66 V cm−1). At the end of electrophoresis, the slides were washed by incubation for 3 × 5 min in Tris base 0.4 M, pH 7.5. In order to obtain permanent preparations, the slides were immersed in absolute ethanol for 10 min to dehydrate, then dried at room temperature. Just prior to analysis, 75 μL of GelRed at 8 mg L−1 were spread over each slide using a cover glass. The slides were placed for at least 1 h in the dark at 4°C for coloration, then analyzed using an optical fluorescence microscope (Olympus BX60, ×40) equipped with a CDD camera (Luca-S, Andor Technology®) and image analysis system (Komet 6, Kinetic Imaging Ltd. ®). At least 50 nuclei were analyzed per slide and the percentage of DNA present in the Comet tail (% Tail DNA) was measured for each observed nucleus.
Statistical Analysis
The statistical analyzes were carried out using software R (R.3.3.1) and Statistica 6.0. For each of the biomarkers, the means of the different conditions were compared with a 1-factor ANOVA test followed by the Tukey's test after verification of the normality of the data (Shapiro-Wilk test) and homogeneity of the variances (Levene test). Kruskal-Wallis tests followed by multiple comparisons were used when these conditions of application were not met and for histopathological parameters (expressed by ordinal values).
Results
Size Characterization of MPs and Analysis in Tissues and Biodeposits
For MP dry particles, all sizes between 0.4 and 720 μm for PE and 0.4 and 950 μm for PP were present in samples with a Gaussian distribution of size around a mean value (± SD) of 287 (± 150) μm for PE and 204 (± 132) μm for PP.
Since we selected environmental concentrations, the suspensions were not concentrated enough to enable granulometric analysis. Therefore, characterization of MP suspensions was carried out by the microscopic observation of an aliquot of several diluted suspensions at 0.1 μg mL−1 (which corresponds to the volume added to aquariums to obtain 0.008 μg L−1). However, once deposited on a glass slide, aggregates were quickly formed making not possible to count and measure each particle individually in order to confirm the precise concentration of suspensions.
In mussel tissues, particles of PE and PP were only observed in the digested digestive gland of organisms exposed to the highest concentration of PE and PP (Figure 1) with a total quantity of MPs of 0.75 ± 0.5 MP per individual and 2.33 ± 0.29 MP per gram of wet soft tissues weight (Table 1). In gills and mussels' remaining tissue, no MPs were observed whatever the tested concentrations of MPs. In biodeposits, particles of PE and PP (Figure 1) were observed from mussels exposed to all concentrations of MPs as presented in Table 1. After the depuration period, no MPs were observed in mussels' tissue, but 1 particle (PP) and 2 particles (1 PE and 1 PP) were identified in biodeposits from organisms previously exposed to 10 and 100 μg L−1, respectively.
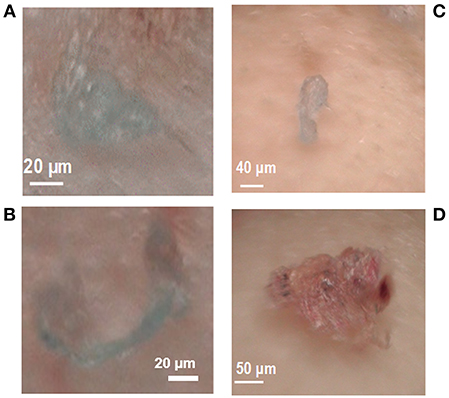
Figure 1. Particles of PE and PP in the digestive gland (A,B) of mussels Mytilus spp. exposed to 100 μg L−1 and biodeposits (C,D) of mussels exposed to 10 and 100 μg L−1, respectively.
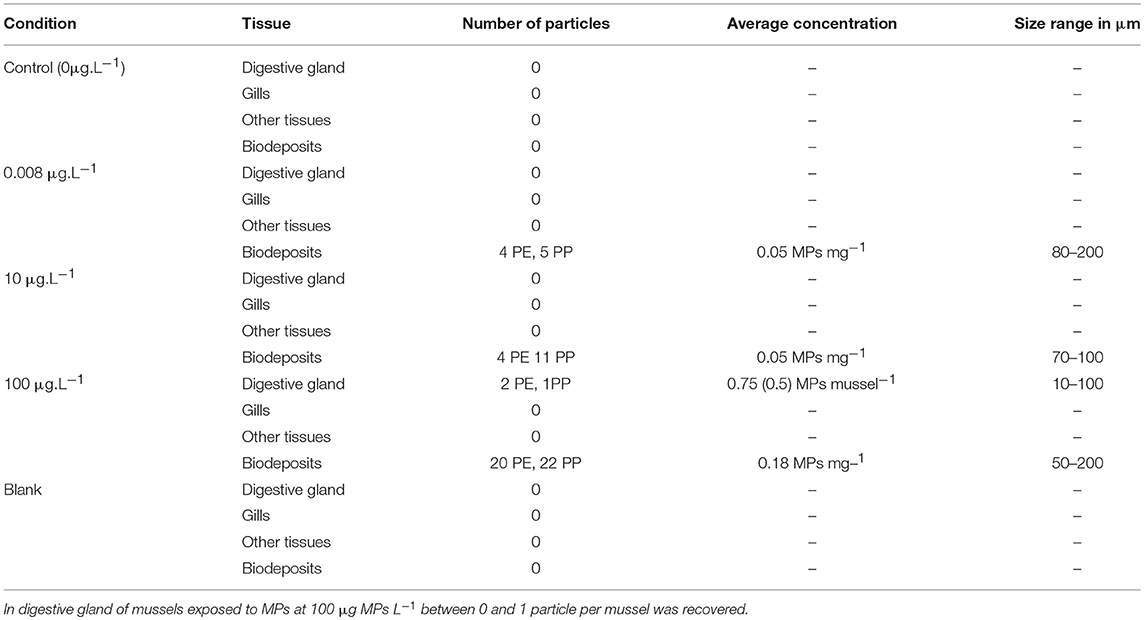
Table 1. Average concentration and size range of microplastics recovered from tissue and biodeposits (feces and pseudofaeces) of Mytilus spp. after 10 days of exposure (values in parentheses represent the standard deviation).
Clearance Rate and Condition Index
Nine percent of mortality at maximum was observed over the exposition period of 10 days (T10). As measured at the end of the exposure and depuration period, MP contamination had no significant impact on the clearance rate of mussels irrespective to MP concentration and measurement time (Supplementary Material S3).
No statistically significant alteration in the CI was observed in mussels between the beginning of the experiment at T0 (21.1 ± 2.9) and the control after 10 days of exposure (25.2 ± 3.5) and between the control and all exposure concentrations (0.008 μg L−1: 25.8 ± 2.7, 10 μg L−1: 26.1 ± 3, and 100 μg L−1: 23.5 ± 3.5) after 10 days of exposure.
