- Department of Renewable Resources, University of Alberta, Edmonton, AB, Canada
The mining of oil sands in Alberta, Canada is a large-scale disturbance that requires land reclamation to equivalent land capability. Cover soils used for upland forest reclamation are forest floor mineral-mix (FFM) sourced from upland forest ecosystems and peat mineral-mix (PM) sourced from lowland ecosystems. Spatial heterogeneity and quantity of soil resources, especially nutrient bioavailability, is important because it affects the establishment of native vegetation in forest ecosystems and soil respiration is an indicator for overall soil biologic activity. We studied spatial heterogeneity of soil nutrients as well as seasonal and spatial patterns of soil respiration in two sites reclaimed either with FFM or PM and two reference sites recovering either from harvest or fire. Contrary to our initial hypothesis, we identified spatial heterogeneity in some bioavailable nutrients in FFM and PM cover soil, indicating that standard placement processes can recreate spatial heterogeneity. However, some nutrients such as P, K and S showed no heterogeneity in PM at all. P and K availability was significantly lower and S was significantly higher in PM than in FFM and reference sites. Seasonal pattern of respiration showed variability on natural reference sites and on FFM, indicating that disturbance had not removed belowground function completely. PM reclaimed sites showed no strong seasonal respiration patterns indicating homogeneous belowground function. Surprisingly PM treatments were not characterized by highest rates of soil respiration, while having highest amounts of total organic carbon. We conclude that FFM reclaimed sites may be more successfully reclaimed than PM sites because they are more similar to reference sites in terms of nutrient status and seasonal respiration patterns.
Introduction
Surface mining of oil sands in the Athabasca Oil Sands Region (AOSR), Alberta, Canada creates a large-scale ecosystem disturbance requiring land reclamation (Alberta, 2014). At the end of 2015, 94,095 ha have been disturbed by oil sands surface mining, 6,163 ha have been permanently reclaimed, and 104 ha of the total disturbed area has been certified reclaimed (Environment and Parks, 2015). Operators are required to reclaim disturbed land to equivalent land capability (Alberta, 2014). The presence and growth of local plant species are used to assess recovery of boreal forests on these disturbed areas (Alberta, 2013; Environment and Parks, 2015). Reclamation targeting upland forests is realized by placing cover soil over suitable subsoil or overburden and planting locally common tree species. Cover soil materials used for reclamation are forest floor mineral-mix (FFM) when salvaged from upland forest ecosystems (Alberta Environment Water, 2012), and peat or peat mineral-mix (PM), when salvaged from bogs and fens, which are characterized by higher contents of organic materials (Alberta Environment Water, 2012). Early vegetation recovery using FFM as cover soil has been demonstrated to be more successful (Mackenzie and Naeth, 2010). This may be caused by the root propagules and seeds present in FFM in contrast to PM but also by differences in nutrient status of these materials. However, the material is less abundant than PM in the AOSR, therefore PM will be used in most reclamation sites targeting upland forest (Alberta Environment Water, 2012; Pinno and Hawkes, 2015). The successful establishment of native plant species is becoming a more important parameter in the context of certification of reclaimed sites, and there is an interest to understand what drives this in an economical but also ecological context in boreal Alberta. However, the potential of FFM and PM reclamation to support native plant species is uncertain.
Boreal ecosystems are naturally disturbed by fire, insect or disease outbreaks, windthrow, landslides, and erosion, which depending on intensity, type and prevalence affect vegetation and biogeochemical cycling (Maynard et al., 2014). However, wildfire is the most significant non-anthropogenic disturbance in the boreal forest (Armstrong, 1999) and is the major stand-renewing event in Canada's boreal zone (Brandt et al., 2013). Human based disturbances of boreal forest are related to resource extraction (e.g., harvesting and mining), but also to acid deposition and flooding (Maynard et al., 2014). For boreal mixedwood forests of Alberta, it has been demonstrated that 10 years after clearcut harvesting, soil properties are not adversely affected (Kishchuk et al., 2015). Harvested and post-fire sites are known to reestablish native vegetation in forests (Halpern, 1989). Given the successful regeneration of forest stands following harvesting or fire, we used a burned and harvested site as a reference to evaluate the performance of upland mine reclamation sites.
Spatial heterogeneity of soil nutrients has been demonstrated to be an essential factor in recovering forests, as low resource heterogeneity might support the establishment of species that can cope with high levels of competition; or weaken species that need unique regeneration niches for successful reestablishment (Fraterrigo et al., 2005). Nutrient heterogeneity is also influencing spatial heterogeneity of other organisms (e.g., ants and soil biota) indirectly by influencing plant community composition (Fraterrigo et al., 2005). Spatial patterns of nutrient availability might also be important for quantifying the successful reestablishment of native plant species on reclamation sites in the AOSR but has not been studied to date.
Soil respiration is an important component of belowground function. It is driven by heterotrophic respiration from microbes and soil fauna, and autotrophic respiration from roots (Hanson et al., 2000). The dissolution of carbonates can affect the C flux from soil and may lead to an over estimate of biologic activity (Tamir et al., 2011). Soil respiration is known to be one of the largest fluxes of the terrestrial carbon cycle and the primary path for returning CO2 fixed by plants to the atmosphere (Schlesinger and Andrews, 2000). Small changes in soil respiration at the regional scale, such as with reclamation in the AOSR, could potentially have large influences on the atmospheric CO2 concentration (Schlesinger and Andrews, 2000). Adding organic C to soils results in increased rates of soil respiration (Högberg and Ekblad, 1996). This indicates that using PM for land reclamation might increase the CO2 flux on a landscape scale in the AOSR and result in functional differences compared to established forest ecosystems (source vs. sink). However, this has not been investigated to date. A recent study conducted on aspen stands recovering from fire in the AOSR detected that above- and belowground functions were linked to soil respiration and suggested that reclamation success might be evaluated by spatial heterogeneity of this parameter (Das Gupta and Mackenzie, 2016).
The goal of this study is to identify if reclaimed sites can provide similar niches for organisms in terms of nutrient availability and its spatial heterogeneity that can be found in reference sites present in the AOSR which are recovering from fire or harvest. These sites are used as a reference because they are known to re-establish the native vegetation targeted by reclamation. Furthermore, we investigate spatial and seasonal patterns of soil respiration in order to assess the success of early ecosystem recovery.
We hypothesized that harvested and post-fire sites will exhibit a more spatially heterogeneous pattern with differences in soil nutrient availability, as both cover soil salvage and placement will lead to homogenization and reduced spatial heterogeneity of soil nutrient availability. We also hypothesize that soil nutrient status will differ between reclaimed and reference sites as handling of soil will lead to a tillage like effect that will increase nutrient mineralization. Thirdly, we hypothesize that soil respiration will follow a seasonal pattern on reference sites and FFM reclaimed site where vegetation recovery proceeds faster compared to PM.
