- 1Leibniz-Institute for Baltic Sea Research Warnemünde, Rostock, Germany
- 2University of Greifswald, Biological Station Hiddensee, Kloster, Germany
- 3Marine Research Institute, Klaipeda University, Klaipeda, Lithuania
Finding suitable places to establish a mussel farm is challenging, as many aspects like mussel growth, clearance effect and the risk of low oxygen conditions, have to be considered. We present a tailor-made approach, combining field experiments with a spatially explicit model tool, to support the planning process. A case study was set up in the German part of Szczecin (Oder) Lagoon (Baltic Sea), as it shows all typical eutrophication problems and has a strong need and high potential for nutrient retention measures. Farming zebra mussels (Dreissena polymorpha) is an innovative approach that utilizes a species which is often perceived as a pest. The practical applicability and water quality improvement potential was proven by a pilot farm. Combining the gained knowledge with the simulation model led to a cascade of mussel farm options that differ in purpose, location, and biomass. Placing a mussel farm in an enclosed bay resulted in a remarkable water quality improvement (Secchi Depth increased up to 2 m), but the effect stayed local, the growth was limited and the potential annual nutrient removal reached a threshold of ~30 t N and 2.8 t P. The same nutrient removal could be reached with much smaller farms in an open sea area, whereas the change of water transparency or bottom oxygen conditions were neglectable. A maximal nutrient removal potential of 1,750 t N and 160 t P per year was estimated, when nearly the entire German part of Szczecin Lagoon with mussel farms was used. This led to a strong reduction of phytoplankton and an increase of Secchi Depth, but also a rising risk of anoxia. Overall, all mussel farm options are only a supportive measure, but not sufficient to reach the Good Environmental Status demanded by the Water Framework Directive. At once, the nutrient export from Szczecin Lagoon to the open Baltic was reduced by up to 3,500 t N and 420 t P per year, making the large-scale mussel farm option also a potential measure within the Marine Strategy Framework Directive.
Introduction
Eutrophication and its consequences are a wide spread problem for many coastal waters (Duarte, 2009). Reaching a Good Environmental (Ecological) Status (GES) as required by overarching European legislations like the Marine Strategy Framework Directive (MSFD, 2008/56/EC) or the Water Framework Directive (WFD, 2000) is a binding duty for all EU member states within a very short period of time (MSFD: until 2020, WFD, 2021). The development and implementation of measures is an urgent task for scientists, stakeholders and authorities all over Europe.
The gap between present state and GES is tremendous in the Baltic Sea, as only 17 of 247 assessment units are in a good state (HELCOM, 2017). High nutrient loads and long residence times formed an unholy alliance (Voss et al., 2011; Carstensen et al., 2014; Andersen et al., 2017). Key problem is a too high phytoplankton density, resulting in a strongly reduced water transparency and a decline of submerged macrophytes, which are a central water quality indicator under the WFD (Hering et al., 2010; Carstensen et al., 2013; Zettler et al., 2017a). Concerted efforts are undertaken to further reduce the nutrient inputs within the HELCOM Baltic Sea Action Plan (BSAP; HELCOM, 2007, 2013), but coastal waters and their specific reduction needs were not included sufficiently in the BSAP (Schernewski et al., 2015). Considering the hysteresis-effect (Scheffer et al., 2001), the long residence times of nutrients in the Baltic Sea (30 to 50 years; Radtke et al., 2012) and the necessary time to re-establish a persistent submerged vegetation, reaching the GES seems impossible in most coastal waters of the Baltic Sea. This is especially true if measures are exclusively undertaken to reduce nutrient loads.
A meaningful extension of the existing measures could be waterborne (internal) actions, as applied often for lake restoration (Cooke et al., 2016). For the western Baltic Sea, the adaptation of low cost mussel farm techniques to mitigate nutrients is proved and tested (Lindahl et al., 2005; Petersen et al., 2012, 2014, 2016; Nielsen et al., 2016), while the experience from oligo- and mesohaline waters is still limited. Farming filter feeders combines the production of a protein-rich seafood, which is not fed externally, with a strong and fast improvement of water transparency (Schröder et al., 2014; Nielsen et al., 2016) and the potential to boost the growth of submerged macrophytes (Klamt and Schernewski, 2013). Prior to the construction of a mussel farm, its social, ecological and economic risks and potentials have to be considered, as well as the carrying capacity of the water body (Petersen et al., 2014, 2016). To estimate it, coupled 3D-simulation models are a suitable tool (Marinov et al., 2007), which include the hydrographical conditions near and far of a mussel farm, as well as the lower trophic food web, as nutrients and phytoplankton are impacted. Such spatially explicit models are a central key for the public acceptance and the stakeholder involvement in the planning process (Schumacher et al., 2018), while box models (Grant et al., 2007; Schernewski et al., 2012) are not suited to predict the spatial effects, which are often requested by stakeholders (Schernewski et al., 2018). If proven to be operated in a sustainable way, mussel farms allow to combine a spatial and temporal restricted effect with the necessary ecological improvements and the production of highly valuable protein-rich food for which there is a growing demand and market (Weber and Windisch, 2017), following the EU Blue Growth Strategy (European Commission, 2014).
Szczecin (Oder) Lagoon on the southern shore of the Baltic Sea is strongly eutrophied (BLANO, 2015) and plays an important role as a coastal filter (Asmala et al., 2017). At the moment, 55% (63%) of the Nitrogen (Phosphorus) loads entering the lagoon are transported to the open Baltic (Pastuszak et al., 2005). Enhancing the nutrient retention within the lagoon could therefore be a supporting tool to achieve the marine water quality targets of the Baltic Sea. Schernewski et al. (2012) estimated a potential Secchi Depth increase of up to 30 cm (50% of the present state) by mussel farms using zebra mussels (Dreissena polymorpha; Pallas, 1771), which due to the low salinity are the only cultivable filter feeder in Szczecin Lagoon (Wolnomiejski and Witek, 2013). Zebra mussels are often seen as a pest when they invade an ecosystem, like in Northern America, where the first occurrence was reported for 1986 (Carlton, 2008). The invasion led to strong changes of the ecosystem (Hebert et al., 1989; Ricciardi et al., 1998) and had also negative economic effects, e.g., by plugging cooling systems of power plants (Griffiths et al., 1991; Nalepa and Schloesser, 1992; Miehls et al., 2009). On the other hand, zebra mussels are also known for their high filtration efficiency (Kumar et al., 2016) and phytoplankton reduction. They are able to decrease summer primary production up to 50% (Stoeckmann and Garton, 2011). This resulted in an improved Secchi Depth (e.g., reported for Lake Huron by Fahnenstiel et al., 1995) and often led to the resettlement of submerged macrophytes (Skubinna et al., 1995; MacIsaac, 1996). Up to now, zebra mussels are only cultivated in small scale pilot farms (Lindahl, 2013) and are 2 to 3 cm at harvest to ensure the further processing (Schernewski et al., 2018). However, their potential is attracting more attention, e.g., in Curonian Lagoon (Bagdanaviciute et al., 2018). Dense aggregations of zebra mussels were already mentioned during the 19th century in Szczecin Lagoon (Brandt, 1894/96; Woznicka et al., 2016). They have distributed throughout the entire lagoon (Wolnomiejski and Witek, 2013), but are restricted to the lagoon due to the salinity increase outside (Stybel et al., 2009; Fenske et al., 2013). Altogether, Szczecin Lagoon shows all typical eutrophication problems and a strong need and high potential for nutrient retention measures. It was therefore chosen as representative to demonstrate the spatial potential of water quality improvements by an innovative mussel farm approach using a filter feeder species not used yet for aquaculture. Accounting the potential risks, the results are transferrable to oligohaline systems like Curonian or Vistula Lagoon in the Baltic Sea, but also, for example to Chesapeake Bay (USA), where Dreissena polymorpha is reported to have occurred since 1991 (Ashton and Klauda, 2015).
Accordingly, the study aims to: (a) present results of an experimental zebra mussel cultivation in Szczecin Lagoon; (b) present a spatially high resolved 3D-ecosystem model suitable to simulate effects of zebra mussel farms; (c) calibrate and validate the model based on long-term monitoring data, field studies and laboratory experiments, estimate the sensitivity of major parameters; (d) apply the model to analyze spatio-temporal ecological effects of different hypothetical farming options; (e) analyze mussel farms as potential measure within recent environmental policies (WFD, MSFD) and discuss its usability for water quality management, as well as the potential transfer to other coastal waters.
Materials and Methods
Case Study Site
Szczecin (Oder) Lagoon is a transboundary, transitional coastal water located on the southern shore of the Baltic Sea (Figure 2A), mainly used for recreation (tourism), fisheries and shipping. With an area of ~687 km2, it is the third largest lagoon in the Baltic and among the largest in Europe. It is divided in Large Lagoon (Polish territory) and Small Lagoon (shared between Poland and Germany). It is shallow (average depth of 3.8 m) and characterized by a low water exchange, resulting in an average water residence time of 55 days (Radziejewska and Schernewski, 2008). Thereby the water renewal is faster in the eastern part compared to Small Lagoon. This is reflected by the salinity which increases toward the west from 1 to 3 PSU, but also higher phytoplankton densities. The freshwater input is dominated by the Oder (Odra) river, which is with an average flow of 574 m3 s−1 (Pastuszak et al., 2012) one of biggest Baltic rivers. Further, two of the three outflows (Peenestrom, Swine and Dziwna) to the open Baltic Sea are located at the eastern part. Oder also determines the nutrient budgets (Schernewski et al., 2012). Annual loads of up to 100 kt Nitrogen (N) and 8.5 kt Phosphorus (P; Pastuszak et al., 2012) caused a strong decrease of water quality in the lagoon over the last century. The German part fails to reach any GES-target (Table 1) so far, with the strongest deviation for summer Chlorophyll a (Chl-a) being 4 times above the GES-threshold due to strong and long-lasting phytoplankton blooms. This prevents other water quality targets from being fulfilled since phytoplankton contributes to TN and TP and dominates the strong light attenuation reflected by the low Secchi Depth. Sporadic submerged macrophytes are present to a depth of 1.5 m (M. v. Weber, State Agency for Environment, Nature Conservation and Geology Mecklenburg-Vorpommern LUNG, pers. Comm.). Oxygen concentrations one meter above the bottom have their annual minimum around 6 ml l−1 (except 2007, when 1.75 ml l−1 were observed), while biochemical oxygen demand is extremely high (up to 6 mg l−1 in late summer), resulting in frequent anoxia and a strong P release from the sediment (Schernewski et al., 2011).
Filtration Experiments and Pilot Farm in Lake Usedom
To determine the effect of Dreissena polymorpha on the water quality under natural conditions, a series of filtration experiments with varying setups and time lengths were conducted. Mussels were collected from Small Lagoon and placed in either an in situ tube system or an ex situ aquarium on land. Both setups used natural sea water from Small Lagoon. For the tube system, two tubes were placed in the water column, with both sides open and one side above the water surface. One tube was filled with a defined biomass of zebra mussels while the other was empty, serving as a reference to include natural sinking of phytoplankton. Chlorophyll a was constantly measured using a fluorometer (AlgaeTorch®, bbe-moldaenke) in each tube. Additionally, water samples were taken to measure nutrients colorimetrically according to Grasshoff et al. (1999) by means of a Seal Analytical QuAAtro automated constant flow analyzer and to measure Chlorophyll a fluorometric (Turner fluorometer TD-10AU-005) after membrane filtration (GFF, 45 μm) and acidification. Water samples were kept cold until filtering within 24 h and then frozen by −20°C until further processing. For the aquarium, sampling procedure was analog and air bubbles provided continuous mixing of the system.
Between 2012 and 2014, in a semi-enclosed bay (Lake Usedom; Figure 2A) in the north-western part of Small Lagoon, a pilot farm was established, utilizing a newly developed net (Figure S1, on which the mussels stay throughout their whole life cycle (Schulze-Böttcher, 2014; Schernewski et al., 2018). The nets were deployed on six longlines which were locked with gravity anchors. The longlines were successfully lowered down to overwinter the mussels.