Histopathology
Overall, control and exposed mussels showed few tissue alterations revealing a “relatively good health status”. The average histopathological indices ranged from 0 (presence of copepods Mytilicola sp. In the lumen of digestive tract) to 3.4 ± 0.6 (SEM) (myodegenerations) (Supplementary Material S4). For muscular degenerations, the index increased with the concentration of MPs but the differences were non-significant (Kruskal- Wallis test, p = 0.16). Moreover, no significant differences were found irrespective to tissue alterations and exposure conditions (control and mussels exposed to the 3 concentrations of MPs) (Kruskal- Wallis tests, p > 0.05).
Enzymatic Activities, Gene Expression and ROS Measurement
In the digestive gland, CAT and SOD activities significantly increased in organisms exposed to 0.008 and 10 μg L−1 compared to the control organisms (p < 0.01). A decrease in GST activity was measured in organisms exposed to the highest concentration (100 μg L−1) compared to the control organisms (p < 0.01) (Figure 2). No significant alteration of amylase activity was measured (data not shown).
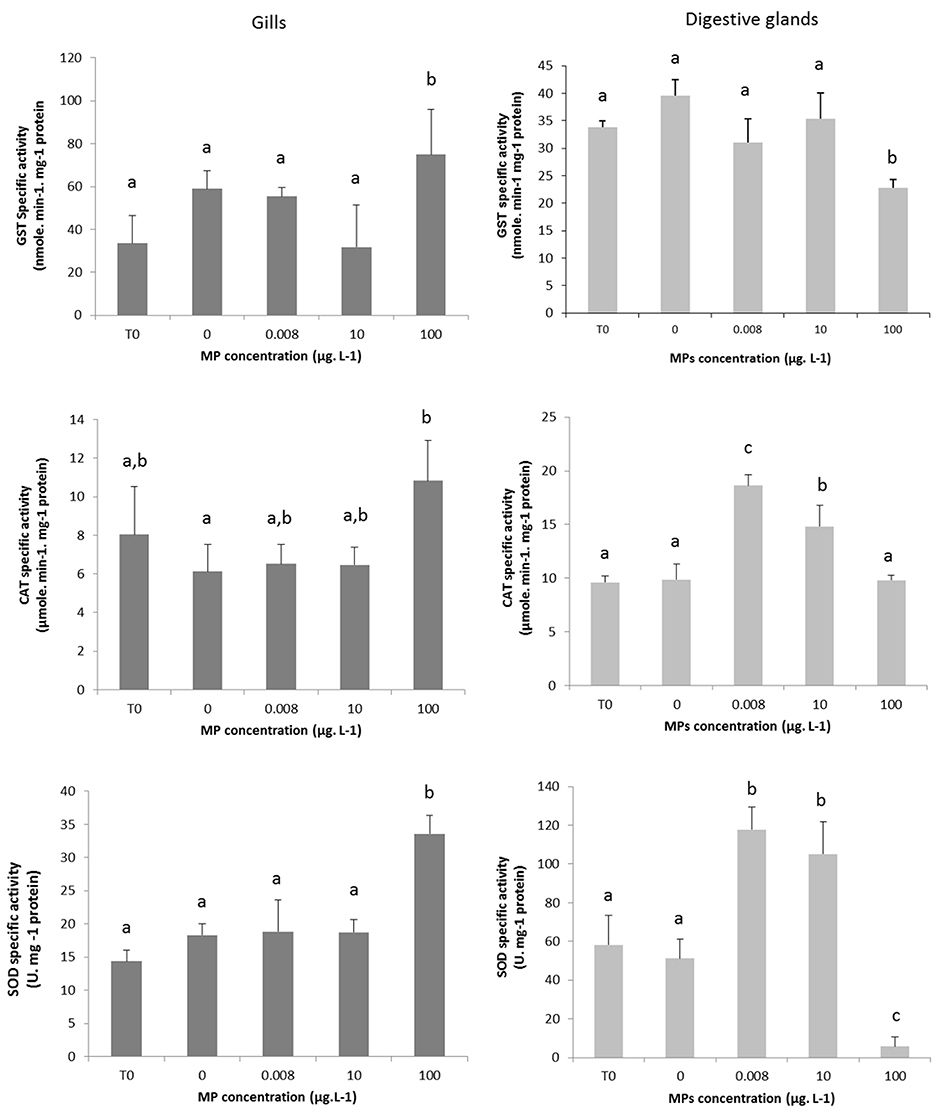
Figure 2. Enzymatic activity of GST, SOD, and CAT in gills and digestive gland of mussels Mytilus spp. at T0 and after 10 days in control mussels (0 MPs L−1) and in mussels exposed to PE and PP microplastics at 0.008, 10, and 100 μg MPs L−1 (mean + SD, N = 3). Different lower-case letters indicate significant differences between control and microplastic exposed treatment (p < 0.01).
In gills, CAT and SOD activities were significantly higher in organisms exposed to the highest tested concentration of 100 μg L−1 compared to the control organisms at T10 (p < 0.01) and the two lower concentrations of MPs tested in the experiment. An increase in GST activity was measured in organisms exposed to 100 μg L−1 of MPs compared to the control organisms (p < 0.01) and the two lower concentrations of MPs tested in the experiment. (Figure 2).
No significant differences in Gpx, SOD and CAT gene expression level was observed in gills or in the digestive gland (Figure 3). Similarly, no differences in ROS production was measured between control and exposed organisms whatever the tested concentrations (Figure 4).
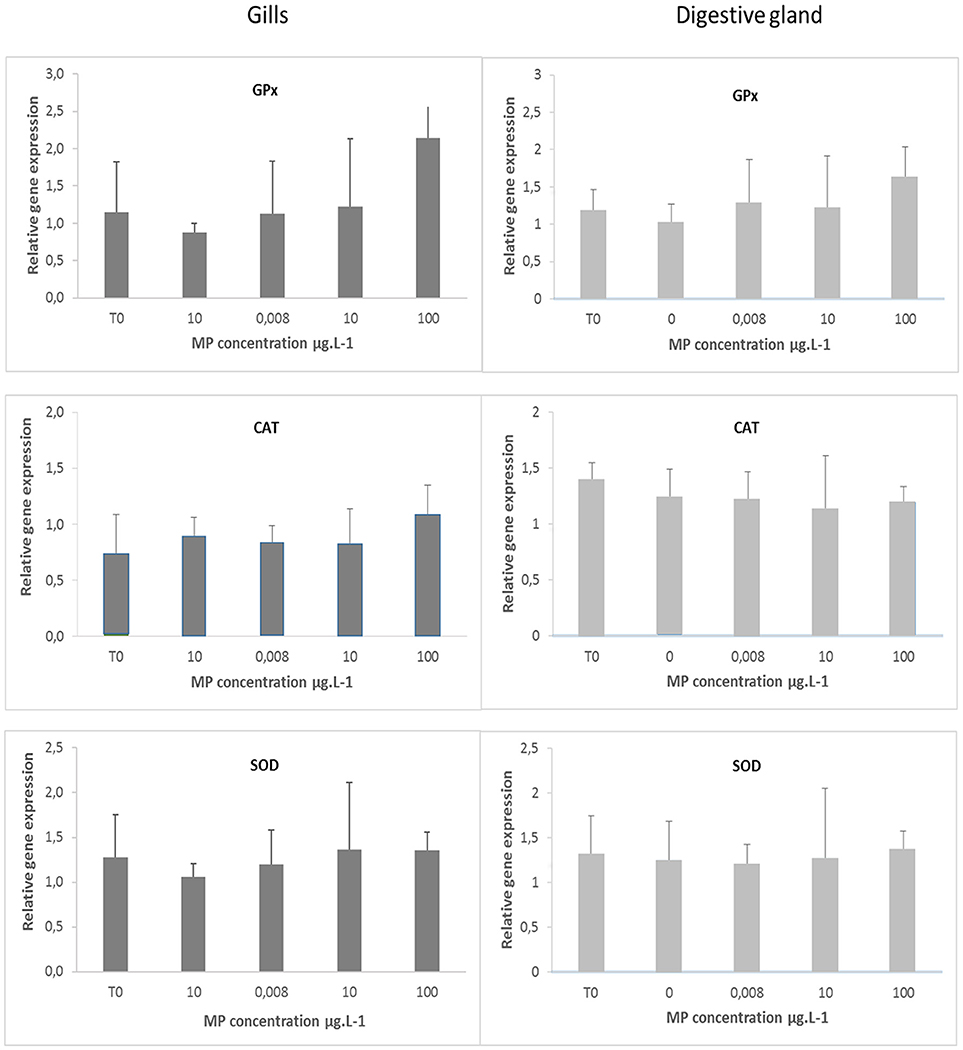
Figure 3. Relative gene expression for GPx, CAT, and SOD in gills and digestive gland of mussels Mytilus spp. at T0 and after 10 days in control mussels (0 MPs L−1) and in mussels exposed to PE and PP microplastics at 0.008, 10, and 100 μg MPs L−1 (mean + SD, N = 5).
