- 1William H. Miner Agricultural Research Institute, Chazy, NY, United States
- 2Department of Plant and Soil Science, University of Vermont, Burlington, VT, United States
Riparian zones can release bioavailable phosphorus and contribute to water quality degradation. Here, we measured phosphorus (P) release to soil porewater (PW) and overlying floodwater (FW) in 12 riparian buffer and 2 agricultural floodplain soils from northwestern Vermont, USA. The objective was to measure P desorption potential and mobilization to floodwater under moderately reducing conditions designed to mimic saturated riparian environments. Duplicate samples (field-moist) were flooded with distilled water in polyethylene beakers modified for PW sampling. Soluble reactive P (SRP) (PW and FW) and PW ferrous iron (Fe2+) were measured over a 75-day period in the laboratory. Soluble unreactive P (SUP) in PW was also measured twice. Samples from two sites were also flooded after air-drying to assess the effect of drying soil on SRP release. Results indicated PW-SRP tended to increase over time, whereas FW-SRP tended to decrease. The ratio of PW-SRP on day-75 to initial concentrations ranged from 0.21 to 8.4 (mean = 3.2 ± 2.7), while the ratio for FW-SRP was 0.19 to 1.3 (mean = 0.63 ± 0.39). Mean PW- and FW-SRP ranged from 0.03 to 2.2 mg/L and 0.01 to 0.33 mg/L, respectively. Reduction occurred in 13/14 soils as indicated by PW-Fe2+, while FW remained oxidized. Mean PW- (R2 = 0.48, P = 0.006) and FW-SRP (R2 = 0.47, P = 0.007) increased with pH, whereas PW-SUP increased at lower pH (R2 = 0.44, P = 0.01). Mean ratio of PW-SRP:FW-SRP was 3.5 ± 1.9 and increased with soil pH (R2 = 0.59, P = 0.001). Modified Morgan extractable P was a good predictor of both mean FW- (R2 = 0.75, P < 0.0001) and PW-SRP (R2 = 0.77, P < 0.0001) and release over time. Flooding air-dried soil decreased FW dissolved oxygen concentrations and increased PW-Fe2+, PW-SRP, and SRP mobilization to FW relative to a field-moist state. Results indicate that while PW-SRP release was substantial, mobilization to overlying FW was limited by resorption of released P. Our results highlight the importance of integrating labile soil P measures with hydrologic flow pathways in models to better predict P transport in riparian landscapes.
Introduction
Phosphorus (P) is a critical nutrient for crop production and is also the limiting nutrient for freshwater eutrophication (Carpenter et al., 1998). Unlike nitrate-nitrogen, where leaching and atmospheric losses are substantial, P readily accumulates in soils above natural background levels (i.e., legacy P) where it can be transported in runoff water and contribute to water quality degradation (Jarvie et al., 2013; Sharpley et al., 2013). In mixed land use watersheds, both agricultural and urban activities contribute P to streams.
Lake Champlain is a 1,331 km2 freshwater lake providing drinking water to >100,000 residents in New York, Vermont, and Canada with a history of nuisance algae blooms since the 1970's. Nonpoint sources are estimated to contribute from 42 to >90% of the P to Lake Champlain (Smeltzer et al., 2009). Agriculture is assumed to contribute 38% of the total P load, however urban (16%), forest (21%), and stream bank erosion (18%) are also large sources (Lake Champlain Basin Program, 2015). In addition to farm practices, implementing riparian buffers is considered an important practice to reduce P loss from uplands to streams and is mandatory for many farms in Vermont under new state regulations. While riparian buffers can remove P from runoff, they may also have little impact on P transport and in some cases contribute to P loading through riparian stream bank erosion and/or dissolved P losses in surface or subsurface runoff. Phosphorus loading from stream bank erosion is considered an important but poorly understood P source in the Lake Champlain Basin and other mixed land use watersheds (Sekely et al., 2002; Zaimes et al., 2008; Kline and Cahoon, 2010; Kronvang et al., 2012; Langendoen et al., 2012; Ishee et al., 2015).
Riparian buffers have long been considered a conservation best management practice due to their ability to remove nitrate-N, sediment, and P in runoff (Cooper and Gilliam, 1987; Muscutt et al., 1993; Hill, 1996; Dorioz et al., 2006; Hoffmann et al., 2009), however efficacy varies widely depending on hydrogeologic setting, runoff flow pathways, soil type, labile soil P concentrations, buffer width, vegetation, redox conditions, and management of adjacent cropland (Novak et al., 2004; Song et al., 2007; Surridge et al., 2007; Loeb et al., 2008; Fuchs et al., 2009; Hoffmann et al., 2009). In general, buffers are more effective at particulate P removal in surface runoff compared to dissolved P. Numerous studies have noted buffers can be a source of P, particularly with respect to shallow subsurface groundwater flow. Roberts et al. (2012) reviewed P dynamics in vegetated buffer strips and concluded dissolved P loss risk was elevated where labile P is subject to mobilization during infiltration excess overland flow events. Soil and landscape variability (e.g., drainage, texture, slope/water table dynamics) affect runoff flow paths, redox potential, P chemistry and ultimately riparian P fluxes in runoff and shallow subsurface flows (Dorioz et al., 2006; Scalenghe et al., 2007; Young and Briggs, 2008; Hoffmann et al., 2009; Vidon et al., 2010).
Hydrology is intimately tied to riparian P behavior since it controls the physical transport/deposition of particulate P while simultaneously imposing biogeochemical constraints on labile P reactions. Extended water saturation can elicit reduction of solid phase ferric phosphates (Fe3+) leading to desorption of molybdate reactive P (i.e., orthophosphate) to solution and potentially loss in runoff waters (Sims and Pierzynski, 2005; Hoffmann et al., 2009; Henderson et al., 2012; Parsons et al., 2017). Significant P release from both agricultural and wetland soils can occur when soil becomes reduced (Moore and Reddy, 1994; Moore et al., 1998; Young and Ross, 2001; Scalenghe et al., 2002; Loeb et al., 2008; Henderson et al., 2012; Amarawanshaa et al., 2015). Surridge et al. (2007) studied P release in UK wetland soils using peat cores and measured pore water molybdate reactive P concentrations as high as 3 mg L−1 within a month of flooding. They found a strong relationship between molar concentrations of ferrous Fe (Fe2+) and porewater molybdate reactive P, suggesting Fe was likely controlling P solubility. Vidon et al. (2010) hypothesized that the balance of reducing and oxidizing conditions is a primary driver of when riparian zones act as “hot spots” of soluble reactive P release. The authors suggest P desorption risk is elevated where higher sediment-bound P concentrations experience lower redox conditions, leading to P release from Fe-P forms. Roberts et al. (2012) suggested that rather than being a final sink for P, vegetated buffers act as a “modifying loop in the P transfer continuum” and that more research on P re-mobilization mechanisms is warranted.
Improved understanding of biogeochemical mechanisms affecting P transport in riparian zones will enhance prediction of P removal effectiveness and ultimately water quality modeling efforts. Riparian soils experience variable saturation and redox potential. Measuring P release over a range of redox potentials is important for developing practical soil P loss indices. In the Lake Champlain Basin and other watersheds globally, riparian zones are considered important for mitigating P transport to streams. An important knowledge gap is a detailed understanding of mechanisms controlling desorption and mobility of labile inorganic P (i.e., orthophosphate-P) to surface and subsurface runoff flows (Hoffmann et al., 2009). Few studies focusing on riparian soil P have simultaneously measured P release in moderately reduced porewater and mobilization to overlying water (typical of flooded riparian systems). Here, our objectives were to: (1) measure soluble reactive P (SRP) release to porewater under moderately low redox potentials (designed to mimic saturated riparian zones) and subsequent mobilization to floodwater, and (2) determine the efficacy of routinely measured soil properties (modified Morgan P/labile P, total soil P, water-extractable SRP, pH, organic matter content) to predict P release to porewater and floodwater.
