- 1Institute of Soil Science, Leibniz Universität Hannover, Hannover, Germany
- 2Soil Science and Soil Protection, Martin Luther University Halle-Wittenberg, Halle, Germany
Nutrient supply in phosphorus (P)-limited ecosystems, with most P being associated with secondary minerals, has to rely on efficient nutrient allocation strategies, such as those involving mycorrhizal symbioses. Yet, little is known about the extent of photo-assimilate transfer to the fungal partner, who in turn mobilizes mineral-bound P sources required by the plant. This study aims to explore the carbon (C)–P trade between an arbuscular mycorrhizal (AM) plant and its ability to incorporate P from differently accessible P sources. We compared P uptake rates of AM plants for orthophosphate (OP) and phytic acid (PA), applied to mesocosms in either dissolved form or bound to goethite (α-FeOOH). The design of the mesocosms allowed the plant to only access the P in the fungal compartment via the AM hyphae. We hypothesized the AM plant to invest more C into the symbiosis, if P is present in the less accessible form. To estimate the C budget of the symbiosis, we determined total organic carbon (OC), 16:1ω5c phospholipid fatty acid (PLFA; AM fungi extraradical mycelium), 16:1ω5c neutral lipid fatty acid (NLFA; AM fungi energy storage), and CO2 cumulative respiration in the fungal compartment. A ratio to the total C translocated into the fungal compartment (OC+CO2-C cumulative respiration) and the P incorporated into the AM plant (Total C/P) was calculated to estimate the C investment made by the AM plant into its symbiotic partner. AM plants incorporated P derived from all four P sources exclusively via the mycorrhizal pathway in different amounts and kinetics. The Total C/P ratio was significantly larger for those AM plants accessing the goethite-bound P compounds. They also transferred significantly larger amounts of PLFA and NLFA to their fungal partner, both indicating a larger plant C investment per P taken up. Our data provide first evidence about the ability of an AM plant to incorporate P from an organic source bound to a secondary mineral. The different C investments of AM plants into P allocation from variably available sources suggests a broad nexus between P mining strategies, resource partitioning in soil, and the amounts of C accumulated in terrestrial soils.
Introduction
Phosphorus (P) is an essential element for plant growth and productivity. While excessive utilization of P as fertilizer has led to widespread eutrophication of inland and coastal waters (Rowe et al., 2016), its deficiency is still a major constraint to agricultural productivity, affecting an estimated area of >20 million km2 worldwide (Oberson et al., 2001). This is especially true for tropical soils where secondary minerals immobilize P to a large extent. In 2014, phosphate rocks were included in the list of the 20 critical raw materials of the European Commission (George et al., 2016), suggesting that this finite resource could be exhausted within this century (Gilbert, 2009). Consequently, all plant strategies which increase P uptake and improve its use-efficiency will be increasingly valuable to prevent P loss to adjacent ecosystems and lower the need of P fertilizers (Smith and Smith, 2011).
Plants can only take up P as free phosphate anions, either as H2 or as (Becquer et al., 2014). Phosphate concentrations in soil solutions span from 1 to 10 μM, being about 1,000-fold smaller than cellular contents in plants, thus are often insufficient for optimal growth (Smith and Smith, 2012). Soil P occurs in forms of varying accessibility to plants with >90% of it being bound in plant-inaccessible forms (Mengel and Kirkby, 2001). Phosphorus occurs either as inorganic phosphates, primarily in associations with Ca, Fe, and Al, or in organic forms. Organic P constitutes 20–80% of total soil P and includes phosphomonoesters such as inositol phosphates (IP), phosphodiesters as ribonucleic acid, deoxyribonucleic acid, lipoteichoic acid, phospholipid fatty acids, and organic polyphosphates, e.g., adenosine triphosphates. The most prevalent phosphomonoester is myo-IP6, which contains six phosphate groups bound to cyclohexane and has nine stereoisomers. The myo-IP6 stereoisomer or phytic acid (PA) is the most common form of inositol phosphates and represents >50% of the organic P in soils (Ognalaga et al., 1994; Nash et al., 2014).
Phosphorus deficiency of plants is caused by strong adsorption of inorganic and organic P to Al and Fe hydroxides and oxides (summarized as “oxides”), making large proportions of total P unavailable to plants (Osorio and Habte, 2001; Javaid, 2009). The specific adsorption and high affinity to soil oxides is greater for the organic P forms (He and Zhu, 1998). As compared to inorganic P forms, the dynamics of organic P species in soils are much less investigated. This is surprising since this fraction is highly relevant to the supply of P to crops in deeply weathered soils like Oxisols (Rodrigues et al., 2016). To overcome soil P limitation, plants developed different strategies to acquire P from soil solution. One strategy is to increase the exchange-surface of the root–soil interface; another strategy lies in solubilizing complexed P by root exudates, such as low-molecular-weight organic acids and phosphatases. The most widespread strategy nevertheless is to rely on symbiotic associations with mycorrhizal fungi and P-solubilizing or organic P-mineralizing bacteria (Richardson et al., 2009; Giles et al., 2012).
In P-deficient environments, e.g., Spodosols, Ultisols, or Oxisols (Yang et al., 2013), selection pressure commonly results in the proliferation of free-living microorganisms and symbiotic associations with mycorrhizal fungi which have the potential to mobilize and mineralize unavailable organic P (Nash et al., 2014). Most terrestrial plants form symbioses with one or more kinds of mycorrhizal fungi. About 80% of plant species are associated with arbuscular mycorrhizal fungi (AMF) (Cairney, 2000; Smith et al., 2008; Johnson, 2010), which enhance the plant's access to limiting belowground resources (Read, 1991).
Plants that establish symbiosis with an AMF can acquire part or almost all of the phosphorus for their metabolic activities, over this pathway (Javot et al., 2007; Willis et al., 2013; Lambers et al., 2015). As they are able to mine soils for P, research on P release rates from secondary minerals is important to improve models on plant nutrient cycling by AMF (Cardoso et al., 2006). Though some studies have shown that mycorrhizal and non-mycorrhizal plants seem to use the same labile P sources (Bolan et al., 1984; García, 2000; Frossard et al., 2011), others demonstrated that mycorrhizal plants obtain P from usually unavailable sources of organic and inorganic P (Rychter et al., 2016). In addition, Bolan et al. (1987) proposed that AMF may cleave the bond between Fe and P and thus release P, but they did not depict the underlying mechanisms.
Turner (2008) suggested that the different soil P species constitute a gradient of biological availability based on the plant's investment to access the phosphate. For example, phytate, being most resistant to hydrolysis, is hypothesized as the metabolically most expensive P source. This makes necessary to understand if there exists a C-P trading in arbuscular mycorrhizal symbiosis.
In return for providing almost all P needed by the AM plant, the fungus receives up to 20% of net plant photosynthates under ambient atmospheric CO2 (Pfeffer et al., 2004). The C assimilates are transported mainly as lipid droplets and glycogen to the AM fungal hyphae (Bago et al., 2002). Recently, three publications have shown that AMF do not have the capacity to produce fatty acids completely on their own (Bravo et al., 2017; Keymer et al., 2017; Luginbuehl et al., 2017). Instead, AMF were found completely depending on plant-derived fatty acids. These studies suggest a model in which a chain length of 16 carbons (C16) b-monoacylglycerol molecules are transported from the root cell through the periarbuscular membrane to the fungus. Fatty acids of chain lengths up to C16 are needed, because the fungus apparently lacks genes encoding multi-domain cytosolic fatty acid synthase subunits. This makes fatty acids a major good of trade between plant and fungus in AM.
To compare the true costs of incorporating different P forms into the plant by the AMF pathway requires the quantification of the C fluxes. The development of AM itself already presents a complex series of trade-offs between the C cost of the fungus and the benefits of enhanced nutrient supply to the plant (Cavagnaro et al., 2008). Quantification of the fluxes in mycorrhiza is one of the most important, yet little explored tasks of mycorrhizal physiology and ecology. In order to identify the behavior of AM in mobilizing P from differently accessible P forms, we carried out a mesocosm experiment under controlled conditions. Our work aimed at (i) clarifying whether the AM plant can take up P from less accessible sources and (ii) to determine whether there exists a trading between C and P over the AM. We hypothesize that less accessible P sources cause larger photoassimilate investments by the plant partner, resulting in differing kinetics of the AMF providing P to the host plant.
Materials and Methods
Plant Growth and Mycorrhization
We selected the AM mycorrhizal association between Solanum lycopersicum L. (var. Moneymaker) x Rhizophagus irregularis (DAOM 197198) for our experiment. The association has been previously investigated to elucidate processes related with the nutritional benefits offered by the AMF to the tomato plant (Nagy et al., 2005; Schaarschmidt et al., 2006; Giovannetti et al., 2012). The reason for using the AMF R. irregularis is due to its global distribution and adaptation to intensive agricultural practices. This ubiquitous occurrence indicates that R. irregularis is compatible with a wide range of soil conditions like pH (5.6–8.0), P availability (0.3–18.8 mg/kg), sand content (17.5–57.0%), and C content (1.0–10.5%) (Köhl et al., 2016). Rhizophagus irregularis DAOM 197198 (syn: Glomus irregulare) recently reassigned from Glomus intraradices Schenck and Smith (Krüger et al., 2012) was used as the mycorrhizal inoculum. It consisted of 0.4 g of spores, hyphae, and root fragments from a Sorghum bicolor trap plant culture (Brundrett, 1996). Seeds of Solanum lycopersicum L. var. Moneymaker (Volmary GmbH) were surface sterilized with 5% H2O2 for 10 min and washed three times with sterile distilled water. Seeds were germinated on petri dishes for 3 days at 27°C. Moneymaker germinated seeds were sowed on 75 ml pots QP96 (HerkuPlast Kubern GmbH) containing the AM inoculum and 70 ml autoclaved acid washed quartz sand, which has been successfully used as plant and fungal growth substrate before (Johansen et al., 1996; Olsson and Johansen, 2000). Allowing the AM mycelium to grow from a colonized root into purified quartz sand, a relatively pure mycelium could be extracted and used for our studies. Mycorrhized and non-mycorrhized control tomato plants were grown in a greenhouse (16/8 light/dark, 24/20°C light/dark, 50–60% relative humidity, photon flux density of 175–230 μmol/m/s). Seedlings were watered every day with 10 ml deionized water and were fertilized with 5 ml low P (0.32 mM) Long Ashton nutrient solution pH 6.5 (Hewitt, 1966) on alternate days. The complete root system of 10 individuals was processed to test for the presence of arbuscular mycorrhiza in the roots of 4 week old plants before transplanting. A root subsample was digested with 10% KOH (35 min, 95°C) and stained using the ink and vinegar staining technique for AMF (Vierheilig et al., 1998b). Stained root fragments were mounted on glass slides and observed at 400 × magnification using an Olympus BH2 microscope (Olympus Optical Company Ltd, Tokyo, Japan), to determine the mycorrhizal status using the methodology from McGonigle et al. (1990), before the plants were transplanted to the mesocosms.