Simulation Model Including a Mussel Farm Module
To estimate the spatial effects of mussel farms, a tailor-made 3D model system was established (Figure 1). Therefore, a hydrological model (in our case GETM; General Estuarine Transport Model; www.getm.eu; Burchard and Bolding, 2002) was coupled to an ecosystem model (in our case ERGOM; Ecological Regional Ocean Model; www.ergom.net; Neumann et al., 2002) within the MOSSCO framework (Modular System for Shelves and Coasts; www.mossco.de; Lemmen et al., 2018) providing the necessary input for the mussel module: (i) water temperature and salinity; (ii) available food via phytoplankton and detritus concentrations. The simulation model had a horizontal resolution of 150 m and 20 vertical layers, which adapted their thickness to the density (Hofmeister et al., 2010; Gräwe et al., 2015). The model was run for 9 years (2007–2015), forced with realistic weather conditions, provided by the operational model of the National German Weather Service with a spatial resolution of 7 km and a temporal resolution of 3 h. Nutrient loads for Oder river were taken from Pastuszak et al. (2018) and were provided for the German rivers by LUNG. Boundary data was taken from a previous simulation covering the western Baltic Sea with a horizontal resolution of 600 m (Leipe et al., 2017; Zettler et al., 2017b; Beisiegel et al., 2018). To calculate Secchi Depth, the light attenuation was computed as function of phytoplankton and detritus (serving as proxy for the resuspension of organic and inorganic material), combined with a constant background attenuation. Validation of the model data was done by comparing it to regular monitoring data by LUNG and complemented by own observations.
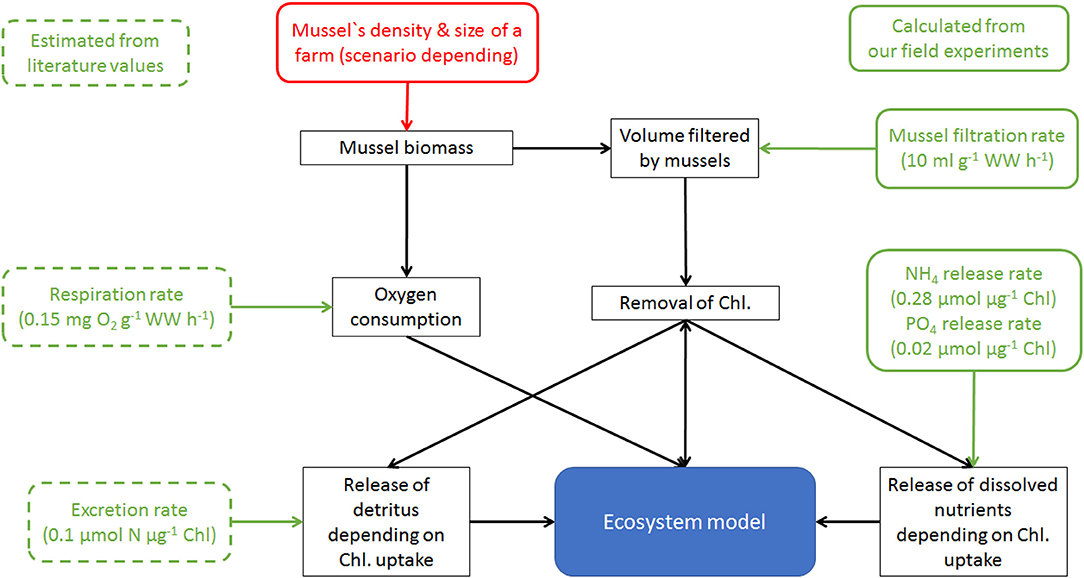
Figure 1. Simplified sketch of the mussel farm module (black rectangles) and its integration into the model system by calculating changes of Chlorophyll, detritus, , and Oxygen. Green are incorporated parameters, taken either from own field experiments (solid lines) or literature (dotted).
The newly developed mussel module (Figure 1) based on Saraiva et al. (2011) and Schröder et al. (2014). It included the principles of filtration, assimilation, excretion and respiration as: (I) filtration effect by the reduction of phytoplankton and detritus; (II) assimilation of ingested food to estimate the growth; (III) release of dissolved inorganic nutrients ( and ) and fast-sinking detritus (both were coupled to the food uptake); (IV) oxygen consumption by the mussels. Following McLaughlan and Aldridge (2013), the molecular N:P ratio of Dreissena polymorpha was assumed as 24:1, which was above Redfield (16:1), the ratio assumed in ERGOM for phytoplankton and detritus (Neumann et al., 2002). A molecular N:P ratio of 14:1 for the released dissolved nutrients was chosen so that the incorporated food had the same N:P ratio as the mussels and could be used directly to estimate the growth (Figure 1). The oxygen consumption rate was adapted as a linear function of the mussels' biomass from the observations by Orlova and Panov (2004), Caraco et al. (2000) and Schneider (1992), scaled with the water temperature following Schneider (1992). The release of fast-sinking detritus (sinking velocity 50 m d−1; Callier et al., 2006) was assumed as proportional to the uptaken food using a constant ratio of 20% (Schneider, 1992). Following the advection-diffusion schemes, the fast-sinking detritus was transported with the simulated currents and bounded to the sediment with the same rate as detritus. The filtration was adapted depending on the abiotic conditions following Schneider (1992) for temperature and McMahon (1996) and Kilgour et al. (1994) for salinity.
Following McLaughlan and Aldridge (2013) and Goedkoop et al. (2011), 10.1% (0.93%) of the mussel's dry weight was assumed as Nitrogen (Phosphorus). Combined with the estimation of Schneider (1992) that dry weight was 15% of the wet weight, 1 ton harvested mussels got equivalent to the removal of 15.1 kg Nitrogen and 1.4 kg Phosphorus.
Results
Lake Usedom: Pilot Farm and Simulation of a Small-Scale Mussel Farm in an Enclosed Bay
The pilot farm in Lake Usedom was successfully run with a mussel density of up to 31,700 individuals per m2 (Schulze-Böttcher, 2014). The biomass was between 5.2 and 22.6 kg m−2. Mussel larvae attached without external support and mussels grew up to 1 cm per year (Schulze-Böttcher, 2014) meaning that after two to 3 years the mussels were big enough for harvest. The settling was thereby more densely as in open waters trials of Small Lagoon and concentrations of pollutants in the mussels were below the thresholds for forage (Grov, 2015). A critical issue was the overgrowing of the mussels by Bryozoans (Plumatella fungosa) within the second year, which led to a die off of most mussels. This could be prevented if the mussels are harvested early enough, by a raised maintenance, or by choosing a location where the background conditions are more suitable, especially the currents should be at least 4 cm s−1 on average (Prendergast, 2010), while they are in Lake Usedom only 2.4 cm s−1.
In September 2013, surface salinities of maximal 1.3 and Chlorophyll concentrations (Chl-a) of up to 120 mg m−3 were measured (Figures 2B,C) which fits well to the model results (Figure S2a). In August 2014, after removing the mussel farm, concentrations of more than 200 mg m−3 Chl-a, a turbidity of up to 30 FTU and a Secchi Depth of < 20 cm were measured. This extraordinary high turbidity was enforced by strong south-westerly winds (up to 6 m s−1) and currents (Figure S2b), which are mainly well reproduced by the model. The pilot farm in Lake Usedom had a strong clearance effect on the surrounding water, as seen in Figures 2B,C where measured chlorophyll values are illustrated in and around the mussel farm. Filtration by mussels led to a 10% decrease of surface Chl-a.
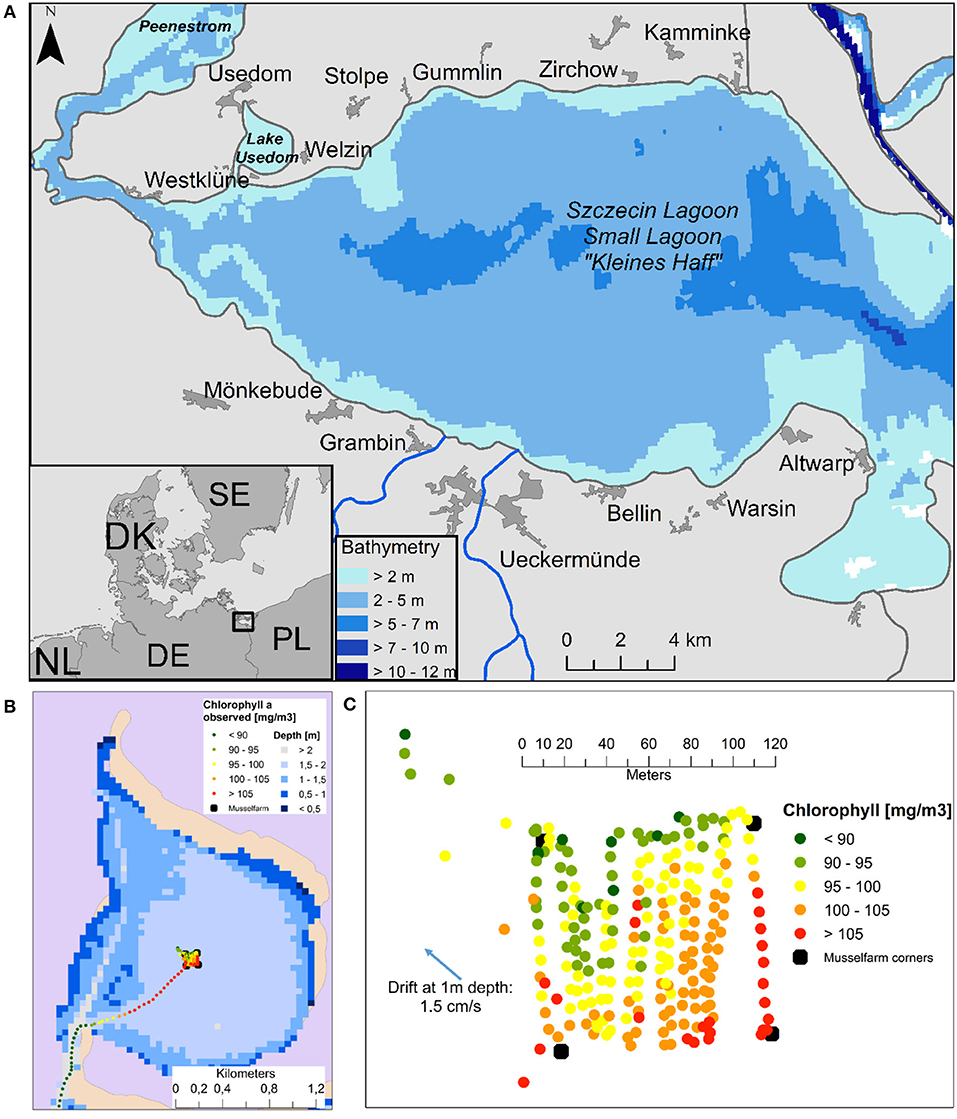
Figure 2. (A) The German part of Szczecin Lagoon (B) surface Chlorophyll a concentrations [mg m−3] from 25.9.2013 in Lake Usedom and (C) zoomed into the pilot farm.
Based on the successful pilot farm, the simulation model was then applied. Therefore, mussels with a density of 1 g wet weight per liter (afterwards abbreviated as g l−1) were placed in one grid cell (22,500 m2) at the center of Lake Usedom. This resulted in the simulation in a less dense settled farm (1.3 kg m−2) and a less strong reduction of Chl-a (on average Chl-a was ~5 mg m−3 smaller compared to the simulation without mussels). As the simulation model gave reasonable results, it was then used to scale up the farm to the entire bay. Choosing Lake Usedom as case study for an enclosed bay, had the advantages that a practical implementation is possible (although the extension of the pilot farm may fail due to Bryozoans), the average Chl-a concentration is higher than in the open parts of Small Lagoon (Figure 2B), while the clearance effect could be measured even at a low mussel biomass. Following the construction principles of longline mussel farms, mussel collectors began in the model 50 cm below the water surface and ended 50 cm above the sediment. Due to the maximum water depth of 2 m, collector lines were at most 1 m long. Overall, an area of ~2.24 km2 was covered (framed in Figure 3) with mussels (density: 1 g l−1), which resulted in a total biomass of 1,366 t (0.6 kg m−2). The mussel farm led to an average reduction1 of surface Chl-a in Lake Usedom of up to 55 mg m−3 (~40% less) and an increase of summer Secchi Depth of up to 50 cm (~80% more). Nevertheless, this change was only present in Lake Usedom and negligible beyond the bay (Figure 3). Due to the shallowness of the bay, the mussels' oxygen consumption is balanced by atmospheric oxygen, so that no anoxia occurred. Increasing the assumed mussel density led to a further increase of Summer Secchi Depth (up to 2 m, Figure 4), while the Chl-a decrease slowed down at higher mussel densities. Above a density of 1 g l−1 the risk of low oxygen conditions (< 6 mg l−1) was enhanced, while the annual mussel growth slowed down rapidly, leading to a threshold for the potential annual harvest of ~2,000 t (Figure 4).