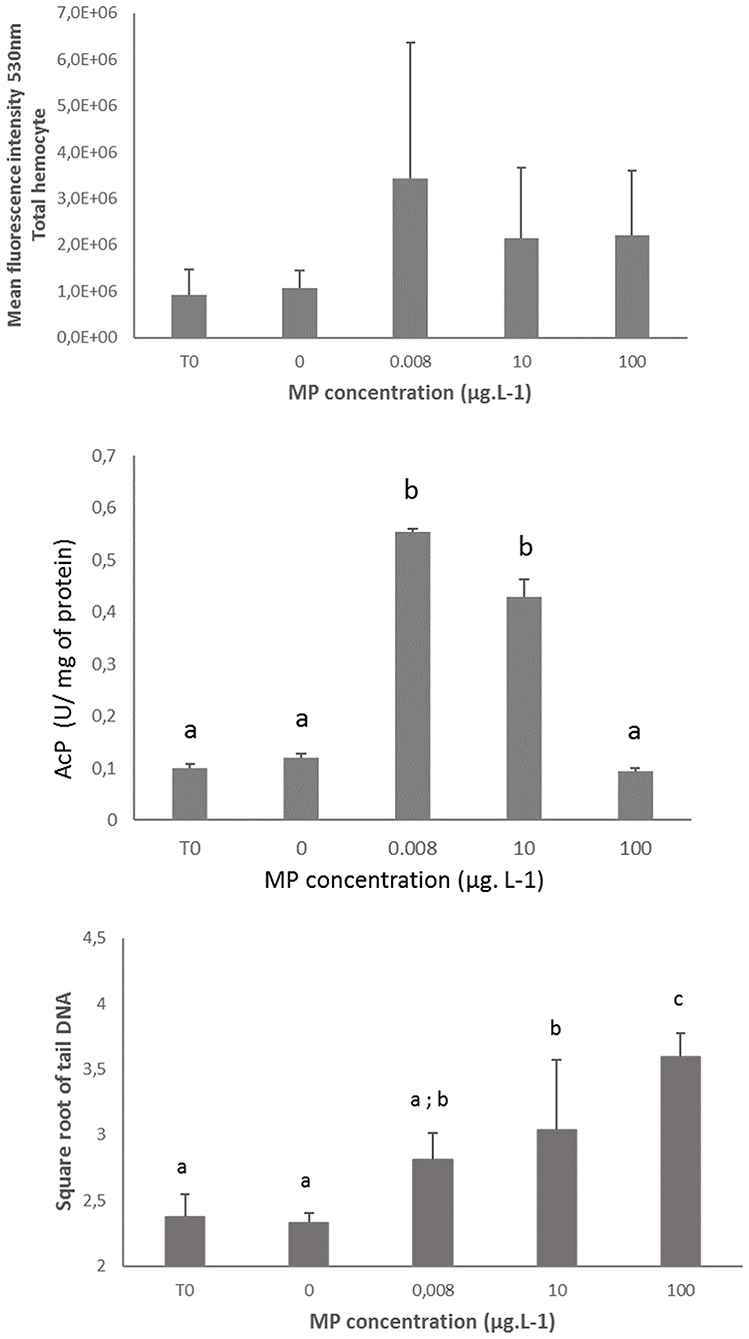
Figure 4. Reactive oxygen species (ROS) production, Acid phosphatase (AcP) activity and DNA integrity in hemolymphe or hemocytes of mussel Mytilus spp at T0 and after 10 days in control mussels (0 MPs L−1) and in mussels exposed to PE and PP microplastics at 0.008, 10 and 100 μg MPs L−1 (mean + SD, N = 5 for ROS and DNA and mean + SD, N = 3 for AcP). Different lower-case letters indicate significant differences between control and microplastic exposed treatment (p < 0.01).
Immune Markers and DNA Damage
Significant increase in AcP activity was measured in organisms exposed to 0.008 and 10 μg L−1 compared to the control organisms and the concentration of 100 μg L−1 (p < 0.01) (Figure 4).
The genotoxic effects of MPs, analyzed by the comet assay and expressed as % of tail DNA, are shown in Figure 4. No changes in the background level of DNA damage (mean Tail DNA value of 5.5%) was observed in hemocytes from control organisms during the 10 days experiment period (t-test for independent samples, p = 0.61). A statistically significant increase in DNA damage was observed in mussels exposed to 10 and 100 μg L−1 compared to the control (+30 and +54%, respectively), with a concentration-dependent trend (p < 0.001). However, the % Tail DNA remained at a low value (<12% Tail DNA) even following exposure to the highest concentration.