Mesocosm Experiment
Each mesocosms consisted of a plant compartment connected to a fungal compartment in which only the mycelium was able to develop and access the different P sources due to two barriers (Figure 1). Each mesocosms was fabricated from a Nalgene® 250 ml square polypropylene bottle cut at 45° angle. The threaded section of the bottle was glued with Pattex ® hot glue on the side with the largest surface of the bottle base. A second Nalgene®100 ml square polypropylene bottle was cut into two sections, one as irrigation compartment (30 ml) and the second as support for the mesocosms. Through a 3 mm diameter perforation at the base of the plant compartment, a 3 mm diameter fiberglass wick (Ortmann Kapillarbewässerung, Vlotho, Germany) was passed through, leaving 5 cm of the wick inside the irrigation compartment, where twice a week 10 ml deionized water were applied (Supplementary Figure 1). The wick irrigation system operates in a closed cycle, without runoff and covers maximum water requirements of the plant (Son et al., 2006; Kuntz, 2013). At the plant compartment side a polyamide mesh with a pore size of 20 μm and 30 mm side (Franz Eckert GmbH) separated mycorrhizal roots and mycelium (Watkins et al., 1996; Fitter et al., 1998). The second barrier was a 25 mm diameter polytetrafluoroethylene membrane with a pore size of 5–10 μm (Pieper Filter GmbH). The second membrane allowed the AM hyphae to cross but prevented mass flow and diffusion of ions into the plant compartment (Mäder et al., 1993, 2000; Vierheilig et al., 1998a). Hence, P sources were exclusively accessible to the plant by the hyphae. There were no other P sources present in the system as the cultivation substrate in the plant compartment consisted of acid washed quartz sand (1–2 mm) free of nutrients (data not shown). Four-week-old mycorrhized and non-mycorrhized seedlings were transplanted into each mesoscosm and placed in a glasshouse under controlled climatic conditions (24/20°C light/dark; photoperiod 16/8 h light/dark; 50–60% relative humidity; photon flux density of 175–230 μmol/m2/s).
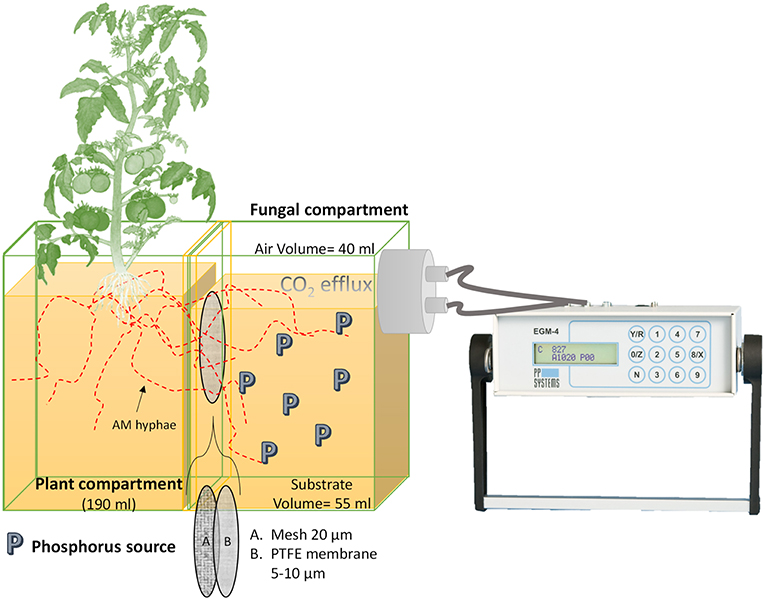
Figure 1. Mesocosm design. The mesocosms is made of a plant compartment (PC) linked to a fungal compartment (FC). Only the AMF is able to access the P sources located in the FC. In between both compartments, two membranes prevent roots from entering the FC, a 20- μm polyamide mesh and a polytetrafluoroethylene (PTFE) membrane with a pore size of 5–10 μm. The PTFE membrane does not allow mass flow and diffusion of ions to the PC. The FC is connected to the CO2 measuring device (EGM-4) allowing its assembly every time a measurement is made.
Once a week all mesocosms were rotated to achieve homogeneous growth conditions and were fertilized twice a week on top of the plant substrate with 5 ml no-P Long Ashton nutrient solution at pH 6.5 (Hewitt, 1966). The P sources offered in the fungal compartment, including the control treatments, are summarized in Table 1. The 55 ml volume of the fungal compartment was amended by a total P amount of 30 mg and water volume was adjusted to field capacity. The water volume at the fungal compartment was determined with a time-domain reflectometry probe Trime Pico connected to a Trime-FM version P2 (Imko Micromodultechnik GmbH, Ettlingen, Germany). The treatments containing quartz sand as a substrate (60 g) in the fungal compartment exhibited 19% water volume while the ones containing goethite (24.3 g) exhibited 41% water volume. The water volume was checked once a week and amended with autoclaved MilliQ water to field capacity. A nylon black cloth with a 1 mm pore was placed on the mouth of the fungal compartment to prevent algae growth, water evaporation and to facilitate gas diffusion to the outside of the fungal compartment (Supplementary Figure 1).
The P uptake and C investment was tracked for 91 days. The first harvest point was on the day of transplanting (day 0) to determine the P stocks of the plants (n = 5), followed by another six sampling points at days 7, 21, 35, 49, 77, and 91. In each of the six harvest points, three biological replicates of each treatment were processed.
Preparation of Phosphorus Sources and Phosphorus Desorption
Each P source was placed inside the fungal compartment as explained in Table 1. The orthophosphate (OP) source was added as KH2PO4 (Sigma Aldrich, Taufkirchen, Germany) and the organic P source was added as phytic acid (PA) sodium salt hydrate (Sigma Aldrich). Two adsorption complexes were produced using the OP and the PA bound to goethite. The adsorption complexes were prepared equilibrating the P compounds with goethite (Bayferrox 920 Z). First, 50 g of goethite were equilibrated for 16 h in 250 ml MilliQ H2O adjusted to pH 4. Then, 250 ml MilliQ H2O containing either 17 g KH2PO4 or 0.7191 g C6H18O24P6 were added to the goethite suspension and equilibrated for 48 h on an overhead shaker. The goethite-P suspensions were centrifuged (3,000 × g, 15 min) and the pellets were subsequently washed with MilliQ H2O until the electric conductivity was <40 μS/cm. The resulting goethite-P associations were shock-frozen in liquid N2, and freeze-dried. The OP and PA bound to the goethite adsorption complexes was determined in triplicate by hydrolysing 5 mg of the goethite-P associations in concentrated HNO3 and subsequent measurement of P contents by ICP-MS Agilent 7500C (Agilent Technologies Ireland Ltd., Cork, Ireland). The adsorption complexes contained 1.24 mg P/g in case of GOE-OP and 1.79 mg P/g for GOE-PA, respectively. A second analysis on the adsorption complexes was carried out to determine the amount of desorbable P, by shaking 1.0 g of each goethite-P association (n = 3) in 30 ml of MilliQ water for 24 h on an overhead shaker. The centrifuged supernatant (3,000 × g, 15 min) was filtered through a 0.45 μm syringe filter (PVDF) and 1 ml was mixed with 1 ml 30% HNO3 and the mixture was filled up to 10 ml with MilliQ water and P content was then measured by ICP-MS.
Plant and Fungal Phosphorus Contents
At each harvest point, shoots and roots were air dried (70°C, 48 h), weighed and ball milled. Plant samples were incinerated at 480°C for 8 h, digested with 1 ml 30% HNO3, filtered and analyzed by ICP-MS. The P stocks were determined for the whole plant. The amount of total P incorporated by the AM plant over time was calculated as the difference of the P stock at each harvest point and the P stock initially present in the transplanted plant on day 0 (determined from five subsamples). The separation of hyphae/spores took place on day 91 following a modified method of Brundrett (1996). First, 4 g of goethite-P associations (GOE-OP, GOE-PA) or 10 g of sand (OP, PA) were sampled from each fungal compartment in 50 ml Falcon test tubes. Tubes were then filled with a fixative solution (0.9 g/l NaCl plus 3% glutaraldehyde), equilibrated for 2 h on an overhead shaker, and then centrifuged (3,000 × g, 15 min). The supernatant was filtered through a polyethersulfone 0.45 μm filter (Supor® PES membrane disc filters, Pall Life Sciences, Hampshire, UK), using a vacuum pump system. Pellets were re-suspended in a 50% sucrose solution by vigorously shaking plus an equilibration step (1 h) on an overhead shaker. The samples were then centrifuged for 15 min at 3,000 × g to facilitate the separation of hyphae and spores in the glucose density gradient. Immediately after centrifugation, hyphae and spores in the sucrose supernatant were poured onto the same polyethersulfone 0.45 μm filter and carefully washed with MilliQ H2O to remove the sucrose and the fixative solution. This step was repeated three times per sample. After rinsing the hyphae and spores, the filters were placed in petri dishes. The hyphae/spores were collected under a stereomicroscope with a glass pipette and deposited in 2 ml Eppendorf tubes. The fungal material was air-dried at 50°C for 96 h. The P content (P mg /hyphae mg) was determined for an aliquot of fungal material. Samples were incinerated at 480°C for 8 h, digested with 1 ml 30% HNO3, filtered, and analyzed by ICP-MS. Additionally, the P stock incorporated into the plant tissues at day 91 was calculated as the percentage of the initial total 30 mg P offered at the fungal compartment, for each P source.
Organic Carbon and Carbon Dioxide Production at the Fungal Compartment
At each harvest point, 2 g from each fungal compartment were air-dried (70°C, 48 h), weighed, and ball milled. The C content of these samples was measured using an Elementar vario MICRO cube C/N analyzer (Elementar GmbH, Hanau, Germany). For the calculation of the C stock in the fungal compartment, it was multiplied the C content (mg/g), determined at the analyzer, by the total weight of substrate in the fungal compartment reported in Table 1. As there was no inorganic C in the fungal compartment, all C measured in the fungal compartment was considered as organic carbon (OC).
The CO2 efflux (mmol CO2 m2 h−1) was measured in the fungal compartment twice a week using an EGM-4 infrared gas analyzer (PP-systems, Hitchin, UK), a close dynamic system (Vermue et al., 2008). At each measuring point, the black cloth was removed, the airtight lid was placed in the mouth of the fungal compartment and the inlet and outlet tubes were connected to the EGM-4 (Supplementary Figure 1). CO2 efflux was automatically calculated for a headspace volume of 40 ml and an exposed area of 0.0012 m2 of the fungal compartment. The CO2 content was measured every 4.8 s and the CO2 flux was measured for at least 3 min or until a good quadratic fit was obtained. Cumulative CO2 production was calculated in mg by interpolation using a cubic spline function (Gentsch et al., 2018) for each fungal compartment.
At each time point, the Total C/P ratio was used to evaluate and estimate the investment made by the AM plant into its symbiotic partner at the fungal compartment per plant P incorporated (Equation 1), since all the C measured at the fungal compartment, in the form of total OC and cumulative CO2-C will be exclusively carried by the AMF.
Where OC is the total C (mg) per fungal compartment; cumulative CO2-C is the total C present in the cumulative respired CO2 (mg) at the fungal compartment.