Methods
Study Sites
Data was collected during the 2013 growing season in the AOSR north of Fort McMurray (57°20'12.25“N 111°32'22.92”W), Alberta, Canada. Two reclamation sites at Syncrude's Aurora Soil Capping study (ASCS) were used, one reclaimed with FFM in 2012 (Figure 1a) and one reclaimed with PM in 2012 (Figure 1b). On reclamation sites aspen, jack pine, and white spruce seedlings and a mix of understory species were planted before measurements were taken. However, no effects on reclaimed soils and soil respiration measurements at this point were anticipated as root networks likely did not develop toward respiration collars. Reclaimed sites were compared to a reference site naturally recovering from harvesting in 2006 (Figure 1c), classified as type ab ecosite (Beckingham and Archibald, 1996), and a site regenerating from wildfire in 2012 (Figure 1d), classified as type b ecosite (Beckingham and Archibald, 1996). The xeric to subxeric and nutrient-poor type a ecosites are characterized by jack pine, bearberry, bog cranberry, blueberry, twin-flower, sand heather, wild lily-of-the-valley, Schreber's moss, awned hair-cap, and reindeer lichen (Beckingham and Archibald, 1996). The for type a ecosites typical Jack pine stands are commonly open canopied and lichen cover the forest floor, which is characterized by a thin organic layer (Beckingham and Archibald, 1996). Type b ecosites are characterized by subxeric to submesic moisture regimes and poor to medium nutrient regimes (Beckingham and Archibald, 1996). Vegetation typical for type b ecosites includes for example jack pine, aspen, white birch, bog cranberry, blueberry, green alder, bearberry, labrador tea, twin-flower, buffalo-berry, or prickly rose (Beckingham and Archibald, 1996). Summers in the region are short and warm while winters are long and cold (Natural Regions Committee, 2006). The coversoil on the FFM reclaimed site consisted of 20 cm FFM over 130 cm of subsoil salvaged from below 100 cm, while the coversoil on the PM reclaimed site consisted of 30 cm of peat over 120 cm subsoil. FFM was salvaged from the LFH and the top 10–15 cm of soil from a sand-textured upland type a or b ecosites. The texture of the topsoil (0–10 cm depth) of the FFM site was characterized as sandy loam to loamy sand. PM was salvaged from a j ecosite (poor fen), which was characterized by an intermediate nutrient regime and a subhydric moisture regime (Beckingham and Archibald, 1996), as well as from a k ecosite (rich fen), which is characterized by a rich nutrient regime and a subhydric moisture regime (Beckingham and Archibald, 1996). Soils of both fen ecosite types were composed of organic matter (OM) derived from sedges, golden, tufted and brown moss, and peat moss (Beckingham and Archibald, 1996). Soils found on the reference sites were characterized as Orthic Dystric Brunisol at the Harvestsite with sandy loam to loamy sand texture (0–10 cm depth); and Orthic Gray Luvisol with loamy texture (0–10 cm depth) at the Fire site. Basic soil characteristics of topsoils collected at all sites are reported in Table 1. All plots were set up in plane areas to minimize effects of slope and resulting effects on the moisture regime. Due to the high sampling intensity per treatment replicates of treatments were not established.
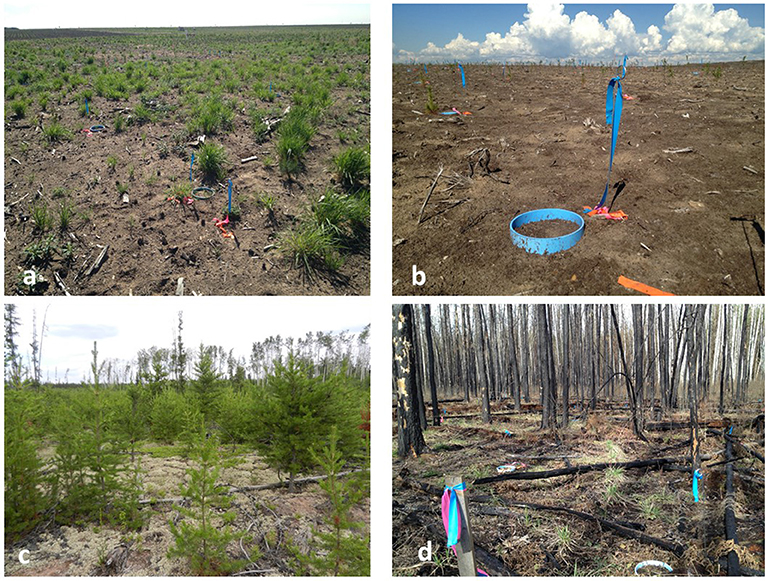
Figure 1. Pictures of sites compared in this study. (a) Forest Floor Mineral Mix (FFM) reclaimed site; (b) Peat Mineral Mix (PM) reclaimed site; (c) Harvested ab ecosite; (d) Fire b ecosite.
Spatial Sampling
A spatial sampling protocol was used to collect data on bioavailable nutrients (81 points) (Figure 2A) and soil respiration (42 points) (Figure 2B) in a 20 × 50 m plot. We followed the approach used by Smithwick et al. (2005) who suggested cyclic sampling design, which is reversed in the middle three rows to account for anisotropy. Using a cyclic sampling design allowed us the study of spatial patterns due to the establishment of comparable power at diverse lag distances and maximized the effectiveness of sampling (Clayton and Hudelson, 1995). The minimum detectable spatial lag was 0.5 m for bioavailable nutrients and 2 m for soil respiration. Collected spatial data is available as Supplementary Material.
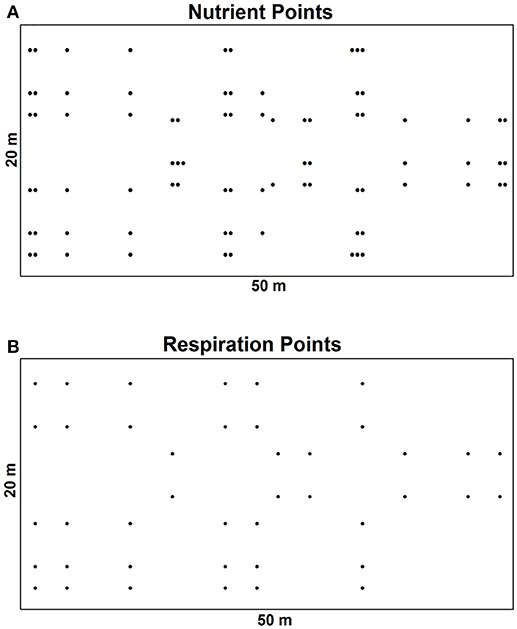
Figure 2. Spatial sampling protocol for data collection during the growing season of 2013 in sites in the Athabasca oils sands region. (A) Design for measurement of bioavailable nutrients with 81 sampling points. (B) Design for measurement of soil respiration with 42 sampling points.
Bioavailable Nutrients
Bioavailable nutrients were measured by installing Plant-Root-Simulator™ probes (PRS) in situ (n = 81) during the growing season in the topsoil of research sites according to the manufacturer instructions (Western Ag Innovations, Saskatoon, SK). Probes were installed over a 57-day period from June–August 2013 in the topsoil (0–10 cm depth) of all sites. PRS™ probes employ membrane-bound ionic exchange resins and are designed to capture nutrient anions and cations available in the soil solution. Probes were incubated in situ during the growing season of 2013. The use of PRS technology allows a more realistic approach than conventional soil tests for evaluating rhizosphere conditions as labile inorganic nutrients are measured in-situ with little disturbance and avoid the use of extractants on soil (Qian and Schoenau, 2002). Upon removal from sites at the end of August, probes were immediately cooled, transported to the lab and rinsed with de-ionized water and sent to Western Ag Innovations. Probes were extracted with 0.5 M HCl and analyzed for NH and NO by colorimetry (FIAlab 2600), and by ICP-OES (Perkin Elmer ICP-OES 8300) for Al, B, Cu, P, K, Mn, Zn, Fe, Mg, Ca, and S.
Soil Respiration
Soil respiration was measured monthly during the growing season in May, June, July, and August using a LiCor LI-8100 Soil Gas Flux System (LI-COR, Lincoln, Nebraska, USA) on preinstalled custom made 8” diameter respiration collars. Collars were installed to a depth of 2”, and headspace was measured to ensure the accuracy of measurements. Measuring soil respiration allows to evaluate in-situ soil metabolic activity producing CO2, resulting out of autotrophic and heterotrophic respiration, and eventually the chemical oxidation of carbonaceous compounds (Lundegårdh, 1927; Bunt and Rovira, 1954).
Statistical Analysis
R 2.1.5 (R Core Team, 2012) was used for statistical analyses with agricolae package (de Mendiburu, 2014) for post-hoc testing with Tukey HSD test, following permutational ANOVA in the lmPerm package (Wheeler, 2010) for significance testing to compare means of both nutrient bioavailability and soil respiration. Permutational ANOVA was chosen for analysis to account for violation of ANOVA assumptions and sample size.
Multivariate statistics were used to examine the overall nutrient profile. Principal component analysis (PCA) was carried out with the ggbiplot package (Vu, 2011) in R3.3.1 (R Core Team, 2016). Data were standardized and normalized before PCA by log transformation and adding a constant [log10(x + 100)].
Isotropic variograms were fitted with GS+ 10.0 (Gamma Design Software). Four variogram models were tested (Linear, Exponential, Spherical, and Gaussian) to identify the model that best fitted the data. The residual sum of squares (RSS) was used to identify the best model fit. Following ordinary and standardized kriging maps were plotted to visualize spatial characteristics using GS + 10.0 (Gamma Design Software). Cross-validation was used to evaluate kriging interpolations, which is based on a comparison of estimated vs. actual measured values.