Discussion
Ingestion of PE and PP MPs
In the present study, we evaluated the presence and toxicity of a mixture of PE and PP MPs in mussels Mytilus spp. exposed to environmentally relevant concentrations (0.008, 10, and 100 μg L−1). Assessment of MP impact on aquatic organisms is particularly challenging and several uncertainties lie on MPs research due to the diversity of particles characteristics and experimental set up that can influence their behavior and biological effects. In the aquatic environment, organisms are exposed to different types of polymers (PE, PP, PS, Polyvinylchloride, etc.) with different shapes and sizes and they can be associated with additives (phthalates, BPA, etc.) or environmental contaminants such as persistent organic pollutants. Important difficulties in laboratory experiments lie on the choice of MPs (microbeads vs. more realistic MPs such as fibers and fragments; without additives), the exposure concentrations (generally higher than those observed in the field and expressed in terms of particle number or mass) and the dispersion technique (use of solvents or air pump) especially for PE and PP which floats on the water surface (Phuong et al., 2016). Here, taking into account technical difficulties and limitations, we attempted to conduct the experiment at the most possible relevant conditions in terms of MP type (PE and PP) and origin (commercial products). The mean size of PE and PP particles, measured by granulometry, were 287 and 204 μm, respectively, which is consistent with the mode of preparation of particles (sieving under 400 μm). However, the analysis of the size distribution of each powder showed us a small proportion of particles with size larger than expected (720 and 950 μm instead of 400 μm) which could be due to the agglomeration of a part of the particles. Concerning the smallest particles, the granulometric analysis showed the presence in small quantities of particles < 1 μm and down to 400 nm which is the slowest size detected by the instrument. Nevertheless, a very small number of particles smaller than 400 nm as isolated or aggregated particles could also be present. In this study, we found both PE and PP in tissues and biodeposits of mussels exposed to three environmental concentrations of MPs, confirming their ingestion by bivalves. MPs were only found in the digestive gland from mussels exposed to the highest tested concentration (100 μg L−1) and a very low number of particles was observed. Whatever the exposure concentrations of PE and PP, particles between 50 and 200 μm were filtered and excreted by mussels. These results suggest that MPs did not accumulate in mussels tissues and were rapidly eliminated with feces or pseudofaeces like it was previously observed in oysters Crassostrea gigas exposed to PS (2–6 μm, 0.023 mg L−1) (Sussarellu et al., 2016). It is also possible that smaller particles such as nanoplastic particles were accumulated in mussels, but there are technically undetectable as μFTIR allows particle analysis at a lower size limit of 20 μm. Moreover, the number of MPs found in biodeposits increased with exposure concentration confirming that the more particles were added to the test media, the more MPs were ingested by bivalves.
No Impact of Microplastic Exposure at the Individual Level
In the present study, CI values were representative of previously published data on M. edulis (Garen et al., 2004) and no alteration in the CI was observed in mussels between the control and all MP treatments.
The ecophysiology evaluation did not demonstrate any alteration in the clearance rate of mussels exposed to MPs. A previous study reported the decrease in the filtration activity of M. edulis exposed to nano-PS showing the ability to mussels to detect nano-PS and to decrease filtration in their presence (Wegner et al., 2012). However, unlike our study, organisms were exposed to nanomicrobeads at very high concentrations (0.1–0.3 g L−1). A decrease in clearance rate was measured in clams Atactodea striata exposed to microgranules of PS (10 items L−1 and 1,000 items L−1, 63–250 μm) (Xu et al., 2016).
Histopathology parameters showed no signs of inflammation or tissue alterations in mussels exposed to MPs at all concentrations tested. Only myodegenerations of the anterior pedal-byssal retractor muscles seemed to increase along with MPs concentrations but it was not statistically significant which could be due to the low number of mussels analyzed and high interindividual variability. By contrast, previous studies on M. edulis have shown an inflammatory response with formation of granulocytomas after 6 h of exposure to virgin high-density PE at 2.5 g L−1 (von Moos et al., 2012) but also increase in ceroids and hemocyte infiltrations in mussels exposed to micro-PS at 32 μg L−1 (Paul-Pont et al., 2016).
Modulation of Anti-oxidant Enzymes and ROS Production by Combined exposure of PE and PP MPs
Evaluation of oxidative stress is one of the most commonly measured biomarkers for environmental contaminants exposure including MPs (Ruiz et al., 2015; Sussarellu et al., 2016). In the present study, flow cytometry analyses did not show any increase in ROS production, which could be due to the sensitivity of the technique and/or the sampling time chosen for its measurement. Since ROS production is transitory, in measuring it after 10 days there is a possibility that we missed the increase expected by MP exposure. Choi et al. (2018) showed an increase in ROS generation in the sheepshead minnow (Cyprinodon variegatus) exposed for 4 days to spherical (150–180 μm) or fragmented microsphere (300–355 μm) microspheres of PE (50–250 mg L−1). Moreover, despite flow cytometry analyses did not show significant changes in ROS production between control and exposed organisms, the combined exposure to PE and PP significantly modulated the anti-oxidant enzyme activities, with differential responses between gills and digestive gland. An increase in SOD activity was observed in mussel gills and digestive gland which indicates that after the exposure to PE and PP particles, there is a need to counter the excess of superoxide radical () into the less damaging hydrogen peroxide (H2O2) to prevent cellular oxidative damage. Similar results were observed in mussel M. edulis exposed to MPs of polystyrene (PS) for 14 days at 32 μg L−1 (2 and 6 μm) (Paul-Pont et al., 2016) and in the clam Scrobicularia plana exposed to PS at 1 mg L−1 (20 μm) (Ribeiro et al., 2017).
CAT plays an important role in the defense against oxidative stress through the removal of H2O2 which is the major precursor of hydroxyl radical (Soldatov et al., 2013). In our study, significant increase in CAT activity was observed in both organs suggesting that CAT acts as a defense mechanism against MPs. Previous studies showed an increase of CAT activity in the clam S. plana exposed to PS MPs (Ribeiro et al., 2017) but also an inhibition in the digestive gland of the marine mussels M. galloprovincialis and M. edulis (Avio et al., 2015; Paul-Pont et al., 2016; Ribeiro et al., 2017).
GST is associated with phase II biotransformation process. It allows the conjugation of the reduced form of glutathione (GSH) to xenobiotic compounds. GST also plays a protective role against oxidative stress by detoxification of ROS through GST peroxidase activity (Lesser, 2006). In the present work, a decreased in GST activity was measured in gills and the digestive gland which was also observed in mussels M. edulis exposed to PS at 32 μg L−1 for 7 days (Paul-Pont et al., 2016). The inactivation of GST by other xenobiotics such as metals or pharmaceuticals has been previously reported (Uguz et al., 2003). In the present study, a possible explanation for the decrease in GST activity could be the degradation of the enzyme. However, this involves an oxidation of the thiol groups at the surface of the enzyme which can eventually lead to a lose of enzyme function (Letelier et al., 2006). Furthermore, GSH will be solicited to restore GST's original reduced state (Klaassen and Lu, 2008), but because GSH can be oxidized as well, both GST and GSH can be inactivated, especially when an important ROS production takes place and the system becomes overwhelmed (Barata et al., 2005). Ultimately, GST can be completely inactivated, malfunction, or even ruined. No gene expression alteration was measured which could be link to the duration of exposure since gene responses are more rapid than enzymatic activities to pollutants (Prego-Faraldo et al., 2017).