Fatty Acid Analysis and R. irregularis Biomass Estimation
Using a chloroform-methanol-citrate buffer (1:2:0.8 v/v/v), lipids were extracted twice from 16 g of fungal compartments containing quartz sand or 8 g for the ones with goethite. Thereafter, extracts were fractionated by solid phase extraction with activated Silica gel (Sigma Aldrich, pore size 60 Å, 70–230 mesh) into neutral lipid fatty acids (NLFA), glycolipids, and phospholipid fatty acids (PLFA) by elution with 5 ml of chloroform, 20 ml of acetone, and 20 ml of methanol, respectively. The PLFA and NLFA samples were subjected to mild alkaline methanolysis, which transformed the neutral lipids and the phospholipids into free fatty acid methyl esters, as outlined in Frostegård et al. (1991) with modifications by Bischoff et al. (2016). The methyl esters were then separated by gas chromatography using an Agilent 7890A GC system (Agilent Technologies Ireland Ltd., Cork, Ireland) equipped with a 60 m Zebron capillary GC column (0.25 mm diameter and 0.25 μm film thickness; Phenomenex, Torrance, California, USA) and quantified with a flame ionization detector, using He as carrier gas. Glyceryl tridodecanoate (25 μg) and nonadecanoic acid (25 μg) were used as internal standards during the extraction and tridecanoic acid methyl ester (15 μg) was added to each sample and standard before analysis as a recovery standard. Identification of the fatty acids was achieved by use of the relative retention times, in comparison to that of the internal standard using the GC ChemStation (B.03.02.341) software (Agilent Technologies Ireland Ltd., Cork, Ireland).
The AMF R. irregularis DAOM 197198 model organism (Daubois et al., 2016) has a fatty acid composition ranging from C16:0 to C22:2 with 16:1ω5 as major fatty acid (Aarle and Olsson, 2003; Calonne et al., 2010; Wewer et al., 2014). The PLFA 16:1ω5c can be used for evaluating the amount of extraradical mycelia of AMF, making it a good predictor for the amount of C allocated to AMF (Olsson and Johansen, 2000; Aarle and Olsson, 2003; Marschner, 2007). In addition, the NLFA 16:1ω5c is considered a good predictor on energy storage by the fungus (Bååth, 2003). NLFA are stored in intraradical vesicles, spores, extraradical mycelium and make up a large proportion of the AM fungal biomass. They are metabolized in the mycelium through the glyoxalate cycle, and might provide the major fungal energy source as respiratory substrate (Olsson and Wilhelmsson, 2000; Aarle and Olsson, 2003; Olsson and Johnson, 2005). Therefore, PLFA and NLFA 16:1ω5c were analyzed to assess the biomass and energy storage of AMF in the fungal compartment, respectively (Johansen et al., 1996; Olsson et al., 1997, 2002; Larsen et al., 1998; Stumpe et al., 2005). Ratios of 16:1ω5c PLFA to plant P uptake (Equation 3) and NLFA to plant P uptake (Equation 4), were used to estimate the investment made by the tomato plants into their fungal partner in either biomass or energy storage to obtain P from each source, respectively. The NLFA 16:1ω5c/PLFA 16:1ω5c ratio (Equation 5) was calculated as an index for the growth strategy of R. irregularis (Green et al., 1999; Rinnan and Bååth, 2009). For each P source, the mean NLFA 16:1ω5c/PLFA 16:1ω5c ratio was calculated for sampling points belonging to the periods where no P incorporation was detected in the AM plant tissue as well as for those where we detected P in the AM plant.
A high NLFA 16:1ω5c/PLFA 16:1ω5c ratio indicates preferential C allocation to storage products in form of neutral lipids. Moreover, the ratio identifies the origin of the 16:1ω5c fatty acid. At NLFA 16:1ω5c/PLFA 16:1ω5c > 1, the majority of this fatty acid is considered to originate from AM fungi and not from bacteria (Olsson, 1999; Hammer et al., 2011a; Vestberg et al., 2012; Cozzolino et al., 2016). To estimate the amount of AMF grown in the fungal compartment, we used a conversion factor of 1.2 nmol PLFA 16:1ω5c per mg dry hyphae (Olsson and Johansen, 2000; Olsson and Wilhelmsson, 2000; van Diepen et al., 2010). The estimated total AM biomass was used together with the fungal P content to infer the P stock incorporated by the AMF. Furthermore, we calculated the percentage of the initial total 30 mg P offered at the fungal compartment incorporated into the AMF biomass at day 91, for each P source.
Data Analysis
The normality of the data was verified with the Shapiro-Wilk's test and homogeneity of variances using the Levene's test. One-way ANOVA analysis of the variance and the Tukey post-hoc test (Supplementary Tables 1–6) were employed to test for differences of mean values (P < 0.05) of measured variables between the treatment groups, presented by the different P sources offered in the fungal compartment. Data analysis was performed using SPSS v.24 for Windows (IBM Corporation, 2016).
Results
Phosphorus derived from each offered source was incorporated by the AM tomato plants, while none of the controls (GOE, M+, and M–; Table 1) showed P incorporation (Figure 2). Those AM plants which had access to OP incorporated the highest amount of P, followed by PA, GOE-OP, and GOE-PA. The first P incorporation was detected at day 49 in case of the treatments offering OP and PA and at day 77 in case of GOE-OP and GOE-PA as P sources. At day 91, AM plants reached the maximum P incorporation, with P taken up in the following order: OP (30.4% of the initially added P) > PA (10.4%) > GOE-OP (5.9%) > GOE-PA (2.1%) (Figure 3).
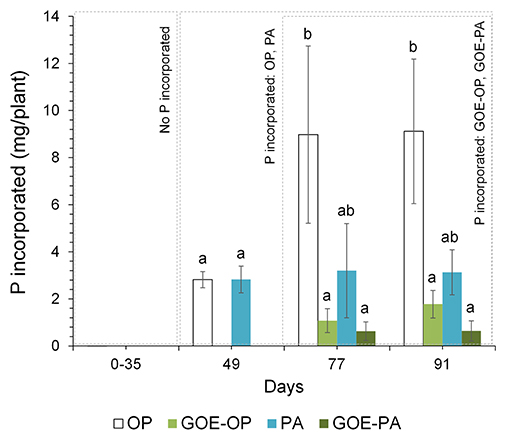
Figure 2. Total P (mg) incorporated by the AM tomato plant from the different P sources over 91 days. Each bar indicates the mean of three biological replicates and error bars are standard error. Within each P source and day, treatments with significant differences are labeled with different letters (p < 0.05) as result of a one-way ANOVA.
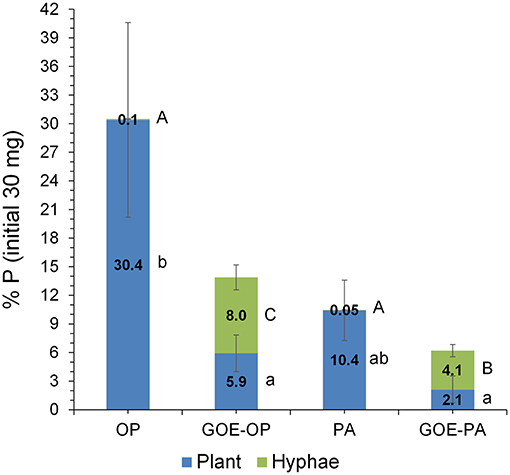
Figure 3. P stocks compartmentalized into plant tissues and external hyphae at day 91 (percentage of the initial total 30 mg P offered at the fungal compartment). Bars indicate the mean of three biological replicates and error bars are standard error. Within each P source and plant/hyphae, treatments with significant differences (P < 0.05) are labeled with different lowercase letters for P% Plant stock and capital letters for P% hyphae stock lowercase as result of a one-way ANOVA.
AM plants with access to OP had significantly larger P contents in their hyphae and spores, followed by plants supplied with GOE-OP and PA. The GOE-PA and M+ (day 0) treatments exhibited significantly smaller P contents in the AMF (Figure 4). Although the hyphal content of P was significantly larger for the fungal compartment containing OP, the total amount of P accumulated in the hyphae was smaller, as there was significantly less AMF biomass. On average, the extraradical hyphae accumulated less P in the fungal compartment containing OP (0.03 mg P; 0.1%) and PA (0.015 mg P; 0.05%) as compared to the goethite-bound forms GOE-OP (2.39 mg P; 8%), and P for GOE-PA (1.22 mg P; 4.1%) (Figure 3). When these P amounts are summed up to the P incorporated by the plant, R. irregularis mobilized 9.15 mg P (30.5% of the initially added P) from the OP, followed by GOE-OP (4.17 mg P; 13.9%), PA (3.14 mg P; 10.5%), and GOE-PA (1.86 mg P; 6.2%) (Figure 3). Compared to that, P desorption from the goethite in water was 7.4% for GOE-OP and 1.2% for GOE-PA. These proportions corresponded to P amounts of 2.2 ± 0.25 mg in the GOE-OP treatment and 0.35 ± 0.008 mg P in the GOE-PA treatment, which likely were mobilized by desorption processes. Hence, the difference between the P incorporated in the plant tissues/AM mycelium and the P desorbed in water of the goethite-bound P sources, resulted in a net P mobilization from the goethite complexes by the AMF between 5% (GOE-PA) and 6.5% (GOE-OP) of the initially added P.
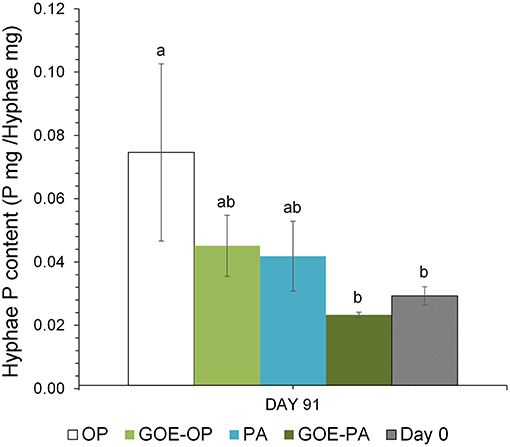
Figure 4. Total P content in the hyphae (P mg/Hyphae mg) grown at the fungal compartment for the different P sources on day 91, when the maximum P mobilization was achieved in the four treatments. Each bar indicates the mean of three biological replicates and error bars are standard error. Within each P source, treatments with significant differences are labeled with different letters (P < 0.05) as result of a one-way ANOVA.
Organic C contents (mg/g fungal compartment) show that the M- control treatment did not accumulate any C throughout the experiment. From day 7 to day 91, fungal compartments containing OP, PA and M+ control showed significantly smaller accumulation of OC compared to GOE-OP, GOE-PA, and GOE control. From day 7 to 77, the fungal compartment containing PA showed significantly larger OC contents than those with OP and control without P (M+) (Figure 5A). The same occurred for fungal compartments containing GOE-PA as compared to those with GOE-OP and GOE control (Figure 5A).
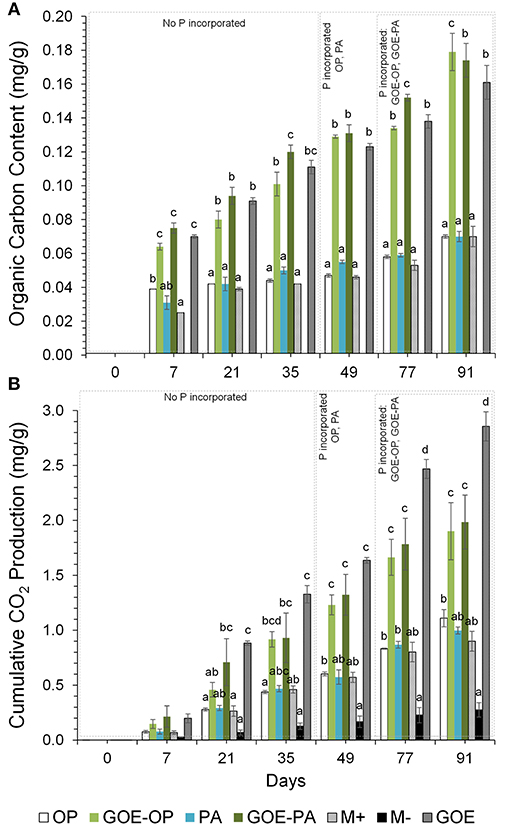
Figure 5. (A) Organic carbon content (mg/g fungal compartment). Each bar indicates the mean of three biological replicates and error bars are standard error. (B) Cumulative CO2 production (mg/g fungal compartment). The bars show the mean of three biological replicates and error bars are standard error. Within each P source and day, treatments with significant differences are labeled with different letters (P < 0.05) as result of a one-way ANOVA.
