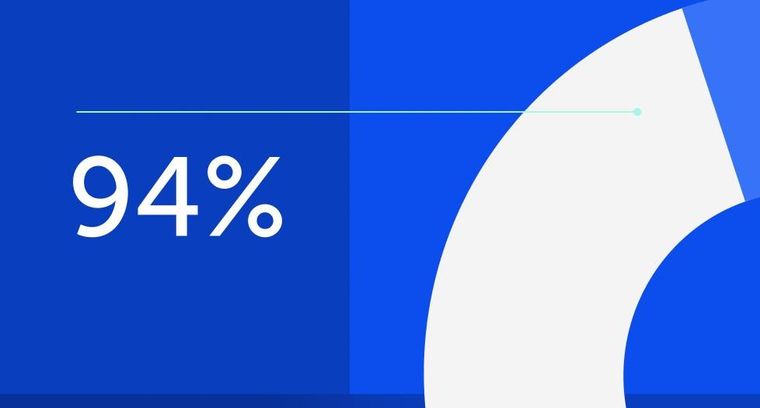
94% of researchers rate our articles as excellent or good
Learn more about the work of our research integrity team to safeguard the quality of each article we publish.
Find out more
ORIGINAL RESEARCH article
Front. Environ. Sci., 16 October 2018
Sec. Soil Processes
Volume 6 - 2018 | https://doi.org/10.3389/fenvs.2018.00117
This article is part of the Research TopicRhizosphere Functioning and Structural Development as Complex Interplay between Plants, Microorganisms and Soil MineralsView all 18 articles
Arbuscular mycorrhizal fungi (AMF) are important symbionts for plant nutrient uptake, but their exact role in plant nitrogen (N) nutrition is unclear. Protists on the other hand play an acknowledged role in plant N acquisition, and there is increasing evidence for a close interaction with AMF. In a split root set up, we investigated the distinct roles of mycorrhiza (Rhizophagus irregularis), protists (Acanthamoeba castellanii), and their interaction on plant N uptake, within-root system allocation patterns, and shoot-to-root ratio of winter wheat. In addition, we applied a quantitative metabolomics approach to characterize associated changes in soil microbial communities by microbial phospholipid fatty acid (PLFA) analysis from rhizosphere soil. AMF markedly altered plant shoot-to-root allometry by reducing root biomass of wheat, and mycorrhiza partly took over root system functioning. Protists promoted shoot and root growth, and improved plant N uptake by the release of N from consumed bacterial biomass, a mechanism known as microbial loop. The shoot system however responded little to these alterations of the root system and of the rhizosphere community composition, indicating that the plants optimized shoot growth despite varying investment into roots. Mycorrhiza reduced root biomass and plant N, especially in the combined treatments with protists by changing within root system allocation of N and root biomass. These systemic effects on root allocation pattern suggest that mycorrhiza also gained control over N provided by protist grazers. Protists and mycorrhiza altered rhizosphere bacterial communities in contrasting but consistent ways as shown by quantitative shifts in microbial PLFA profiles. Remarkably, the changes in bacterial community composition were systemically conveyed within the root system to the split-root chamber where the symbionts were lacking. Accordingly the synergistic effects of protists and mycorrhiza indicated systemic effects on nutrient- and on root-allocation within root systems as an emergent property that could not be predicted from single treatments with mycorrhiza or protists alone. The tight plant and microbial feed backs uncovered in this study have far reaching implications for understanding the assembly of plant microbiomes, and testify central roles of both protists and mycorrhizas in the assembly process.
Frameworks on shoot-to-root allometry of plants are based on models of competition between shoots and roots for plant carbohydrates and nutrients (Bloom et al., 1985; Van Noordwijk and De Willigen, 1987; Ericsson, 1995). Recently, models on resource stoichiometry (Johnson, 2010) and microbial markets (Werner et al., 2014; Revillini et al., 2016) have been applied to plant-symbiont interactions, but these approaches have not been rigorously tested on the individual plant level. In particular root traits have been considered to be determined by plant physiology and genetic disposition (Hoad et al., 2001; Wasson et al., 2012) or by external factors, such as different forms of nutrients (López-Bucio et al., 2003; Tian et al., 2014). On the other hand an abundant literature exists on influence of soil microorganisms affecting the root architecture of plants.