Materials and Methods
Site Description and Soil Sampling
The riparian sites sampled for this study are located in northwest Vermont, USA and part of a related study on riparian P availability (Young et al., 2012, 2013). Twelve surface soil samples (sites 1–12) were collected from riparian buffers along two small streams, Lewis Creek and Rugg Brook, both of which drain to Lake Champlain. Two samples were also taken from adjoining agricultural fields managed as corn silage (Lewis Creek site). Buffers at both locations were mainly comprised of grasses and were previously managed as long-term pasture. Based on information from landowners, there was no recent P fertilizer or manure applied. Surrounding land was forested or managed in pasture/hay or corn. Parent materials are a mix of alluvial, glaciolacustrine, and glacial outwash (Griggs, 1971; Flynn and Joslin, 1979). At each buffer location, 4 soil samples were randomly taken from a 1 m2 area using a bucket auger (15 cm length, 5 cm inside diameter) and composited in the field. For additional details on site and buffer characteristics, refer to Young et al. (2012) and Young et al. (2013). All samples were returned to the laboratory on the day of collection, placed in polyethylene bags and stored at 5°C until initiating the flooding experiment. A subsample of soil from each location was taken for chemical analysis. Air-dried subsamples were put through a 2 mm sieve then pulverized (< 2 mm) before chemical extraction.
Soil Chemical Analyses
The modified Morgan soil test extractant (a modification of the original Morgan procedure; Morgan, 1941) was used to extract labile P and select cations (Al, Ca, Fe, K, Mg, Mn). Phosphorus extracted by ammonium acetate (NH4OAc) is an indicator of plant availability and the basis for P recommendations in much of Vermont and New England (McIntosh, 1969; Jokela et al., 1998); it is also used in Scotland for agronomic and environmental P assessment (Lumsdon et al., 2016). Here, duplicate 4-g samples were extracted for 15 min with 1.25 mol L−1 NH4OAc buffered at pH 4.8 using a 1:5 (v/v) soil/solution ratio. Extracts were immediately filtered (Whatman no. 2 filter paper) followed by determination of molybdate reactive P using the molybdate-stannous chloride method (Jackson, 1958). Phosphorus measured by this method is considered mainly orthophosphate and was abbreviated as “MMP-Color” (i.e., modified Morgan extractable P, measured by molybdate colorimetry). Phosphorus in the same extract was also measured by inductively coupled plasma–optical emission spectroscopy (ICP) (Optima 2000, PerkinElmer Corp., Norwalk, CT). Phosphorus measured by ICP represents total P in solution (orthophosphate + organic P) and was abbreviated as “MMP-ICP” (Young et al., 2017). Total soil P was determined by ICP following digestion in sulfuric acid-hydrogen peroxide-hydrochloric acid (O'Halloran and Cade-Menun, 2008). Soil organic matter was determined by loss-on-ignition and converted to organic matter equivalents based on a regression between organic matter measured by chemical oxidation and loss on ignition (Hoskins, 1997). Soil pH was measured in distilled water using a 2:1 soil to solution ratio with a Beckman Futura rugged bulb pH electrode and a Fisher Accumet calomel reference electrode. Water-extractable molybdate reactive P was determined following the procedure of Turner et al. (2002).
Soil Microcosms
Microcosms were used to simulate flooded conditions and constructed of 1-liter polyethylene beakers modified for pore water sampling (Young and Ross, 2001). Holes were drilled (1.75 cm diam.) at approximately 5 cm above the bottom and plastic check valves were installed and sealed with silicone to prevent leakage. Each check valve was covered with 4-μm nylon mesh to filter particulates. Beakers and check valves were washed in a 10% HCl solution and triple rinsed with distilled water prior to the experiment. For each soil sample, approximately 500 mL of field moist soil was placed in duplicate microcosms. Individual microcosms were gently flooded with distilled water by applying water to the sides of beakers to minimize soil disturbance using a 1-L squeeze bottle. Enough water was applied to bring the volume up to 1-L, resulting in a water column depth of approximately 7 cm. Porewater (PW) was sampled by allowing water to drain via gravity into 50 mL glass beakers. Approximately 5 mL of sample was allowed to drain and discarded prior to taking a sample for P and Fe analysis. Check valve ends were wrapped with Parafilm to prevent drainage in between samplings. Floodwater (FW) was sampled by carefully inserting a 10-mL pipette midway between the soil surface and the top of the water column. The first set of PW and FW samples was collected 2 days after initial flooding (flooding was initiated on 12/18/07). Thereafter, FW/PW was sampled approximately weekly except for the last two samplings which were taken at 2 and 3 week intervals. Dissolved oxygen (DO) concentration of floodwater was also measured periodically by inserting a probe (Hach Company, Loveland, CO) midway between the soil surface and top of the water column. Evaporative and sample water losses were replaced by carefully adding distilled water after each sampling and when necessary in between samplings to bring the total volume back to 1 L (soil + distilled water). All beakers were left uncovered during the experiment to allow oxygen diffusion into floodwater to mimic field conditions. The experiment was conducted in a laboratory with exposure to fluorescent light only during the time microcosm measurements were made. The average temperature of the laboratory was approximately 20°C during the study. Since drying soils can alter microbial and P dynamics upon rewetting (Turner and Haygarth, 2001; Song et al., 2007), two of the soils (2 and 5) were also flooded in duplicate after air-drying to evaluate the effect of soil drying on SRP and ferrous iron (Fe2+) release.
Aqueous Phosphorus and Iron Measurements
All samples were filtered through 0.45-μm membrane filters prior to SRP and Fe2+ analysis. Soluble reactive P (SRP) was measured colorimetrically by the chlorostannous-reduced molybdophosphoric blue method (Jackson, 1958). SRP is mainly considered orthophosphate, but can include small amounts of complex inorganic P. The spectrophotometer was zeroed with water and a sample blank (without reagents) was used to provide matrix correction. On day-36 and day-49 of the flooding experiment, PW soluble unreactive P (SUP) was also determined. Samples were digested using the persulfate oxidation method (American Public Health Association, 1989) and total dissolved P was measured on a spectrophotometer as described above. Differences between total dissolved P and SRP were used to estimate of soluble unreactive P (SUP). Ferrous iron (Fe2+) was measured in PW as an indicator of soil redox status using the 2,2 dipyridyl colorimetric method after Muss and Mellen (1942). For samples that contained high dissolved organic material, absorbance was measured prior to adding color reagents to provide matrix correction.
Calculations and Statistics
Descriptive statistics (mean and standard deviations) were calculated using Microsoft Excel. SRP and Fe2+ concentrations were expressed as the mean of duplicate microcosm measurements and plotted as function of the number of days after flooding. Linear regression was used to quantify relationships among FW-SRP, PW-SRP, and select soil properties (total soil P, Modified Morgan extractable P, soil pH, water-extractable P, soil organic matter content) to determine predictive relationships. FW- and PW-SRP were regressed against the above soil properties for each sampling time point and for means calculated across time (n = 8) to provide a more generalized index of P release across the soils. Regression analyses were performed using the Proc Reg procedure of SAS version 8e (SAS Institute, 1999). Some variables were logarithmically (base 10) transformed prior to performing regression to meet normality assumptions. A student's t-test was used to compare PW-SRP, FW-SRP, PW-Fe2+, and FW dissolved oxygen concentrations between field-moist vs. dry soil (sites 2 and 5 were averaged) assuming equal variance. A P-value ≤ 0.05 was used to declare statistical significance.
Results
Soil Properties
Soil drainage class ranged from poorly to excessively well drained and represented a range of texture and parent material (Table 1). Soil pH, organic matter, and total P ranged from 5.4 to 7.3, 24 to 92 g kg−1, and 362 to 1615 mg kg−1, respectively (Table 1). Concentrations of MMP-Color were relatively low for buffer sites, ranging from 1.3 to 4.2 mg kg−1 (MMP-ICP ranged from 1.9 to 8.1 mg kg−1). Not including sites 13 and 14, average MMP-Color and MMP-ICP were 2.2 ± 1.1 mg kg−1 and 4.4 ± 1.5 mg kg−1, respectively. These are considered in the “medium” (2.1 to 4.0 mg kg−1) and optimum (4.1 to 7.0 mg kg−1) categories for agronomic P availability, respectively (Jokela et al., 2004). On average, MMP-ICP concentrations were 2.1-fold greater than MMP-Color values (Table 1). Sites 13 and 14 were corn silage production fields and had the highest MMP-Color, MMP-ICP, and water extractable SRP concentrations. These two samples were both in the “high” category (7.1 to 20 mg kg−1) based on MMP-Color and no further P inputs would be recommended (Jokela et al., 2004).