Differential Response Between Gills and Digestive Glands
In our study, we observed a clear and consistent pattern for SOD and CAT enzymes activity with an increase in both anti-oxidant enzyme activities in digestive glands of mussels exposed to low and medium concentrations of MPs. In gills, a different but also consistent pattern was observed with an increase in both enzyme activities but at the highest tested concentration. The reasons why responses in gills and digestive glands are different for each exposition concentration could be linked to natural differences in physiology of different organs. However, one other hypothesis could be related to the size dispersity of the plastic particles in stock suspensions that were used in the study. When preparing the suspensions of MPs for exposure, a few aggregates were visually detected in the most concentrated stock suspensions (1 and 0.1 mg mL−1) whereas no aggregates were present in the diluted suspension (0.1 μg mL−1). As aggregation is related to the concentration of particles, it can be assumed that particles are less aggregated in the low concentrated suspensions, leading to an exposition where the average size of particles decreases with concentration. It is possible that bigger particles or aggregates present in the highest concentration (100 μg L−1) could have induced an inflammation response in the gills tissue corresponding to the significant increase in SOD and CAT activity measured in gills. The digestive gland is involved in the feeding through digestion of phytoplancton. Since the diluted suspension (0.1 μg mL−1) used to obtain the final concentration of 0.008 μg L−1 contained less, but potentially smaller particles, digestive glands seemed to have been exposed to smallest particles, capable of being filtered by gills (inducing limited or no stress in this organ). This could potentially lead to induction of oxidative stress in digestive glands. However, the degree of responses remained low and longer-term studies should be conducted to clarify the effect of MPs on oxidative stress and the influence of MP size and aggregation status. A previous study conducted in the fish sheepshead minnow (Cyprinodon variegatus) has shown differential impact of MPs on gene expression related to oxidative stress depending on their size and shape (Choi et al., 2018). Few studies investigated the long-term effect of plastic particles with one of them reporting no physiological or cellular changes in the mussel Perna perna exposed for 90 days to PVC particles (Santana et al., 2018). However, another study measured a reduction in feeding rate, body mass, and metabolic rate in Nephrops norvegicus exposed to MP fibers for 8 months (Welden and Cowie, 2016).
Induction of oxidative stress could also have been induced by additives since we used commercial plastics in order to evaluate the toxicity of environmentally relevant plastic particles. In our study, plastics were colored indicating that metals such as cadmium, chromium or lead compounds could have been added as inorganic pigments for coloration (Hahladakis et al., 2018). Previous studies have shown induction of oxidative stress by metal exposures, and also tissue-specific responses to cadmium and lead exposure in bivalves. In green-lipped mussel Perna canaliculus, Chandurvelan et al. (2013) observed an increase in CAT activity in digestive gland but not in gills from mussel exposed to cadmium (200 μg L−1) for 21 and 28 days. Aouini et al. (2018) measured an increase in SOD activity in gills but not in digestive gland from clams Ruditapes philippinarum exposed to lead (10 μg L−1) for 14 days. Tissue-specific responses can be linked to differential bioaccumulation, concentration-dependent or time-dependent patterns (Benito et al., 2017).
Furthermore, results of anti-oxidant enzyme activities in digestive glands of mussels follow a similar pattern as the results of hydrolase AcP in hemolymphe which activity increased in mussels exposed to low (0.008 μg L−1) and medium (10 μg L−1) MPs concentrations.
Limited Impact of Combined Exposure of PE and PP MPs on Immune Parameters and DNA Damage
The measurement of hydrolase AcP activity has been identified as a relevant marker to evaluate the immunity alteration in contaminated environments (Wootton et al., 2003). Increase in AcP activity in mussels exposed to low and medium MP concentrations is an indication of the lysosomes overload leading to the release of hydrolases in extracellular environment. As it was explained in section Differential Response Between Gills and Digestive Glands, the variability in particle size and particle aggregation between the most concentrated stock suspension (few aggregates observed), and the diluted suspension (no aggregates observed) could explain the differential responses between the three tested concentrations. Since aggregation is linked to the concentration of particles, particles that are less aggregated in the diluted suspension could have led to an exposition of MP with a lower average size. When larger particles could be present in the open circulatory system, the smallest particles could interact with hemocytes. Induction of AcP has been already observed in bivalves exposed to different contaminants such as metals and nanomaterials (Buffet et al., 2014).
The comet assay is a rapid and sensitive test enabling the detection of DNA strand breaks and it has been developed for various aquatic organisms including bivalves, gastropods, or fish (Frenzilli et al., 2009). In this study, increase in DNA damage was observed in mussels exposed to the medium and high MP concentration, however the level of DNA damage measured in the hemocytes remained low (below 15% of DNA breaks). Such an effect was already observed in S. plana exposed to MPs PS at 1 mg L−1 (Ribeiro et al., 2017) and M. galloprovincialis exposed to nano-PS at 0.05–50 mg L−1 (Brandts et al., 2018). Since no ROS overproduction was measured in the hemocytes, there is a slight possibility that smaller plastic particles (nanoplatics < 400 nm) interacted with DNA rather than producing ROS and inducing oxidative stress as observed in bivalves exposed to nanoparticles (Katsumiti et al., 2014). Another possibility is that we missed the overproduction of ROS, which may have occurred before the end of the exposure and did induced slight DNA damages.
Conclusion
The impact of a mixture of PE and PP plastic particles in mussels Mytilus spp. exposed at environmentally relevant concentrations were investigated by identifying their presence using infrared spectroscopy, and assessing their effects on organism physiology, tissue structure, and several biomarker responses. Here, we used a mixture of commonly found MPs (PE and PP) from commercially available products with various shapes and a wider size range (<400 μm) than most studies (von Moos et al., 2012; Farrell and Nelson, 2013; Avio et al., 2015; Rainieri et al., 2018). This work shows the difficulty to conduct environmentally relevant laboratory exposure with plastic particles of various sizes and which are very hydrophobic, complicating their dispersion in suspensions. Our results indicate that after exposure to environmental concentrations of MPs, both PE and PP are present in biodeposits confirming the ingestion of MPs by mussels. However, in organisms, MPs were only found in the digestive gland from mussels exposed to the highest concentration. Moreover, an increase in antioxidant enzyme activities was measured with differential responses between tissues (gills, digestive glands) which could be linked to the differential exposure level between tissues due to particle aggregation in stock suspensions. In hemolymph, an increase in AcP was measured for the lowest concentration such as a slight increase in DNA breaks at the medium and highest MP concentrations. Toxicity mechanisms should be further examined. This study brings new results on the potential sublethal effects of MPs, the potential role of particles sizes, and raise questions on their accumulation in marine organisms.
Ethics Statement
The use of mussels Mytilus spp. in laboratory experiments did not require the approval of the ethical committee according to the French regulations.
Author Contributions
CM, MR, FA, FL, and RS designed the outline of the study. MR, HP-E, AC, and MB were involved in the exposure setup and sampling. MR, FA, RS, and JR performed sampling and biological analyses. PD, MR, and BC performed ecophysiology measurements and analysis. KC conducted histopathology evaluation and analysis. MR performed FTIR and FL characterization of MPs. MR, FA, RS, and JR conducted statistical analyses. MR, CM, FL, AC, FA, and RS contributed to the results discussion. MR wrote the manuscript with the contribution of FL, FA, RS, KC, and the revision by all other authors.
Funding
This research is part of the MiPlaqua project which was funded by the region Pays de la Loire (Convention N°2015-10778) who also financed the contribution of MR.