In the past decade much has been learned about how free-living rhizosphere microorganisms shape plant traits (Friesen et al., 2011; Lau and Lennon, 2012). Arbuscular mycorrhizal fungi (AMF) are among the most important mutualists improving nutrient acquisition of plants (Smith and Read, 2008; Bonfante and Genre, 2010). This is well confirmed for plant phosphorus uptake (Sanders and Tinker, 1971; Olsson et al., 2010; Smith F. A. and Smith, 2011), but the role of AMF in plant nitrogen (N) uptake is controversial (Smith S. E. and Smith, 2011; Veresoglou et al., 2012a). Although AMF hyphae have been shown to preferentially colonize N-containing organic patches in soil (Bukovská et al., 2016) and can aid in plant N-uptake (Hodge et al., 2001), they also function as N sink (Hodge and Fitter, 2010). Moreover, AMF lack the enzymatic machinery for N mineralization (Smith S. E. and Smith, 2011) and hence rely on other microorganisms for the supply of mineral N (Herman et al., 2012; Koller et al., 2013a).
Protists are ubiquitous predators of soil bacteria in the plant rhizosphere and release considerable amounts of N from consumed bacterial prey (Kuikman et al., 1991), a mechanism described as “microbial loop” (Clarholm, 1985; Bonkowski, 2004). While it has been shown that bacteria suppress AMF colonization of roots when competing for N (Leigh et al., 2011; Hodge and Storer, 2014), protist predation shifts nutrient competition in favor of the mycorrhizal symbiont since AMF hyphae effectively take up the N released by protist predators (Herdler et al., 2008; Koller et al., 2013b; Bukovská et al., 2018).
There is also potential conflict between AMF and protists in respect to the allocation of plant C in the rhizosphere. AMF act as strong sink for host plant C (Gange and Ayres, 1999; Klironomos, 2003; Lerat et al., 2003) and generally lead to reduced root growth (Veresoglou et al., 2012b). AMF also reduce the concentration of carbohydrates in root exudates (Graham et al., 1981; Ryan et al., 2012), resulting in reduced substrate supply to free-living rhizosphere bacteria and their consumers. Acanthamoebae have been found to stimulate lateral root branching and root growth, thereby likely enhancing root exudation and triggering a positive feedback in the rhizosphere microbial food web (Bonkowski and Brandt, 2002; Kreuzer et al., 2006; Krome et al., 2009, 2010; Bonkowski and Clarholm, 2012). These effects are reminiscent of the stimulation of root growth by plant growth promoting rhizobacteria (Bonkowski and Brandt, 2002; Bonkowski, 2004). In accordance with this view, changes in the rhizosphere bacterial community due to protist predation are non-random (Kreuzer et al., 2006; Jousset et al., 2008, 2009; Rosenberg et al., 2009), but also AMF have been reported to affect rhizosphere bacterial communities in specific ways (Marschner and Baumann, 2003; Rillig et al., 2006; Scheublin et al., 2010; Nuccio et al., 2013; Bukovská et al., 2016), suggesting additive effects on bacterial community assembly.
Here we investigated the specific roles of AMF and protists in determining nutrient fluxes in the rhizosphere. Further, we explore the interaction of AMF and protists as major rhizosphere symbionts and their effects on rhizosphere bacterial communities. A split root experiment was established to investigate systemic effects, and the role of the plant in mediating AMF and protist interactions. Rhizosphere nutrient fluxes were quantified by measuring plant biomass and nutrient concentrations in roots and shoots. Changes in the microbial community composition and activity were measured using phospholipid fatty acid (PLFA) profiles and microbial respiration.
The following hypotheses were tested:
1) From the perspective of the plant mycorrhiza and protists appear complementary. Mycorrhizal hyphae take over root system functions by capturing and transporting nutrients to the plant, while protists increase nutrient availability by mineralizing nutrients locked in bacterial biomass.
2) Both, mycorrhiza and protists alter the structure of the bacterial community in the rhizosphere with mycorrhiza increasing and protists decreasing bacterial biomass.
A clay loess soil was taken from a meadow (“Molino-Arrhenatheretea”) on a basalt outcropping near Darmstadt, Germany. The soil was sampled from 5 to 20 cm depth and sieved (5 mm) to remove roots and stones. The soil was autoclaved (121°C, 110 kPa, 30 min). The soil pH(KCl) was 6.9 and the C:N ratio 18 (16 mg g−1 C and 0.9 mg g−1 N).
Winter wheat (Triticum aestivum L. var. Naxos) seeds were sterilized according to Hensel et al. (1990), by subsequent treatment with 96% ethanol (2 min), and 5% NaOCl (5 min) under vacuum in a desiccator. After rinsing with distilled water the seeds were germinated in 100 μl Neff's Modified Amoeba Saline (NMAS; Page, 1976) in individual wells of 96 well microtitre plates (Greiner, Solingen Germany) in darkness at 20°C. After 4 days, sterile seedlings were transferred on sterile 1% Agar containing nutrient broth (NB; Merck, Darmstadt, Germany) and NMAS (at 1:9 v:v; NB-NMAS) in a Petri dish and stored in a climate chamber (20°C, 70% relative humidity, 14/10 h light/ dark cycle). A total of 25 sterile wheat seedlings of 8 cm shoot length and multiple secondary roots of over 5 cm length were selected for the experiment.