The nugget coefficient, nc, which is calculated from total variance (c0 + c1) and the associated nugget variance (c0) was used to calculate spatial dependence. If the nugget coefficient is >75 strong spatial dependence is indicated, 25–75 indicates moderate spatial dependence, and a value <25 indicates poor or no spatial dependence (Isaaks and Srivastava, 1989). Also, the coefficient of variation (CV) was calculated to provide a measure of global variation. The CV is calculated by dividing the standard deviation by the mean and was reported as a percentage. Additionally, the spatial range which is the distance beyond two points where no more spatial autocorrelation can be detected was calculated in GS+ to allow for conclusions on the scale of underlying processes.
Results
Ordination of Bioavailable Nutrients
Macronutrients
To evaluate the overall differences in the macronutrient profiles of reference and reclamation sites PCA was conducted on the data (Figure 3). PC1 and PC2 explained 78.4% of the variation within the four treatments. Differences between reference and reclamation treatments are mainly explained on the axis of PC1, which explained 59.9 % variation (Figure 3). Increased bioavailable P and K was positively associated with the Harvest and the Fire site and shifted those treatments to the right of PC 1 (Figure 3). Reclamation treatments PM and FFM were positively associated with increased N, S, and Ca, which shifted those treatments to the left of PC 1 (Figure 3).
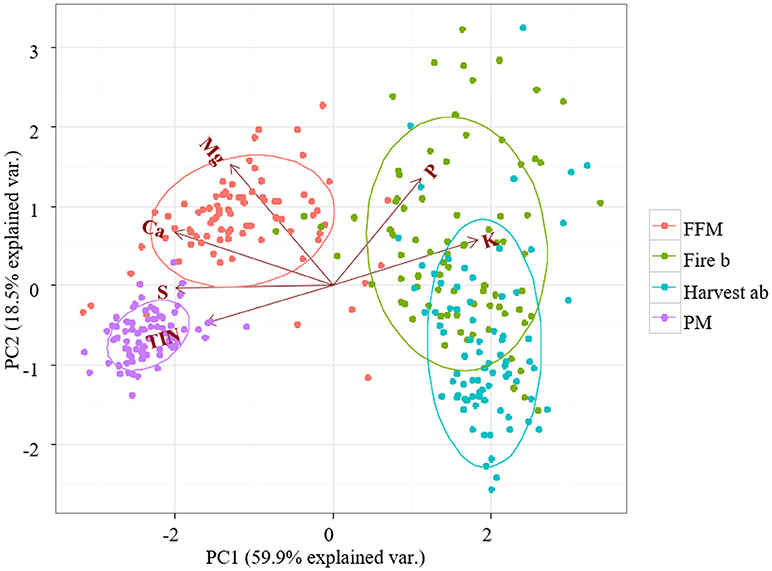
Figure 3. Principal component analysis (PCA) of bioavailable macronutrients. Nutrient bioavailability was measured with PRS™ probes. Peat mineral-mix (PM), forest floor mineral-mix FFM, Harvest ab by timber harvesting disturbed a/b ecosite, Fire b a by forest fire disturbed type b ecosite.
The axis of PC 2, which explained 18.5% variation was positively associated to increased P and Mg availability and shifted FFM and the Fire treatment upwards compared to the PM and Harvest treatments (Figure 3).
Ellipses are indicating the 68% confidence interval for treatments overlapped for the Fire and Harvest site indicating great similarity. The 68% confidence interval ellipse for FFM was closer to the reference treatments than the ellipse for PM, indicating greater similarity of FFM to reference sites. The ellipse for PM was smaller compared to other treatments indicating a reduced overall variability.
Micronutrients
PCA of bioavailable micronutrients explained a total of 64.3% on the axes of PC1 and PC2. All treatments overlapped within their 68% confidence interval (Figure 4). The reclamation treatments PM and FFM showed a shift to the left on the axis of PC1, which was associated with increased B, Fe, Al, and Cu availability. A downward shift of FFM, Harvest, and the Fire site on the axis of PC2 were associated with increased Mn and Zn availability (Figure 4).
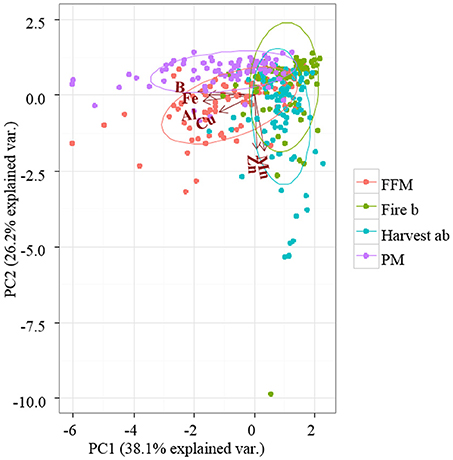
Figure 4. Principal component analysis (PCA) of measured bioavailable micronutrients. Nutrient bioavailability was measured with PRS™ probes. Peat mineral-mix (PM), forest floor mineral-mix (FFM), Harvest ab by timber harvesting disturbed a/b ecosite, Fire b a by forest fire disturbed type b ecosite.
Spatial Patterns of Bioavailable Nutrients
Nitrogen
Mean bioavailable N was highest on the PM treatment, which was significantly greater than the mean N availability on FFM, Harvest, and Fire (Table 2). Mean N availability on PM was about 100% greater than on FFM, and more than 1,000% greater than on the reference sites (Table 2). Global variation (CV) was greatest on FFM, followed by Fire, Harvest, and lowest on PM.
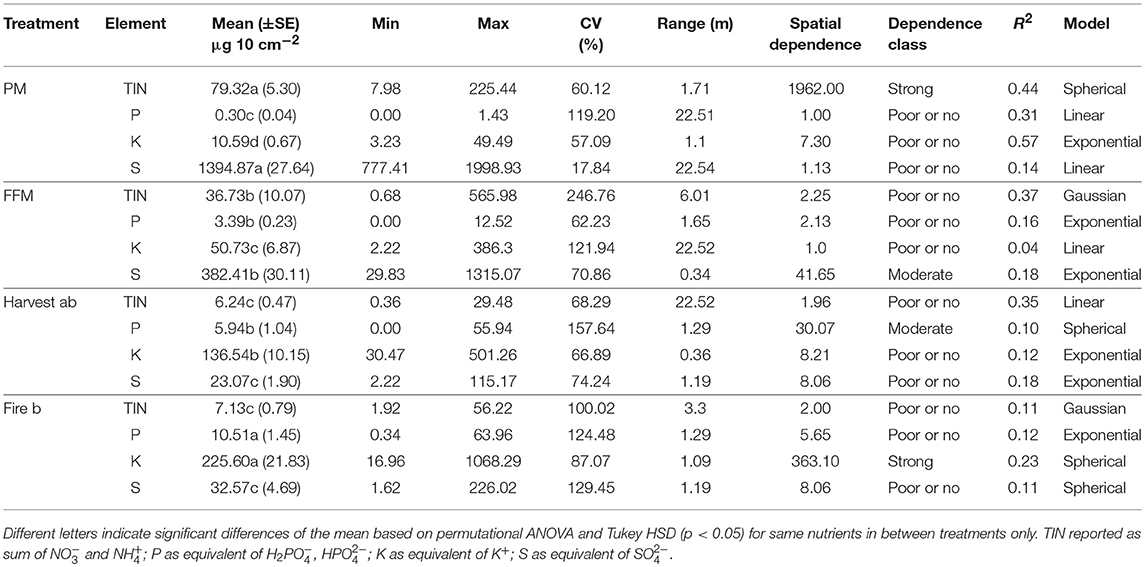
Table 2. Selected macro nutrient characteristics for sites including peat mineral-mix (PM), forest floor mineral-mix (FFM), Harvest (ab) by timber harvesting disturbed a/b ecosite, Fire (b) a by forest fire disturbed type b ecosite.
Nitrogen had the finest spatial range on PM, followed by Fire, FFM, and Harvest which showed larger scale spatial autocorrelation than other sites (Table 2). Kriging maps supported and visualized those findings showing fine points with lower N availability, but also higher N availability than the mean N availability in PM. The left side of the kriging map showed a vast area with higher variation in N bioavailability, while to the right N bioavailability ranged from 100 to 200 μg 10 cm−2 N showing low variability (Figure 5). The Fire site showed predicted variation at much lower N bioavailability, which was slightly higher than on FFM (Figure 5). On FFM kriging maps (right) areas were predicted that were similar in N bioavailability as on the reference sites Fire and Harvest, however also areas with high N bioavailability (left, Figure 5). The Harvest site showed predicted vast areas with low N bioavailability (Figure 5). Strong spatial dependency of N bioavailability was only observed for the PM reclaimed sites, while all other sites showed poor or no spatial dependency.