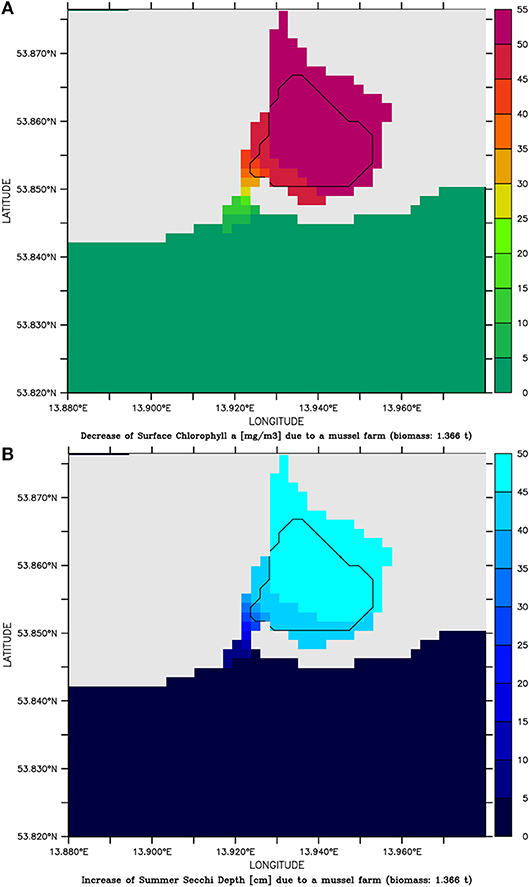
Figure 3. (A) decrease of Chlorophyll a conc. [mg m−3] and (B) increase of Summer Secchi Depth [cm] and in the simulation assuming a mussel farm with a density of 1 g l−1 in Lake Usedom compared to the simulation without mussels.
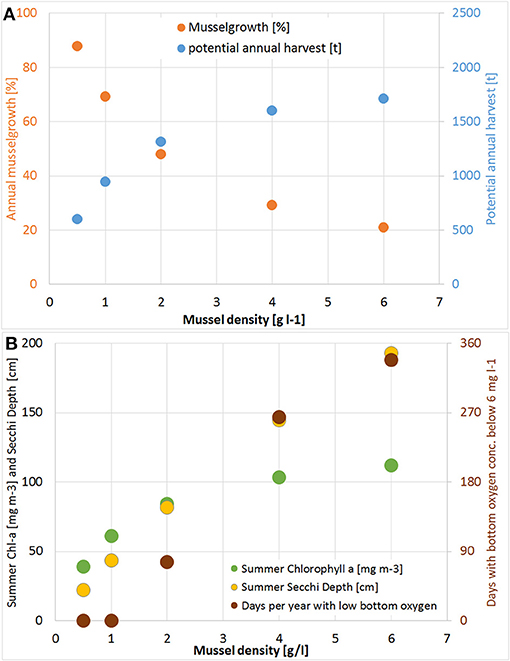
Figure 4. (A) Change of annual potential harvest [t a−1; blue] and mussel growth (harvest divided by mussel biomass; orange); (B) decrease of Summer Chlorophyll a [mg m−3; green] and increase of Secchi Depth [cm; yellow] and days per year with bottom oxygen concentration below 6 mg l−1 [brown] compared to the simulation without mussels for different mussel densities in Lake Usedom.
Combined with the experience from the pilot farm, this led to the conclusions that (i) mussel farming in Small Lagoon is possible; (ii) mussel farming has a positive impact on water transparency; (iii) deploying a farm in a very sheltered area can be problematic due to the potential overgrowing by Bryozoans and (iv) as the competition between the mussels was strong, only a slow growth could be achieved.
Estimating the Potential Carrying Capacity of Small Lagoon
The positive experiences from Lake Usedom motivated the questions, how large the maximal potential and what the ecological limits of mussel farms in Small Lagoon are. Therefore, mussel farms were assumed in the simulation model all over the areas of Small Lagoon with a depth of at least 4 m (~134 km2; framed area in Figure 8). Potential spatial conflicts, e.g., with shipping routes, were ignored to maximize the spatial extent. No mussels were assumed in the upper 50 and lower 150 cm of the water column.
Prior to the simulation of the large scale approach, a model validation with all available observation data was conducted. In Figure 5, Secchi Depth and Chl-a for the WFD-monitoring station KHO, located near to the city of Ueckermünde, is shown. Although some deviations occur, the general trend with high Chl-a, resp. low Secchi Depth during the growing season is well reproduced. To justify the simulations, tailor-made experiments (Figure 6) were conducted to determine the filtration and nutrient release rates in Small Lagoon under natural conditions. Thereby, the observed filtration rates varied strongly (Figure S4), especially when the Chl-a reduction was calculated from filtered water samples (2.6–88.1 ml h−1 g−1 WW). Using an AlgaeTorch instead to measure Chl-a, resulted in less variations (9.4–22.9 ml h−1 g−1 WW). Finally, the filtration rate was set for the model to 10 ml h−1 g−1 WW, but also varied for some sensitivity runs. At once, an increase of and (Figure 6) was seen when Dreissena polymorpha was present in the experiments. Using all experiments, a mean release of 0.28 μmol and 0.02 μmol for every μg of uptaken Chl-a was assumed for the model, although the variability between the experiments was significant (Figure S3).
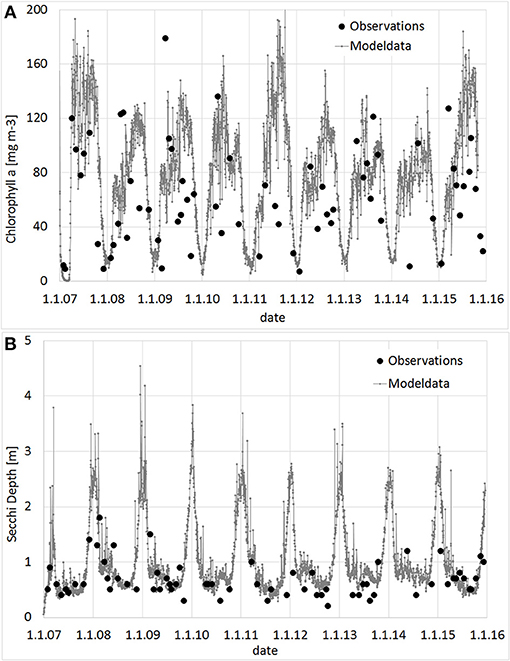
Figure 5. Observed and modeled values for Chlorophyll a (A; mg m−3) and Secchi Depth (B, m) at station KHO (near Ueckermünde). Observation provided by the State Agency for Environment, Nature Conservation and Geology Mecklenburg-Vorpommern.
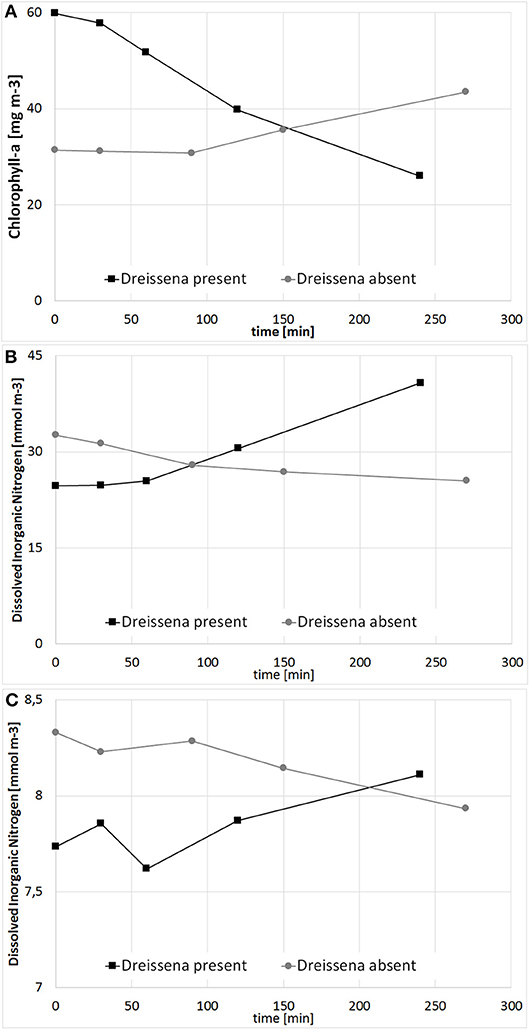
Figure 6. Change of Chlorophyll a (A; mg m−3), Dissolved Inorganic Nitrogen (B; mmol m−3) and Phosphorus (C; mmol m−3) in a filtration experiment with and without Dreissena polymorpha.
The large scale simulations were conducted with different mussel densities between 0.1 and 1.5 g l−1 to estimate the carrying capacity, resulting in an overall mussel biomass between 31,000 t and 470,000 t. With increasing mussel density, Chl-a was decreasing and Secchi Depth was rising. At once, the mussels' growth was slowing down and the risk of anoxia increased (Figure 7). It turned out that at the density of 0.7 g l−1, positive and negative effects are balanced. This corresponded to a mussel biomass of ~220,000 t (1.6 kg m−2) and resulted in a Chl-a decrease of up to 80 mg m−3 (Figure 8A) and a Summer Secchi Depth increase of up to 60 cm (Figure 8B). The risk of anoxia (expressed by the number of days with bottom oxygen concentration below 1 mg l−1; Figure 8C) increased erratically with especially high values in the northern part of the lagoon. The competition for food between the mussels was strong and resulted in an average annual growth of only 53% (compared to the initial biomass). This would allow to remove ~1,750 t N and 160 t P every year at harvest, which is 3% resp. Six persent of the annual riverine N and P loads. Due to the prevailing westward currents, a gradient with faster growth rates in the eastern part (up to 70% mussel growth per year; Figure 8D) occurred. Mussels in the western part of the lagoon received more already filtered water with less food (seen in the higher decrease of Chl-a), resulting in a potential mussel growth of < 50% per year (Figure 8D). The mussel feces accumulated substantially within the farm area (Figure S4) before they got bound into the sediment. Nearly no feces occurred outside Small Lagoon or in the enclosed bays like Lake Usedom. At once, the total amount of organic material in the deepest water layer decreased (with a comparable spatial gradient as for surface Chl-a; Figure S5b), leading also to an overall reduction of Nitrogen bound to the sediment (Figure S5c).
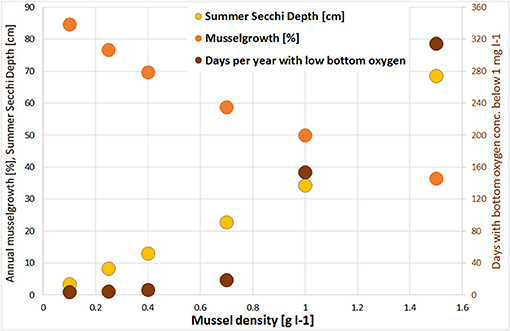
Figure 7. Change of annual potential mussel growth (harvest divided by mussel biomass; orange); and increase of Summer Secchi Depth [cm; yellow] and days per year with bottom oxygen concentration below 1 mg l−1 [brown] for different mussel densities in the large scale mussel farm option. Always in relation to the simulation without mussels.