Conflict of Interest Statement
The authors declare that the research was conducted in the absence of any commercial or financial relationships that could be construed as a potential conflict of interest.
Supplementary Material
The Supplementary Material for this article can be found online at: https://www.frontiersin.org/articles/10.3389/fenvs.2019.00033/full#supplementary-material
References
AFNOR (1985). Norme Française. Huitres creuses. Dénominations et Classification, NF V 45-056. Publication de l'association française de normalisation (AFNOR).
Akcha, F., Hubert, F., and Pfhol-Leszkowicz, A. (2003). Potential value of the comet assay and DNA adduct measurement in dab (Limanda limanda) for assessment of in situ exposure to genotoxic compounds. Mutat. Res. (2003) 534, 21–32. doi: 10.1016/S1383-5718(02)00244-9
Aouini, F., Trombini, C., Volland, M., Elcafsi, M., and Blasco, J. (2018). Assessing lead toxicity in the clam Ruditapes philippinarum: bioaccumulation and biochemical responses. Ecotoxicol. Environ. Safe. 158, 193–203. doi: 10.1016/j.ecoenv.2018.04.033
Avio, C. G., Gorbi, S., Milan, M., Benedetti, M., Fattorini, D., d'Errico, G., et al. (2015). Pollutants bioavailability and toxicological risk from microplastics to marine mussels. Environ. Pollut. 198, 211–222. doi: 10.1016/j.envpol.2014.12.021
Barata, C., Varo, I., Navarro, J. C., Arun, S., and Porte, C. (2005). Antioxidant enzyme activities and lipid peroxidation in the freshwater cladoceran Daphnia magna exposed to redox cycling compounds. Comp. Biochem. Physiol. C Toxicol. Pharmacol. 140, 175–186. doi: 10.1016/j.cca.2005.01.013
Barranger, A., Akcha, F., Rouxel, J., Brizard, R., Maurouard, E., Pallud, M., et al. (2014). Study of genetic damage in the Japanese oyster induced by an environmentally-relevant exposure to diuron: evidence of vertical transmission of DNA damage. Aquat. Toxicol. Amst. Neth. 146, 93–104. doi: 10.1016/j.aquatox.2013.10.032
Benito, D., Niederwanger, M., Izagirre, U., Dallinger, R., and Soto, M. (2017). Successive onset of molecular, cellular and tissue-specific responses in midgut gland of Littorina littorea exposed to sub-lethal cadmium concentrations. Int. J. Mol. Sci. 18, 1815. doi: 10.3390/ijms18081815
Berthelin, C. H., Laisney, J., Espinosa, J., Martin, O., Hernandez, G., Mathieu, M., et al. (2001). Storage and reproductive strategy in Crassostrea gigas from two different growing areas (Normandy and the Atlantic coast, France). Invertebr. Reprod. Dev. 40, 79–86. doi: 10.1080/07924259.2001.9652500
Brandts, I., Teles, M., Gonçalves, A. P., Barreto, A., Franco-Martinez, L., Tvarijonaviciute, A., et al. (2018). Effects of nanoplastics on Mytilus galloprovincialis after individual and combined exposure with carbamazepine. Sci. Total Environ. 643, 775–784. doi: 10.1016/j.scitotenv.2018.06.257
Browne, M. A., Dissanayake, A., Galloway, T. S., Lowe, D. M., and Thompson, R. C. (2008). Ingested microscopic plastic translocates to the circulatory system of the mussel, Mytilus edulis (L.). Environ. Sci. Technol. 42, 5026–5031. doi: 10.1021/es800249a
Buffet, P.-E., Zalouk-Vergnoux, A., Châtel, A., Berthet, B., Métais, I., Perrein-Ettajani, H., et al. (2014). A marine mesocosm study on the environmental fate of silver nanoparticles and toxicity effects on two endobenthic species: the ragworm Hediste diversicolor and the bivalve mollusc Scrobicularia plana. Sci. Total Environ. 470–471, 1151–1159. doi: 10.1016/j.scitotenv.2013.10.114
Buisson, S., Bouchart, V., Guerlet, E., Malas, J. P., and Costil, K. (2008). Level of contamination and impact of pesticides in cupped oyster, Crassostrea gigas, reared in a shellfish production area in Normandy (France). J. Environ. Sci. Health B 43, 655–664. doi: 10.1080/03601230802352732
Chandurvelan, R., Marsden, I. D., Gaw, S., and Glover, C. N. (2013). Biochemical biomarker responses of green-lipped mussel, Perna canaliculus, to acute and subchronic waterborne cadmium toxicity. Aquat. Toxicol. 140–141, 303–313. doi: 10.1016/j.aquatox.2013.06.015
Choi, J. S., Jung, Y.-J., Hong, N.-H., Hong, S. H., and Park, J.-W. (2018). Toxicological effects of irregularly shaped and spherical microplastics in a marine teleost, the sheepshead minnow (Cyprinodon variegatus). Mar. Pollut. Bull. 129, 231–240. doi: 10.1016/j.marpolbul.2018.02.039
Claiborne, A. (1985). “Catalase activity,” in Handbook of Methods for Oxygen Radical Research, ed R. A. Greenwald (Boca Raton, FL; London; New York, NY: CRC Press), 283–284.
Cole, M., Lindeque, P., Fileman, E., Halsband, C., Goodhead, R., Moger, J., et al. (2013). Microplastic ingestion by zooplankton. Environ. Sci. Technol. 47, 6646–6655. doi: 10.1021/es400663f
Colton, J. B., Burns, B. R., and Frederick, D., and Knapp (1974). Plastic Particles in surface waters of the Northwestern Atlantic. Science 185, 491–497. doi: 10.1126/science.185.4150.491
Cózar, A., Echevarría, F., González-Gordillo, J. I., Irigoien, X., Úbeda, B., Hernández-León, S., et al. (2014). Plastic debris in the open ocean. Proc. Natl. Acad. Sci. U.S.A. 111, 10239–10244. doi: 10.1073/pnas.1314705111
Desforges, J.-P. W., Galbraith, M., Dangerfield, N., and Ross, P. S. (2014). Widespread distribution of microplastics in subsurface seawater in the NE Pacific Ocean. Mar. Pollut. Bull. 79, 94–99. doi: 10.1016/j.marpolbul.2013.12.035
Duchemin, M. B., Wessel, N., Fournier, M., and Auffret, M. (2008). Flow cytometric measurement of the clearance rate in the blue mussel Mytilus edulis and the development of a new individual exposure system for aquatic immunotoxicological studies. Environ. Pollut. 153, 492–496. doi: 10.1016/j.envpol.2007.08.017
Farrell, P., and Nelson, K. (2013). Trophic level transfer of microplastic: Mytilus edulis (L.) to Carcinus maenas (L.). Environ. Pollut. 177, 1–3. doi: 10.1016/j.envpol.2013.01.046
Frenzilli, G., Nigro, M., and Lyons, B. P. (2009). The Comet assay for the evaluation of genotoxic impact in aquatic environments. Mutat. Res. 681, 80–92. doi: 10.1016/j.mrrev.2008.03.001
Gagnaire, B., Gay, M., Huvet, A., Daniel, J.-Y., Saulnier, D., and Renault, T. (2007). Combination of a pesticide exposure and a bacterial challenge: in vivo effects on immune response of Pacific oyster, Crassostrea gigas (Thunberg). Aquat. Toxicol. 84, 92–102. doi: 10.1016/j.aquatox.2007.06.002
Garen, P., Robert, S., and Bougrier, S. (2004). Comparison of growth of mussel, Mytilus edulis, on longline, pole and bottom culture sites in the Pertuis Breton, France. Aquaculture 232, 511–524. doi: 10.1016/S0044-8486(03)00535-0
Goldstein, M. C., Rosenberg, M., and Cheng, L. (2012). Increased oceanic microplastic debris enhances oviposition in an endemic pelagic insect. Biol. Lett. 8, 817–820. doi: 10.1098/rsbl.2012.0298
Gomes, T., Araújo, O., Pereira, R., Almeida, A. C., Cravo, A., and Bebianno, M. J. (2013). Genotoxicity of copper oxide and silver nanoparticles in the mussel Mytilus galloprovincialis. Mar. Environ. Res. 84, 51–59. doi: 10.1016/j.marenvres.2012.11.009
Haberkorn, H., Lambert, C., Le Goïc, N., Quéré, C., Bruneau, A., Riso, R., et al. (2014). Cellular and biochemical responses of the oyster Crassostrea gigas to controlled exposures to metals and Alexandrium minutum. Aquat. Toxicol. 147, 158–167. doi: 10.1016/j.aquatox.2013.12.012
Habig, W. H., Pabst, M. J., and Jakoby, W. B. (1974). Glutathione S-transferases. The first enzymatic step in mercapturic acid formation. J. Biol. Chem. 249, 7130–7139.