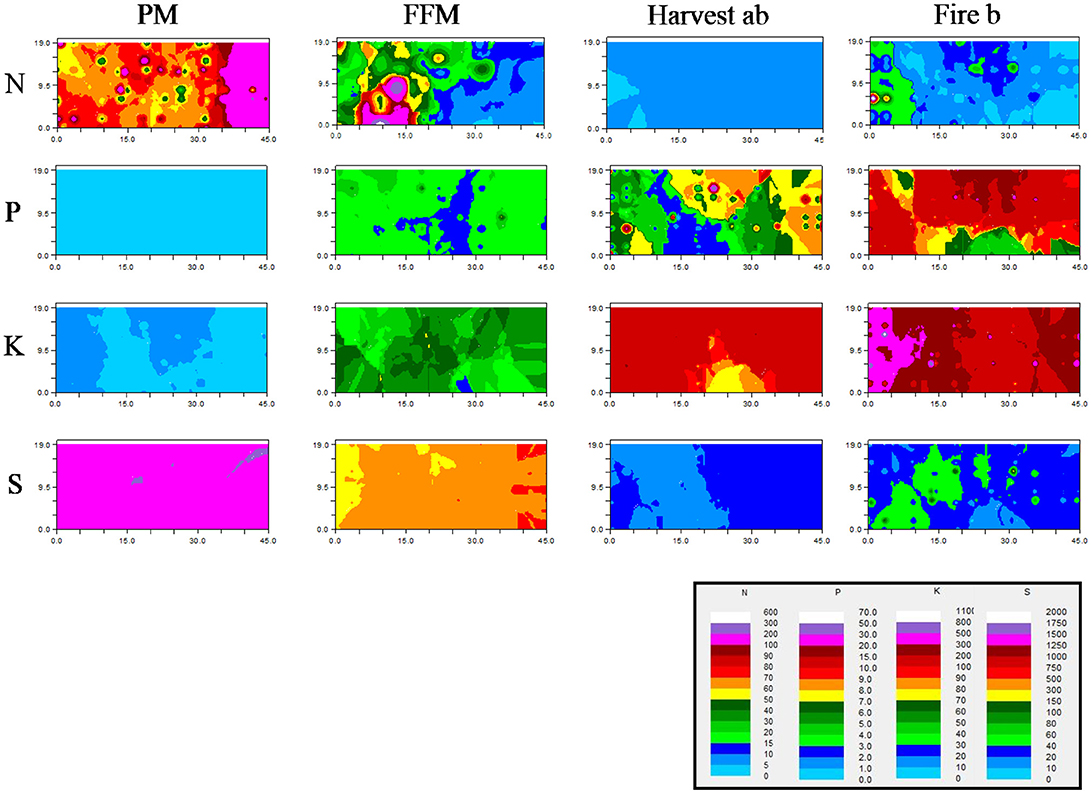
Figure 5. Kriging maps of bioavailable N, P, K, and S based on fitted variogram models. Modeled spatial nutrient bioavailability is expressed in μg 10 cm−2. Peat mineral-mix (PM), forest floor mineral-mix (FFM), Harvest ab by timber harvesting disturbed a/b ecosite, Fire b a by forest fire disturbed type b ecosite. TIN reported as sum of NO and NH; P as equivalent of H2PO, HPO; K as equivalent of K+; S as equivalent of SO.
Phosphorus
Mean bioavailable P was highest on the Fire site, followed by Harvest and FFM, which both showed no significant difference in mean P bioavailability (Table 2). PM had a significantly lower mean P bioavailability compared to all other treatments (Table 2). Global variation of P was greatest on Harvest followed by Fire, PM and was smallest on FFM (Table 2).
The spatial range of P was coarse on PM with an autocorrelation >22 m while being fine on all other sites where spatial autocorrelation was detected at a range of about 1.5 m (Table 2). Kriging maps predicted a very low to not existing P availability with little to no variation on PM (Figure 5). Kriging maps of FFM indicated greater variability and increased availability of P compared to PM, but visually lower than on the Harvest site, which showed areas with lower availability, but also hot spots. The Fire site was predicted to have bigger areas with high P availability and some spots with moderate P availability compared to other sites but also some smaller hotspots (Figure 5). Spatial dependency was poor or not existent for all sites except for Harvest where a moderate spatial dependency was detected.
Potassium
Mean K bioavailability was highest on Fire, followed by Harvest, FFM, and PM (Table 2). The mean K bioavailability was more than 2,000% greater on Fire compared to PM (Table 2), and K availability on Harvest was about 1,300% times greater compared to PM (Table 2).
The global variation was greatest on FFM, followed by Fire, Harvest, and PM (Table 2).
Spatial autocorrelation of K was very fine with a range of 0.36 m on the Harvest site (Table 2), followed by Fire and PM, which were also characterized by a finer spatial range compared to FFM were a coarse autocorrelation with a range >22 m was detected (Table 2). Kriging maps predicted larger areas with lower K bioavailability but including spots with slightly increased or reduced variability within those for PM (Figure 6). In FFM kriged maps showed a random pattern with three hotspots (Figure 6). The Harvest kriged map predicted wide areas with higher K availability and some areas with slightly lower K bioavailability (Figure 6), which compared to the Fire site showed lower availability and variability (Figures 2–6). A hotspot with up to 1,100 μg K 10 cm−2 was predicted for the Fire site (Figure 6).
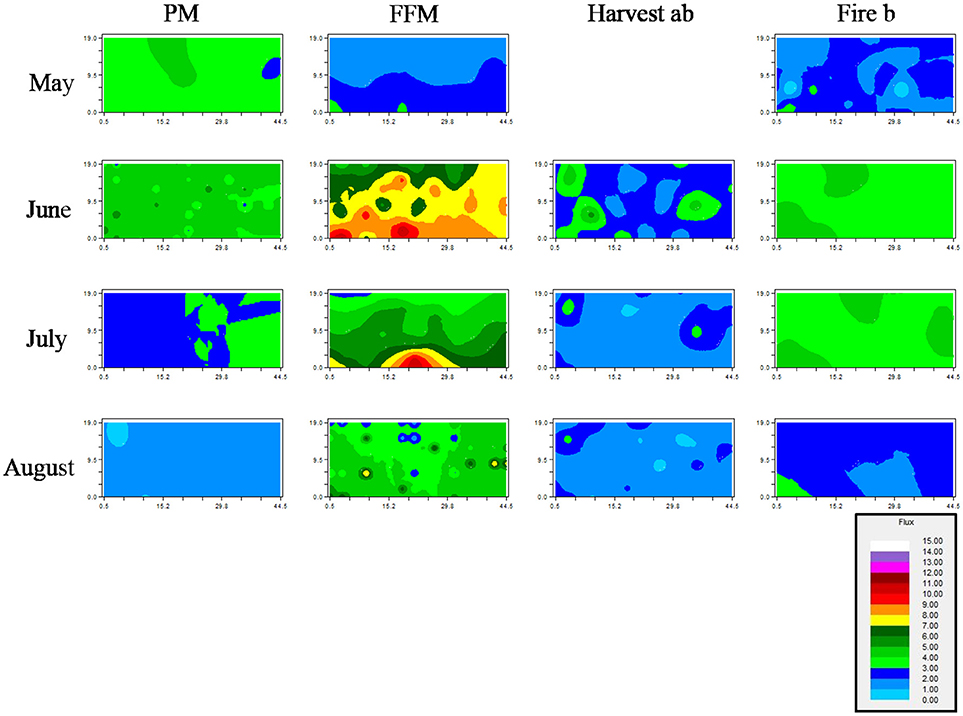
Figure 6. Kriging maps of soil respiration based on fitted variogram models. Modeled spatial soil respiration is expressed in μmol CO2 m−2 s−1. Peat mineral-mix (PM), forest floor mineral-mix (FFM), Harvest ab by timber harvesting disturbed a/b ecosite, Fire b a by forest fire disturbed type b ecosite.
No or poor spatial dependence was detected for K bioavailability at PM, FFM, and Harvest, while moderate spatial dependence was detected for Fire (Table 2).