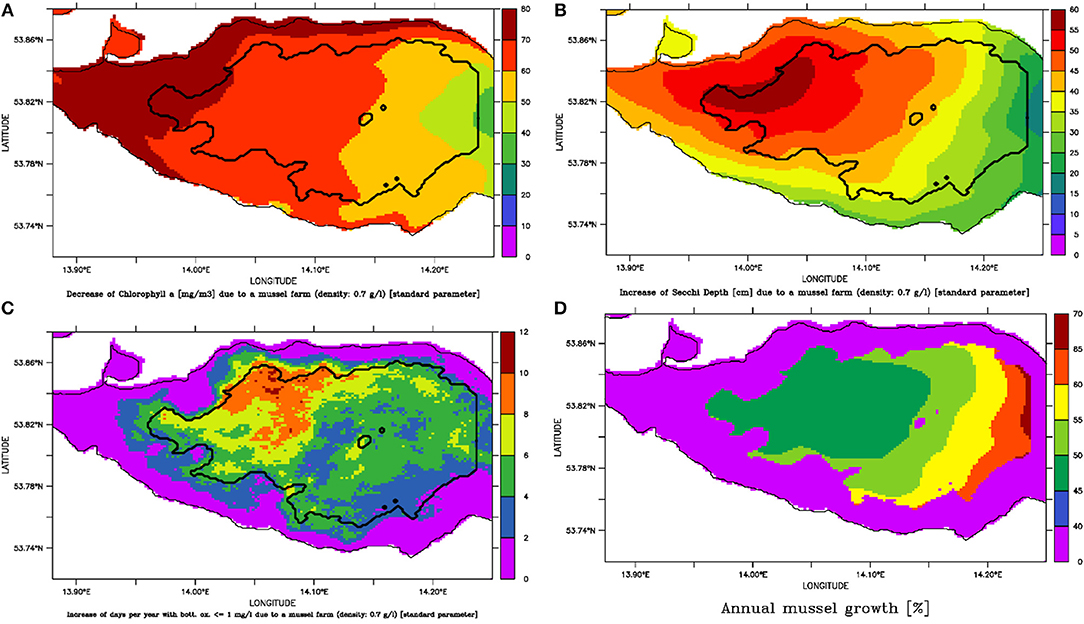
Figure 8. Assuming the large-scale mussel farm option with a mussel density of 0.7 mg l−1 results in (A) a decrease of Summer Chlorophyll a (mg m−3); (B) an increase of Summer Secchi Depth (cm); (C) an increase of number of days per year with bottom oxygen below 1 mg l−1 and (D) a potential mussel growth per year (potential harvest divided by the biomass).
The improvement and positive effect on water quality parameters used within the WFD was not strong enough to achieve the GES-thresholds (Figure 9). At once, the nutrient outflow from Szczecin Lagoon to the Pomeranian Bight (and the open Baltic Sea) was reduced by ~3,500 t N and 420 t P per year, which was substantially above the estimated annual nutrient removal. The annual export via Swina was thereby reduced by ~1,050 t N (4.4%) and 125 t P (6.8%) compared to the simulation without mussel farms. The export from Small Lagoon to Peenestrom was decreased substantially with 27% for N (2,450 t) and even 41% for P (295 t). This resulted in a Summer Secchi Depth increase by 30 cm in Achterwasser, although no mussels were placed there directly. The nutrient export via Dziwna (at the easternmost part of Szczecin Lagoon) remained unaffected.
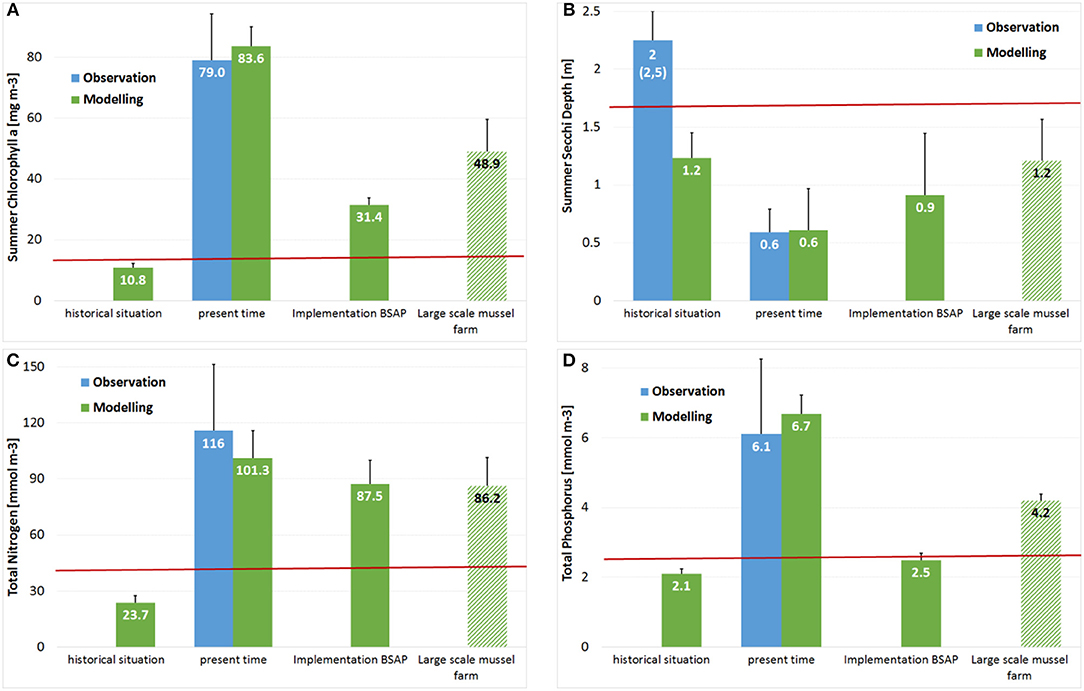
Figure 9. Change of water quality parameters used by EU Water Framework Directive in Small Lagoon assuming the maximal mussel farm option (A) Summer Chlorophyll-a; (B) Summer Secchi Depth; (C) Total Nitrogen; (D) Total Phosphorus in comparison to the GES-thresholds (Table 1; red lines), the historical and present states, as well as a future state assuming the full implementation of HELCOM BSAP.
To estimate the influence of key parameters (Figure 1), a couple of sensitivity runs (Table 2) was conducted with respect to (i) the filtration rate; (ii) the respiration rate; (iii) the ratio of released dissolved nutrients, feces and available energy for growth; and (iv) the feces sinking velocity. Elevating the filtration rate resulted in an increased uptake of Chl-a, a higher Summer Secchi Depth and a boosted mussel growth, as these values are directly coupled to the removal of organic material due to filtration. Further, the risk of anoxia decreased with an increasing filtration rate as the total amount of organic material diminished, while the respiration by the mussels remained unchanged. Varying the respiration rates influenced only the risk of anoxia, while other water quality parameters were unaffected. Changing the excretion of fast-sinking detritus, the nutrient release rates and the mussel growth was only possible at the same time, because these parameters were strongly linked. They determined how much of the uptaken energy was used for growth and if the nutrients that leave the mussels again were directly usable for primary production. Increasing the excretion rate and decreasing the nutrient release rates raised Secchi Depth substantially (as less organic material contributed to the near-surface light attenuation), while the risk of anoxia rise (as there was more organic material reaching the bottom, where it got remineralized by oxygen-consuming processes). The decreased amount of removed Chl-a indicated the impact of the released dissolved inorganic nutrients from the mussels on the primary production. The reduced oxygen production, due to the missing phytoplankton, was further contributing to the increased risk of anoxia. Reducing the assumed sinking velocity of the feces in the model resulted in a wider distribution of feces, which were then also present in the Peenestrom, while the Secchi Depth improvement was less strong and the risk of anoxia rose.
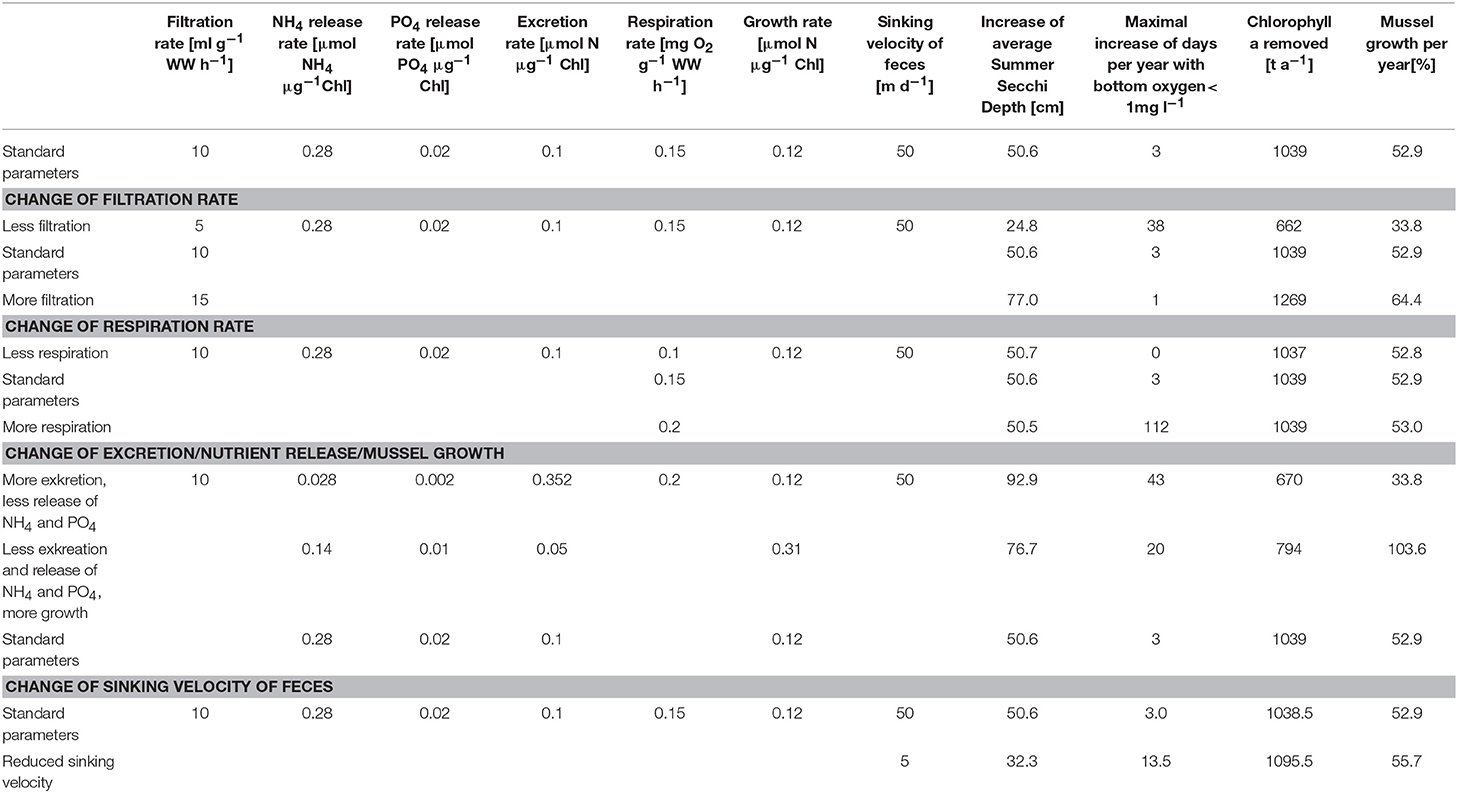
Table 2. Simulation results (increase of Summer Secchi Depth, days per year with bottom oxygen below 1 mg/l, removed Chl-a and mussel growth), if single parameters (filtration and respiration rates; excretion, nutrient release and mussel growth; sinking velocity of feces) are varied.
In general, due to the mussel farms water quality could be improved, but the nutrient removal stayed small compared to the high riverine nutrient inputs. The implementation of this maximal farming approach remains unrealistic, because of (i) nature protection rules, as Dreissena polymorpha is still treated as alien species; (ii) strong utilization conflicts; (iii) a small potential nutrient removal compared to the nutrient inputs; resulting in (iv) a low efficiency compared to the potential costs; and (v) unpredictable impacts on higher trophic levels. Nevertheless, the simulation model was proven to be well suited to support the planning process by providing reasonable, spatially explicit results on the environmental impacts.
Simulation of Several Small-Scale Farms
After seeing the practical limitations of the first two approaches, a third mussel farm option was developed. It addressed the question how much nutrient removal could be achieved based on a realistic farm size, where a deployment permission would be possible and mussel growth would not be yet hampered. Results are then transferable to other systems (e.g., Curonian Lagoon) and even to other filter feeder species, like Mytilus spp., as the simulation module is universal. To analyze the spatial differences, five locations were chosen nearby harbors (to reduce potential costs) but comparable regarding the background conditions (Table 3). The initial farm size at each site was ~0.45 km2 (20 horizontal grid cells) and the total biomass constant at 2,000 t, which was the maximal production potential estimated for Lake Usedom. All sites have a depth of at least 4 m, so that 1.5 m above the bottom and 0.5 m below the surface were kept free of mussels.