Hahladakis, J. N., Velis, C. A., Weber, W., Iacovidou, E., and Purnell, P. (2018). An overview of chemical additives present in plastics: migration, release, fate and environmental impact during their use, disposal and recycling. J. Haz. Mater. 344, 179–199. doi: 10.1016/j.jhazmat.2017.10.014
Katsumiti, A., Gilliland, D., Arostegui, I., and Cajaraville, M. P. (2014). Cytotoxicity and cellular mechanisms involved in the toxicity of CdS quantum dots in hemocytes and gill cells of the mussel Mytilus galloprovincialis. Aquat. Toxicol. 153, 39–52. doi: 10.1016/j.aquatox.2014.02.003
Klaassen, C. D., and Lu, H. (2008). Xenobiotic transporters: ascribing function from gene knockout and mutation studies. Toxicol. Sci. 101, 186–196. doi: 10.1093/toxsci/kfm214
Lacaze, E., Pédelucq, J., Fortier, M., Brousseau, P., Auffret, M., Budzinski, H., et al. (2015). Genotoxic and immunotoxic potential effects of selected psychotropic drugs and antibiotics on blue mussel (Mytilus edulis) hemocytes. Environ. Pollut. 202, 177–186. doi: 10.1016/j.envpol.2015.03.025
Lenz, R., Enders, K., and Nielsen, T. G. (2016). Microplastic exposure studies should be environmentally realistic. Proc. Natl. Acad. Sci. U.S.A. 113, E4121–E4122. doi: 10.1073/pnas.1606615113
Lesser, M. P. (2006). Oxidative stress in marine environments: biochemistry and physiological ecology. Annu. Rev. Physiol. 68, 253–278. doi: 10.1146/annurev.physiol.68.040104.110001
Letelier, M. E., Martínez, M., González-Lira, V., Faúndez, M., and Aracena-Parks, P. (2006). Inhibition of cytosolic glutathione S-transferase activity from rat liver by copper. Chem. Biol. Interact. 164, 39–48. doi: 10.1016/j.cbi.2006.08.013
Luna-Acosta, A., Bustamante, P., Godefroy, J., Fruitier-Arnaudin, I., and Thomas-Guyon, H. (2010). Seasonal variation of pollution biomarkers to assess the impact on the health status of juvenile Pacific oysters Crassostrea gigas exposed in situ. Environ. Sci. Pollut. Res. 17, 999–1008. doi: 10.1007/s11356-009-0287-1
Mathalon, A., and Hill, P. (2014). Microplastic fibers in the intertidal ecosystem surrounding Halifax Harbor, Nova Scotia. Mar. Pollut. Bull. 81, 69–79. doi: 10.1016/j.marpolbul.2014.02.018
McCord, J. M., and Fridovich, I. (1969). Superoxide dismutase. An enzymic function for erythrocuprein (hemocuprein). J. Biol. Chem. 244, 6049–6055.
Moore, C. J. (2008). Synthetic polymers in the marine environment: a rapidly increasing, long-term threat. Environ. Res. 108, 131–139. doi: 10.1016/j.envres.2008.07.025
NOOA National Oceanic and Atmospheric Administration. (2008). Proceedings of the International Research Workshop on the Occurrence, Effects and Fate of Microplastic Marine Debris. eds C. Arthur, J. Baker, and H. Bamford. Technical Memorandum NOS-OR&R-30. University of Washington Tacoma, Tacoma, WA.
Paul-Pont, I., Lacroix, C., González Fernández, C., Hégaret, H., Lambert, C., Le Goïc, N., et al. (2016). Exposure of marine mussels Mytilus spp. to polystyrene microplastics: toxicity and influence on fluoranthene bioaccumulation. Environ. Pollut. 216, 724–737. doi: 10.1016/j.envpol.2016.06.039
Pernet, F., Tremblay, R., Redjah, I., Sévigny, J.-M., and Gionet, C. (2008). Physiological and biochemical traits correlate with differences in growth rate and temperature adaptation among groups of the eastern oyster Crassostrea virginica. J. Exp. Biol. 211, 969–977. doi: 10.1242/jeb.014639
Pfaffl, M. W. (2001). A new mathematical model for relative quantification in real-time RT–PCR. Nucleic Acids Res. 29, e45. doi: 10.1093/nar/29.9.e45
Phuong, N. N., Zalouk-Vergnoux, A., Kamari, A., Mouneyrac, C., Amiard, F., Poirier, L., et al. (2017). Quantification and characterization of microplastics in blue mussels (Mytilus edulis): protocol setup and preliminary data on the contamination of the French Atlantic coast. Environ. Sci. Pollut. Res. Int. 25, 6135–6144. doi: 10.1007/s11356-017-8862-3
Phuong, N. N., Zalouk-Vergnoux, A., Poirier, L., Kamari, A., Châtel, A., Mouneyrac, C., et al. (2016). Is there any consistency between the microplastics found in the field and those used in laboratory experiments? Environ. Pollut. 211, 111–123. doi: 10.1016/j.envpol.2015.12.035
Plastics Europe (2016). Plastics - the Facts 2016: an Analysis of European Plastics Production, Demand and Waste Data.