Sulfur
Mean Sulfur bioavailability was significantly higher on PM, compared to FFM, Harvest, and Fire (Table 2). Sulfur bioavailability significantly greater as on Harvest and Fire (Table 2). The Sulfur availability on PM was about 400% greater compared to FFM and more than 4,000% greater compared to Harvest and Fire (Table 2). The global variation for S was greatest on Fire, followed by Harvest, and FFM while being much lower on PM (Table 2).
Spatial autocorrelation was very coarse on PM with a range >22 m and very fine on FFM with a range of 0.34 m (Table 2). On Harvest and Fire a fine range was detected (Table 2). The kriging map for S bioavailability showed little to no variability in PM and overall very high S bioavailability compared to all other treatments (Figure 6). FFM showed compared to Harvest and Fire an overall higher availability of S, with greater variation compared to PM (Figure 6). Harvest showed wide ranges with similar low (right) to very low (left) while Fire showed areas with slightly higher variability and patches with lower and higher availability (Figure 6). Poor or no spatial dependency was detected for PM, Harvest, and Fire, while FFM showed moderate spatial dependency (Table 2).
Spatial and Seasonal Patterns of Soil Respiration
Peat mineral-mix showed no consistent pattern when visually comparing soil respiration rates from May to August (Figure 6). FFM showed a recurring pattern with higher respiration rates in lower parts of kriged maps and reduced respiration rates in upper parts of kriged maps, which were especially observable for months May, June, and July (Figure 6). On the Harvest site returning patterns with higher and lower activity could be observed as well, when comparing kriged maps for June, July, and August (Figure 6). For example, the top left corner, as well as the bottom left corner, showed higher rates of soil respiration throughout the months of measurements (Figure 6). Similarly, this observation could be made on Fire where areas with returning rates of increased soil respiration could be identified for example in the bottom left corner throughout May, June, July, and August (Figure 6).
May
Mean soil respiration in May was greatest on PM and significantly higher than on FFM and Fire, no measurements could be reported for Harvest due to limitations in site access (Table 3). Soil respiration on PM exceeded FFM by 50% and Fire by about 100%. Global variation was about 300% greater on Fire compared to FFM and about 100% greater compared to PM (Table 3).
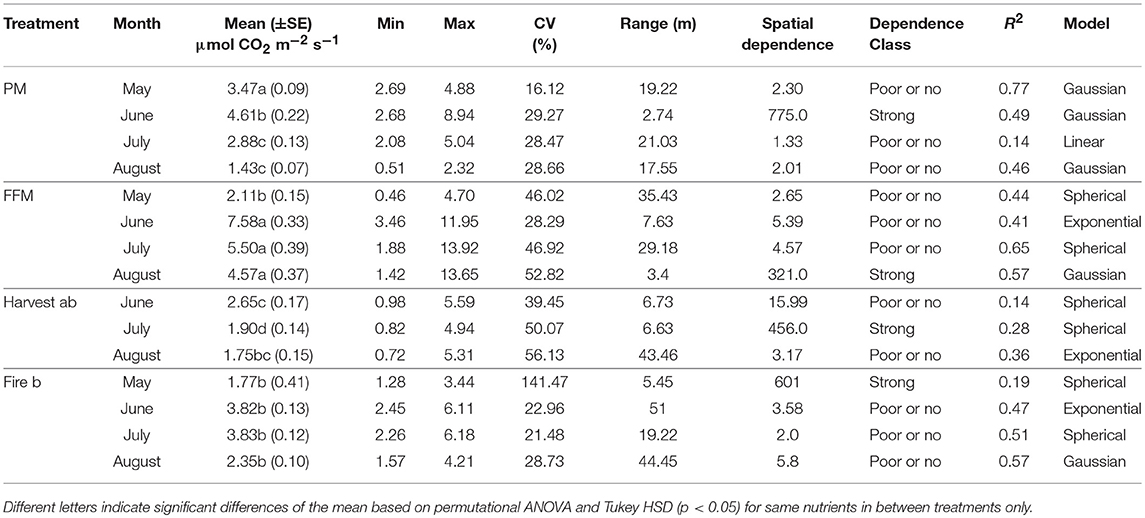
Table 3. Soil respiration of research sites including peat mineral-mix (PM), forest floor mineral-mix (FFM), Harvest ab by timber harvesting disturbed a/b ecosite, Fire b a by forest fire disturbed type b ecosite.
Spatial autocorrelation was fine on Fire, followed by PM and FFM (Table 3). Reclamation sites showed a coarser range, which was greater >22 m on FFM (Table 3). Kriging maps for soil respiration in May predicted higher respiration rates on PM comparted to FFM and Fire (Figure 6). Kriging maps also showed the smaller scale variability on Fire compared to PM and FFM (Figure 6). Spatial dependency was poor or not existent for PM and FFM but strong on Fire (Table 3).
June
Mean soil respiration in June was highest on FFM and significantly exceeded respiration rates of all other sites (Table 3). Respiration rates on PM were second highest but not significantly higher than on Fire (Table 3). Mean respiration was lowest on Harvest (Table 3). Global variation in June was greatest on Harvest followed by PM, FFM, and Fire (Table 3).
Spatial autocorrelation was detected at a fine scale in PM, FFM, and Harvest and at a very coarse scale at Fire (Table 3). Kriging maps of soil respiration confirmed those findings showing smaller patches on PM, FFM, and Harvest compared to Fire b (Figure 6). Spatial dependency of soil respiration was strong on PM but poor or not existent at FFM, Harvest, and Fire (Table 3).
July
Mean soil respiration was highest on FFM followed by Fire, PM and Harvest (Table 3). Global variation was highest on Harvest followed by FFM and on both sides about 75% greater compared to PM and Fire (Table 3).
Spatial autocorrelation was very coarse (>22 m on FFM), and coarse on PM followed by Fire (Table 3). Harvest showed a finer autocorrelation with a range of 6.63 m (Table 3). Kriging maps predicted lower variability on PM and Fire compared to FFM and Harvest (Figure 6). Spatial dependency was poor or not existent during July on PM, FFM, and Fire, while strong dependency was detected on Harvest (Table 3).
August
Mean respiration rates in August were highest on FFM, where respiration rates exceeded respiration of the second highest site Fire by close to 100% (Table 3). Respiration rates on Fire were slightly higher compared to Harvest and significantly higher compared to PM (Table 3). Respiration on Harvest was not significantly higher than on PM (Table 3). Second highest was Harvest followed by Fire, the maximum values here were close to 100% higher than on PM (Table 3). Global variation in August was highest on Harvest followed by FFM, Fire, and PM (Table 3).
Spatial autocorrelation was very coarse (>22 m) on Fire, on Harvest compared to PM also coarse autocorrelation was detected (Table 3). FFM was characterized by a fine scale autocorrelation (Table 3). Kriging maps predicted low respiration rates with little variation for PM (Figure 6). FFM and Harvest, but also Fire showed greater variation (Figure 6). Especially FFM and Harvest showed patches with higher but also lower soil respiration (Figure 6). Spatial dependency was poor or not existent at PM, Harvest, and Fire but strong at FFM (Table 3).
Discussion
Ordination of Bioavailable Nutrients
Macronutrients
Biomass accumulation in forest stands following severe disturbance appears to be linked to nutrient availability (Johnson et al., 2000). Reference sites recovering from disturbance showed measurable differences in bioavailable macronutrients compared to the tested reclamation treatments with FFM being more similar to reference sites than PM. Research has shown that plants species adapted to infertile conditions differ in ecophysiological traits compared to plants that thrive in fertile systems (Wardle, 2002). It is understood that under conditions of fluctuating resource availability, sites are more susceptible to the invasion of non-native or unwanted species (Davis et al., 2000). This indicates that young PM reclamation sites with elevated nutrient profiles (especially N) and no seed bank for vegetation establishment might be prone to invasion of undesirable species. Additionally, the availability of soil minerals, which is low in PM sites, is most likely playing a key role in providing beneficial soil microorganisms (e.g., mycorrhizal fungi) to plants (Reynolds et al., 2003). For forest ecosystems, it is suggested that resource quantity is a key driver for understory diversity during stages of stem exclusion and mature stages when resource quantity is low, while resource heterogeneity might be of greater importance for understory diversity in old growth forests (Bartels and Chen, 2010). Hence, it might be beneficial to make profiles of bioavailable nutrients in both resource quantity and heterogeneity more similar to natural references when targeting type a or b ecosites as an end goal for oil sands mine reclamation.