All five spots showed a high growth potential (85–90% of the assumed biomass per year) and only a slight water quality improvement (Figure 10), e.g., Summer Secchi Depth increased only around 8 cm at the farm locations and < 3 cm everywhere else, so that environmental impacts of a farm would be neglectable. Regional differences occurred with strongest positive effects near Ueckermünde, while the north-western site (Gummlin) was most endangered for low oxygen conditions and had the lowest growth straight away. Condensing the farms to less grid cells (9 or 1 instead of 20) and enhancing the density so that the total biomass stayed constant, resulted in an increased risk of low oxygen conditions at the farm spots (Figure 10) and a reduced growth up to 70% (Figure 11). Secchi Depth stayed mostly unaffected outside the mussel farms (Figure 10), while the maximal increase near the farms rose. The potential mussel growth would be equivalent to an annual removal potential at harvest of 21.5 – 27.5 t N and 2 – 2.55 t P.
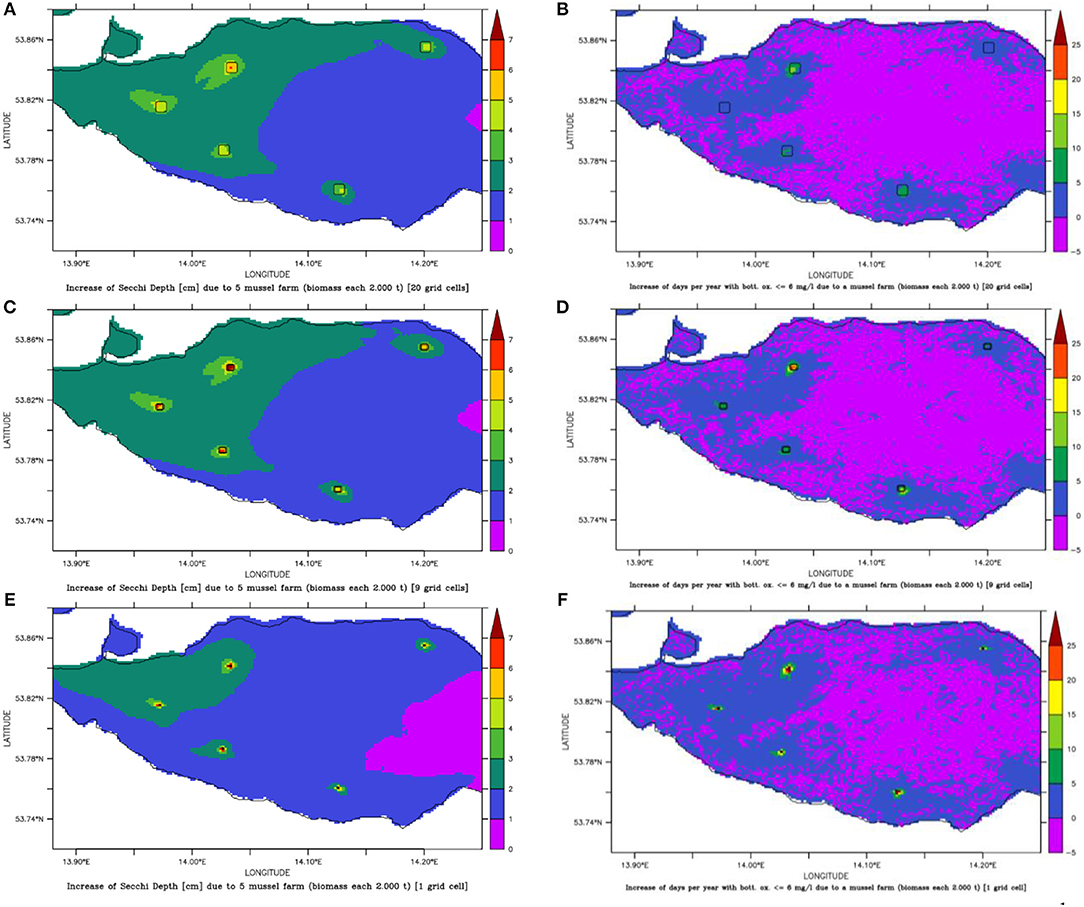
Figure 10. Change of Secchi Depth (A,C,E) and days per year with bottom oxygen below 6 mg l−1 (B,D,F) for mussel farm option 3 with 5 small scale farms (framed areas) of different sizes: either 0.45 km2 (A,B), 0.2 km2 (C,D) or 0.02 km2 (E,F).
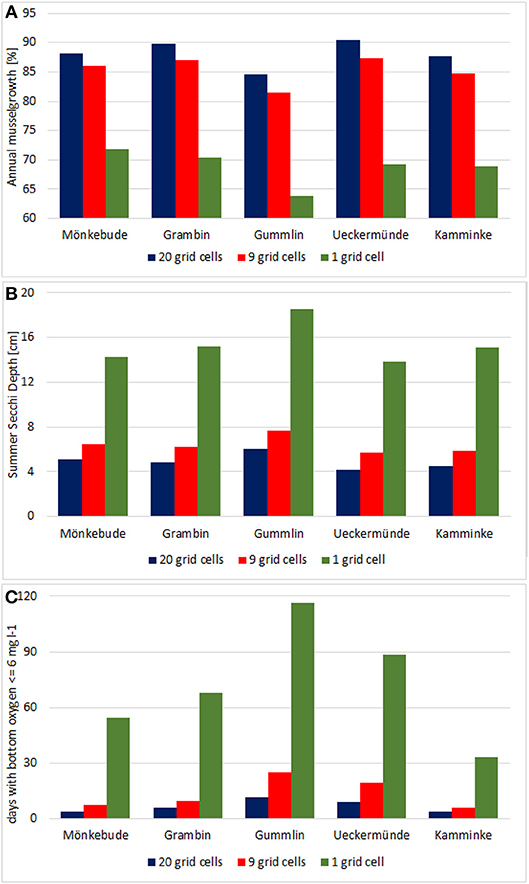
Figure 11. Condensing the 5 small scale farms in option 3 to less grid cells but fixing the mussel biomass at 2,000 t results in a reduced growth (A), and an increase of the maximal Secchi Depth improvement (B) and the days per year with bottom oxygen below 6 mg l−1 (C).
Discussion
Mussel farms are a widely discussed measure to mitigate eutrophication effects (Lindahl et al., 2005; Petersen et al., 2014, 2016). They allow an efficient reduction of phytoplankton (Qualls et al., 2007) and the active withdrawal of nutrients from strongly eutrophied coastal waters, which will fail to achieve the Good Ecological Status (GES) as claimed by overarching European legislations like the Water Framework Directive (WFD, 2000). Harvesting the farmed mussels is thereby necessary to ensure the active nutrient removal and allows to produce a highly valuable protein-rich product, utilizable i. a. in zoos (Schernewski et al., 2018), as forage (McLaughlan et al., 2014) or for the biogas production (Wollak et al., 2018). Even further uses of the shells are under development (Morris et al., 2018). Although, this new markets are developing, the pure commercial operating of mussel farms utilizing zebra mussel (Dreissena polymorpha) seems not promising (Schernewski et al., 2018). Instead, the combination with positive side effects, like an intensified use of bathing waters due to an increased water transparency (Schernewski et al., 2018) or an improved water quality, seems most promising.
Until now, farming filter feeders focused on Mytilus spp., mainly due to their higher commercial potentials. But in oligo- and mesohaline waters, like Szczecin Lagoon, Curonian Lagoon (both Baltic Sea), or Chesapeake Bay (USA), this is not possible and an alternative species must be utilized. While zebra mussels are invasive and a danger in many regions, their potential is acknowledged (McLaughlan and Aldridge, 2013). Beside the zebra mussel, the quagga mussel (Dreissena rostriformis bugensis; Andrusov, 1897) invades the same ecosystems and is also present in Szczecin Lagoon (our case study; Woznicka et al., 2016). Since both species follow the same life cycle, they will most likely both settle on mussel farm collectors. This species mixture in the mussel farm and the yield must be recognized and considered in a risk assessment before establishing a mussel farm in regions were both species occur. But as knowledge from zebra mussel farms is missing, suitable pilot farms and further studies on differences and similarities between these species are needed to estimate risks and potentials.
In the presented study, a newly developed, spatially explicit mussel farm simulation model was combined with field data from a pilot farm located in Lake Usedom, a semi-enclosed bay of Szczecin Lagoon. Cultivation of mussels was successful and resulted in reduced near-surface phytoplankton (Chl-a). In the model simulations, the mussels led to a higher water transparency (Secchi Depth) but the risk of anoxia remained to be the urgent ecological threat. While the estimated annual nutrient removal potential at the carrying capacity (1,750 t N, 160 t P) was of the same magnitude as previous studies, e.g., Schernewski et al. (2012) estimated 1,000 t N and 70 t P, it is not sufficient to achieve the water quality targets of the WFD (Figure 9). However, the open Baltic Sea, managed within the Marine Strategy Framework Directive (MSFD, 2008) and addressed in its poor ecological state by HELCOM Baltic Sea Action Plan (HELCOM, 2007, 2013), is strongly affected by nutrient export from coastal waters (Asmala et al., 2017). This export was reduced at the carrying capacity by ~3,500 t N and 420 t P per year, meaning that mussel farms could be a supportive measure to achieve the targets for both legislations (MSFD and WFD). Thereby, the nutrient export reduction is substantially above the pure nutrients bound in the harvest mussels. Nevertheless, compared to the high nutrient loads, mussel farms in Szczecin Lagoon are inefficient in reducing the overall nutrient concentrations. However, establishing farms is possible and environmental friendly (no external mussels are introduced, no external feeding), if the risks are assessed properly and the carrying capacity is not exceeded. Mussel farms may even support the re-occurrence of submerged macrophytes (Schernewski et al., 2018). Applying the model to sheltered systems with a weaker supply of external nutrients seems thus more promising. Using the simulation model in the planning phase allows to determine the carrying capacity by estimating the optimal density where growth and clearance are maximized, while the risk of anoxia is still low and the mass balance principle is held (Petersen et al., 2016). For Szczecin Lagoon, anoxia are a strong risk, as they lead to the release of adsorbed phosphorus from the sediments in the magnitude of even four-month-river-load (Bangel et al., 2004). The optimal mussel density could be thereby achieved by adjusting the space between the single longlines, so that the carrying capacity would correspond to a threshold spacing, as calculated by Rosland et al. (2011).
The main aim of the developed model was to raise the awareness and demonstrate potentials of mussel farms (Figure 1). Due to the missing experience, several risks were omitted such as the interaction with other benthic species (Zaiko and Daunys, 2015) and potential species composition changes (Wolnomiejski and Witek, 2013). Applying more detailed growth models, e.g., the Dynamic Energy Budget (Saraiva et al., 2011; Thomas et al., 2011; Maar et al., 2015) may give more sophisticated results, but a broad calibration data set is then needed, which was not available for our case study. Too complex models are a further obstacle for the stakeholder involvement in the planning process of a mussel farm (Schumacher et al., 2018).
As far as possible, experiments were conducted to determine the necessary model parameters under natural conditions (Figure 1). The experiments revealed a wide range of filtration rates (Figure S4), but the finally used rate (10 ml h−1 g−1 WW) was in the range of previous studies. Based on experiments by Fenske (2003), Stybel et al. (2009) calculated a mean filtration rate of 11.4 ml h−1 g−1 WW for mussel beds in Szczecin Lagoon, while Schneider et al. (1998) reported a mean clearance rate of 7.9 ml h−1 g−1 WW. Nevertheless, the reported filtration rates from field studies vary widely, e.g., Kotta et al. (1998) observed in mussel beds around Gulf of Riga filtration rates between 2 and 80 ml h−1 g−1 WW. Yu and Culver (1999) reported rates between 15.3 and 68.6 ml h−1 ind−1 from Hargus Lake (USA). Our sensitivity runs with varied filtration rates (Table 2) revealed that a stronger filtration led to reduced Chl-a concentrations and therefore an increased water transparency, while at once, the risk of anoxia decreased at the higher filtration rates. This indicated that there is a tipping point at which the reduction of organic material (due to filtration) balances the increased oxygen consumption, which would allow to cultivate the mussels at a higher density without violating the carrying capacity. A more sophisticated filtration model approach could improve the results in the future and increase the model's reliability.