In case of cumulative CO2 production (mg/g fungal compartment), the M- treatment, without mycorrhiza and without P, showed significantly smaller production than any other treatment (Figure 5B). Fungal compartments containing OP, PA and the M+ control showed significantly smaller cumulative CO2 production from day 7 to 91 as compared to the production of GOE-OP, GOE-PA, and the GOE control. The cumulative CO2 production showed no significant difference between those containing OP, PA and the M+ control. Throughout the experiment, the GOE control showed significantly larger cumulative CO2 production values than any other treatment.
At days 77 and 91, when AM plants reached their maximum P incorporation, the Total C/P was significantly higher for those AM plants that had access to GOE-OP, GOE-PA, and PA, as compared to OP (Figure 6). The P incorporated from GOE-OP showed larger PLFA 16:1ω5c/P ratios than the OP and PA treatments at days 77 and 91, while for GOE-PA this ratio was larger than for OP and PA at day 91 only (Figure 7A). The NLFA 16:1ω5c/P was significantly larger for both P sources bound to goethite in comparison to OP and PA (Figure 7B). For all P sources, the NLFA 16:1ω5c/PLFA 16:1ω5c ratio was above one, meaning that the PLFA and NLFA originated largely from AM fungi (Figure 7C). The NLFA 16:1ω5c/PLFA 16:1ω5c ratios increased for all AM plants accessing a P source in the fungal compartment during the period of P incorporation. The NLFA 16:1ω5c/PLFA 16:1ω5c ratio showed always significant larger values for the treatment offering GOE-OP as P source.
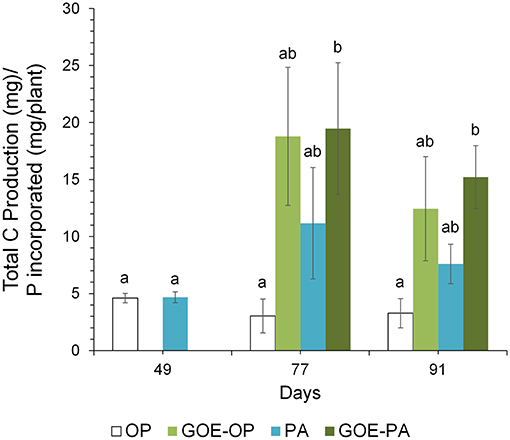
Figure 6. Mean values and standard errors (n = 3) of the total C/P ratio. Within each P source and day, treatments with significant differences are labeled with different letters (P < 0.05) as result of a one-way ANOVA.
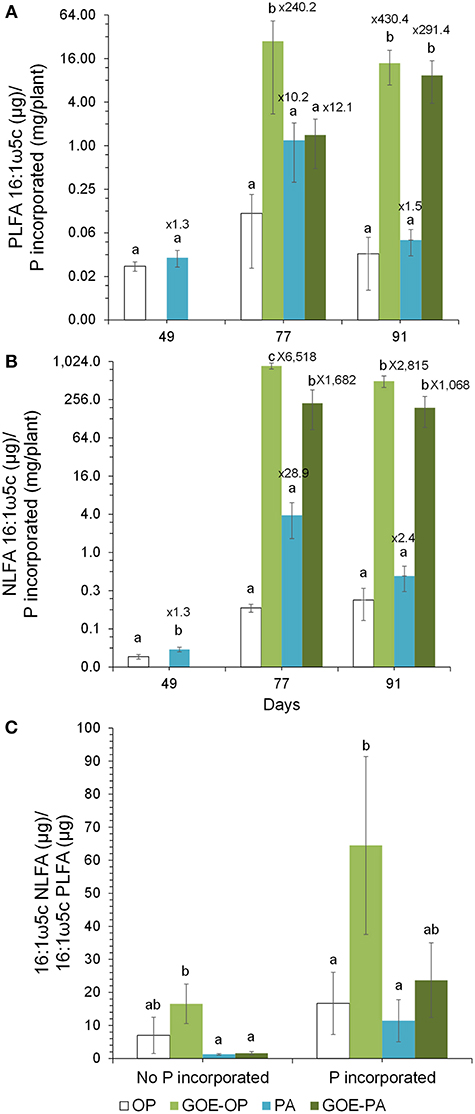
Figure 7. Ratios of PLFA 16:1ω5c to P incorporated within the tomato plant (A) and ratio of NLFA16:1ω5c to P incorporated within the tomato plant (B) from the different P sources along the time course experiment. Shown are mean values and standard errors (n = 3). Within each P source and day, treatments with significant differences are labeled with different letters (P < 0.05) as result of a one-way ANOVA. The numbers on top of each bar represent a multiplication factor compared to the P source with the lower ratio value. In all cases, the P source with the lowest ratios was OP. (C) Ratio of NLFA 16:1ω5c to PLFA 16:1ω5c. Shown are the mean values and standard errors of the NLFA 16:1ω5c/PLFA 16:1ω5c ratio for the sampling points belonging to the periods where no P incorporation was detected in the AM plant tissue as well as for those where we detected P in the AM plant. Within each P source, treatments with significant differences are labeled with different letters (P < 0.05) as result of a one-way ANOVA.
Discussion
Phosphorus Plant Uptake From Different Sources
We tested the role of AMF in taking up P from four different sources having a different accessibility. For this, a mesocosm was designed where exclusively the AM hyphae were involved in plant P acquisition. Our results indicate that all AM plants colonized with R. irregularis incorporated P derived from inorganic (OP) or organic (PA) sources, no matter if added either in their free form or bound to goethite. Phosphorus from the different P forms was taken up in different amounts and kinetics via the mycorrhizal pathway. In contrast to other studies where roots and hyphae are only separated by a 20 μm nylon mesh (Argüello et al., 2016; Jakobsen et al., 2016; Zhang et al., 2016; Sawers et al., 2017), the hydrophobic polytetrafluoroethylene membrane used in our mesocosms avoided not only the direct P uptake by roots, but also the influence of root exudates and the diffusive transport from the fungal compartment to plant compartment, as well as the P absorption by the roots. This membrane type has been successfully used in other studies investigating on nitrogen transfer by AMF (Mäder et al., 1993, 2000; Frey et al., 1998), but not for P nutrition via the mycorrhizal pathway. The hydrophobic and selective nature of this barrier, however, is of paramount importance for separating the effects of the root and the fungus and quantifying the nutrient fluxes. These first results do thus not only give evidence of the importance for mycorrhiza in the uptake of P but also proof the advantage of hydrophobic polytetrafluoroethylene membranes (Figure 1).
Plant P incorporation appeared quickest in OP and PA treatments, followed 1 month later by the P forms bound to goethite. In addition, P from the freely accessible forms of OP and PA was incorporated to a greater extent (Figure 2). The faster plant P uptake in case of OP can be explained by the fact that an AM plant can absorb OP directly via the mycorrhizal pathway (Smith et al., 2003). Usually most of the studies on plant nutrition only consider inorganic phosphate—but not the organic P fraction—as biologically available, even though organic P represents the major part of the total soil P (Turner, 2008). To be available for the plant, PA has to be hydrolyzed first by phytases, a group of phosphatases, which are either of plant or microbial origin (Li et al., 1997; Baldwin et al., 2001, 2008; Javaid, 2009). In our study we did not measure enzymatic activities, but it has been demonstrated that R. irregularis DAOM 197198 is able to secrete acid phosphatase, which contributes to the mineralization of P-bearing organic compounds in soil and thus increases the concentration of available inorganic P (Tisserant et al., 2012). Tarafdar and Marschner (1994a,b) reported the mobilization of organic P sources for plant P uptake by exudation of fungal acid phosphatase in an experiment using Glomus mosseae. Accumulating evidence suggests that Rhizophagus species can hydrolyze organic P forms and the resultant inorganic P can be taken up and transported to host roots (Joner et al., 2000; Koide and Kabir, 2000; Sato et al., 2015). Utilization of organic P is thus assumed to contribute in a comparable manner to AM plant P nutrition as inorganic P (Feng et al., 2003), as we did not find significant differences among the amount of P mobilized from OP and PA.
We also did not find differences in the amount of P incorporated into the plant between the treatments offering GOE-OP and GOE-PA as P sources. Phosphorus from both P-goethite associations was incorporated by the mycorrhizal plant on average less and also later than free OP. Concerning OP, our results support earlier conclusions by Parfitt (1979), who provided the only available study addressing the mobilization of OP adsorbed to goethite. They showed that Lolium perenne mycorrhized with Glomus tenuis desorbed phosphate from goethite after 55 days. However, in this experiment there was no physical separation between the plant and hyphae. For that reason, Parfitt's results should be interpreted with caution, as desorption may be partially caused by the acidification of the rhizosphere mediated by root exudates containing an excess of protons, and not exclusively by the AMF (Hinsinger and Gilkes, 1996). To our knowledge, this is the first experiment proving that an AM plant incorporated P from PA bound to a secondary mineral. Noteworthy, the amounts of mobilized P were larger than the portion of most weakly sorbed PA, as determined in the separate desorption experiment. This finding suggests that AMF play an active role in the mobilization of mineral-bound P. A possible mechanism used by AMF to desorb P from the surface of the goethite is through the release of exudates containing organic acids, as observed by Tawaraya et al. (2006). The delay to incorporate P from GOE-OP and GOE-PA associations may be due to two reasons: firstly, both P forms had to be desorbed from the goethite by means of organic acids; with the P diffusion being likely impaired in the goethite substrate. Secondly, in contrast to the desorbed OP, the released PA had still to be hydrolyzed through the action of phosphatases.
AMF not only supplied P to the plant, but also accumulated it into the hyphae grown in the fungal compartment. On the one hand, the P contents in the AM hyphae were larger for the treatments offering free P forms OP and PA, followed by those treatments offering GOE-OP and GOE-PA as P sources (Figure 4). On the other hand, the P stocks in the fungal biomass for OP and PA were significantly smaller than those determined in GOE-OP and GOE-PA (Figure 3). Thus, the P from the goethite-bound forms was evenly distributed between the fungus and the plant, while the non-bound forms were preferably incorporated into the plant. Our results point to a smaller P content inside the hyphae growing in the fungal compartments with the lees P accessible sources, GOE-OP and GOE-PA. This result would be in accordance with the observations made by Ezawa et al. (2002), who found that the P content in the hyphae appears to respond flexibly to P availability in the environment, varying its internal content proportionally.