The autoclaved soil was immediately inoculated with a bacterial filtrate from meadow rhizosphere soil to re-establish a diverse bacterial community. The protist-free bacterial filtrate was obtained by suspending 50 g fresh meadow soil (Biology Campus, TU Darmstadt) in 200 ml sterile tap water and filtering it through paper filters (Schleicher & Schuell, Dassel, Germany) and subsequent 5.0 and 1.2 μm Isopore filters (Millipore, Schwalbach, Germany). The filtrate was incubated at 15°C for 7 days and controlled daily for protist contaminations.
Acanthamoeba castelanii Neff, a ubiquitous naked amoeba in soils was used as model protist (Fiore-Donno et al., 2016). Acanthamoeba castelanii was isolated from a beechforest soil and kept in NB-NMAS at 5°C on native soil bacteria as food (Bonkowski and Brandt, 2002). The amoebae were separated from the bacteria using repeated centrifugation (1 min, 1,000 rpm) and washing with NB-NMAS (Herdler et al., 2008). The resulting protists suspension was used to inoculate the protist treatments (0.5 ml per chamber). The supernatant containing bacteria from the amoeba culture was filtered (3 μm, Millipore Corporation, Bedford MA, USA) to obtain a protist-free mock community and to inoculate all non-protist treatments.
An AMF in vitro culture of Rhizophagus irregularis (previously Glomus intraradices; Stockinger et al., 2009) was sourced from the Mycothèque de l'Université Catholique de Louvain (MUCL, Belgium) and used as a mycorrhizal inoculum (Bonkowski, 2018).
Split root containers were constructed out of PVC with inner dimensions of 8 × 7.5 × 2 cm of each root compartment (Figure 1). The front and back walls were transparent, allowing for monitoring root growth and soil moisture. The containers were divided into equally dimensioned right and left chambers. The only connection point between the two chambers was a groove introduced at the top of the split root container to house the plant's root crown. The plant groove and the mating faces of the chamber walls were coated with high viscosity silicone paste (Baysilone, Bayer, Leverkusen, Germany) to completely isolate each soil chamber, and the walls were secured at multiple points with screws. The top of each chamber contained a 10 mm diameter aeration opening. Each chamber was watered in an automatic way using a glass fiber capillary wick (Ortmann, Hilden, Germany) housed in a PE hose fed into through the bottom of the chamber, leading into a 250 ml PP wide neck bottle. This enabled the individual plant roots to adjust their water uptake according to demand. This is crucial in the early growth phase, as water demand of individual root systems in a split root chamber cannot be exactly determined by weighing and external watering.
Figure 1. Technical sketch of the split root container, outer dimensions were 18.0 × 15.6 × 2.6 cm. Irrigation system only shown for the right chamber.
The containers were sterilized in 70% ethanol and the irrigation systems were autoclaved at 121°C and 110 kPa for 30 min prior to construction. Each chamber was filled with soil corresponding to 200 g dry weight. A seedling was set into the plant groove and its roots were equally separated into both root chambers. A 1 cm2 piece of agar containing spores and hyphae of R. irregularis was applied to the roots in chambers designated for mycorrhiza. Each chamber was inoculated with 1 ml bacterial suspension. Chambers designated for protists were inoculated with 1 ml amoeba suspension, all other chambers received 1 ml NB-NMAS containing the mock community from amoeba cultures. The aeration openings were closed by sterile cotton plugs to protect the soil from airborne contamination. After inoculation, the chambers were wrapped in aluminum foil to prevent light exposure of soil and roots.
The experiment was set up in a two-factorial design with the factors protists (A. castelanii) and mycorrhiza (R. irregularis). Five treatments and treatment combinations were set up, each with five replicates: (1) control (0-0) with only bacterial inoculum in both chambers of the split-root container, (2) protists (0-P) as 0-0, but inoculated additionally with A. castellanii in one chamber, (3) mycorrhiza (0-M) as 0-0, but inoculated additionally with R. irregularis in one chamber, (4) as 0-0, but protists and mycorrhiza (0-PM) inoculated together in one chamber, (5) protists and mycorrhiza inoculated on separate chambers (P-M), i.e., one chamber containing protists and the other mycorrhiza. The split root containers were arranged in a randomized complete block design with five containers of each treatment in each of five blocks, which were clockwise rotated every 3 days. During these rotations the poly-propylen water suppply bottles (Figure 1) were weighed to check for water loss. These measurements allowed calculating the water use of each plant.