Microcosm SRP and Ferrous Iron (Fe2+) Release
In general, PW-SRP increased with time of flooding, whereas FW-SRP tended to decrease (Figure 1). Concentrations of PW- and FW-SRP remained low for site 1 with no measurable ferrous Fe (Fe2+) indicating limited soil reduction. All other sites experienced reduction as indicated by increasing PW-Fe2+ over time. Most of the sites showed a substantial increase in PW-SRP and PW-Fe2+ after 3 to 4 weeks of flooding (Figures 1A–N). PW-SRP and Fe2+ increased rapidly for site 2 after day 23. PW Fe2+ increased 10-fold for site 4 from day-23 to day-75, while PW- and FW-SRP remained low (Figures 1N,O). PW-SRP for site 5 increased strongly with time, while FW-SRP tended to decrease. For sites 6, 8, and 9, both PW-SRP and PW-Fe2+ increased on day-36 (Figure 1). In contrast to most other sites, PW-SRP decreased consistently over time for site 7. For sites 10, 11, and 12, PW-SRP either increased with time or stayed relatively consistent compared to initial concentrations, while FW-SRP decreased (Figure 1). In the two agricultural soils, PW-SRP concentrations were much higher and increased 3.7-fold by day-75 for soil 13 and 6.3-fold by day-36 for soil 14. While PW-SRP increased strongly over the flood duration, FW-SRP decreased over time (Figure 1). PW- and FW-SRP in these soils stayed well above reported eutrophication threshold (0.03 mg SRP L−1), with PW-SRP >1 mg SRP L−1 on day-75 for site 13 and nearly 3 mg SRP L−1 for site 14 (Figure 1). The large increase in PW-SRP for the two agricultural sites was also accompanied by a sharp increase in PW-Fe2+. In general, concentrations of PW- and FW-SRP (expressed as a logarithm) were strongly related (Supplementary Table 1). Average soluble unreactive P concentrations (SUP) ranged from 0.035 to 0.30 mg/L (mean = 0.13 ± 0.13) and tended to be higher in lower pH soils.
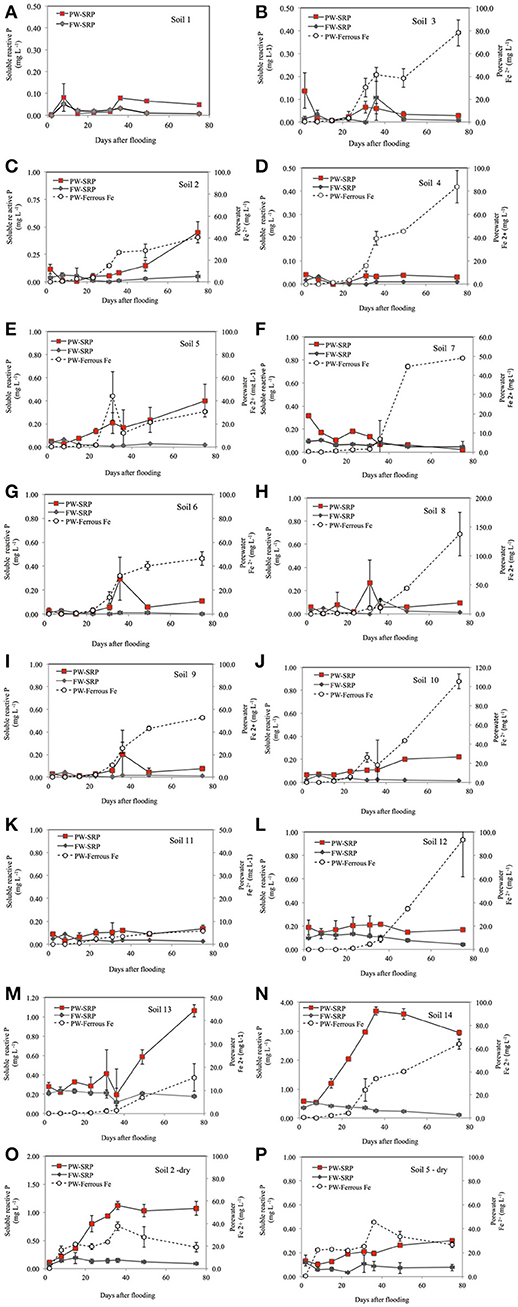
Figure 1. Porewater (PW-SRP) soluble reactive phosphorus, floodwater soluble reactive phosphorus (FW-SRP), and porewater ferrous Fe concentrations (PW-Fe2+) over the 75-day flooding period for soils 1–14 (A–N) and sites 2 and 5 (O,P) after air-drying soil prior to flooding. Error bars represent the standard deviation for the mean of duplicate microcosms.
Relationships Between Phosphorus Release and Soil Properties
At each sampling time, PW- and FW-SRP concentrations were strongly related with MMP-Color, MMP-ICP, and water-extractable SRP concentrations (WEP) (Supplementary Tables 2, 3). Mean PW- and FW-SRP concentrations also increased significantly with soil pH and TP, however relationships were weaker compared to labile soil P measures (Figure 2). To provide a more generalized index of potential SRP release over the experiment, mean PW- and FW-SRP concentrations (expressed as a logarithm) were regressed against TP, MMP-Color, MMP-ICP, and WEP. While all of these soil P measures were significantly related to PW- and FW-SRP, MMP-Color was the best predictor (Figures 2A–G). Mean PW- and FW-SRP also increased with soil pH (Figure 2E), while the ratio of PW-SRP to FW-SRP (averaged over the experiment) also increased significantly with pH (Figure 2F). In contrast, SUP concentration (averaged over for the two dates sampled) increased with decreasing pH (Figure 2G). With the exception of soil 1 where PW-Fe2+ was never within detection limits, PW-Fe2+ increased over time indicating progressively lower redox potential. While PW-Fe2+ increased simultaneously with PW-SRP in several soils, there were no significant correlations between PW-Fe2+ and PW-SRP. There were also no significant correlations between soil organic matter content and PW- or FW-SRP.
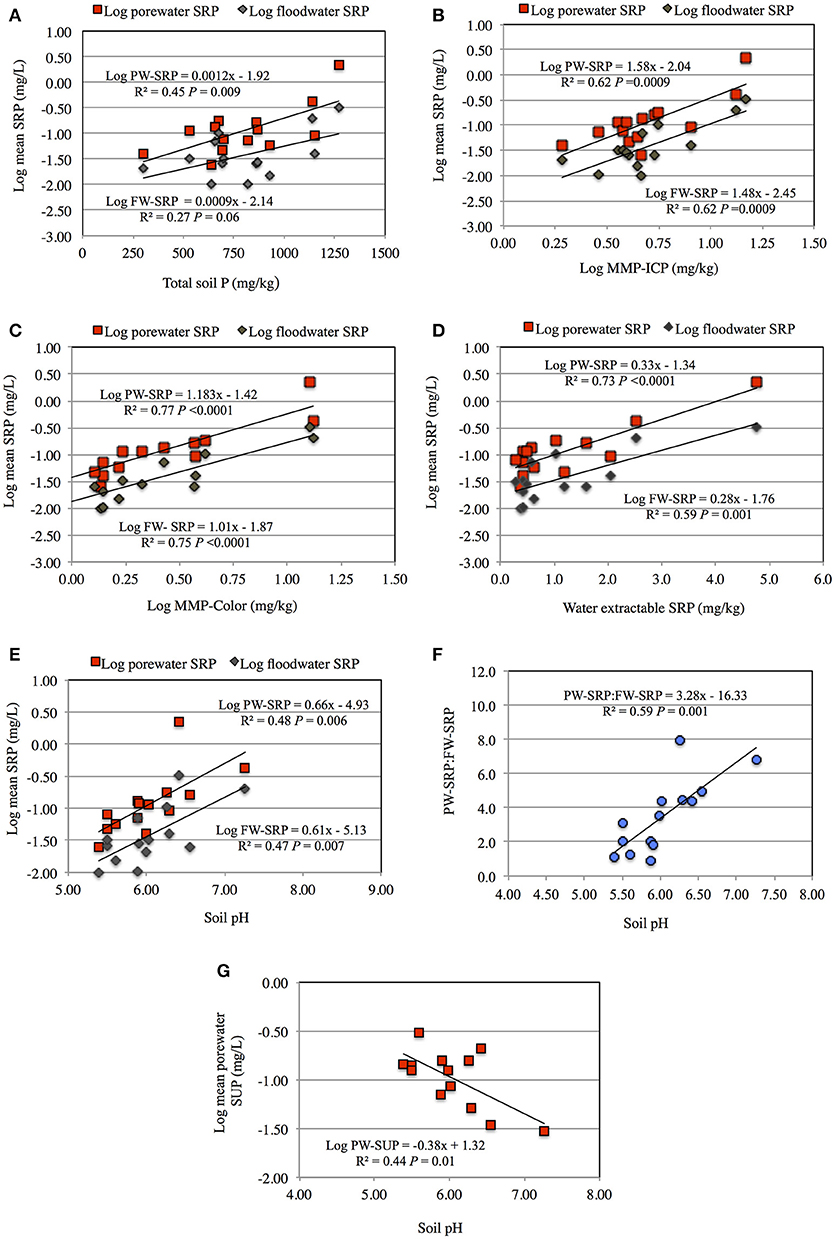
Figure 2. Porewater soluble reactive phosphorus (PW-SRP) and floodwater soluble reactive phosphorus (FW-SRP) averaged over the study period (n = 8) as a function of total soil phosphorus (A), modified Morgan extractable soil P measured by ICP (MMP-ICP) (B), modified Morgan extractable P measured by the molybdate colorimetric procedure (MMP-Color) (C), water extractable soluble reactive phosphorus (D) and soil pH (E). Mean ratio of PW-SRP to FW-SRP over the study period as a function of soil pH (F) and average PW-soluble unreactive P (SUP) as a function of soil pH (G).