The used oxygen consumption rate of 0.15 mg O2 h−1 g−1 WW was adapted from observations by Orlova and Panov (2004), Caraco et al. (2000) and Schneider (1992). It was in accordance to own experiments (at 15.8.16 caused 0.67 kg Dreissena polymorpha an oxygen decline of 4.0 mg over 4 h, giving a rate of 0.13 mg O2 h−1 g−1 WW). The sensitivity runs with different respiration rates revealed that the risk of anoxia and so the derived carrying capacity depended strongly on the respiration rate (Table 2). To enhance the model's reliability, a more sophisticated approach combined with more precise field data seems necessary, e.g., by including the feedback of low oxygen concentrations on the mussels as suggested by Gelda and Effler (2000).
In the simulation model, the nutrient release by the mussels was included. It was based on experiments under natural conditions (Figure S3), but the excretion of dissolved inorganic P was also stated by Orlova and Panov (2004) for mussel beds in Neva Estuary (Baltic Sea). Higgins and Zanden (2010) reported a strong increase of DIP in freshwater ecosystems due to Dreissena polymorpha, while DIN stayed mostly unchanged. Although, the nutrient release rates were highly variable in our experiments, they boosted phytoplankton growth as seen in the sensitivity runs (Table 2). Determining and implementing more precise release rates would be beneficial. When the release of and was reduced and instead the feces excretion was raised, Summer Secchi Depth improved strongest throughout all simulations (92.9 cm on average), as more organic material was moved to the bottom, substantially increasing the risk of anoxia there (combined with a decreased primary production). The released DIN and DIP boost phytoplankton blooms—especially outside the mussel farms where more light and nutrients become available, an effect also described for wind parks in the North Sea by Slavik et al. (2018).
The estimated N and P content of the harvested mussels (1.5% for N and 0.14% for P of the wet weight) originated from Mytilus farmed at low salinities in the Baltic Sea. The values were above Diaz and Kraufvelin (2013; 0.88% for N and 0.07% for P) or Stybel et al. (2009; 1% for N and 0.075% for P). Hence, the estimated annual N and P removal from the harvested mussels is uncertain and could also be 1,027 t N (instead of 1,750 t) or 80 t P (instead of 160 t) following Diaz and Kraufvelin (2013). These values must be substantiated in order to analyze the economics of mussel farms (as done by Schernewski et al.,(2018). Since WFD demands the cost-efficiency of a measure compared with land-based measures (Gren et al., 2009; Allin et al., 2017), possible benefits due to the nutrient removal need to be determined precise. On the other hand, the overall change of TN and TP concentrations of the surface water (Figure 9) was calculated from the ecosystem model, based on the changed concentrations of inorganic nutrients and organic matter and was therefore unaffected by the uncertainty induced by the mussels' nutrient contents.
Applying the developed simulation model to smaller farms revealed that in the enclosed bay (Lake Usedom) up to 2,000 t zebra mussels could be harvested every year (equivalent to an annual removal of 30.2 t N and 2.8 t P). To achieve the full potential yield, all other uses, like shipping or fishing, would get strongly restricted, and only the shipping route along the west coast might be kept free. On the other hand, the environmental impact would stay restricted to the bay (Figure 3). Almost the same potential harvest was achieved with much smaller farms in the open parts of Small Lagoon where the mussels benefit from stronger currents (average 4.5 cm s−1, Lake Usedom has 2.4 cm s−1). While the pilot farm was suffering from an overgrowing of the mussels from Bryozoan, this would be much less in a system with stronger currents (Prendergast, 2010). Regional differences between the open sea farms occurred which revealed again the importance of spatially explicit models. While the two southern sites (Grambin and Ueckermünde) had nearly the same background conditions (Table 3), the water quality improvement and growth was higher at Grambin, while the risk of low oxygen conditions rose at Ueckermünde. Optimal growth and strongest water quality improvement are not achievable at one spot and prior to the planning of a mussel farm, its main purpose has to be clarified. Accordingly, Bagdanaviciute et al. (2018) adjusted their set of criteria for the site-selection in Curonian Lagoon to the farm's aim.
The strong increase of days with low bottom oxygen concentrations is a foreseeable ecological risk of mussel farms from the model simulations. But the central parts of Small Lagoon, where the highest amount of mussel feces occurred in the large scale simulation (Figure S5), are characterized by organic-rich and anoxic sediments with nearly no living benthic organisms. This raises the question if a temporal restricted farm would not be beneficial on longer time scales. In our simulation, the amount of N bound to the sediments was decreased by up to 50% (Figure S5). Therefore, the long-term effects of mussel farms must be considered stronger, also with respect to changes of the nutrient cycles (Stadmark and Conley, 2011), as Lavrentyev et al. (2000) reported an increase of denitrifications rates due to zebra mussels. More observations on benthic nutrients fluxes, how they change due to mussels, as well as a more sophistic early-diagnosis model, like the one developed by Radtke et al. (2018), seem necessary to understand the full spectrum of ecological changes related to mussel farms and their usability as nutrient removal measure.
Conclusion
Mussel farms utilizing zebra mussels are possible. They can have a positive effect on the water quality and be environmental friendly, as long as the risks are respected. They close a gap as a support to reach the targets of MSFD and WFD in low saline systems, although in Szczecin Lagoon their nutrient removal potential is inefficient compared to the high nutrient loads. Applying spatially explicit model simulations in the planning process is suitable to determine the carrying capacity and to find optimal locations for a mussel farm—depending on its purpose, as maximal growth and clearance effect will not be achieved all at once. Nevertheless, more experience from suitable pilot farms is needed, as well as a more sophisticated model system.
Author Contributions
RF was responsible for overall structure of the manuscript, running the simulations, and for writing the manuscript. A-LB and GS contributed to the writing, A-LB performed the filtration experiments and SD ran the pilot farm in Lake Usedom.
Funding
The work was financially supported by projects BONUS BaltCoast, BONUS OPTIMUS and MOSSCO-Synthese. BONUS BaltCoast and OPTIMUS have received funding from BONUS (Art 185) funded jointly by the European Union's Seventh Programme for research, technological development and demonstration, and from Baltic Sea national funding institutions (BMBF 03F0717A; 03F0769A). MOSSCO-Synthese was funded by the German Federal Ministry for Education and Research within KÜNO (03F0740B). Supercomputing power was provided by HLRN (North-German Supercomputing Alliance).
Conflict of Interest Statement
The authors declare that the research was conducted in the absence of any commercial or financial relationships that could be construed as a potential conflict of interest.
Acknowledgments
We like to thank C. Burmeister, L. Meyers, C. Kruspe, M. v. Weber (LUNG-MV) for supporting the work or for providing data and information and K. Klingbeil, U. Gräwe and H. Radtke for the code maintenance of GETM and technical support. We like to thank J. Hofmann, S. Felsing and M. Inacio for corrections and comments on the manuscript.
Supplementary Material
The Supplementary Material for this article can be found online at: https://www.frontiersin.org/articles/10.3389/fenvs.2018.00158/full#supplementary-material
Footnotes
1. ^Throughout the whole study, terms like “reduction” or “increase” correspond to the change computed between a simulation assuming a certain mussel farm option and the simulation without any mussels.
References
Allin, A., Schernewski, G., Friedland, R., Neumann, T., and Radtke, H. (2017). Climate change effects on denitrification and associated avoidance costs in three Baltic river basin - coastal sea systems. J. Coast. Conserv. 21, 561–569. doi: 10.1007/s11852-017-0530-8
Andersen, J. H., Carstensen, J., Conley, D. J., Dromph, K., Fleming-Lehtinen, V., Gustafsson, B. G., et al. (2017). Long-term temporal and spatial trends in eutrophication status of the Baltic Sea. Biol Rev. 92, 135–149. doi: 10.1111/brv.12221
Ashton, M. J., and Klauda, R. J. (2015). The spread of zebra mussel (Dreissena polymorpha) from the lower Susquehanna River into the upper Chesapeake Bay, Maryland, USA. Bioinvasions Rec. 4, 195–199. doi: 10.3391/bir.2015.4.3.07
Asmala, E., Carstensen, J., Conley, D. J., Slomp, C. P., Stadmark, J., and Voss, M. (2017). Efficiency of the coastal filter: nitrogen and phosphorus removal in the Baltic Sea. Limnol. Oceanogr. 62, 222–238. doi: 10.1002/lno.10644
Bagdanaviciute, I., Umgiesser, G., Vaičiute, D., Bresciani, M., Kozlov, I., and Zaiko, A. (2018). GIS-based multi-criteria site selection for zebra mussel cultivation: addressing end-of-pipe remediation of a eutrophic coastal lagoon ecosystem. Sci. Total Environ. 634, 990–1003. doi: 10.1016/j.scitotenv.2018.03.361
Bangel, H., Schernewski, G., Bachor, A., and Landsberg-Uczciwek, M. (2004). “Spatial pattern and long-term development of water quality in the Oder Estuary,” in The Oder Estuary – Against the Background of the European Water Framework Directive, eds G. Schernewski, and T. Dolch (Warnemünde: Marine Science Reports 57), 17–65.
Beisiegel, K., Darr, A., Zettler, M. L., Friedland, R., Gräwe, U., and Gogina, M. (2018). Understanding the spatial distribution of subtidal reef assemblages in the southern Baltic Sea using towed camera platform imagery. Estuar. Coast. Shelf Sci. 207, 82–92. doi: 10.1016/j.ecss.2018.04.006
BLANO (2015). Harmonisierte Hintergrund- und Orientierungswerte für Nährstoffe und Chlorophyll-a in den deutschen Küstengewässern der Ostsee sowie Zielfrachten und Zielkonzentrationen für die Einträge über die Gewässer (in German). Hrsg.: Bundesministerium für Umwelt, Naturschutz und Reaktorsicherheit. Available online at: https://www.meeresschutz.info/sonstige-berichte.html
Brandt, K (1894/96). Über das Stettiner Haff (in German). Wiss. Meeresuntersuchungen, Band 1 (Heft 2), Kiel und Leipzig 147p.
Burchard, H., and Bolding, K. (2002). GETM—a General Estuarine Transport Model. Scientific documentation. Technical Report EUR 20253 EN, European Commission
Callier, M., Weise, A., McKindsey, C., and Desrosiers, G. (2006). Sedimentation rates in a suspended mussel farm (Great-Entry Lagoon, Canada): biodeposit production and dispersion. Mar. Ecol. Prog. Ser. 322, 129–141. doi: 10.3354/meps322129
Caraco, N. F., Cole, J. J., Findlay, S. E. G., Fischer, D. T, Lampman, G. G., Pace, M. L., and Strayer, D. L. (2000). Dissolved oxygen declines in the hudson river associated with the invasion of the Zebra Mussel (Dreissena polymorpha). Environ. Sci. Tech. 34, 1204–1210. doi: 10.1021/es990565z
Carlton, J. T. (2008). The zebra mussel Dreissena polymorpha found in North America in 1986 and 1987. J. Great Lakes Res. 34, 770–773. doi: 10.1016/S0380-1330(08)71617-4
Carstensen, J., Andersen, J. H., Gustafsson, B. G., and Conley, D. J. (2014). Deoxygenation of the Baltic Sea. Proc. Natl. Acad. Sci. 111, 5628–5633. doi: 10.1073/pnas.1323156111
Carstensen, J., Krause-Jensen, D., Markager, S., Timmermann, K., and Windolf, J. (2013). Water clarity and eelgrass responses to nitrogen reductions in the eutrophic Skive Fjord, Denmark. Hydrobiologia 704, 293–309. doi: 10.1007/s10750-012-1266-y
Cooke, G. D., Welch, E. B., Peterson, S., and Nichols, S. A. (2016). Restoration and Management of Lakes and Reservoirs. Boca Raton, FL: CRC, Taylor & Francis.