Rhizophagus irregularis did not transfer all of the mobilized P from the goethite sources to the plant but stored it within its hyphae, as a potential strategy of the fungus to accumulate P under deficient conditions to keep a well-balanced homeostasis (Solaiman et al., 1999). Another possible interpretation of the P accumulation within the hyphae/spores in a broader sense, could be significant from the perspective of the AM plant in the common mycorrhizal network, where accumulated P indicates a likely support for the growth of a new mycelium and maybe even act as an “entrance fee” for a new colonization (Olsson et al., 2008; Hammer et al., 2011b). Likewise, this accumulation of P would not be a futile waste of energy, due to the fact that P storage within microbial biomass may account for 1–10% (10–100 kg of P/ha) of the total soil P (Richardson, 2001) and may become available to plants once the microbes die (Deubel and Merbach, 2005).
Carbon and Phosphorus Trading
No OC was detected in the fungal compartment for M- control treatment, while treatments containing PA and goethite-P associations showed larger OC contents as compared to those fungal compartments for OP and control M+ (Figure 5A). AMF tends to develop more readily under nutrient-poor conditions than under nutrient-rich or heavily P-fertilized conditions (Bryla and Eissenstat, 2005; Olsson et al., 2010), as would be the case for those fungal compartments where there was GOE, PA and sources of goethite-P. Before any P was incorporated between days 0 and 35, the GOE-OP, GOE-PA and GOE treatments exhibited significantly higher OC contents. The data suggests that OC increased more rapidly in those fungal compartments containing goethite compared to fungal compartments with OP or PA. Supposedly, this is due to a greater investment of photoassimilates via the AMF to mobilize less accessible forms before and during the P acquisition. The organic substances exuded by the AMF may be also well stabilized against fast microbial decomposition, as goethite effectively binds organic molecules via ligand exchange below its point of zero charge (Kaiser and Guggenberger, 2007).
Along with the larger OC contents in the fungal compartment of the AM plants, we detected a larger CO2 production at all sampling points in any of the AM plant treatments, especially those where goethite was present, as for the M- treatment (Figure 5B). The total CO2 production might have been a bit overestimated as we also measured a small CO2 production in the M- treatment, which was likely caused by gas permeability of the two membranes between the plant and the fungal compartment. We also cannot exclude the presence of root CO2 production in treatments with AM plants. However, similar mesocosm systems were used to indirectly estimate CO2 production of extraradical mycelium (Heinemeyer et al., 2006; Nottingham et al., 2010; Karasawa et al., 2012), and different calculations were applied to estimate the contribution of AM extraradical mycelium, such as the difference in total CO2 emission between AM and non-AM fungal compartments (Karasawa et al., 2012). We did not indirectly estimate the CO2 production of the extraradical mycelium as a variable soil nutrient availability is known to demand different amounts of root and respiratory energy (Atkin et al., 2009). In line with Atkin et al. (2009), we could show that AM tomato plants were associated with larger CO2 rates in the fungal compartment compared with M- plants and increased soil CO2 release. The nature of the respired CO2 at the fungal compartment, is the sum of the autotrophic and the heterotrophic respirations. The autotrophic respiration comprises the fraction derived from current photosynthates (Olsson et al., 2005). In our case includes the respiration by mycorrhizal fungi, and likely other microorganisms using C that has been recently fixed by plant photosynthesis, since we inoculated the plants with R. irregularis carrying the microorganisms naturally associated with its hyphosphere. The heterotrophic respiration is due to the decomposition of OC (Kuzyakov and Gavrichkova, 2010) made up mostly of dead hyphae and bacteria, mediated by the microbes present at the fungal compartment. Taking together the results of OC content and CO2 production in the fungal compartment, we can assume that treatments containing less accessible phosphorus sources showed higher C investments into autotrophic and heterotrophic metabolic processes, before and during the incorporation of P in the AM plant. Consequently, the Total C/P ratio was significantly larger in those fungal compartments containing free PA and both P sources bound to goethite (Figure 6). The total C as the sum of total OC and cumulative CO2 accounted for most of the C exclusively carried by the AMF into the fungal compartment. The results point to a greater investment of plant photoassimilates compared to the free OP treatment and controls. Similarly, a more active metabolism for the less accessible P sources was indicated by a larger CO2 production for the same amount of P mobilized from PA, GOE-OP and GOE-PA compared to OP. Respiratory energy is required by the microbiont for constructing new intraradical and extraradical fungal tissue (including reproductive structures), for maintenance and repair of existing fungal tissue and for cellular processes in the fungal tissue associated with the absorption and transfer of nutrients to the host (Bryla and Eissenstat, 2005). The photoassimilate investment into respiration (fast) or structural (slow) C pools in soil has been reported frequently before (Zhu and Miller, 2003), but was not related to a direct nutrient allocation mechanism as in our case. Staddon et al. (2003) suggested AM hyphae do not contribute substantially to C sequestration in soil. In contrast, our results point toward larger C sequestration rates and metabolic activity in the less accessible sources, being consistent with the general line of argumentation of Turner (2008). This energy investment into fungal compartments with less accessible P sources, taken together with more structural C contents found in the respective compartments clearly show that AM fungi are able to adjust to the requirements imposed by a given nutrient source.
Growth of R. irregularis Under Variable Phosphorus Availability
The PLFA 16:1ω5c /P and NLFA 16:1ω5c/P showed a significant larger amount of both fatty acids accumulated into the fungal compartment relative to P uptake, in those fungal compartments containing GOE-OP and GOE-PA compared to OP and PA (Figures 7A,B). The fungal lipids extracted from the fungal compartment indicate a different growth strategy to mobilize P from the goethite-bound compounds compared to the free forms. The AMF grown in those fungal compartment containing GOE-OP and PA accumulated two and three orders of magnitude more PLFA and NLFA, respectively, compared to OP and PA. This observation confirms a larger C investment in energy storage and mycelium per unit of P incorporated into the AM plant. Rhizophagus irregularis grown in the fungal compartment containing GOE-OP accumulated significant more NLFA, as indicated by the ratio NLFA 16:1ω5c/PLFA 16:1ω5c, meaning that the AMF stored energy in the form of neutral lipids before and during the P mobilization, compared to GOE-PA and the free P forms (Figure 7C). One potential explanation for the more pronounced development of fungal infrastructure in the presence of P forms bound to goethite is the need to establish a sufficient extraradical hyphae of R. irregularis to mobilize and transport P more efficiently to the plant (Abbott and Robson, 1982; Joner et al., 2000; Smith et al., 2003). According to Bryla and Eissenstat (2005), mycorrhizas tend to be favored under nutrient-poor conditions, as in case of the goethite-P treatments. The reduced hyphal growth in both fungal compartment containing OP and PA with a high P content indicate that the roots may reduce the C flux to the fungus under larger P availability (Olsson et al., 1997, 2002). It has been suggested that a reduced carbohydrate allocation would be a regulatory saving mechanism by the plant host where there is an excess of phosphate ion (Torres de los Santos et al., 2016; Konvalinková et al., 2017). Bååth (2003) showed the NLFA 16:1ω5c/PLFA 16:1ω5c is a good indicator to express preferential C allocation to storage products, when comparing different nutritional scenarios. Our results corroborate this observation and suggest that the preferential energy storage in the form of neutral lipid could be used to support the extensive growth of R. irregularis mycelium in the goethite systems.
The plant is often considered as being in control of the symbiosis, and this “phytocentric” view is supported by the idea that AM symbiosis is a strategy that could be suppressed by the plants at sufficient P supply. But in natural ecosystems, instead of luxurious P availability, suppression of AM colonization by the plant is not the normal situation (Smith and Smith, 2012), as plants have evolved diverse adaptations to acquire different forms of soil P (Ceulemans et al., 2017). Hammer et al. (2011b) suggest a fungal control mechanism of the P transfer to the plant, and that the C-P exchange between the symbionts is closely linked. Our results, showing a different content and partitioning of C into NLFA and PLFA 16:1ω5c for those R. irregularis grown in treatments with mineral-bound P sources, corroborate that point. This more “mycocentric” view of symbiosis explains the evolutionarily stable mutualistic relationship between these symbionts. If AMF are able to reduce the delivery of P to plants with a limited supply of C (Kiers et al., 2011), they possess an important function to actively increase their fitness by choosing the best plant partner, which in turn could increase selection pressure on the plant species within a community (Hammer et al., 2011b). Under our experimental conditions, where the tomato plants could only acquire the P through the AMF, we observed different trading costs between C for P, distinct P acquisition strategies resulting in differing C costs for the AM plant. Our results show that the mobilization of a certain P source by a plant, comes at differing photoassimilatory costs and, by this, necessarily changes the functional trait of this plant. Thus, we propose this mechanism as a potential driver of nutritional partitioning in plant communities, as described by e.g., Turner (2008).
Concluding Remarks
Our study provided evidence that AM plants are able to mobilize P sources differing in their accessibility. This mobilization happened exclusively via the mycorrhizal pathway at contrasting kinetics and accumulation rates, and was driven by the amount of photoassimilates needed for the mobilization of the P sources. The mobilized P was redistributed between fungus and plant in differing amounts, which again was influenced by the P species. Both P sources adsorbed to goethite (GOE-OP, GOE-PA) facilitated a greater investment of the AM plant into the production of fungal vegetative structures than in case of the free OP and PA. Larger contents of PLFA 16:1ω5c and NLFA 16:1ω5c were observed especially in systems with goethite-associated P, suggesting the accumulation of significantly larger amounts of extraradical hyphae. Further, the lipid accumulation in form of PLFA 16:1ω5c and NLFA 16:1ω5c suggests different growth strategies to mobilize P from the goethite-bound compounds. This is also mirrored by the larger C investment per P incorporated, as compared to OP and PA. Our data also suggest that the C investment by mycorrhized plants into P acquisition from differently available P sources has a direct effect on the amount of C accumulating in soils.
Author Contributions
JB, GG, AA, and RM designed the experiment. AA prepared the plant and fungal material. AA conducted the experiment and analyzed the data. JB, LS, GG, and RM supervised the research. AA wrote the paper with contributions from JB, GG, LS, and RM.
Funding
We want to thank the German Federal Ministry of Education and Research for the funding of this project in the framework of the DFG-RTG 1798 Signaling at the Plant-Soil Interface as well as the DFG priority program SPP 1685 Forest Strategies for limited Phosphorus Resources. The publication of this article was funded by the Open Access fund of Leibniz Universität Hannover.
Conflict of Interest Statement
The authors declare that the research was conducted in the absence of any commercial or financial relationships that could be construed as a potential conflict of interest.
Acknowledgments
We are thankful for the great help and guidance received by Silke Bokeloh, Elke Eichmann-Prusch, Ulrike Pieper, Heike Steffen, and Michael Klatt.