Influence of Drying Soils Prior to Flooding
When soil 2 was dried and then flooded, PW-SRP, PW-Fe2+, and FW-SRP increased more rapidly and to a larger extent compared to flooding in a field moist state (Figures 1, 3). When soil 5 was flooded after drying, initial PW- and FW-SRP were higher compared to flooding field-moist and PW-Fe2+ was measureable within the first week. Compared to flooding field-moist, drying increased mean PW-Fe2+ over the experiment by 1.4- to 1.8-fold, PW-SRP by 1.2- to 6.1-fold, FW-SRP by 3- to 4-fold, while decreasing FW-DO by 32 to 41%. When results were averaged for sites 2 and 5, flooding dry soil vs. field-moist increased PW-Fe2+ by 60% (P = 0.05), indicating greater Fe solubility and lower redox. The significant drop in FW-DO of the dried soils (P = 0.01) also supports the notion that drying intensified soil reduction (Figure 3). While not statistically significant, PW- and FW-SRP trended higher by an average of 3.2- (P = 0.36) and 3.6-fold (P = 0.10), respectively, suggesting greater soil P desorption and mobilization to FW after soils were dried and then flooded.
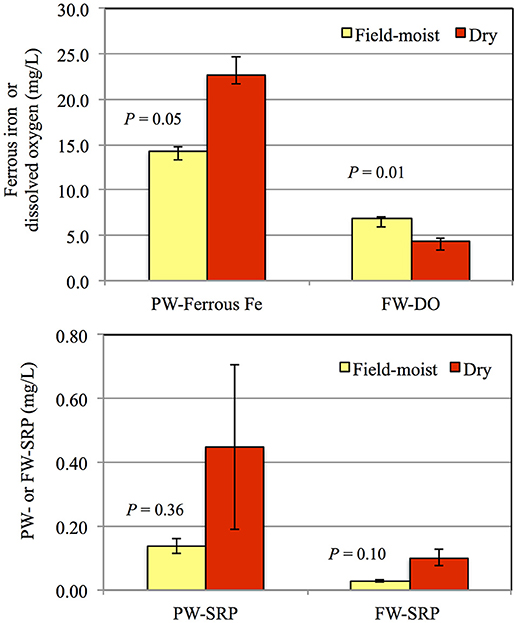
Figure 3. Average porewater ferrous Fe (PW-Fe2+), floodwater dissolved oxygen (FW-DO), porewater soluble reactive phosphorus (PW-SRP), and floodwater soluble reactive phosphorus concentrations (FW-SRP) for field-moist soil versus flooding after drying. Results were averaged for sites 2 and 5. Error bars represent the standard error of the mean (SD/20.5) and P-values were calculated based on a student's t-test.
Discussion
Soil Properties and Phosphorus Concentrations
Based on MMP-Color values, 11/12 riparian samples were categorized as “low” or “medium” with respect to UVM field crop P guidelines, whereas ICP measurement resulted in 10/12 samples classified as “medium” and “optimum.” Phosphorus measured by ICP measures all P in solution as compared to colorimetric determination, which mainly reflects orthophosphate. The consistently higher P measured by ICP may reflect unreactive/organic P extracted during the modified Morgan procedure (Young et al., 2013, 2017; Young and Ross, 2016). The agricultural sites (soils 13 and 14) had high TP and the highest concentrations of labile P (MMP-Color, MMP-ICP, and water extractable SRP). While both of the agricultural soils had high labile P concentrations, similar to riparian samples, they also had relatively high water-extractable unreactive P, some of which may be bioavailable (Young et al., 2013). From an agronomic standpoint, no further P inputs would be recommended for optimum crop production potential for these two fields. In general, the more poorly drained riparian sites with finer-textured soils contained greater TP and MMP, suggesting higher native P availability and/or P sorption potential was higher in these soils compared to better drained, coarser-textured soils (Young et al., 2012, 2013).
Influence of Redox and pH on Phosphorus Release
Results showed a tendency for continued orthophosphate desorption to PW over the flood duration. For 13/14 microcosms, PW-Fe2+ increased substantially over time, indicating declining soil redox as microbes reduced Fe3+ compounds to Fe2+ (Vadas and Sims, 1998). Assuming Fe3+ was reduced to Fe2+ at +150 to +250 mV (Ponnamperuma, 1972), PW redox in the later stages of flooding was likely below this range. Whereas PW redox declined sharply with time, FW remained oxidized (mean DO = 6.8 to 7.2 mg L−1). Relative to PW-SRP, FW-SRP concentrations varied less with time and tended to decrease. Vadas and Sims (1999) showed a decrease in P sorption capacity in flooded surface soils from Inland Bays, Delaware, USA and greater bioavailable P desorption (estimated by equilibrium P concentrations at zero sorption via isotherms) when soils were more reduced. In a wide range of European agricultural soils high in P, Scalenghe et al. (2002) reported initial Eh values after flooding of 305 to 515 mV, which dropped to −157 to −195 mV after 32 d of flooding. Whereas they found maximum P release rates at 1 to 3 weeks after flooding, most soils in our trial had large PW-SRP increases after 3 weeks of flooding. The simultaneous release of PW-SRP and Fe2+ in several soils is likely due to reductive dissolution of Fe-P compounds (Moore and Reddy, 1994; Moore et al., 1998; Fisher and Reddy, 2001; Hoffmann et al., 2009). In grassland soils receiving long-term biannual poultry litter applications in the UK, Henderson et al. (2012) showed that Fe3+ acted as “cement” for nanoparticulate P (1–415 nm particle diameter) by binding colloidal-bound P under oxidizing conditions and releasing colloidal and nanoparticulate P upon reduction of Fe3+ to Fe2+. Surridge et al. (2007) reported PW dissolved P of up to 3 mg L−1 under reducing conditions (0.8 mg P L−1 in overlying water) in UK wetland mesocosms and a strong relationship (R2 = 0.95) between PW-Fe2+ and PW-SRP. The fact that FW in our study remained oxidized probably limited PW-SRP mobility to FW. For example, Moore and Reddy (1994) showed oxidized floodwater (Eh >300 mV) had lower P fluxes to overlying water, (2.8 and 0.04 mg P m−2 d−1 for reduced and oxidized floodwater, respectively), thus redox potential of both PW and FW are important for predicting P transport in flooded riparian soils/sediments. We hypothesize that reductive dissolution of Fe-phosphates was an important mechanism for P release in our study, while re-oxidation of Fe2+ to Fe3+ at aerobic microsites likely limited P mobilization to FW by resorbing released P (Parsons et al., 2017). Soils in our study ranged from acidic to neutral, therefore aluminum, calcium, manganese, and/or magnesium phosphates may have also contributed to SRP desorption/sorption dynamics (Amarawanshaa et al., 2015).