Diaz, E. R., and Kraufvelin, P. (2013). Methodology for Monitoring and Evaluation. The Baltic EcoMussel project final report. Available online at: http://projects.centralbaltic.eu/images/files/result_pdf/BALTIC_ECOMUSSEL_result1_Final_report_small.pdf
Duarte, C. M. (2009). Coastal eutrophication research: a new awareness. Hydrobiologia 629, 263–269. doi: 10.1007/s10750-009-9795-8
European Commission (2014). Information From Website. Available online at: http://ec.europa.eu/maritimeaffairs/policy/blue_growth/index_en.htm
Fahnenstiel, G. L., Lang, G. A., Nalepa, T. F., and Johengen, T. H. (1995). Effects of zebra mussel (Dreissena polymorpha) colonization on water quality parameters in Saginaw Bay, Lake Huron. J. Great Lakes Res. 21, 435–448. doi: 10.1016/S0380-1330(95)71057-7
Fenske, C. (2003). Die Wandermuschel (Dreissena polymorpha) im Oderhaff und ihre Bedeutung für das Küstenzonenmanagement (in German). Greifswald: Inauguraldissertation an der Univ. Available online at: http://databases.eucc-d.de/files/documents/00000098_Fenske_DieWandermuschelimOderhaff.pdf
Fenske, C., Zaiko, A., Wozniczka, A., Dahlke, S., and Orlova, M. I. (2013). “Variation in length–frequency distributions of zebra mussels (Dreissena polymorpha) within and between Three Baltic Sea Subregions Szczecin Lagoon, Curonian Lagoon, and Gulf of Finland,” in Quagga and zebra mussels: Biology, Impacts and Control, eds T. F. Nalepa, D. W. Schlosser (Boca Raton, FL; London; New York, NY: CRC Press Taylor & Francis Group), 725–740.
Fürhaupter, K., and Meyer, T. (2015). Handlungsanweisung zum Bewertungsverfahren PHYBIBCO - Bewertung des ökologischen Zustandes der Makrophyten in den inneren Küstengewässern der Ostsee nach den Vorgaben der WRRL. Availble online at: http://www.gewaesser-bewertung.de/files/handlungsanweisung_phybibco_mai2015_deu.pdf
Gelda, R. K., and Effler, S. W. (2000). A river water quality model for chlorophyll and dissolved oxygen that accommodates zebra mussel metabolism. Water Qual. Ecosyst. Model. 1, 271–309. doi: 10.1023/A:1013951003172
Goedkoop, W., Naddafi, R., and Grandin, U. (2011). Retention of N and P by zebra mussels (Dreissena polymorpha Pallas) and its quantitative role in the nutrient budget of eutrophic Lake Ekoln, Sweden. Biol. Invasions. 13:1077. doi: 10.1007/s10530-011-9950-9
Grant, J., Curran, K. J., Guyondet, T. L., Tita, G., Bacher, C., Koutitonsky, V., et al. (2007). A box model of carrying capacity for suspended mussel aquaculture in Lagune de la Grande-Entrée, Iles-de-la-Madeleine, Québec. Ecol. Modell. 200, 193–206. doi: 10.1016/j.ecolmodel.2006.07.026
Grasshoff, K., Kremling, K., and Ehrhardt, M. (eds.). (1999). Methods of Seawater Analysis - 3rd Edn. Weinheim: Wiley-VCH. 159–228.
Gräwe, U., Holtermann, P., Klingbeil, K., and Burchard, H. (2015). Advantages of vertically adaptive coordinates in numerical models of stratified shelf seas. Ocean Model. 92, 56–68. doi: 10.1016/j.ocemod.2015.05.008
Gren, I.-M., Lindahl, O., and Lindqvist, M. (2009). Values of mussel farming for combating eutrophication: an application to the Baltic Sea. Ecol. Eng. 35, 935–945. doi: 10.1016/j.ecoleng.2008.12.033
Griffiths, R. W., Schloesser, D. W., Leach, J. H., and Kovalak, W. P. (1991). Distribution and dispersal of the zebra mussel (Dreissena polymorpha) in the Great Lakes region. Can. J. Fish. Aquat. Sci. 48, 1381–1388 doi: 10.1139/f91-165
Grov, M. (2015). Untersuchungen zur Kultivierung filtrierender Organismen im Usedomer See. Greifswald: Master of Science Thesis, Landschaftsökologie und Naturschutz, University of Greifswald.
Hebert, P. D., Muncaster, B. W., and Mackie, G. L. (1989). Ecological and genetic studies on Dreissena polymorpha (Pallas): a new mollusc in the Great Lakes. Can. J. Fish. Aquat. Sci. 46, 1587–1591 doi: 10.1139/f89-202
HELCOM (2007). Baltic Sea Action Plan. HELCOM Ministerial Meeting Krakow. Available online at: http://www.helcom.fi/Documents/Baltic%20sea%20action%20plan/BSAP_Final.pdf
HELCOM (2013). HELCOM Copenhagen Ministerial Declaration: Taking Further Action to Implement the Baltic Sea Action Plan - Reaching a Good environmental Status for a Healthy Baltic Sea. Copenhagen: HELCOM Declaration.
HELCOM (2017). First Version of the ‘State of the Baltic Sea' Report – June 2017 – to be Updated in 2018. Available online at: http://stateofthebalticsea.helcom.fi
Hering, D., Borja, A., Carstensen, J., Carvalho, L., Elliott, M., Feld, C. K., et al. (2010). The European Water Framework Directive at the age of 10: a critical review of the achievements with recommendations for the future. Sci. Total Environ. 408, 4007–4019. doi: 10.1016/j.scitotenv.2010.05.031
Higgins, S. N., and Zanden, M. J. (2010). What a difference a species makes: a meta–analysis of dreissenid mussel impacts on freshwater ecosystems. Ecol. Monogr. 80, 179–196. doi: 10.1890/09-1249.1
Hofmeister, R., Burchard, H., and Beckers, J. M. (2010). Non-uniform adaptive vertical grids for 3d numerical ocean models. Ocean Model. 33, 70–86. doi: 10.1016/j.ocemod.2009.12.003
Kilgour, B. W., Mackie, G. L., Baker, M. A., and Keppel, R. (1994). Effects of salinity on the condition and survival of zebra mussels (Dreissena polymorpha). Estuaries 17:385. doi: 10.2307/1352671
Klamt, A., and Schernewski, G. (2013). “Climate change – a new opportunity for mussel farming in the southern baltic?” in Climate Change Adaptation in Practice, eds P. Schmidt-Thomé and J. Klein (Chichester: Wiley-Blackwell), 171–184. doi: 10.1002/9781118548165.ch13
Kotta, J., Orav, H., and Kotta, I. (1998). Distribution and filtration activity of the zebra mussel, Dreissena polymorpha, in the Gulf of Riga and the Gulf of Finland. Proc. Eston. Acad. Sci. Biol. Ecol. 47, 32–41.
Kumar, R., Varkey, D., and Pitcher, T. (2016). Simulation of zebra mussels (Dreissena polymorpha) invasion and evaluation of impacts on Mille Lacs Lake, Minnesota: an ecosystem model. Ecol. Modell. 331, 68–76. doi: 10.1016/j.ecolmodel.2016.01.019
Lavrentyev, P. J., Gardner, W. S., and Yang, L. (2000). Effect of the zebra mussel on nitrogen dynamics and the microbial community at the sediment-water interface. Aquat. Microb. Ecol. 21, 187–194. doi: 10.3354/ame021187
Leipe, T., Naumann, M., Tauber, F., Radtke, H., Friedland, R., Hiller, A., et al. (2017). Regional distribution patterns of chemical parameters in surface sediments of the south-western Baltic Sea and their possible causes. Geo Mar. Lett. 37, 593–606. doi: 10.1007/s00367-017-0514-6
Lemmen, C., Hofmeister, R., Klingbeil, K., Nasermoaddeli, M. H., Kerimoglu, O., Burchard, H., et al. (2018). Modular System for Shelves and Coasts (MOSSCO v1.0) – a flexible and multi-component framework for coupled coastal ocean ecosystem modelling. Geosci. Model Dev.11, 915–935. doi: 10.5194/gmd-11-915-2018.
Lindahl, O. (2013). Mussel Meal Production Based on Mussels From the Baltic Sea. Reports of Aquabest Project.
Lindahl, O., Hart, R., Hernroth, B., Kollberg, S., Loo, L.-O., Olrog, L., et al. (2005). Improving marine water quality by mussel farming: a profitable solution for swedish society. AMBIO 34, 131–138. doi: 10.1579/0044-7447-34.2.131
Maar, M., Saurel, C., Landes, A., Dolmer, P., and Petersen, J. K. (2015). Growth potential of blue mussels (M. edulis) exposed to different salinities evaluated by a Dynamic Energy Budget model. J. Mar. Syst. 148, 48–55. doi: 10.1016/j.jmarsys.2015.02.003
MacIsaac, H. J. (1996). Potential abiotic and biotic impacts of zebra mussels on the inland waters of north America. Amer. Zool. 36, 287–299. doi: 10.1093/icb/36.3.287
Marinov, D., Galbiati, L., Giordani, G., Viaroli, P., Norro, A., Bencivelli, S., et al. (2007). An integrated modelling approach for the management of clam farming in coastal lagoons. Aquaculture 269, 306–320. doi: 10.1016/j.aquaculture.2007.04.071
McLaughlan, C., and Aldridge, D. C. (2013). Cultivation of zebra mussels (Dreissena polymorpha) within their invaded range to improve water quality in reservoirs. Water Res. 47, 4357–4369. doi: 10.1016/j.watres.2013.04.043
McLaughlan, C., Rose, P., and Aldridge, D. C. (2014). Making the best of a pest: The potential for using invasive zebra mussel (Dreissena polymorpha) biomass as a supplement to commercial chicken feed. Environ. Manage. 54, 1102–1109. doi: 10.1007/s00267-014-0335-6
McMahon, R. F. (1996). The physiological ecology of the zebra mussel, Dreissena polymorpha, in North America and Europe. Integr. Comp. Biol. 36, 339–363. doi: 10.1093/icb/36.3.339
Miehls, A. L. J., Mason, D. M., Frank, K. A., Krause, A. E., Peacor, S. D., and Taylor, W. W. (2009). Invasive species impacts on ecosystem structure and function: a comparison of Oneida Lake, New York, USA, before and after zebra mussel invasion. Ecol. Modell. 220, 3194–3209. doi: 10.1016/j.ecolmodel.2009.07.020
Morris, J. P., Backeljau, T., and Chapelle, G. (2018). Shells from aquaculture: a valuable biomaterial, not a nuisance waste product. Rev. Aquacult. doi: 10.1111/raq.12225
MSFD (2008). Commission of the European communities directive 2008/56/EC of the European parliament and of the council of 17 June 2008 establishing a framework for community action in the field of marine environmental policy (marine strategy framework directive). Off. J. Eur. Union L 164, 19–40.
Nalepa, T. F., and Schloesser, D. W. (eds.). (1992). Zebra Mussels Biology, Impacts, and Control. Boca Raton, FL: CRC Press.
Neumann, T., Fennel, W., and Kremp, C. (2002). Experimental simulations with an ecosystem model of the Baltic Sea: a nutrient load reduction experiment. Glob. Biogeochem. Cycles 16, 7–1–7–19. doi: 10.1029/2001GB001450
Nielsen, P., Cranford, P. J., Maar, M., and Petersen, J. K. (2016). Magnitude, spatial scale and optimization of ecosystem services from a nutrient extraction mussel farm in the eutrophic Skive Fjord, Denmark. Aquacult. Environ. Interact. 8, 311–329. doi: 10.3354/aei00175
Orlova, M. I., and Panov, V. E. (2004). “Establishment of the zebra mussel, Dreissena polymorpha (Pallas), in the Neva Estuary (Gulf of Finland, Baltic Sea): distribution, population structure and possible impact on local unionid bivalves,” in Biology of the Baltic Sea. Developments in Hydrobiology, Vol 176, eds H. Kautsky, and P. Snoeijs (Dordrecht: Springer). doi: 10.1007/978-94-017-0920-0_19
Pastuszak, M., Bryhn, A. C., Håkanson, L., Stålnacke, P., Zalewski, M., and Wodzinowski, T. (2018). Reduction of nutrient emission from Polish territory into the Baltic Sea (1988-2014) confronted with real environmental needs and international requirements. Oceanol. Hydrobiol. Stud. 47, 140–166.