Supplementary Material
The Supplementary Material for this article can be found online at: https://www.frontiersin.org/articles/10.3389/fenvs.2019.00026/full#supplementary-material
References
Aarle, I. M. V., and Olsson, P. A. (2003). fungal lipid accumulation and development of mycelial structures by two arbuscular mycorrhizal fungi fungal lipid accumulation and development of mycelial structures by two arbuscular mycorrhizal fungi. Appl. Environ. Microbiol. 69, 6762–6767. doi: 10.1128/AEM.69.11.6762-6767.2003
Abbott, L. K., and Robson, A. D. (1982). The role of vesicular arbuscular mycorrhizal fungi in agriculture and the selection of fungi for inoculation. Aust. J. Agric. Res. 33, 389–408. doi: 10.1071/AR9820389
Argüello, A., O'Brien, M. J., van der Heijden, M. G. A., Wiemken, A., Schmid, B., and Niklaus, P. A. (2016). Options of partners improve carbon for phosphorus trade in the arbuscular mycorrhizal mutualism. Ecol. Lett. 19, 648–656. doi: 10.1111/ele.12601
Atkin, O. K., Sherlock, D., Fitter, A. H., Jarvis, S., Hughes, J. K., Campbell, C., et al. (2009). Temperature dependence of respiration in roots colonized by arbuscular mycorrhizal fungi. New Phytol. 182, 188–199. doi: 10.1111/j.1469-8137.2008.02727.x
Bååth, E. (2003). The use of neutral lipid fatty acids to indicate the physiological conditions of soil fungi. Microb. Ecol. 45, 373–383. doi: 10.1007/s00248-003-2002-y
Bago, B., Zipfel, W., Williams, R. M., Jun, J., Arreola, R., Lammers, P. J., et al. (2002). Translocation and utilization of fungal storage lipid in the arbuscular mycorrhizal symbiosis. Plant Physiol. 128, 108–124. doi: 10.1104/pp.010466
Baldwin, J. C., Karthikeyan, A. S., Cao, A., and Raghothama, K. G. (2008). Biochemical and molecular analysis of LePS2;1: a phosphate starvation induced protein phosphatase gene from tomato. Planta 228, 273–280. doi: 10.1007/s00425-008-0736-y
Baldwin, J. C., Karthikeyan, A. S., and Raghothama, K. G. (2001). LEPS2, a phosphorus starvation-induced novel acid phosphatase from tomato. Plant Physiol. 125, 728–737. doi: 10.1104/pp.125.2.728
Becquer, A., Trap, J., Irshad, U., Ali, M. A., and Claude, P. (2014). From soil to plant, the journey of P through trophic relationships and ectomycorrhizal association. Front. Plant Sci. 5:548. doi: 10.3389/fpls.2014.00548
Bischoff, N., Mikutta, R., Shibistova, O., Puzanov, A., Reichert, E., Silanteva, M., et al. (2016). Land-use change under different climatic conditions: consequences for organic matter and microbial communities in Siberian steppe soils. Agric. Ecosyst. Environ. 235, 253–264. doi: 10.1016/j.agee.2016.10.022
Bolan, N. S., Robson, A. D., and Barrow, N. J. (1987). Effects of vesicular-arbuscular mycorrhiza on the availability of iron phosphates to plants. Plant Soil 99, 401–410. doi: 10.1007/BF02370885
Bolan, N. S. S., Robson, A. D. D., Barrow, N. J. J., and Aylmore, L. A. G. A. G. (1984). Specific activity of phosphorus in mycorrhizal and non-mycorrhizal plants in relation to the availability of phosphorus to plants. Soil Biol. Biochem. 16, 299–304. doi: 10.1016/0038-0717(84)90023-3
Bravo, A., Brands, M., Wewer, V., Dörmann, P., and Harrison, M. J. (2017). Arbuscular mycorrhiza-specific enzymes FatM and RAM2 fine-tune lipid biosynthesis to promote development of arbuscular mycorrhiza. New Phytol. 214, 1631–1645. doi: 10.1111/nph.14533
Brundrett, M. (1996). Working With Mycorrhizas in Forestry and Agriculture. Canberra: Australian Centre for International Agricultural Research.
Bryla, D. R., and Eissenstat, D. M. (2005). “Respiratory costs of Mycorrhizal Associations,” in Plant Respiration, eds H. Lambers and M. Ribas-Carbo (Berlin; Dordrecht: Springer-Verlag), 207–219.
Cairney, J. W. G. (2000). Evolution of mycorrhiza systems. Naturwissenschaften 87, 467–475. doi: 10.1007/s001140050762
Calonne, M., Fontaine, J., Debiane, D., Laruelle, F., Grandmougin-ferjani, A., and Sahraoui, A. L. (2010). “Propiconazole toxicity on the non-target organism, the arbuscular Mycorrhizal fungus, Glomus irregulare,” in Fungicides, ed O. Carisse (InTech), 325–346. doi: 10.5772/10482
Cardoso, I. M., Boddington, C. L., Janssen, B. H., Oenema, O., and Kuyper, T. W. (2006). Differential access to phosphorus pools of an oxisol by mycorrhizal and nonmycorrhizal maize. Commun. Soil Sci. Plant Anal. 37, 1537–1551. doi: 10.1080/00103620600710074
Cavagnaro, T. R., Langley, A. J., Jackson, L. E., Smukler, S. M., and Koch, G. W. (2008). Growth, nutrition, and soil respiration of a mycorrhiza-defective tomato mutant and its mycorrhizal wild-type progenitor. Funct. Plant Biol. 35:228. doi: 10.1071/FP07281
Ceulemans, T., Bodé, S., Bollyn, J., Harpole, S., Coorevits, K., Peeters, G., et al. (2017). Phosphorus resource partitioning shapes phosphorus acquisition and plant species abundance in grasslands. Nat. Plants 3:16224. doi: 10.1038/nplants.2016.224
Cozzolino, V., Di Meo, V., Monda, H., Spaccini, R., and Piccolo, A. (2016). The molecular characteristics of compost affect plant growth, arbuscular mycorrhizal fungi, and soil microbial community composition. Biol. Fertil. Soils 52, 15–29. doi: 10.1007/s00374-015-1046-8
Daubois, L., Beaudet, D., Hijri, M., and De La Providencia, I. (2016). Independent mitochondrial and nuclear exchanges arising in Rhizophagus irregularis crossed-isolates support the presence of a mitochondrial segregation mechanism Ecological and evolutionary microbiology. BMC Microbiol. 16:11. doi: 10.1186/s12866-016-0627-5
Deubel, A., and Merbach, W. (2005). “Influence of microorganisms on phosphorus bioavailability in soils,” in Microorganisms in Soils: Roles in Genesis and Functions, Vol 3, eds A. Varma and F. Buscot (Berlin; Heidelberg: Springer-Verlag), 177–191.
Ezawa, T., Smith, S. E., and Smith, F. A. P. (2002). P metabolism and transport in AM fungi. Plant Soil 244, 221–230. doi: 10.1023/A:1020258325010
Feng, G., Song, Y. C., Li, X. L., and Christie, P. (2003). Contribution of arbuscular mycorrhizal fungi to utilization of organic sources of phosphorus by red clover in a calcareous soil. Appl. Soil Ecol. 22, 139–148. doi: 10.1016/S0929-1393(02)00133-6
Fitter, A. H., Graves, J. D., Watkins, N. K., Robinson, D., and Scrimgeour, C. (1998). Carbon transfer between plants and its control in networks of arbuscular mycorrhizas. Funct. Ecol. 12, 406–412. doi: 10.1046/j.1365-2435.1998.00206.x
Frey, B., Brunner, I., Christie, P., Wiemken, A., and Mäder, P. (1998). “The use of Polytetrafluoroethylene (PTFE) hydrophobic membranes to study transport of 15N by Mycorrhizal Hyphae,” in Mycorrhiza Manual, ed A. Varma (Berlin: Springer), 151–158.
Frossard, E., Achat, D. L., Bernasconi, S. M., Bünemann, E. K., Fardeau, J.-C., Jansa, J., et al. (2011). “The use of tracers to investigate phosphate cycling in soil-plant systems,” in Phosphorus in Action. Soil Biology, Vol 26, eds E. Bünemann, A. Oberson, and E. Frossard (Berlin; Heidelberg: Springer), 59–91.
Frostegård, Å., Tunlid, A., and Bååth, E. (1991). Microbial biomass measured as total lipid phosphate in soils of different organic content. J. Microbiol. Methods 14, 151–163. doi: 10.1016/0167-7012(91)90018-L
García, G. H. G. C. A. (2000). Behaviour of arbuscular-mycorrhizal fungi on Vigna luteola growth and its effect on the exchangeable (32 P) phosphorus of soil. Biol. Fertil. Soils 31, 232–236. doi: 10.1007/s003740050650
Gentsch, N., Wild, B., Mikutta, R., Capek, P., Diáková, K., Schrumpf, M., et al. (2018). Temperature response of permafrost soil carbon is attenuated by mineral protection. Glob. Chang. Biol. 24, 3401–3415. doi: 10.1111/gcb.14316
George, T. S., Hinsinger, P., and Turner, B. L. (2016). Phosphorus in soils and plants: facing phosphorus scarcity. Plant Soil 401, 1–6. doi: 10.1007/s11104-016-2846-9
Gilbert, N. (2009). Environment: the disappearing nutrient. Nature 461, 716–718. doi: 10.1038/461716a
Giles, C. D., Richardson, A. E., Druschel, G. K., and Hill, J. E. (2012). Organic anion-driven solubilization of precipitated and sorbed phytate improves hydrolysis by phytases and bioavailability to Nicotiana tabacum. Soil Sci. 177, 591–598. doi: 10.1097/SS.0b013e318272f83f
Giovannetti, M., Avio, L., Barale, R., Ceccarelli, N., Cristofani, R., Iezzi, A., et al. (2012). Nutraceutical value and safety of tomato fruits produced by mycorrhizal plants. Br. J. Nutr. 107, 242–251. doi: 10.1017/S000711451100290X
Green, H., Larsen, J., Olsson, P. A., Jensen, D. F., and Jakobsen, I. (1999). Suppression of the biocontrol agent Trichoderma harzianum by mycelium of the arbuscular mycorrhizal fungus Glomus intraradices in root-free soil. Appl. Environ. Microbiol. 65, 1428–1434.
Hammer, E. C., Nasr, H., Pallon, J., Olsson, P. A., and Wallander, H. (2011a). Elemental composition of arbuscular mycorrhizal fungi at high salinity. Mycorrhiza 21, 117–129. doi: 10.1007/s00572-010-0316-4
Hammer, E. C., Pallon, J., Wallander, H., and Olsson, P. A. (2011b). Tit for tat? a mycorrhizal fungus accumulates phosphorus under low plant carbon availability. FEMS Microbiol. Ecol. 76, 236–244. doi: 10.1111/j.1574-6941.2011.01043.x
He, Z., and Zhu, J. U. N. (1998). Microbial utilization and transformation of phosphate adsorbed by variable charge minerals. Soil Biol. Biochem. 30, 917–923. doi: 10.1016/S0038-0717(97)00188-0
Heinemeyer, A., Ineson, P., Ostle, N., and Fitter, A. H. (2006). Respiration of the external mycelium in the arbuscular mycorrhizal symbiosis shows strong dependence on recent photosynthates and acclimation to temperature. New Phytol. 171, 159–170. doi: 10.1111/j.1469-8137.2006.01730.x
Hewitt, E. J. (1966). Sand and Water Culture Methods Used in the Study of Plant Nutrition. Kent: University of Bristol Agricultural and Horticultural Research Station, Bristol.