We found a strong relationship between soil pH and PW- and FW-SRP concentrations. In addition, mean PW-SRP/FW-SRP increased strongly with pH, indicating lower SRP mobility at lower pH. Labile P is often strongly related to soil pH, with maximum availability occurring at a pH close to 7.0 (Pierzynski et al., 2005; Sims and Pierzynski, 2005). In Vermont agricultural soils, reactive aluminum generally increases at lower pH and readily binds phosphate (Bartlett, 1982; Jokela et al., 1998; Magdoff et al., 1999). Our findings support this in that PW- and FW-SRP decreased at lower pH. In contrast, PW-SUP concentrations increased significantly with decreasing pH. Young and Ross (2016) also reported higher labile unreactive P concentrations (defined as the numeric difference between MMP-ICP and MMP-Color) at lower pH in a large set of riparian samples from northwestern Vermont. Greater organic P concentrations at lower pH could be related to lower organic carbon oxidation rates and/or slower hydrolysis of organic P, resulting in higher organic P concentrations (Dalai, 1977; Harrison, 1987). Soluble organic P is also considered less strongly sorbed than SRP, and at lower pH, the relative proportion of organic P may increase (Anderson and Magdoff, 2005; Condron et al., 2005; Turner, 2005). Our results here show that pH significantly influenced inorganic and organic P desorption potential. Labile organic P is differentially bioavailable and can contribute to water quality degradation once hydrolyzed to orthophosphate (Turner et al., 2003; Quiquampoix and Mousain, 2005). Since our focus was on SRP, limited unreactive P measurements were made, however, our results showed organic P and orthophosphate desorption during flooding, highlighting the need to better account for both forms in modeling P release from riparian buffers. While we hypothesize labile inorganic P forms associated with mineral surfaces (Al, Ca, Fe) largely contributed to SRP release, microbial hydrolysis of organic P to SRP was not measured. Release of microbial biomass P during 6 months of flooding was hypothesized to be the main P source in riparian forest mesocosms from Georgia, USA (Wright et al., 2001). We cannot exclude the possibility that some SRP released to porewater was from microbial hydrolysis of organic P.
Predicting Phosphorus Release to Porewater and Mobilization to Floodwater
Labile extractable soil P forms were strongly related to PW- and FW-SRP release, however TP was not a good predictor of SRP release. The poor relationship between TP and SRP is likely due to the fact that TP measures all soil P including labile P. For example, TP concentration for site 11 was relatively high (1,615 mg kg−1) but with low MMP-Color concentration (3.8 mg kg−1) and low SRP release potential. MMP-Color was the best predictor of PW- and FW-SRP concentrations. MMP-Color mainly reflects labile inorganic P and is used to determine P fertility recommendations; it is also an important input for P loss risk tools (e.g., Vermont P site index). Magdoff et al. (1999) showed that MMP-Color was a good predictor of both plant availability and P desorption risk as measured by common labile soil P tests (i.e., iron oxide strip-P, calcium chloride extractable P, equilibrium P at zero sorption). They suggested MMP-Color was an index reflecting the extent of previously sorbed P. In agricultural soils from northern, NY, USA, MMP-Color was a good predictor of mean PW-SRP release in flooded microcosms (Young and Ross, 2001). Different labile soil P indices have been used to predict SRP release potential in wetland and riparian soils. For example, the ratio of Fe-bound P to ammonium oxalate extractable Fe was found to be a good predictor of porewater reactive P release in floodplain soil cores from Poland (Loeb et al., 2008). In agricultural soils, it is well established that P desorption potential increases with labile soil P concentrations, including widely used agronomic soil P tests (Sims et al., 1998; Maguire et al., 2005; Vadas et al., 2005) used in P transport models (Nelson and Parsons, 2006). While our study only included 14 soils, results indicate MMP-Color was an effective measure of SRP desorption potential to PW and subsequent mobilization to FW.
Manure and/or fertilizer history strongly affects labile P concentrations in agricultural soils. The two agricultural soils in our study had a greater propensity for SRP release, with average PW- and FW-SRP ranging from 0.42 to 2.2 mg L−1 and 0.20 to 0.33 mg L−1, respectively. When these two sites were not included in the predictive relationships between labile soil P and SRP release, overall trends were generally similar, however regression statistics (slopes/y-intercepts, significance level) changed (data not shown). It is common to include a range of soil P levels in P release studies to aid interpretation and modeling of P behavior (e.g., sorption isotherms). In addition, labile soil P can be spatially variable in cropland and buffers (Lyons et al., 1998; Young and Briggs, 2008; Young et al., 2012, 2013, 2017; Young and Ross, 2016), which some P loss models can account for. Given these reasons, we felt it was practically relevant to include the cropland sites in the predictive relationships for SRP release.
Effect of Soil Drying on Phosphorus Release
Flooding soils after drying substantially hastened soil reduction, while increasing PW-SRP release and mobilization to floodwater. On average, drying more than doubled mean PW- and FW-SRP concentrations compared to flooding field-moist. Results indicate that drying soils prior to P solubility experiments has a risk of over-estimating P release potential and that changes in field soil moisture could affect P desorption potential. Dieter et al. (2015) found that drying lake sediments prior to re-flooding increased the relative fractions of labile and reductant-soluble P forms, increased mineralization of organic P, and decreased P sorption capacity. In pasture soils from the UK, P extractability increased dramatically following drying and rewetting (Turner and Haygarth, 2001, 2003; Turner et al., 2002). Erich and Hoskins (2011) found that soil drying increased inorganic and organic P extractability in agricultural soils from Maine. Greater P solubility after drying may be attributed to greater surface acidity, enhanced organic matter solubility, resulting in enhanced microbial activity upon rewetting compared to a field-moist state (Barlett and James, 1980). In our study, drying appeared to increase microbial activity as evidenced by faster appearance of Fe2+ and lower FW-DO, which was probably due to enhanced organic matter solubility. We hypothesize that drying caused more rapid oxygen consumption upon flooding and therefore lower reoxidation of Fe2+ to Fe3+, facilitating more SRP diffusion to floodwater compared to higher redox potential under flooding field-moist. Our results suggest changes in riparian soil moisture may have important implications for P release dynamics in field settings. Song et al. (2007) reported greater phosphate solubility in two Korean wetland soils after drying, attributing increases to higher P desorption potential after drying, along with higher phosphatase activity. Other field studies have shown large P release to overlying water upon re-flooding of wetland sediments (Kinsman-Costello et al., 2014). Our results indicate that flooding dry soil enhanced PW-SRP release and mobility to FW compared to flooding the same soils in a field-moist state. The extent of drying (e.g., air-dried in a lab vs. drying conditions in the field) necessary to produce this effect is not yet clear.
Implications for Phosphorus Mobility in Riparian Zones
Our results illustrate that both short- and longer-term flooding released bioavailable P to porewater, with varying degrees of mobilization to overlying water. Results also suggest that riparian sediments derived from these soils have the potential to desorb significant quantities of orthophosphate in aquatic environments. For example, soils with relatively low labile P concentrations still released P at solution concentrations at or exceeding the commonly reported eutrophication threshold (0.03 mg SRP L−1). Mean PW-SRP was ≥0.03 mg SRP L−1 in 13/14 soils while 7/14 soils had mean FW-SRP ≥0.03 mg SRP L−1. Since most crops require soil solution P concentrations of at least 0.1 mg L−1 to support optimum productivity, balancing agronomic and environmental aspects of P is challenging. While our results indicate riparian soils relatively low in labile P may still contribute P to runoff waters, these soils may also sorb P when stream and runoff waters are elevated in SRP. If runoff/floodwater P concentrations exceed equilibrium P concentrations of the sediment being transported, SRP sorption by sediment is expected (Hoffmann et al., 2009). Partitioning of reactive P among dissolved and particulate phases in runoff also depends on runoff sediment concentrations and its degree of P sorption saturation (Sharpley et al., 1981). Riparian soils may alternate between being a source of P and a sink for P depending on biogeochemical dynamics (i.e., redox, pH, microbial activity) and sediment equilibrium P concentrations (Cooper and Gilliam, 1987; Daniels and Gilliam, 1996; Lyons et al., 1998; Hoffmann et al., 2009; Vidon et al., 2010). While SRP release trends varied widely among soils in our study, there was a tendency for net SRP desorption from soils and SRP sorption from FW.