Prego-Faraldo, M. V., Vieira, L. R., Eirin-Lopez, J. M., Méndez, J., and Guilhermino, L. (2017). Transcriptional and biochemical analysis of antioxidant enzymes in the mussel Mytilus galloprovincialis during experimental exposures to the toxic dinoflagellate Prorocentrum lima. Mar. Environ. Res. 129, 304–315. doi: 10.1016/j.marenvres.2017.06.009
Rainieri, S., Conlledo, N., Larsen, B. K., Granby, K., and Barranco, A. (2018). Combined effects of microplastics and chemical contaminants on the organ toxicity of zebrafish (Danio rerio). Environ. Res. 162, 135–143. doi: 10.1016/j.envres.2017.12.019
Ribeiro, F., Garcia, A. R., Pereira, B. P., Fonseca, M., Mestre, N. C., Fonseca, T. G., et al. (2017). Microplastics effects in Scrobicularia plana. Mar. Pollut. Bull. 122, 379–391. doi: 10.1016/j.marpolbul.2017.06.078
Ruiz, P., Katsumiti, A., Nieto, J. A., Bori, J., Jimeno-Romero, A., Reip, P., et al. (2015). Short-term effects on antioxidant enzymes and long-term genotoxic and carcinogenic potential of CuO nanoparticles compared to bulk CuO and ionic copper in mussels Mytilus galloprovincialis. Mar. Environ. Res. 111, 107–120. doi: 10.1016/j.marenvres.2015.07.018
Santana, M. F. M., Moreira, F. T., Pereira, C. D. S., Abessa, D. M. S., and Turra, A. (2018). Continuous Exposure to microplastics does not cause physiological effects in the cultivated mussel Perna perna. Arch. Environ. Contam. Toxicol. 74, 594–604. doi: 10.1007/s00244-018-0504-3
Soldatov, A. A., Gostyukhina, O. L., Borodina, A. V., and Golovina, I. V. (2013). Qualitative composition of carotenoids, catalase and superoxide dismutase activities in tissues of the bivalve mollusc Anadara inaequivalvis (Bruguiere, 1789). J. Evol. Biochem. Physiol. 49, 389–398. doi: 10.1134/S0022093013040026
Sul, J. A. I., do, Costa, M. F., and Fillmann, G. (2014). Microplastics in the pelagic environment around oceanic islands of the Western Tropical Atlantic Ocean. Water Air Soil Pollut. 225:2004. doi: 10.1007/s11270-014-2004-z
Sussarellu, R., Suquet, M., Thomas, Y., Lambert, C., Fabioux, C., Pernet, M. E. J., et al. (2016). Oyster reproduction is affected by exposure to polystyrene microplastics. Proc. Natl. Acad. Sci. U.S.A. 113, 2430–2435. doi: 10.1073/pnas.1519019113
Uguz, C., Iscan, M., Ergüven, A., Isgor, B., and Togan, I. (2003). The bioaccumulation of nonyphenol and its adverse effect on the liver of rainbow trout (Onchorynchus mykiss). Environ. Res. 92, 262–270. doi: 10.1016/S0013-9351(03)00033-1
Untergasser, A., Cutcutache, I., Koressaar, T., Ye, J., Faircloth, B. C., Remm, M., et al. (2012). Primer3—new capabilities and interfaces. Nucleic Acids Res. 40, e115. doi: 10.1093/nar/gks596
Utermöhl, H. (1958). Zur Vervollkommnung der quantitativen Phytoplankton-Methodik. Mitteilungen-Int. Ver. Für Limnol. 9, 1–38.
Van Cauwenberghe, L., Claessens, M., Vandegehuchte, M. B., and Janssen, C. R. (2015). Microplastics are taken up by mussels (Mytilus edulis) and lugworms (Arenicola marina) living in natural habitats. Environ. Pollut. 199, 10–17. doi: 10.1016/j.envpol.2015.01.008
Van Cauwenberghe, L., and Janssen, C. R. (2014). Microplastics in bivalves cultured for human consumption. Environ. Pollut. 193, 65–70. doi: 10.1016/j.envpol.2014.06.010
von Moos, N., Burkhardt-Holm, P., and Köhler, A. (2012). Uptake and effects of microplastics on cells and tissue of the blue mussel Mytilus edulis L. after an experimental exposure. Environ. Sci. Technol. 46, 11327–11335. doi: 10.1021/es302332w
Weber, A., Scherer, C., Brennholt, N., Reifferscheid, G., and Wagner, M. (2018). PET microplastics do not negatively affect the survival, development, metabolism and feeding activity of the freshwater invertebrate Gammarus pulex. Environ. Pollut. 234, 181–189. doi: 10.1016/j.envpol.2017.11.014
Wegner, A., Besseling, E., Foekema, E. M., Kamermans, P., and Koelmans, A. A. (2012). Effects of nanopolystyrene on the feeding behavior of the blue mussel (Mytilus edulis L.). Environ. Toxicol. Chem. 31, 2490–2497. doi: 10.1002/etc.1984
Welden, N. A. C., and Cowie, P. R. (2016). Long-term microplastic retention causes reduced body condition in the langoustine, Nephrops norvegicus. Environ. Pollut. 218, 895–900. doi: 10.1016/j.envpol.2016.08.020
Wootton, E. C., Dyrynda, E. A., Pipe, R. K., and Ratcliffe, N. A. (2003). Comparisons of PAH-induced immunomodulation in three bivalve molluscs. Aquat. Toxicol. 65, 13–25. doi: 10.1016/S0166-445X(03)00098-5
Wright, S. L., Rowe, D., Thompson, R. C., and Galloway, T. S. (2013). Microplastic ingestion decreases energy reserves in marine worms. Curr. Biol. 23, R1031–R1033. doi: 10.1016/j.cub.2013.10.068
Keywords: microplastics, polyethylene, polypropylene, Mytilus, oxidative stress, biomarkers
Citation: Revel M, Lagarde F, Perrein-Ettajani H, Bruneau M, Akcha F, Sussarellu R, Rouxel J, Costil K, Decottignies P, Cognie B, Châtel A and Mouneyrac C (2019) Tissue-Specific Biomarker Responses in the Blue Mussel Mytilus spp. Exposed to a Mixture of Microplastics at Environmentally Relevant Concentrations. Front. Environ. Sci. 7:33. doi: 10.3389/fenvs.2019.00033
Received: 28 September 2018; Accepted: 27 February 2019;
Published: 21 March 2019.
Edited by:
Bruno Silva Nunes, University of Porto, PortugalReviewed by:
Bruno B. Castro, University of Minho, PortugalCamilo Dias Seabra Pereira, Federal University of São Paulo, Brazil
Copyright © 2019 Revel, Lagarde, Perrein-Ettajani, Bruneau, Akcha, Sussarellu, Rouxel, Costil, Decottignies, Cognie, Châtel and Mouneyrac. This is an open-access article distributed under the terms of the Creative Commons Attribution License (CC BY). The use, distribution or reproduction in other forums is permitted, provided the original author(s) and the copyright owner(s) are credited and that the original publication in this journal is cited, in accordance with accepted academic practice. No use, distribution or reproduction is permitted which does not comply with these terms.
*Correspondence: Messika Revel, bXJldmVsQHVjby5mcg==; bWVzc2lrYS1yZXZlbEBob3RtYWlsLmZy