The experiment was run for 65 days in a climate chamber at 20 ± 2°C and a relative humidity of 70%. The climate chamber was illuminated with HI-R lights (Osram, Munich Germany) with 350 μmol m−2 s−1 and a 14/10 h day/night cycle. After 4 weeks, plant water requirements exceeded the capacity of the capillary wicks, and root chambers were irrigated through the aeration openings with equal volumes of supplementary sterile distilled water every 3 days to adjust for weight lost.
Plant roots were carefully picked and sieved from the soil, and the collected roots from both chambers were washed separately and dried, along with the shoots, at 60°C for 72 h, then weighed to obtain dry weight biomass. The dried shoots were blender homogenized (Krups; Solingen, Germany). One gram of shoot material and 1 g of root material taken from the center of the root sample of each root halve was pulverized in a ball mill (MM 200 Retsch, Haan, Germany), and its C and N concentration measured using an elemental analyser (Na1500; Carlo Erba; Milan, Italy). In addition, 200 mg of the milled shoots and roots were dissolved in 2 ml 65% nitric acid and digested using a closed vessel microwave digester (Agazzi and Pirola, 2000). The concentrations of P, Mn, Mg, Ca, K, Al, Na, Fe, and S were determined using Inductive Coupled Plasma—Atom Emission Spectral analysis (ICP-AES, Thermo Scientific iCAP6500).
Soil remaining after the collection of roots was sieved (2 mm), and a sample corresponding to 2 g dry weight was brought to a moisture content of 30% with distilled water. The O2 consumption rate of this soil was measured every hour over 48 h using a respirometer (Scheu, 1992). Microbial basal respiration (μl O2 g−1 h−1) was measured over 24 h. Microbial biomass (Cmic; μg C g−1 dw) was measured by substrate-induced respiration (SIR; Anderson and Domsch, 1978) after adding glucose (8 mg g−1 dry wt soil), and calculated according to Beck et al. (1997). Microbial specific respiration was calculated as the quotient of basal respiration and microbial biomass.
Nutrient limitation of the microbial community was investigated by adding N [as NH4NO3(aq)] and P [as KH2PO4(aq)] along with C [as glucose(aq); 8 mg g−1 dry wt] at a C:N:P mass ratio of 10:2:1 (Anderson and Domsch, 1980) and measuring the slope of the corresponding microbial growth curve (log transformed) using the O2 microcompensation system (Griffiths et al., 2001).
Protist density (Figure S2) was determined by the Most Probable Number technique (MPN; Darbyshire et al., 1974). Five g fresh weight soil from different locations of each chamber were suspended in 20 ml NMAS by shaking (100 rpm, 20 min). Four replicated 100 μl aliquots suspension per sample were 3-fold diluted in NB-NMAS in 96-well microtiter plates and incubated in darkness at 20°C. Wells were checked daily for amoebae, flagellates and ciliates over a period of 10 days using an inverse microscope (100 −400x; Fluovert FU, Leitz; Marburg, Germany).
A quantitative metabolomics approach was used to characterize shifts in microbial communities. The phospholipid fatty acids (PLFAs) composing the membrane lipids of the microorganisms in soil were quantitatively extracted from 1 g soil following Frostegård et al. (1991) with Bligh/Dyer solution [chloroform, methanol, citrate buffer (pH = 4; 1:2:0.8; v/v/v)] and the solution passed through silica acid columns (0.5 g silicic acid, 3 ml; Varian Medical Systems, Palo Alto, California).
PLFAs were transformed into fatty acid methyl esters (FAMEs) by mild alkaline methanolysis as described in Frostegård et al. (1991). The FAMEs were measured using an AutoSystem XL gas chromatograph (Perkin-Elmer Corporation, Norwalk, CT, USA). GC settings were as in Kramer et al. (2013). Identification of FAMEs was based on their retention time assessed with a FAME and a bacterial acid methyl ester mix standard (Sigma-Aldrich, St. Louis, USA). An internal FAME standard (Sigma-Aldrich, St. Louis, USA) added to the samples before methanolysis was used for quantification.