Micronutrients
Boron deficiency has been reported to have the potential to cause stunted growth, poor tree survival, and stem defects in natural forest stands following macronutrient fertilization, fire, or erosion (Stone, 1990). For forests stands in the Pacific Northwest, it has also been shown that Mo can limit asymbiotic N fixation (Silvester, 1989). Other studies conducted with slash pine and loblolly pine for which micronutrients deficiencies are uncommon showed that Mn additions can influence growth (Jokela et al., 1991). Data for bioavailable B, Fe, Al, Cu, Zn, and Mn showed a great deal of similarity, but also showed that there is some separation (Figure 4). It is important to understand that micronutrient deficiencies can significantly affect vegetation performance on reclamation sites. This is especially relevant on PM where bioavailability of Cu or Mn might be reduced due to complexation in substrates with high organics and high pH (Broadley et al., 2012). We suggest that micronutrient bioavailability in cover soils should be monitored and eventually controlled.
Spatial Pattern of Bioavailable Nutrients
Nitrogen
In the boreal biome, N bioavailability is considered to be of limiting nature in forest growth and is also determining terrestrial biodiversity or a factor in eutrophication of aquatic ecosystems (Sponseller et al., 2016). The forest floor patchiness can influence spatial patterns of revegetation and regenerated vegetation itself interacts with nutrient availability (Guo et al., 2004). Data from our study showed that global variation in nutrient bioavailability was higher in FFM and Fire compared to Harvest and PM. As vegetation is already established on Harvest and is older compared to PM, FFM, and Fire, comparing site nutrient availability is challenging, as vegetation might contain significant amounts of nutrients. Higher nutrient retention in the biomass of the Harvest treatment is likely, as in early stages following disturbance (harvest) biomass accumulates rapidly binding essential or potentially limiting nutrients (Vitousek and Reiners, 1975). As it has been shown that both above and belowground productivity of young regenerating post-logged and post-fire stands does not differ (Yuan and Chen, 2013; Seedre et al., 2014); it can be expected that the postfire site will develop on a similar trajectory as the logged site over time, showing an overall reduced availability of N. Overall we observed that N bioavailability was much higher on FFM and PM compared to reference sites following disturbance, potentially opening a window for invasion of unwanted species following the hypothesis of Davis et al. (2000). To reduce overall N bioavailability on reclamation sites, PM or FFM could be placed shallower over suitable overburden or when salvaging materials higher amounts of suitable underlying materials could be stripped to reduce overall N availability in reclamation cover soils. Kriging maps demonstrated that bioavailability of N on both reclamation sites varies, similar to that on Fire. Future studies should investigate if patterns of variable N availability determine species-specific successful plant reestablishment.
Phosphorus
Phosphorus soil chemistry has been shown to be impacted by plant-soil feedbacks in forest stands (Gómez-Aparicio et al., 2017). Plant-soil feedbacks influence species abundance, coexistence, and succession in plant communities (Gómez-Aparicio et al., 2017). In our study reference sites recovering from harvesting and fire showed an overall higher bioavailability of P compared to FFM and PM, with Harvest not being significantly different from FFM. It has been suggested that during stages of secondary boreal forest succession, soil P resources may be outpaced by their demand (Hume et al., 2016), and for that reason, an overall reduced availability on reclamation sites as observed in our study compared to reference sites has to be evaluated critically. Phosphorus availability, as well as N availability, can affect future productivity and C storage of terrestrial ecosystems (Wieder et al., 2015). Kriging maps in our study demonstrated no variation of P on the PM treatment and variability on the FFM treatment that was low compared to Harvest and Fire treatment. Soil P availability has been shown to be a driving factor regulating plant intra-specific competition (Facelli and Facelli, 2002). Plants growing with high available P develop higher mean shoot and root biomass (Facelli and Facelli, 2002). Hence it can be anticipated that in patches with increased P availability vegetation will establish with greater success. Phosphorus can be volatilized at higher fire temperatures in excess of 774°C, and losses can range from 10 to 50% depending on burning intensity, but studies conducted in the United States also reported increases of P in the litter layer following fire (Neary et al., 1999). We suggest that P bioavailability on reclamation sites should be increased to a level that is similar to Fire, as it can be assumed that P availability on Fire included parts of nutrients that were bound in biomass and released following fire. Large-scale autocorrelation of P at low levels is concerning as it might allow the establishment of undesired, non-native species that can better cope with these conditions than native vegetation.
Potassium
Potassium has been shown to be more heterogeneous in soils following fire than other cations such as Ca and Mg (Outeiro et al., 2008). K, as well as P, is readily cycled by plants and is more concentrated in the topsoil (Jobbágy and Jackson, 2001). Potassium is important for enzyme activation and osmoregulation; deficiency predisposes plants to abiotic and biotic stresses (Hawkesford et al., 2012). Areas with higher availability of K to seedlings might increase chances of successful plant establishment. Our study demonstrated that variability following fire was greater than on the harvested site. Reclamation sites showed some variability in K availability but at lower rates. A study conducted on reclamation sites in Alberta identified only coarse scale patterns for K and associated those to the application of PM reclamation cover soil (Sorenson et al., 2017). However, we found autocorrelation at a fine scale comparable to reference sites. Those findings underline that generalizations in the reclamation environment for cover soil quality cannot be made and that specialized assessments are necessary to optimize reclamation site quality.
Sulfur
Sulfur is a macronutrient that can limit tree seedling growth (Ericsson, 1995). However, in excess S can negatively impact plant metabolism and can affect dry weight accumulation, yield, and delay of flowering (Rennenberg, 1984). Increased levels of S have been shown to negatively affect some macroinvertebrates found in Canadian pine forests (Carcamo et al., 1998). Our study demonstrated significantly increased bioavailability of S on both reclamation sites. Sulfur availability was by magnitudes greater on PM compared to reference sites. Sulfur availability on PM had coarse autocorrelation indicating that high values existed throughout the site. Sorenson et al. (2017) reported varying spatial ranges of S bioavailability, which also changed during seasons but found similarly high values in the spruce stand as in our study on PM. They also suggested that the intercept in between canopy cover and S bioavailability is related to high deposition on oil sands reclamation sites (Sorenson et al., 2017), as the canopy cover may have affected Sulfur deposition. Further field studies should investigate how plant phenology and invertebrates are affected by higher S bioavailability on reclamation sites. Potentially topsoil S concentrations could be reduced by blending PM or FFM with materials that contain lower amounts of S like mineral subsoil to reduce the bioavailability.
Spatial and Seasonal Patterns of Soil Respiration
Our study identified reoccurring patterns of specific respiration activity using kriging maps, especially in Harvest, Fire and to some degree in FFM. PM showed no reoccurring pattern suggesting that no biologic legacy governing biologic activity is established at this early stage. In a chronosequence of boreal aspen stands spatial patterns of soil respiration have been reported to link above and below ground function at the canopy closure and mature stage (Das Gupta and Mackenzie, 2016). Our study demonstrates that a similar pattern can be identified visually on forest sites following harvesting monthly, indicating a link of above and belowground function may exist. Contrary to findings of Das Gupta and Mackenzie (2016) we could identify this effect also following fire and to some degree on FFM reclaimed sites. It is likely that the identified link is related to density of plant propagules contained in topsoil of the Fire and FFM site, which are quickly recovering following disturbance. It has been shown that boreal forest plants successfully recover due to resprouting from an intact rhizome, germination from buried seed propagule banks, or dispersal of propagules (Rydgren et al., 1998). Hence, dispersal of seeds might not have played an important role in measured patterns of respiration at this early stage, as roots of dispersed seedlings would not have been capable of forming detectable interactions. Future studies should investigate if reclamation sites develop similar detectable patterns as these potentially could be used as an indicator for a successfully established link of above and belowground ecosystem function.
Possible Methodological Limitations
The spatially explicit sampling design with a high sample size per site, but no site replication, allowed capturing site individual spatial heterogeneity but disallowed significance testing for variogram parameters and global measures of spread. This is a common problem for studies capturing spatial heterogeneity (Smithwick et al., 2005; Bengtson et al., 2007; Lavoie and Mack, 2012). As cover soil materials, but also cover soil placement varies within the AOSR, detected spatial soil resource heterogeneity and quantity can only be extrapolated to sites reclaimed under comparable circumstances. The sampling design for nutrient bioavailability differed from the sampling design for soil respiration and did not allow to link both parameters. However, it allowed evaluating the pattern in respiration described by Das Gupta and Mackenzie (2016). Additionally, heterogeneity of bioavailable nutrients could be evaluated in greater detail. Given the different characteristics of cover soils, there is potential for an error related to the dissolution of carbonates as this may affect the C flux from soil and can lead to an over estimate of biologic activity (Tamir et al., 2011).