Pastuszak, M., Stalnacke, P., Pawlikowski, K., and Witek, Z. (2012). Response of Polish rivers (Vistula, Oder) to reduced pressure from point sources and agriculture during the transition period (1988 - 2008). J. Mar. Syst. 94, 157–173. doi: 10.1016/j.jmarsys.2011.11.017
Pastuszak, M., Witek, Z., Nagel, K., Wielgat, M., and Grelowski, A. (2005). Role of the Oder estuary (southern Baltic) in transformation of the riverine nutrient loads. J. Mar. Syst. 57, 30–54. doi: 10.1016/j.jmarsys.2005.04.005
Petersen, J. K., Hasler, B., Timmermann, K., Nielsen, P., Bruunshøj Tørring, D., Larsen, M. M., et al. (2014). Mussels as a tool for mitigation of nutrients in the marine environment. Mar. Pollut. Bull. 82, 137–143. doi: 10.1016/j.marpolbul.2014.03.006
Petersen, J. K., Saurel, C., Nielsen, P., and Timmermann, K. (2016). The use of shellfish for eutrophication control. Aquacult. Internat. 24, 857–878. doi: 10.1007/s10499-015-9953-0
Petersen, J. K., Timmermann, K., Carlsson, M., Holmer, M., Maar, M., and Lindahl, O. (2012). Mussel farming can be used as a mitigation tool – A reply. Mar. Poll. Bull. 64, 452–454. doi: 10.1016/j.marpolbul.2011.11.027
Prendergast, G. S. (2010). Settlement and Behaviour of Marine Fouling Organisms. Chichester, UK: Wiley-Blackwell.
Qualls, T. M., Dolan, D. M., Reed, T., Zorn, M. E., and Kennedy, J. (2007). Analysis of the impacts of the zebra mussel, Dreissena polymorpha, on nutrients, water clarity, and the chlorophyll-phosphorus relationship in lower Green Bay. J. Great Lakes Res. 33, 617–626. doi: 10.3394/0380-1330(2007)33[617:AOTIOT]2.0.CO;2
Radtke, H., Lipka, M., Bunke, D., Morys, C., Cahill, B., Böttcher, M. E., et al. (2018). Ecological ReGional Ocean Model with vertically resolved sediments (ERGOM SED 1.0): Coupling benthic and pelagic biogeochemistry of the south-western Baltic Sea. Geosci. Model Dev. Discuss. doi: 10.5194/gmd-2018-109
Radtke, H., Neumann, T., Voss, M., and Fennel, W. (2012). Modeling pathways of riverine nitrogen and phosphorus in the Baltic Sea. J. Geophys. Res. 117, 2156–2202. doi: 10.1029/2012JC008119
Radziejewska, T., and Schernewski, G. (2008). “The Szczecin (Oder-) Lagoon,” in Ecology of Baltic Coastal Waters, ed U. Schiewer (Heidelberg: Springer Berlin), 115–129. doi: 10.1007/978-3-540-73524-35
Ricciardi, A., Neves, R. J., and Rasmussen, J. B. (1998). Impending extinctions of North American freshwater mussels (Unionoida) following the zebra mussel (Dreissena polymorpha) invasion. J. Anim. Ecol. 67, 613–619 doi: 10.1046/j.1365-2656.1998.00220.x
Rosland, R., Bacher, C., Strand, Ø., Aure, J., and Strohmeier, T. (2011). Modelling growth variability in longline mussel farms as a function of stocking density and farm design. J. Sea Res. 66, 318–330. doi: 10.1016/j.seares.2011.04.009
Sagert, S., Selig, U., and Schubert, H. (2008). Phytoplankton indicators for ecological classification of coastal waters along the German Baltic coast, Rostock Meeresbiolog. Beitr 20, 45–69.
Saraiva, S., van der Meer, J., Kooijman, S. A. L. M., and Sousa, T. (2011). Modelling feeding processes in bivalves: a mechanistic approach. Ecol. Modell. 222, 514–523. doi: 10.1016/j.ecolmodel.2010.09.031
Scheffer, M., Carpenter, S., Foley, J. A., Folke, C., and Walker, B. (2001). Catastrophic shifts in ecosystems. Nature 413:591. doi: 10.1038/35098000
Schernewski, G., Friedland, R., Buer, A.-L., Dahlke, S., Drews, B., Höft, S., et al. (2018). Ecological-social-economic assessment of zebra-mussel cultivation scenarios for the Oder (Szczecin) Lagoon. J. Coast. Conserv. 1–17. doi: 10.1007/s11852-018-0649-2
Schernewski, G., Friedland, R., Carstens, M., Hirt, U., Leujak, W., Nausch, G., et al. (2015). Implementation of European marine policy: new water quality targets for German Baltic waters. Mar. Policy 51, 305–321. doi: 10.1016/j.marpol.2014.09.002
Schernewski, G., Neumann, T., and Behrendt, H. (2011). “Sources, Dynamics and Management of Phosphorus in a Southern Baltic Estuary,” in The Baltic Sea Basin, eds J. Harff, S. Björck, and P. Hoth (Heidelberg: Springer Berlin), 373–388. doi: 10.1007/978-3-642-17220-5_18
Schernewski, G., Stybel, N., and Neumann, T. (2012). Zebra mussel farming in the Szczecin (Oder) Lagoon: water-quality objectives and cost-effectiveness. Ecol. Soc. 17:4. doi: 10.5751/ES-04644-170204
Schneider, D., Madon, S., Stoeckel, J., and Sparks, R. E. (1998). Seston quality controls zebra mussel (Dreissena polymorpha) energetics in turbid rivers. Oecologia 117:331. doi: 10.1007/s004420050666
Schneider, D. W. (1992). A Bioenergetics Model of Zebra Mussel, Dreissena polymorpha, Growth in the Great Lakes. Can. J. Fish. Aquat. Sci. 49, 1406–1416. doi: 10.1139/f92-156
Schröder, T., Stank, J., Schernewski, G., and Krost, P. (2014). The impact of a mussel farm on water transparency in the Kiel Fjord. Ocean Coast. Manag. 101, 42–52. doi: 10.1016/j.ocecoaman.2014.04.034
Schulze-Böttcher, K. (2014). Potential und aktueller Stand der Kultivierung von Dreissena polymorpha zur Verbesserung des ökologischen Zustandes des Usedomer Sees. Masterarbeit: Ernst-Moritz-Arndt Universität Greifswald.
Schumacher, J., Schernewski, G., Bielecka, M., Loizides, M. I., and Loizidou, X. I. (2018). Methodologies to support coastal management - A stakeholder preference and planning tool and its application. Mar. Policy 94, 150–157. doi: 10.1016/j.marpol.2018.05.017
Skubinna, J. P., Coon, T. G., and Batterson, T. R. (1995). Increased abundance and depth of submersed macrophytes in response to decreased turbidity in saginaw Bay, Lake Huron. J. Great Lakes Res. 21, 476–488. doi: 10.1016/S0380-1330(95)71060-7
Slavik, K., Lemmen, C., Zhang, W., Kerimoglu, O., Klingbeil, K., and Wirtz, K. W. (2018). The Large Scale Impact of Offshore Windfarm Structures on Pelagic Primary Production in the Southern North Sea. Hydrobiologia. Available online at: https://arxiv.org/abs/1709.02386
Stadmark, J., and Conley, D. J. (2011). Mussel farming as a nutrient reduction measure in the Baltic Sea: consideration of nutrient biogeochemical cycles. Mar. Pollut. Bull. 62, 1385–1388. doi: 10.1016/j.marpolbul.2011.05.001
Stoeckmann, M. A., and Garton, D. (2011). A Seasonal Energy Budget for Zebra Mussels (Dreissena polymorpha) in Western Lake Erie. Can. J. Fish. Aquat. Sci. 54. 2743–2751. doi: 10.1139/cjfas-54-12-2743
Stybel, N., Fenske, C., and Schernewski, G. (2009). “Mussel Cultivation to Improve Water Quality in the Szczecin Lagoon, Journal of Coastal Research, Special,” Proceedings of the 10th International Coastal Symposium ICS 2009, Vol. II (Lisbon), 1459–1463.
Thomas, Y., Mazurié, J., Alunno-Bruscia, M., Bacher, C., Bouget, J.-F., Gohin, F., et al. (2011). Modelling spatio-temporal variability of Mytilus edulis (L.) growth by forcing a dynamic energy budget model with satellite-derived environmental data. J. Sea Res. 66, 308–317. doi: 10.1016/j.seares.2011.04.015
Voss, M., Dippner, J. W., Humborg, C., Hürdler, J., Korth, F., Neumann, T., et al. (2011). History and scenarios of future development of Baltic Sea eutrophication. Estuar. Coast. Shelf Sci. 92, 307–322. doi: 10.1016/j.ecss.2010.12.037
Weber, G. M., and Windisch, W. (2017). Producing Sufficient “Animal-source protein for the growing world population,” in Sustainable Nutrition in a Changing World, eds H. Biesalski, A. Drewnowski, J. Dwyer, J. Strain, P. Weber, and M. Eggersdorfer (Cham: Springer). doi: 10.1007/978-3-319-55942-1_25
WFD (2000). Commission of the european communities. Directive 2000/60/EC of the european parliament and of the council of 23 october 2000 establishing a framework for community action in the field of water policy. Off. J. Eur. Commun. L 327, 1–72.
Wollak, B., Forss, J., and Welander, U. (2018). Evaluation of blue mussels (Mytilus edulis) as substrate for biogas production in Kalmar County (Sweden). Biomass Bioenergy 111, 96–102. doi: 10.1016/j.biombioe.2018.02.008
Wolnomiejski, N., and Witek, Z. (2013). The Szczecin Lagoon Ecosystem: The Biotic Community of the Great Lagoon and its Food Web Model. London: Walter de Gruyter. doi: 10.2478/9788376560502
Woznicka, A., Wawrzyniak-Wydrowska, B., Radziejewska, T., and Skrzypacz, A. (2016). The quagga mussel (Dreissena rostriformis bugensis Andrusov 1897) – another Ponto-Caspian dreissenid bivalve in the southern Baltic catchment: the first record from the Szczecin Lagoon. Oceanologia 58, 154–159. doi: 10.1016/j.oceano.2015.12.002
Yu, N., and Culver, D. A. (1999). Estimating the effective clearance rate and refiltration by zebra mussels, Dreissena polymorpha, in a stratified reservoir. Freshw. Biol. 41, 481–492. doi: 10.1046/j.1365-2427.1999.00393.x
Zaiko, A., and Daunys, D. (2015). Invasive ecosystem engineers and biotic indices: Giving a wrong impression of water quality improvement? Ecol. Indic. 52 292–299. doi: 10.1016/j.ecolind.2014.12.023
Zettler, M. L., Darr, A., Labrenz, M., Sagert, S., Selig, U., Siebert, U., et al. (2017a). Biological indicators. in Biological Oceanography of the Baltic Sea, eds P. Snoeijs-Leijonmalm, H. Schubert, and T. Radziejewska (Dordrecht: Springer). doi: 10.1007/978-94-007-0668-2_14
Keywords: eutrophication, sustainable aquaculture, site selection, ecosystem modeling, WFD, MSFD
Citation: Friedland R, Buer A-L, Dahlke S and Schernewski G (2019) Spatial Effects of Different Zebra Mussel Farming Strategies in an Eutrophic Baltic Lagoon. Front. Environ. Sci. 6:158. doi: 10.3389/fenvs.2018.00158
Received: 04 July 2018; Accepted: 17 December 2018;
Published: 15 January 2019.
Edited by:
Rathinam Arthur James, Bharathidasan University, IndiaReviewed by:
Chellandi Mohandass, National Institute of Oceanography (CSIR), IndiaVignesh Sivanandham, Bharathidasan University, India
Copyright © 2019 Friedland, Buer, Dahlke and Schernewski. This is an open-access article distributed under the terms of the Creative Commons Attribution License (CC BY). The use, distribution or reproduction in other forums is permitted, provided the original author(s) and the copyright owner(s) are credited and that the original publication in this journal is cited, in accordance with accepted academic practice. No use, distribution or reproduction is permitted which does not comply with these terms.
*Correspondence: René Friedland, cmVuZS5mcmllZGxhbmRAaW8td2FybmVtdWVuZGUuZGU=