It is possible our experiment over-estimated P release since distilled water was used as floodwater. Compared to the soil solution or runoff water, distilled water may favor greater P desorption since it does not contain P and has negligible ionic strength. Studies report greater P desorption when soils are extracted with distilled water compared to higher ionic strength water (Magdoff et al., 1999; McDowell and Sharpley, 2001). While distilled water may have increased desorption, we nonetheless observed substantial SRP and SUP release. In addition to SRP mobilization from PW to FW, we suspect some SUP also mobilized to floodwater given the extent of SRP mobilization and the fact that SUP is considered less strongly sorbed than orthophosphate. Whereas SRP is immediately bioavailable, SUP must be hydrolyzed to orthophosphate via phosphatases before biological uptake. Previous work with the same soils showed that nearly half of the mean water extractable molybdate unreactive P was hydrolyzed to SRP (Young et al., 2013), indicating mobile unreactive P forms may pose eventual water quality risk.
The interaction between surface and groundwater and its impact on soil biogeochemistry is a major process affecting P fate and transport in riparian buffers (Hoffmann et al., 2009). Our microcosms simulated P desorption to saturated soils (i.e., porewater) and overlying water, a typical condition in flooded and/or saturated riparian zones. While our study suggests the potential for substantial P desorption to porewater and mobilization to overlying water, the fate of P in a field setting is intimately tied to hydrology and biogeochemical conditions along multiple hydrologic flow paths. Scalenghe et al. (2002) suggested P mobilized from fields during flooding is at risk of being transported to adjoining riparian buffers. Such long-term P transport from cropland to adjoining buffers has implications for P mitigation potential. Field management upslope from buffers can enhance or diminish P removal effectiveness in buffers. Additionally, since buffers are not tilled, organic matter and P tend to build up in surface soils, enhancing P desorption potential (Roberts et al., 2012). It is clear from our results that buffer effectiveness would likely decrease over time with increases in labile soil P, leading to higher likelihood of P desorption to runoff waters. Our results further highlight the importance of integrating labile soil P measures with hydrologic flow paths in models to better predict riparian buffer P removal effectiveness.
Conclusion
Phosphorus (P) desorption to soil porewater (PW) and overlying floodwater (FW) in 12 riparian buffer and 2 agricultural floodplain soils from northwestern Vermont, USA was evaluated under moderately reducing conditions designed to mimic a typical riparian condition. Our results showed that while PW-SRP concentrations tended to increase over time, FW-SRP concentrations tended to decrease, indicating soil acted as a net sink for FW-SRP. Soil labile inorganic P concentration (estimated by modified Morgan and distilled water extraction) was the best predictor of SRP released to porewater and mobilization to floodwater. Flooding dry soil decreased FW dissolved oxygen concentrations and increased PW-Fe2+, PW-SRP, and SRP mobilization to FW relative to a field-moist state. While results indicated substantial P desorption to PW occurred in most soils, mobilization to overlying FW was limited by resorption of released P. Our results highlight the importance of integrating measures of labile soil P with hydrologic flow pathways in models to better predict P transport in riparian landscapes.
Author Contributions
EY was a postdoctoral scientist under the direction of DR. The research underpinning this manuscript was part of a grant that EY and DR collaborated on to investigate phosphorus dynamics in riparian soils. DR was the principal investigator responsible for overall project management and EY was a co-PI and took the lead on field and laboratory aspects. Both EY and DR contributed to the design of the study and writing of this manuscript.
Conflict of Interest Statement
The authors declare that the research was conducted in the absence of any commercial or financial relationships that could be construed as a potential conflict of interest.
Acknowledgments
We thank the Vermont Water Resources and Lake Studies Center for funding through an agreement with the U.S. Geological Survey. Conclusions and opinions are those of the authors and not the Vermont Water Resources and Lake Studies Center or the USGS.
Supplementary Material
The Supplementary Material for this article can be found online at: https://www.frontiersin.org/articles/10.3389/fenvs.2018.00120/full#supplementary-material
References
Amarawanshaa, E. A. G. S., Kumaragamage, D., Flatena, D., Zvomuyaa, F., and Tenutaa, M. (2015). Phosphorus mobilization from manure-amended and unamended alkaline soils to overlying water during simulated flooding. J. Environ. Qual. 44, 1252–1262. doi: 10.2134/jeq2014.10.0457
American Public Health Association (1989). “Phosphorus: 4500-P,” in Standard Methods for the Examination of Waste and Wastewaters, 17th Edn., eds A. E. Greenberg, L. S. Clesceri, and A. D. Eaton (Baltimore, MD: Port City Press), 4.166–4.181.
Anderson, H., and Magdoff, F. R. (2005). Relative movement and soil fixation of soluble organic and inorganic phosphorus. J. Environ. Qual. 34, 2228–2233. doi: 10.2134/jeq2005.0025
Barlett, R. J., and James, B. R. (1980). Studying dried, stored soil samples—some pitfalls. Soil Sci. Soc. Am. J. 44, 721–724. doi: 10.2136/sssaj1980.03615995004400040011x
Bartlett, R. J. (1982). Reactive aluminum in the Vermont soil test. Comm. Soil Sci. Plant Anal. 13, 497–506. doi: 10.1080/00103628209367289
Carpenter, S., Caraco, N. F., Correll, D. L., Horwath, R. W., Sharpley, A. N., and Smith, V. H. (1998). Nonpoint pollution of surface waters with phosphorus and nitrogen. Ecol. Appl. 8, 559–568. doi: 10.1890/1051-0761(1998)008[0559:NPOSWW]2.0.CO;2
Condron, L. M., Turner, B. L., and Cade-Menun, B. J. (2005). “Chemistry and dynamics of soil organic phosphorus,” in Phosphorus: Agriculture and the Environment, eds J. T. Sims and A. N. Sharpley (Madison, WI: ASA, CSSA, and SSSA), 87–121.
Cooper, J. R., and Gilliam, J. W. (1987). Phosphorus redistribution from cultivated fields into riparian areas. Soil Sci. Soc. Am. J. 51, 1600–1604. doi: 10.2136/sssaj1987.03615995005100060035x
Dalai, R. C. (1977). Soil organic phosphorus. Adv. Agron. 29, 83–117. doi: 10.1016/S0065-2113(08)60216-3
Daniels, R. B., and Gilliam, J. W. (1996). Sediment and chemical load reduction by grass and riparian buffers. Soil Sci. Am. J. 60, 246–251. doi: 10.2136/sssaj1996.03615995006000010037x
Dieter, D., Herzog, C., and Hupfer, M. (2015). Effects of drying on phosphorus uptake in re-flooded lake sediments. Environ. Sci. Pollut. Res. 22, 17065–17081. doi: 10.1007/s11356-015-4904-x
Dorioz, J. M., Wang, D., Poulenard, J., and Trevisan, D. (2006). The effect of grass buffer strips on phosphorus dynamics-A critical review and synthesis as a basis for application in agricultural landscapes, in France. Agric. Ecosys. Environ. 117, 4–21. doi: 10.1016/j.agee.2006.03.029
Erich, S. M., and Hoskins, B. R. (2011). Effects of soil drying on soil pH and nutrient extractability. Commun. Soil Sci. Plan. Anal. 42, 1167–1176. doi: 10.1080/00103624.2011.566961
Fisher, M. M., and Reddy, K. R. (2001). Phosphorus flux from wetland soils affected by long-term nutrient loading. J. Environ. Qual. 30, 261–271. doi: 10.2134/jeq2001.301261x
Flynn, D. J., and Joslin, R. V. (1979). Soil Survey of Franklin County. Colchester, VT: USDA Soil Conservation Service.