Conclusions
Our study demonstrates that reclamation procedures can reestablish heterogeneity in bioavailable nutrients. Targeted P and K fertilization might be used to increase bioavailability on PM reclaimed sites to increase similarity to type a and b ecosites targeted in closure plans. Increasing the mineral soil content of PM either during salvage, stockpile or placement, which is done in oil sands reclamation when the opportunity arises, might be an easy remedy to reduce N and S bioavailability in PM. Spatial heterogeneity of nutrient bioavailability on FFM reclaimed sites likely is a factor that positively affects reestablishment of vegetation. It needs to be tested if vegetation recovery on PM reclaimed sites can be improved by increasing heterogeneity of bioavailable nutrients to a level similar to that in reference sites.
Our study demonstrated that only the Fire, Harvest, and FFM sites showed reoccurring patterns of soil respiration during the growing season indicating that a link in between above and below ground function is established. We conclude that FFM reclaimed sites show ecological function at an early stage and sites have the potential to be certified reclaimed at an early stage. Future work should verify our findings in a blocked and replicated design to confirm our findings.
Author Contributions
SD was involved with data collection and analysis, data interpretation, manuscript formation, manuscript editing. MM was involved with funding, study conception and study design, data collection and analysis, data interpretation, manuscript formation, manuscript editing.
Funding
Natural Sciences and Engineering Research Council of Canada (NSERC)-CREATE. Land Reclamation International Graduate School (LRIGS). Syncrude Canada.
Conflict of Interest Statement
The authors declare that the research was conducted in the absence of any commercial or financial relationships that could be construed as a potential conflict of interest.
Acknowledgments
We acknowledge Dr. Sanatan Das Gupta for support of experimental design and fieldwork, and Mark Howell, Sawyer Desaulniers, and Nicole Filipow for support of fieldwork.
Supplementary Material
The Supplementary Material for this article can be found online at: https://www.frontiersin.org/articles/10.3389/fenvs.2018.00126/full#supplementary-material
References
Alberta (2013). Oil Sands Reclamation. Available online at: http://publications.gc.ca/collections/collection_2011/rncan-nrcan/M164-4-7-1-2011-eng.pdf (Accessed January 27, 2017).
Alberta (2014). “Environmental Protection And Enhancement Act,” in Revised Statutes of Alberta 2000, Chapter E-12. Current as of December 17 2014. ed Government of Alberta (Edmonton: Alberta Queen's Printer).
Alberta Environment and Water (2012). Best management practices for conservation of reclamation materials in the mineable oil sands region of alberta. Prepared by MacKenzie, D. for the terrestrial subgroup. Best Management Practices Task Group of the Reclamation Working Group of the Cumulative Environmental Management Association, March 9, 2011 edn (Fort McMurray, AB: Her Majesty the Queen in right of the Province of Alberta 2012).
Armstrong, G. W. (1999). A stochastic characterisation of the natural disturbance regime of the boreal mixedwood forest with implications for sustainable forest management. Can. J. Forest Res. 29, 424–433. doi: 10.1139/x99-010
Bartels, S. F., and Chen, H. Y. H. (2010). Is understory plant species diversity driven by resource quantity or resource heterogeneity? Ecology 91, 1931–1938. doi: 10.1890/09-1376.1
Beckingham, J. D., and Archibald, J. H. (1996). Field Guide to Ecosites of Northern Alberta (Edmonton, AB: Natural Resources Canada; Canadian Forest Service; Northern Forestry Centre).
Bengtson, P., Basiliko, N., Prescott, C. E., Grayston, S. J. (2007). Spatial dependency of soil nutrient availability and microbial properties in a mixed forest of Tsuga heterophylla and Pseudotsuga menziesii in coastal British Columbia. Can. Soil Biol. Biochem. 39, 2429–2435. doi: 10.1016/j.soilbio.2007.04.010
Brandt, J. P., Flannigan, M. D., Maynard, D. G., Thompson, I. D., and Volney, W. J. A. (2013). An introduction to Canada's boreal zone: ecosystem processes, health, sustainability, and environmental issues. Environ. Rev. 21, 207–226. doi: 10.1139/er-2013-0040
Broadley, M., Brown, P., Cakmak, I., Rengel, Z., and Zhao, F. (2012). “Function of nutrients: micronutrients,” in Marschner's Mineral Nutrition of Higher Plants, 3rd Edn. Oxford, UK: Elsevier. 191–248. doi: 10.1016/B978-0-12-384905-2.00007-8
Bunt, J. S., and Rovira, A. D. (1954). Oxygen uptake and carbon dioxide evolution of heat-sterilized soil. Nature 173, 1242–1242. doi: 10.1038/1731242a0
Carcamo, H. A., Parkinson, D., and Volney, J. W. A. (1998). Effects of sulphur contamination on macroinvertebrates in Canadian pine forests. Appl. Soil Ecol. 9, 459–464. doi: 10.1016/S0929-1393(98)00105-X
Clayton, M. K., and Hudelson, B. D. (1995). Confidence intervals for autocorrelations based on cyclic samples. J. Am. Stat. Assoc. 90, 753–757. doi: 10.1080/01621459.1995.10476569
Das Gupta, S., and Mackenzie, M. D. (2016). Spatial patterns of soil respiration links above and belowground processes along a boreal aspen fire chronosequence. PLoS ONE 11:e0165602. doi: 10.1371/journal.pone.0165602
Davis, M. A., Grime, J. P., and Ken, T. (2000). Fluctuating resources in plant communities: a general theory of invasibility. J. Ecol. 88, 528–534. doi: 10.1046/j.1365-2745.2000.00473.x
de Mendiburu, F. (2014). Agricolae: Statistical Procedures for Agricultural Research. (R Package Version 1.1-8 edn).
Environment and Parks (2015). Oil Sands Mine Reclamation and Disturbance Tracking by Year. Available online at: http://osip.alberta.ca/library/Dataset/Details/27# (Accessed April 4, 2017).
Ericsson, T. (1995). Growth and shoot: root ratio of seedlings in relation to nutrient availability. Plant Soil 168, 205–214.
Facelli, E., and Facelli, J. M. (2002). Soil phosphorus heterogeneity and mycorrhizal symbiosis regulate plant intra-specific competition and size distribution. Oecologia 133, 54–61. doi: 10.1007/s00442-002-1022-5
Fraterrigo, J. M., Turner, M. G., Pearson, S. M., and Dixon, P. (2005). Effects of past land use on spatial heterogeneity of soil nutrients in Southern Appalachian forests. Ecol. Monogr. 75, 215–230. doi: 10.1890/03-0475
Gómez-Aparicio, L., Domínguez-Begines, J., Kardol, P., Ávila, J. M., Ibáñez, B., and García, L. V. (2017). Plant-soil feedbacks in declining forests: implications for species coexistence. Ecology 98, 1908–1921. doi: 10.1002/ecy.1864
Guo, D., Mou, P., Jones, R. H., and Mitchell, R. J. (2004). Spatio-temporal patterns of soil available nutrients following experimental disturbance in a pine forest. Oecologia 138, 613–621. doi: 10.1007/s00442-003-1473-3
Halpern, C. B. (1989). Early successional patterns of forest species: interactions of life history traits and disturbance. Ecology 70, 704–720. doi: 10.2307/1940221
Hanson, P. J., Edwards, N. T., Garten, C. T., and Andrews, J. A. (2000). Separating root and soil microbial contributions to soil respiration: a review of methods and observations. Biogeochemistry 48, 115–146. doi: 10.1023/A:1006244819642
Hawkesford, M., Horst, W., Kichey, T. M. R., Lambers, H., Schjørring, J. K., Møller, I. S., et al. (2012). “Chapter 6-functions of macronutrients A2 - marschner, Petra,” in Marschner's Mineral Nutrition of Higher Plants, 3rd Edn (San Diego, CA: Academic Press), 135–189.