Hinsinger, P., and Gilkes, R. J. (1996). Mobilization of phosphate from phosphate rock and alumina-sorbed phosphate by the roots of ryegrass and clover as related to rhizosphere pH. Eur. J. Soil Sci. 47, 533–544. doi: 10.1111/j.1365-2389.1996.tb01853.x
Jakobsen, I., Smith, S. E., Smith, F. A., Watts-Williams, S. J., Clausen, S. S., and Grønlund, M. (2016). Plant growth responses to elevated atmospheric CO 2 are increased by phosphorus sufficiency but not by arbuscular mycorrhizas. J. Exp. Bot. 67, 6173–6186. doi: 10.1093/jxb/erw383
Javaid, A. (2009). Arbuscular mycorrhizal mediated nutrition in plants. J. Plant Nutr. 32, 1595–1618. doi: 10.1080/01904160903150875
Javot, H., Pumplin, N., and Harrison, M. J. (2007). Phosphate in the arbuscular mycorrhizal symbiosis: transport properties and regulatory roles. Plant Cell Environ. 30, 310–322. doi: 10.1111/j.1365-3040.2006.01617.x
Johansen, A., Finlay, R. D., and Olsson, P. A. (1996). Nitrogen metabolism of external hyphae of the arbuscular mycorrhizal fungus Glomus intraradices. New Phytol. 133, 705–712. doi: 10.1111/j.1469-8137.1996.tb01939.x
Johnson, N. C. (2010). Resource stoichiometry elucidates the structure and function of arbuscular mycorrhizas across scales. New Phytol. 185, 631–647. doi: 10.1111/j.1469-8137.2009.03110.x
Joner, E. J., Ravnskov, S., and Jakobsen, I. (2000). Arbuscular mycorrhizal phosphate transport under monoxenic conditions using radio-labelled inorganic and organic phosphate. Biotechnol. Lett. 22, 1705–1708. doi: 10.1023/A:1005684031296
Kaiser, K., and Guggenberger, G. (2007). Sorptive stabilization of organic matter by microporous goethite: sorption into small pores vs. surface complexation. Eur. J. Soil Sci. 58, 45–59. doi: 10.1111/j.1365-2389.2006.00799.x
Karasawa, T., Hodge, A., and Fitter, A. H. (2012). Growth, respiration and nutrient acquisition by the arbuscular mycorrhizal fungus Glomus mosseae and its host plant Plantago lanceolata in cooled soil. Plant Cell Environ. 35, 819–828. doi: 10.1111/j.1365-3040.2011.02455.x
Keymer, A., Pimprikar, P., Wewer, V., Huber, C., Brands, M., Bucerius, S. L., et al. (2017). Lipid transfer from plants to arbuscular mycorrhiza fungi. Elife 6:e29107. doi: 10.7554/eLife.29107
Kiers, E. T., Duhamel, M., Beesetty, Y., Mensah, J. A., Franken, O., Verbruggen, E., et al. (2011). Reciprocal rewards stabilize cooperation in the mycorrhizal symbiosis. Science 333, 880–882. doi: 10.1126/science.1208473
Köhl, L., Lukasiewicz, C. E., and Van der Heijden, M. G. A. (2016). Establishment and effectiveness of inoculated arbuscular mycorrhizal fungi in agricultural soils. Plant Cell Environ. 39, 136–146. doi: 10.1111/pce.12600
Koide, R. T., and Kabir, Z. (2000). Extraradical hyphae of the mycorrhizal fungus Glomus intraradices can hydrolyse inorganic phosphate. New Phytol. 148, 511–517. doi: 10.1046/j.1469-8137.2000.00776.x
Konvalinková, T., Püschel, D., Rezáčová, V., Gryndlerová, H., and Jansa, J. (2017). Carbon flow from plant to arbuscular mycorrhizal fungi is reduced under phosphorus fertilization. Plant Soil 419, 1–15. doi: 10.1007/s11104-017-3350-6
Krüger, M., Krüger, C., Walker, C., Stockinger, H., Schüßler, A., Krüger, M., et al. (2012). Phylogenetic reference data for systematics and phylotaxonomy of arbuscular mycorrhizal fungi from phylum to species level. New Phytol. 193, 970–984. doi: 10.1111/j.1469-8137.2011.03962.x
Kuntz, L. B. (2013). Wick Irrigation Systems for Subsistence Farming. Available online at: https://dspace.mit.edu/handle/1721.1/83726 (Accessed Oct 21, 2018).
Kuzyakov, Y., and Gavrichkova, O. (2010). Time lag between photosynthesis and carbon dioxide efflux from soil: a review of mechanisms and controls. Glob. Chang. Biol. 16, 3386–3406. doi: 10.1111/j.1365-2486.2010.02179.x
Lambers, H., Martinoia, E., and Renton, M. (2015). Plant adaptations to severely phosphorus-impoverished soils. Curr. Opin. Plant Biol. 25, 23–31. doi: 10.1016/j.pbi.2015.04.002
Larsen, J., Olsson, P. A., and Jakobsen, I. (1998). The use of fatty acid signatures to study mycelial interactions between the arbuscular mycorrhizal fungus Glomus intraradices and the saprotrophic fungus Fusarium culmorum in root-free soil. Mycol. Res. 102, 1491–1496. doi: 10.1017/S0953756298006558
Li, M., Osaki, M., Honma, M., and Tadano, T. (1997). Purification and characterization of phytase induced in tomato roots under phosphorus-deficient conditions. Soil Sci. Plant Nutr. 43, 179–190. doi: 10.1080/00380768.1997.10414726
Luginbuehl, L. H., Menard, G. N., Kurup, S., Van Erp, H., Radhakrishnan, G. V., Breakspear, A., et al. (2017). Fatty acids in arbuscular mycorrhizal fungi are synthesized by the host plant. Science 356, 1175–1178. doi: 10.1126/science.aan0081
Mäder, P., Vierheilig, H., Alt, M., and Wiemken, A. (1993). Boundaries between soil compartments formed by microporous hydrophobic membranes (GORE-TEXR) can be crossed by vesicular-arbuscular mycorrizal fungi but not by ions in the soil solution. Plant Soil 152, 201–206. doi: 10.1007/BF00029089
Mäder, P., Vierheilig, H., Streitwolf-Engel, R., Boller, T., Frey, B., Christie, P., et al. (2000). Transport of 15N from a soil compartment separated by a polytetrafluoroethylene membrane to plant roots via the hyphae of arbuscular mycorrhizal fungi. New Phytol. 146, 155–161. doi: 10.1046/j.1469-8137.2000.00615.x
Marschner, P. (2007). “Soil microbial community structure and function assessed by FAME, PLFA and DGGE — advantages and limitations,” in Advanced Techniques in Soil Microbiology, eds A. Varma and R. Oelmüller (Berlin: Springer), 181–200.
McGonigle, T. P., Miller, M. H., Evans, D. G., Fairchild, G. L., and Swan, J. A. (1990). A new method which gives an objective measure of colonization of roots by vesicular- arbuscular mycorrhizal fungi. New Phytol. 115, 495–501. doi: 10.1111/j.1469-8137.1990.tb00476.x
Mengel, K., and Kirkby, E. A. (2001). “Phosphorus,” in Principles of Plant Nutrition, eds. K. Mengel, E. A. Kirkby, H. Kosegarten, and T. Appel (Dordrecht: Springer), 453–479. doi: 10.1007/978-94-010-1009-2
Nagy, R., Karandashov, V., Chague, V., Kalinkevich, K., Tamasloukht, M., Xu, G., et al. (2005). The characterization of novel mycorrhiza-specific phosphate transporters from and Solanum tuberosum uncovers functional redundancy in symbiotic phosphate transport in solanaceous species. Plant J. 42, 236–250. doi: 10.1111/j.1365-313X.2005.02364.x
Nash, D. M., Haygarth, P. M., Turner, B. L., Condron, L. M., McDowell, R. W., Richardson, A. E., et al. (2014). Using organic phosphorus to sustain pasture productivity: a perspective. Geoderma 221–222, 11–19. doi: 10.1016/j.geoderma.2013.12.004
Nottingham, A. T., Turner, B. L., Winter, K., van der Heijden, M. G. A., and Tanner, E. V. J. (2010). Arbuscular mycorrhizal mycelial respiration in a moist tropical forest. New Phytol. 186, 957–967. doi: 10.1111/j.1469-8137.2010.03226.x
Oberson, A., Friesen, D. K., Rao, I. M., Bühler, S., Frossard, E., Buhler, S., et al. (2001). Phosphorus transformations in an oxisol under contrasting land-use systems: the role of the soil microbial biomass. Plant Soil 237, 197–210. doi: 10.1023/A:1013301716913
Ognalaga, M., Frossard, E., and Thomas, F. (1994). Glucose-1-phosphate and myo-inositol hexaphosphate adsorption mechanisms on goethite. Soil Sci. Soc. Am. J. 58, 332–337. doi: 10.2136/sssaj1994.03615995005800020011x
Olsson, P., Linder, S., Giesler, R., and Högberg, P. (2005). Fertilization of boreal forest reduces both autotrophic and heterotrophic soil respiration. Glob. Chang. Biol. 11, 1745–1753. doi: 10.1111/j.1365-2486.2005.001033.x
Olsson, P. A. (1999). Signature fatty acids provide tools for determination of the distribution and interactions of mycorrhizal fungi in soil. FEMS Microbiol. Ecol. 29, 303–310. doi: 10.1111/j.1574-6941.1999.tb00621.x
Olsson, P. A., Bååth, E., and Jakobsen, I. (1997). Phosphorus effects on the mycelium and storage structures of an arbuscular mycorrhizal fungus as studied in the soil and roots by analysis of fatty acid signatures. Appl. Environ. Microbiol. 63, 3531–3538.
Olsson, P. A., Hammer, E. C., Wallander, H., and Pallon, J. (2008). Phosphorus availability influences elemental uptake in the mycorrhizal fungus Glomus intraradices, as revealed by particle-induced X-ray emission analysis. Appl. Environ. Microbiol. 74, 4144–4148. doi: 10.1128/AEM.00376-08
Olsson, P. A., and Johansen, A. (2000). Lipid and fatty acid composition of hyphae and spores of arbuscular mycorrhizal fungi at different growth stages. Mycol. Res. 104, 429–434. doi: 10.1017/S0953756299001410
Olsson, P. A., and Johnson, N. C. (2005). Tracking carbon from the atmosphere to the rhizosphere. Ecol. Lett. 8, 1264–1270. doi: 10.1111/j.1461-0248.2005.00831.x
Olsson, P. A., Rahm, J., and Aliasgharzad, N. (2010). Carbon dynamics in mycorrhizal symbioses is linked to carbon costs and phosphorus benefits. FEMS Microbiol. Ecol. 72, 125–131. doi: 10.1111/j.1574-6941.2009.00833.x
Olsson, P. A., van Aarle, I. M., Allaway, W. G., Ashford, A. E. A. E., and Rouhier, H. (2002). Phosphorus effects on metabolic processes in monoxenic arbuscular mycorrhiza cultures. Plant Physiol. 130, 1162–1171. doi: 10.1104/pp.009639
Olsson, P. A., and Wilhelmsson, P. (2000). The growth of external AM fungal mycelium in sand dunes and in experimental systems. Plant Soil 226, 161–169. doi: 10.1023/A:1026565314345
Osorio, N. W., and Habte, M. (2001). Synergistic influence of an arbuscular mycorrhizal fungus and a P solubilizing fungus on growth and P uptake of Leucaena leucocephala in an Oxisol. Arid L. Res. Manag. 15, 263–274. doi: 10.1080/15324980152119810
Parfitt, R. L. (1979). The availability of P from phosphate-goethite bridging complexes. desorption and uptake by ryegrass. Plant Soil 53, 55–65. doi: 10.1007/BF02181879
Pfeffer, P. E., Douds, D. D., Bücking, H., Schwartz, D. P., and Shachar-Hill, Y. (2004). The fungus does not transfer carbon to or between roots in an arbuscular mycorrhizal symbiosis. New Phytol. 163, 617–627. doi: 10.1111/j.1469-8137.2004.01152.x
Read, D. J. (1991). “Mycorrhizas in ecosystems - Nature's response to the ‘Law of the minimum,”’ in Frontiers in Mycology, ed D. L. Hawksworth (Regensburg: CAB International), 101–130.