Fuchs, J. W., Fox, G. A., Storm, D. E., Penn, C. J., and Brown, G. O. (2009). Subsurface transport of phosphorus in riparian floodplains: influence of preferential flow paths. J. Environ. Qual. 38, 473–484. doi: 10.2134/jeq2008.0201
Griggs, J. E. (1971). Soil Survey of Addison County. Colchester, VT: USDA Soil Conservation Servive.
Harrison, A. F. (1987). Soil Organic Phosphorus: A Review of the World Literature. Wallingford: CAB Int.
Henderson, R., Kabengi, N., Mantripragada, N., Cabrera, M., Hassan, S., and Thompson, A. (2012). Anoxia-induced release of colloid- and nanoparticle-bound phosphorus in grassland soils. Environ. Sci. Technol. 46, 11727–11734. doi: 10.1021/es302395r
Hill, A. R. (1996). Nitrate removal in stream riparian zones. J. Environ. Qual. 25, 743–755. doi: 10.2134/jeq1996.00472425002500040014x
Hoffmann, C. C., Kjaergaard, C., Uusi-Kämppä, J., Hansen, H. C. B., and Kronvang, B. (2009). Phosphorus retention in riparian buffers: review of their efficiency. J. Environ. Qual. 38, 1942–1955. doi: 10.2134/jeq2008.0087
Hoskins, B. R. (1997). Soil Testing Handbook for Professionals in Agriculture, Horticulture, Nutrient and Residuals Management, 3rd Edn. Orono, ME: Maine Forestry and Agriculture Experiment Station.
Ishee, E., Ross, D. S., Garvey, K., Bourgault, R., and Ford, C. (2015). Phosphorus characterization and contribution from eroding streambank soils of Vermont's Lake Champlain Basin. J. Environ. Qual. 44, 1745–1753. doi: 10.2134/jeq2015.02.0108
Jarvie, H. P., Sharpley, A. N., Spears, B., Buda, A. R., May, L., and Kleinman, P. J. A. (2013). Water quality remediation faces unprecedented challenges from legacy phosphorus. Environ. Sci. Technol. 47, 8997–8998. doi: 10.1021/es403160a
Jokela, B., Magdoff, F., Bartlett, R., Bosworth, S., and Ross, D. (2004). Nutrient Recommendations for Field Crops in Vermont. Br. 1390. Burlington, VT: University of Vermont Extension.
Jokela, J. E., Magdoff, F. R., and Durieux, R. P. (1998). Improved phosphorus recommendations using modified Morgan phosphorus and aluminum soil tests. Commun. Soil Sci. Plant Anal. 29, 1739–1749.
Kinsman-Costello, L. E., O'Brien, J., and Hamilton, S. K. (2014). Re-flooding a historically drained wetland leads to rapid sediment. Ecosystems 17, 641–656. doi: 10.1007/s10021-014-9748-6
Kline, M., and Cahoon, B. (2010). Protecting river corridors in Vermont. J. Am. Water Resour. Assoc. 46, 227–236. doi: 10.1111/j.1752-1688.2010.00417.x
Kronvang, B., Audet, J., Baattrup-Pedersen, A., Jensen, H. S., and Larsen, S. E. (2012). Phosphorus load to surface water from bank erosion in a Danish lowland river basin. J. Environ. Qual. 41, 304–313. doi: 10.2134/jeq2010.0434
Lake Champlain Basin Program (2015). Available online at: http://sol.lcbp.org/Phosphorus_where-does-p-come-from.html
Langendoen, E. J., Simon, A., Klimetz, L., Bankhead, N., and Ursic, M. E. (2012). Quantifying Sediment Loadings From Streambank Erosion in Selected Agricultural Watersheds Draining to Lake Champlain. Final report. Washington, DC: National Sedimentation Laboratory, USDA–ARS.
Loeb, R., Lamers, L. P. M., and Roelofs, J. G. M. (2008). Prediction of phosphorus mobilisation in inundated floodplain soils. Environ. Pollut. 156, 325–331. doi: 10.1016/j.envpol.2008.02.006
Lumsdon, D. G., Shand, C. A., Wendler, R., Edwards, A. C., Stutter, M. I., Richards, S., et al. (2016). The relationship between water soluble P and modified Morgan P: results based on data and chemical modelling. Soil Use Manag. 32, 162–171. doi: 10.1111/sum.12265
Lyons, J. B., Gorres, J. H., and Amador, J. A. (1998). Spatial and temporal variability of phosphorus retention in a riparian forest soil. J. Environ. Qual. 27, 895–903. doi: 10.2134/jeq1998.00472425002700040025x
Magdoff, F. R., Hryshko, C., Jokela, W. E., Durieux, R. P., and Bu, Y. (1999). Comparison of phosphorus soil test extractants for plant availability and environmental assessment. Soil Sci. Soc. Am. J. 63, 999–1006. doi: 10.2136/sssaj1999.634999x
Maguire, R. O., Condron, W. J., and Simard, R. R. (2005). “Assessing potential environmental impacts of soil phosphorus by soil testing,” in Phosphorus: Agriculture and the Environment, eds J. T. Sims and A. N. Sharpley (Madison, WI: ASA, CSA, SSSA), 145–180.
McDowell, R. W., and Sharpley, A. N. (2001). Approximating phosphorus release from soils to surface runoff and subsurface drainage. J. Environ. Qual. 30, 508–520. doi: 10.2134/jeq2001.302508x
McIntosh, J. L. (1969). Bray and Morgan soil extractants modified for testing acid soils from different parent materials. Agron. J. 61, 259–265.
Moore, P. A., and Reddy, K. R. (1994). Role of Eh and pH on phosphorus geochemistry in sediments of Lake Okeechobee, Florida. J. Environ. Qual. 23, 955–964.
Moore, P. A., Reddy, K. R., and Fisher, M. M. (1998). Phosphorus flux between sediment and overlying water in Lake Okeechobee, Florida: spatial and temporal variations. J. Environ. Qual. 27, 1428–1439. doi: 10.2134/jeq1998.00472425002700060020x
Morgan, M. F. (1941). Chemical Soil Diagnosis by the Universal Soil Testing System.. New Haven, CT: Connecticut Agricultural Experiment Station.
Muscutt, A. D., Harris, G. L., Bailey, S. W., and Davies, D. B. (1993). Buffer zones to improve water quality: a review of their potential use in UK agriculture. Agric. Ecosyst. Environ. 45, 59–77. doi: 10.1016/0167-8809(93)90059-X
Muss, M. L., and Mellen, M. G. (1942). Colorimetric determination of iron with 2,29-bipyridine and with 2,2',2”-tripyridine. Ind. Eng. Chem. Anal. Ed. 14:862.
Nelson, N. O., and Parsons, J. E. (2006). “Basic approaches to modeling phosphorus leaching,” in Modeling Phosphorus in the Environment, eds D. E. Radcliffe, and M. L. Cabrera (Boca Raton, FL: CRC Press), 81–103.
Novak, J. M., Stone, K. C., Szogi, A. A., Watts, D. W., and Johnson, M. H. (2004). Dissolved phosphorus retention and release from a coastal plain in-stream wetland. J. Environ. Qual. 33, 394–401. doi: 10.2134/jeq2004.3940
O'Halloran, I. P., and Cade-Menun, B. J. (2008). “Total and organic phosphorus,” in Soil Sampling and Methods of Analysis, 2nd Edn eds M. R. Carter and E. G. Gregorich (Boca Raton, FL: CRC Press), 265–291.
Parsons, C. T., Rezanezhad, F., O'Connell, D. W., and Van Cappellen, P. (2017). Sediment phosphorus speciation and mobility under dynamic redox conditions. Biogesciences 14, 3585–3602. doi: 10.5194/bg-14-3585-2017
Pierzynski, G. M., McDowell, R. W., and Sims, J. T. (2005). “Chemistry, cycling, and potential movement of inorganic phosphorus in soils,” in Phosphorus: Agriculture and the Environment, eds J. T. Sims and A. N. Sharpley (Madison, WI: ASA, CSA, SSSA), 53–86.
Ponnamperuma, F. N. (1972). The chemistry of submerged soils. Adv. Agron. 24, 29–96. doi: 10.1016/S0065-2113(08)60633-1
Quiquampoix, H., and Mousain, D. (2005). “Enzymatic hydrolysis of organic phosphorus,” in Organic Phosphorus in the Environment, eds B. L. Turner, E. Frossard, and D. S. Baldwin (Cambridge, MA: CABI), 89–112.