Högberg, P., and Ekblad, A. (1996). Substrate-induced respiration measured in situ in a C3-plant ecosystem using additions of C4-sucrose. Soil Biol. Biochem. 28, 1131–1138. doi: 10.1016/0038-0717(96)00124-1
Hume, A., Chen, H. Y. H., Taylor, A. R., Kayahara, G. J., and Man, R. (2016). Soil C:N:P dynamics during secondary succession following fire in the boreal forest of central Canada. Forest Ecol. Manag. 369, 1–9. doi: 10.1016/j.foreco.2016.03.033
Isaaks, E. H., and Srivastava, R. M. (1989). Applied Geostatistics. New York, NY: Oxford University Press.
Jobbágy, E. G., and Jackson, R. B. (2001). The distribution of soil nutrients with depth: global patterns and the imprint of plants. Biogeochemistry 53, 51–77. doi: 10.1023/A:1010760720215
Johnson, C. M., Zarin, D. J., and Johnson, A. H. (2000). Post-disturbance aboveground biomass accumulation in global secondary forests. Ecology 81, 1395–1401. doi: 10.1890/0012-9658(2000)081[1395:PDABAI]2.0.CO;2
Jokela, E. J., Stone, E. L., and McFee, W. W. (1991). Micronutrient deficiency in slash pine: response and persistence of added manganese. Soil Sci. Soc. Am. J. 55, 492–496. doi: 10.2136/sssaj1991.03615995005500020033x
Kishchuk, B. E., Thiffault, E., Lorente, M., Quideau, S., Keddy, T., and Sidders, D. (2015). Decadal soil and stand response to fire. harvest. and salvage-logging disturbances in the western boreal mixedwood forest of Alberta, Canada. Can. J. Forest Res. 45, 141–152. doi: 10.1139/cjfr-2014-0148
Lavoie, M., and Mack, M. C. (2012). Spatial heterogeneity of understory vegetation and soil in an Alaskan upland boreal forest fire chronosequence. Biogeochemistry 107, 227–239. doi: 10.1007/s10533-010-9547-x
Mackenzie, D. D., and Naeth, M. A. (2010). The role of the forest soil propagule bank in assisted natural recovery after oil sands mining. Restor. Ecol. 18, 418–427. doi: 10.1111/j.1526-100X.2008.00500.x
Maynard, D. G., Paré, D., Thiffault, E., Lafleur, B., Hogg, K. E., and Kishchuk, B. (2014). How do natural disturbances and human activities affect soils and tree nutrition and growth in the Canadian boreal forest? Environ. Rev. 22, 161–178. doi: 10.1139/er-2013-0057
Natural Regions Committee (2006). Natural Regions and Subregions of Alberta. Compiled by D. J. Downing and W. W. Pettapiece. Government of Alberta. Pub. No. T/852, (Edmonton, AB: Her Majesty the Queen in Right of Alberta. as represented by the Minister of Environment).
Neary, D. G., Klopatek, C. C., DeBano, L. F., and Ffolliott, P. F. (1999). Fire effects on belowground sustainability: a review and synthesis. Forest Ecol. Manage. 122, 51–71. doi: 10.1016/S0378-1127(99)00032-8
Outeiro, L., Aspero, F., and Ubeda, X. (2008). Geostatistical methods to study spatial variability of soil cations after a prescribed fire and rainfall. Catena 74, 310–320. doi: 10.1016/j.catena.2008.03.019
Pinno, B., and Hawkes, V. (2015). Temporal trends of ecosystem development on different site types in reclaimed boreal forests. Forests 6:2109. doi: 10.3390/f6062109
Qian, P., and Schoenau, J. J. (2002). Practical applications of ion exchange resins in agricultural and environmental soil research. Can. J. Soil Sci. 82, 9–21. doi: 10.4141/S.00-091
R Core Team (2012). R: A Language and Environment for Statistical Computing. Vienna, R Foundation for Statistical Computing.
R Core Team (2016). R: A Language and Environment for Statistical Computing. (Vienna: R Foundation for Statistical Computing).
Rennenberg, H. (1984). The fate of excess sulfur in higher-plants. Ann. Rev. Plant Physiol. Plant. Mol. Biol. 35, 121–153. doi: 10.1146/annurev.pp.35.060184.001005
Reynolds, H. L., Packer, A., Bever, J. D., and Clay, K. (2003). Grassroots ecology: plant-microbe-soil interactions as drivers of plant community structure and dynamics. Ecology 84, 2281–2291. doi: 10.1890/02-0298
Rydgren, K, Hestmark, G, and Okland, R. H. (1998). Revegetation following experimental disturbance in a boreal old-growth Picea abies forest. J. Veg. Sci. 9, 763–776. doi: 10.2307/3237042
Schlesinger, W. H., and Andrews, J. A. (2000). Soil respiration and the global carbon cycle. Biogeochemistry 48, 7–20. doi: 10.1023/A:1006247623877
Seedre, M., Taylor, A. R., Brassard, B. W., Chen, H. Y. H., and Jõgiste, K. (2014). Recovery of ecosystem carbon stocks in young boreal forests: a comparison of harvesting and wildfire disturbance. Ecosystems 17, 851–863. doi: 10.1007/s10021-014-9763-7
Silvester, W. B. (1989). Molybdenum limitation of asymbiotic nitrogen fixation in forests of Pacific Northwest America. Soil Biol. Biochem. 21, 283–289. doi: 10.1016/0038-0717(89)90106-5
Smithwick, E. A. H., Mack, M. C., Turner, M. G., Chapin, F. S. III, Zhu, J., and Balser, T. C. (2005). Spatial heterogeneity and soil nitrogen dynamics in a burned black spruce forest stand: distinct controls at different scales. Biogeochemistry 76, 517–537. doi: 10.1007/s10533-005-0031-y
Sorenson, P. T., MacKenzie, M. D., Quideau, S. A., and Landhäusser, S. M. (2017). Can spatial patterns be used to investigate aboveground-belowground links in reclaimed forests? Ecol. Eng. 104, 57–66. doi: 10.1016/j.ecoleng.2017.04.002
Sponseller, R. A., Gundale, M. J., Futter, M., Ring, E., Nordin, A., Näsholm, T., et al. (2016). Nitrogen dynamics in managed boreal forests: Recent advances and future research directions. Ambio 45, 175–187. doi: 10.1007/s13280-015-0755-4
Stone, E. L. (1990). Boron deficiency and excess in forest trees: a review. Forest Ecol. Manage. 37, 49–75. doi: 10.1016/0378-1127(90)90046-E
Tamir, G., Shenker, M., Heller, H., Bloom, P. R., and Bar-Tal, P. (2011). Can soil carbonate dissolution lead to overestimation of soil respiration? Soil Sci. Soc. Am. J. 75, 1414–1422. doi: 10.2136/sssaj2010.0396
Vitousek, P. M., and Reiners, W. A. (1975). Ecosystem succession and nutrient retention: a hypothesis. BioScience 25, 376–381. doi: 10.2307/1297148
Wardle, D. A. (2002). Communities and Ecosystems: Linking the Aboveground and Belowground Components (Princeton, NJ: Princeton University Press).
Wieder, W. R., Cleveland, C. C., Smith, W. K., and Todd-Brown, K. (2015). Future productivity and carbon storage limited by terrestrial nutrient availability. Nat. Geosci. 8, 441–444. doi: 10.1038/ngeo2413
Keywords: spatial heterogeneity, boreal, land reclamation, nutrient bioavailability, soil respiration
Citation: Dietrich ST and MacKenzie MD (2018) Comparing Spatial Heterogeneity of Bioavailable Nutrients and Soil Respiration in Boreal Sites Recovering From Natural and Anthropogenic Disturbance. Front. Environ. Sci. 6:126. doi: 10.3389/fenvs.2018.00126
Received: 16 April 2018; Accepted: 10 October 2018;
Published: 01 November 2018.
Edited by:
Maria Luz Cayuela, Centro de Edafología y Biología Aplicada del Segura (CEBAS), SpainReviewed by:
Olga Vindušková, Stanford University, United StatesCarsten W. Mueller, Technische Universität München, Germany
Copyright © 2018 Dietrich and MacKenzie. This is an open-access article distributed under the terms of the Creative Commons Attribution License (CC BY). The use, distribution or reproduction in other forums is permitted, provided the original author(s) and the copyright owner(s) are credited and that the original publication in this journal is cited, in accordance with accepted academic practice. No use, distribution or reproduction is permitted which does not comply with these terms.
*Correspondence: Sebastian T. Dietrich, c2RpZXRyaWNAdWFsYmVydGEuY2E=