Richardson, A. E. (2001). Prospects for using soil microorganisms to improve the acquisition of phosphorus by plants. Aust. J. Plant Physiol. 28, 897–906. doi: 10.1071/PP01093
Richardson, A. E., Hocking, P. J., Simpson, R. J., and George, T. S. (2009). Plant mechanisms to optimise access to soil phosphorus. Crop Pasture Sci. 60, 124–143. doi: 10.1071/CP07125
Rinnan, R., and Bååth, E. (2009). Differential utilization of carbon substrates by bacteria and fungi in tundra soil. Appl. Environ. Microbiol. 75, 3611–3620. doi: 10.1128/AEM.02865-08
Rodrigues, M., Pavinato, P. S., Withers, P. J. A., Teles, A. P. B., and Herrera, W. F. B. (2016). Legacy phosphorus and no tillage agriculture in tropical oxisols of the Brazilian savanna. Sci. Total Environ. 542, 1050–1061. doi: 10.1016/j.scitotenv.2015.08.118
Rowe, H., Withers, P. J. A., Baas, P., Chan, N. I., Doody, D., Holiman, J., et al. (2016). Integrating legacy soil phosphorus into sustainable nutrient management strategies for future food, bioenergy and water security. Nutr. Cycl. Agroecosyst. 104, 393–412. doi: 10.1007/s10705-015-9726-1
Rychter, A., Rao, I., and Cardoso, J. (2016). “Role of phosphorus in photosynthetic carbon assimilation and partitioning,” in Handbook of Photosynthesis, ed M. Pessarakli, 603–625.
Sato, T., Ezawa, T., Cheng, W., and Tawaraya, K. (2015). Release of acid phosphatase from extraradical hyphae of arbuscular mycorrhizal fungus rhizophagus clarus. Soil Sci. Plant Nutr. 61, 269–274. doi: 10.1080/00380768.2014.993298
Sawers, R. J. H., Svane, S. F., Quan, C., Grønlund, M., Wozniak, B., Gebreselassie, M. N., et al. (2017). Phosphorus acquisition efficiency in arbuscular mycorrhizal maize is correlated with the abundance of root-external hyphae and the accumulation of transcripts encoding PHT1 phosphate transporters. New Phytol. 214, 632–643. doi: 10.1111/nph.14403
Schaarschmidt, S., Roitsch, T., and Hause, B. (2006). Arbuscular mycorrhiza induces gene expression of the apoplastic invertase LIN6 in tomato (Lycopersicon esculentum) roots. J. Exp. Bot. 57, 4015–4023. doi: 10.1093/jxb/erl172
Smith, F. A., and Smith, S. E. (2011). What is the significance of the arbuscular mycorrhizal colonisation of many economically important crop plants? Plant Soil 348, 63–79. doi: 10.1007/s11104-011-0865-0
Smith, S. E., Read, D. J., Kiers, E. T., Duhamel, M., Beesetty, Y., et al. (2008). “The symbionts forming VA mycorrhizas,” in Mycorrhizal Symbiosis, eds S. E. Smith and D. J. Read (New York, NY: Academic Press), 11–I. doi: 10.1016/b978-012652840-4/50002-4
Smith, S. E., and Smith, F. A. (2012). Fresh perspectives on the roles of arbuscular mycorrhizal fungi in plant nutrition and growth. Mycologia 104, 1–13. doi: 10.3852/11-229
Smith, S. E., Smith, F. A., and Jakobsen, I. (2003). Mycorrhizal fungi can dominate phosphate supply to plants irrespective of growth responses. Plant Physiol. 133, 16–20. doi: 10.1104/pp.103.024380
Solaiman, M. Z., Ezawa, T., Kojima, T., and Saito, M. (1999). Polyphosphates in intraradical and extraradical hyphae of an arbuscular mycorrhizal fungus, Gigaspora margarita. Appl. Environ. Microbiol. 65, 5604–5606.
Son, J. E., Oh, M. M., Lu, Y. J., Kim, K. S., and Giacomelli, G. A. (2006). Nutrient-flow wick culture system for potted plant production: system characteristics and plant growth. Sci. Hortic. 107, 392–398. doi: 10.1016/j.scienta.2005.11.001
Staddon, P. L., Ramsey, C. B., Ostle, N., Ineson, P., and Fitter, A. H. (2003). Rapid turnover of hyphae of mycorrhizal fungi determined by AMS microanalysis of 14C. Science 300, 1138–1140. doi: 10.1126/science.1084269
Stumpe, M., Carsjens, J.-G. G., Stenzel, I., Göbel, C., Lang, I., Pawlowski, K., et al. (2005). Lipid metabolism in arbuscular mycorrhizal roots of Medicago truncatula. Phytochemistry 66, 781–791. doi: 10.1016/j.phytochem.2005.01.020
Tarafdar, J. C., and Marschner, H. (1994a). Efficiency of VAM hyphae in utilisation of organic phosphorus by wheat plants. Soil Sci. Plant Nutr. 40, 593–600. doi: 10.1080/00380768.1994.10414298
Tarafdar, J. C., and Marschner, H. (1994b). Phosphatase activity in the rhizosphere and hyphosphere of VA mycorrhizal wheat supplied with inorganic and organic phosphorus. Soil Biol. Biochem. 26, 387–395. doi: 10.1016/0038-0717(94)90288-7
Tawaraya, K., Naito, M., and Wagatsuma, T. (2006). Solubilization of insoluble inorganic phosphate by hyphal exudates of arbuscular mycorrhizal fungi. J. Plant Nutr. 29, 657–665. doi: 10.1080/01904160600564428
Tisserant, E., Kohler, A., Dozolme-Seddas, P., Balestrini, R., Benabdellah, K., Colard, A., et al. (2012). The transcriptome of the arbuscular mycorrhizal fungus Glomus intraradices (DAOM 197198) reveals functional tradeoffs in an obligate symbiont. New Phytol. 193, 755–769. doi: 10.1111/j.1469-8137.2011.03948.x
Torres de los Santos, R., Molinero Rosales, N., Ocampo, J. A., and García-Garrido, J. M. (2016). Ethylene alleviates the suppressive effect of phosphate on arbuscular mycorrhiza formation. J. Plant Growth Regul. 35, 611–617. doi: 10.1007/s00344-015-9570-1
Turner, B. L. (2008). Resource partitioning for soil phosphorus: a hypothesis. J. Ecol. 96, 698–702. doi: 10.1111/j.1365-2745.2008.01384.x
van Diepen, L. T. A., Lilleskov, E. A., Pregitzer, K. S., and Miller, R. M. (2010). Simulated nitrogen deposition causes a decline of intra- and extraradical abundance of arbuscular mycorrhizal fungi and changes in microbial community structure in northern hardwood forests. Ecosystems 13, 683–695. doi: 10.1007/s10021-010-9347-0
Vermue, E., Elbers, J., and Hoosbeek, M. (2008). A Comparative Field Study of Four Soil Respiration Systems. Available online at: http://edepot.wur.nl/120659 (Accessed Oct 25, 2018).
Vestberg, M., Palojarvi, A., Pitkanen, T., Kaipainen, S., Puolakka, E., and Keskitalo, M. (2012). Neutral lipid fatty acid analysis is a sensitive marker for quantitative estimation of arbuscular mycorrhizal fungi in agricultural soil with crops of different mycrotrophy. Agric. Food Sci. 21, 12–27. doi: 10.23986/afsci.4996
Vierheilig, H., Alt-Hug, M., Engel-Streitwolf, R., Mäder, P., and Wiemken, A. (1998a). Studies on the attractional effect of root exudates on hyphal growth of an arbuscular mycorrhizal fungus in a soil compartment-membrane system. Plant Soil 203, 137–144. doi: 10.1023/A:1004329919005
Vierheilig, H., Coughlan, A. P., Wyss, U., and Piché, Y. (1998b). Ink and vinegar, a simple staining technique for arbuscular-mycorrhizal fungi. Appl. Environ. Microbiol. 64, 5004–5007.
Watkins, N. K., Fitter, A. H., Graves, J. D., and Robinson, D. (1996). Carbon transfer between C3 and C4 plants linked by a common mycorrhizal network, quantified using stable carbon isotopes. Soil Biol. Biochem. 28, 471–477. doi: 10.1016/0038-0717(95)00189-1
Wewer, V., Brands, M., and Dörmann, P. (2014). Fatty acid synthesis and lipid metabolism in the obligate biotrophic fungus Rhizophagus irregularis during mycorrhization of Lotus japonicus. Plant J. 79, 398–412. doi: 10.1111/tpj.12566
Willis, A., Rodrigues, B. F., and Harris, P. J. C. (2013). The ecology of arbuscular mycorrhizal fungi. Crit. Rev. Plant Sci. 32, 1–20. doi: 10.1080/07352689.2012.683375
Yang, X., Post, W. M., Thornton, P. E., and Jain, A. (2013). The distribution of soil phosphorus for global biogeochemical modeling. Biogeosciences 10, 2525–2537. doi: 10.5194/bg-10-2525-2013
Zhang, L., Xu, M., Liu, Y., Zhang, F., Hodge, A., and Feng, G. (2016). Carbon and phosphorus exchange may enable cooperation between an arbuscular mycorrhizal fungus and a phosphate-solubilizing bacterium. New Phytol. 210, 1022–1032. doi: 10.1111/nph.13838
Keywords: goethite, organic phosphorus, inorganic phosphorus, arbuscular mycorrhiza, carbon-phosphorus trading, PLFA 16:1ω5c, NLFA 16:1ω5c
Citation: Andrino A, Boy J, Mikutta R, Sauheitl L and Guggenberger G (2019) Carbon Investment Required for the Mobilization of Inorganic and Organic Phosphorus Bound to Goethite by an Arbuscular Mycorrhiza (Solanum lycopersicum x Rhizophagus irregularis). Front. Environ. Sci. 7:26. doi: 10.3389/fenvs.2019.00026
Received: 31 August 2018; Accepted: 13 February 2019;
Published: 07 March 2019.
Edited by:
Carsten W. Mueller, Technische Universität München, GermanyReviewed by:
Luis Carlos Colocho Hurtarte, Technische Universität München, GermanyAlix Vidal, Technische Universität München, Germany
Marie Spohn, University of Bayreuth, Germany
Copyright © 2019 Andrino, Boy, Mikutta, Sauheitl and Guggenberger. This is an open-access article distributed under the terms of the Creative Commons Attribution License (CC BY). The use, distribution or reproduction in other forums is permitted, provided the original author(s) and the copyright owner(s) are credited and that the original publication in this journal is cited, in accordance with accepted academic practice. No use, distribution or reproduction is permitted which does not comply with these terms.
*Correspondence: Jens Boy, Ym95QGlmYmsudW5pLWhhbm5vdmVyLmRl