Roberts, W. M., Stutter, M. I., and Haygarth, P. M. (2012). Phosphorus retention and remobilization in vegetated buffer strips: a review. J. Environ. Qual. 41, 389–399. doi: 10.2134/jeq2010.0543
Scalenghe, R., Edwards, A. C., Ajmone Marsan, F., and Barberis, E. (2002). The effect of reducing conditions on P solubility for a diverse range of European agricultural soils. Eur. J. Soil Sci. 53, 439–448. doi: 10.1046/j.1365-2389.2002.00462.x
Scalenghe, R., Edwards, A. C., and Barberis, E. (2007). Phosphorus loss in over- fertilized soils: the selective P partitioning and redistribution between particle size separates. Eur. J. Agron. 27, 72–80. doi: 10.1016/j.eja.2007.02.002
Sekely, A. C., Mulla, D. J., and Bauer, D. W. (2002). Streambank slumping and its contribution to the phosphorus and suspended sediment loads of the Blue Earth River, Minnesota. J. Soil Water Conserv. 57, 243–250.
Sharpley, A., Jarvie, H. P., Buda, A., May, L., Spears, B., and Kleinman, P. (2013). Phosphorus legacy: overcoming the effects of past management practices to mitigate future water quality impairment. J. Environ. Qual. 42, 1308–1326. doi: 10.2134/jeq2013.03.0098
Sharpley, A. N., Menzel, R. G., Smith, S. J., Rhoades, E. D., and Olness, A. E. (1981). Sorption of soluble phosphorus by soil material during transport in runoff from cropped and grassed watersheds. J. Environ. Qual. 10, 211–215. doi: 10.2134/jeq1981.00472425001000020018x
Sims, J. T., and Pierzynski, G. M. (2005). “Chemistry of phosphorus in soils,” in Chemical Processes in Soils, eds M. A. Tabatabai, and D. L. Sparks (Madison, WI: SSSA), 151–192.
Sims, J. T., Simard, R. R., and Joern, B. C. (1998). Phosphorus loss in agricultural drainage: historical perspective and current research. J. Environ. Qual. 27, 277–293. doi: 10.2134/jeq1998.00472425002700020006x
Smeltzer, E., Dunlap, F., and Simoneau, M. (2009). Lake Champlain Phosphorus Concentrations and Loading Rates, 1990–2008. Technical report #57. Lake Champlain Basin Program, Grand Isle, VT.
Song, K. Y., Zoh, K. D., and Kang, H. (2007). Release of phosphate in a wetland by changes in hydrological regime. Sci. Total Environ. 380, 13–18. doi: 10.1016/j.scitotenv.2006.11.035
Surridge, B. W. J., Heathwaite, A. L., and Baird, A. J. (2007). The release of phosphorus to porewater and surface water from River Riparian sediments. J. Environ. Qual. 36, 1534–1544. doi: 10.2134/jeq2006.0490
Turner, B. L. (2005). “Organic phosphorus transfer from terrestrial to aquatic environments,” in Organic Phosphorus in the Environment, eds B. L. Turner, E. Frossard, and D. S. Baldwin (Cambridge, MA: CABI), 269–294.
Turner, B. L., Cade-Menun, B. J., and Westerman, D. T. (2003). Organic phosphorus composition and potential bioavailability in semiarid arable soils of the western United States. Soil Sci. Soc. Am. J. 67, 1168–1179. doi: 10.2136/sssaj2003.1168
Turner, B. L., and Haygarth, P. M. (2001). Phosphorus solubilization in rewetted soils. Nature 411:258. doi: 10.1038/35077146
Turner, B. L., and Haygarth, P. M. (2003). Changes in bicarbonate-extractable inorganic and organic phosphorus by drying pasture soils. Soil Sci. Soc. Am. J. 67, 344–350. doi: 10.2136/sssaj2003.3440
Turner, B. L., McKelvie, I. D., and Haygarth, P. M. (2002). Characterization of water extractable soil organic phosphorus by phosphatase hydrolysis. Soil Biol. Biochem. 34, 27–35. doi: 10.1016/S0038-0717(01)00144-4
Vadas, P. A., Kleinman, P. J. A., Sharpley, A. N., and Turner, B. L. (2005). Relating soil phosphorus to dissolved phosphorus in runoff. J. Environ. Qual. 34, 572–580. doi: 10.2134/jeq2005.0572
Vadas, P. A., and Sims, J. T. (1998). Redox status, poultry litter, and phosphorus solubility in Atlantic Coastal Plain soils. Soil Sci. Soc. Am. J. 62, 1025–1034. doi: 10.2136/sssaj1998.03615995006200040025x
Vadas, P. A., and Sims, J. T. (1999). Phosphorus sorption in manured Atlantic Coastal Plain soils under flooded and drained conditions. J. Environ. Qual. 28, 1870–1877. doi: 10.2134/jeq1999.00472425002800060025x
Vidon, F., Allan, C., Burns, D., Duval, T. P., Gurwick, N., Inamdar, S., et al. (2010). Hot spots and hot moments in riparian zones: potential for improved water quality management. J. Am. Water Resour. Assoc. 46, 278–298. doi: 10.1111/j.1752-1688.2010.00420.x
Wright, R. B., Lockaby, B. G., and Walbridge, M. R. (2001). Phosphorus availability in an artificially flooded southeastern floodplain forest soil. Soil Sci. Soc. Am. J. 65, 1293–1302. doi: 10.2136/sssaj2001.6541293x
Young, E. O., and Briggs, R. D. (2008). Phosphorus concentrations in soil and subsurface water: a field study among cropland and riparian buffers. J. Environ. Qual. 37, 69–78. doi: 10.2134/jeq2006.0422
Young, E. O., and Ross, D. S. (2001). Phosphate release from seasonally flooded soils: a laboratory microcosm study. J. Environ. Qual. 30, 91–101.
Young, E. O., and Ross, D. S. (2016). Total and labile phosphorus concentrations as influenced by riparian buffer soil properties. J. Environ. Qual. 45, 294–304. doi: 10.2134/jeq2015.07.0345
Young, E. O., Ross, D. S., Alves, C., and Villars, T. (2012). Soil and landscape influences on native riparian phosphorus availability in three Lake Champlain Basin stream corridors. J. Soil Water Conserv. 67, 1–7. doi: 10.2489/jswc.67.1.1
Young, E. O., Ross, D. S., Cade-Menun, B. J., and Liu, C. (2013). Phosphorus speciation in riparian soils: a phosphorus-31 nuclear magnetic resonance and enzyme hydrolysis study. Soil Sci. Soc. Am. J. 77, 1636–1647. doi: 10.2136/sssaj2012.0313
Young, E. O., Ross, D. S., and Hoskins, B. R. (2017). Phosphorus in Morgan soil test extracts measured by molybdate colorimetric and inductively coupled plasma spectroscopy methods. Commun. Soil Sci. Plant Anal. 48, 2434–2446. doi: 10.1080/00103624.2017.1411936
Keywords: soluble reactive phosphorus, desorption, redox, riparian buffer, water quality
Citation: Young EO and Ross DS (2018) Phosphorus Mobilization in Flooded Riparian Soils From the Lake Champlain Basin, VT, USA. Front. Environ. Sci. 6:120. doi: 10.3389/fenvs.2018.00120
Received: 28 April 2018; Accepted: 24 September 2018;
Published: 18 October 2018.
Edited by:
Christophe Darnault, Clemson University, United StatesReviewed by:
Meihua Deng, Tsinghua University, ChinaPhilippe Cambier, Institut National de la Recherche Agronomique (INRA), France
Copyright © 2018 Young and Ross. This is an open-access article distributed under the terms of the Creative Commons Attribution License (CC BY). The use, distribution or reproduction in other forums is permitted, provided the original author(s) and the copyright owner(s) are credited and that the original publication in this journal is cited, in accordance with accepted academic practice. No use, distribution or reproduction is permitted which does not comply with these terms.
*Correspondence: Eric O. Young, ZXJpYy55b3VuZ0BhcnMudXNkYS5nb3Y=
†Present Address: Eric O. Young, USDA-ARS Institute for Environmentally Integrated Dairy Management, Marshfield, WI, United States