FAMEs were identified by GC-MS using a HP 5890 Series II Plus coupled with a 5972 mass selective detector (Hewlett Packard/Agilent, Waldbronn, Germany) equipped with a DB-5MS column. GC-MS settings were as in Kramer et al. (2013). A mass range of m/z 50–450 was monitored. The branched fatty acids i15:0, a15:0, i16:0, and i17:0 were considered as Gram positive and the cy17:0 and cy19:0 as Gram negative bacterial markers (Zelles, 1999). The following PLFAs were used to infer microbial community structure: 14:0, i15:0, a15:0, 15:0, i16:0, 16:1ω7, 16:0, i17:0, cy17:0, 17:0, 18:2ω6, 18:2ω6t/18:1ω9/18:3ω3, 18:1ω7/18:1ω9t, 18:0, cy19:0 (Frostegård and Bååth, 1996; Zelles, 1999). Since the PLFA 16:1ω5, often being used as mycorrhizal marker in environmental studies, can be found in both bacteria and mycorrhiza (see Ngosong et al., 2012) it was discarded from the analysis. Likewise, unsaturated C20 PLFAs occur in a wide range of eukaryotes (Ruess and Chamberlain, 2010) and were discarded from the analyses.
The data were analyzed by a two factorial (protists and mycorrhiza) general linear model (GLM) with a priori singular contrasts. Shoot and root data were analyzed separately. In the first step, treatments with protists (0-P) and mycorrhiza (0-M) alone were compared to the control (0-0). Subsequently, the shoot and root data of the combined treatments (0-PM, P-M) were compared to those of treatments with mycorrhiza alone (0-M) and protists alone (0-P). Roots in the split-root containers had a “treated” and a “non-treated” chamber, except in P-M where both root chambers were differently treated. The split-root set up therefore allowed to compare the “treated” chambers between treatments; and further to analyze systemic effects of the “treated” root section on the “non-treated” root section by comparing root chambers within treatments. In the 0-0 treatment one root chamber of each replicate was a priori randomly assigned as control. For between-treatment comparisons, the root chambers treated with protists (0-P) or mycorrhiza (0-M) were compared to the control chamber of the 0-0 treatment, and to the chamber treated with protists and mycorrhiza (0-PM). In the P-M treatment, where protists and mycorrhiza were spatially separated, the protist-inoculated chamber was compared to the protist treated chamber of 0-P, while the mycorrhiza-inoculated chamber was compared to the mycorrhiza treated chamber of 0-M. Statistically significant contrasts between “treated” root chambers are indicated by horizontal bars in figures. Subsequently, systemic effects of the “treated” root section vs. the “non-treated” root section within treatments, and between both treated chambers of the P-M treatment were analyzed by one-way analysis of variance (ANOVA). Data were analyzed using SAS V8.2 software (SAS Institute; Cary, North Carolina, USA). Discriminant function analysis (DFA) was used to inspect the overall structure of the PLFA profiles in order to differentiate the effects of different treatments on soil bacteria. Through subsequent correlation of single fatty acids with both major axes of the DFA, we investigated which fatty acids were responsible for the discrimination of samples. DFA was carried out in R version 3.3.3, using the packages MASS (Venables and Ripley, 2002), and ggplot2 (Wickham, 2016) for plotting.
Plant shoot-to-root allometry and within-root system allometry between both split-root chambers provides information on potential competition between shoots and roots and between roots and microbes for carbohydrates and nutrients. Average total biomass of shoots and roots in the control treatment (0-0) was 4.93 ± 0.7 and 6.14 ± 2.3 g dry wt, respectively. Presence of protists (0-P) caused a significant increase in total plant biomass by 24% as compared to the control (Figure 2A; Table 1). Protists also tended to increase shoot biomass (+22%). Mycorrhiza in contrast had no effect on shoot biomass but significantly increased the shoot-to-root ratio (+74%), due to (in trend) reduced total root biomass [−37%, 0-0 vs. 0-M, F(1, 23) = 3.15; p = 0.089; Figure 2A]. Root biomass of the mycorrhiza chamber in the 0-M treatment appeared particularly low, but the reduction was not statistically significant due to high variation in the non-inoculated root compartment [F(1, 6) = 3.60; p = 0.107]. Total root biomass was also reduced when both mycorrhiza and protists were combined (0-PM, P-M; Table 1). Total root biomass decreased as compared to 0-P by −34 and −31% in the 0-PM and P-M treatments, and resulted in an increased shoot-to-root ratio by 38 and 40% in the 0-PM and P-M treatments, respectively (Figure 2A; Table 1).
Figure 2. Biomass (A) total N mass (mg) (B) and C:N mass ratio (C) of shoots and of roots of Triticum aestivum in both root chambers of control plants (0-0), plants treated with protists in the right root chamber (0-P), or treated with mycorrhiza in the right root chamber (0-M), or treated with protists and mycorrhiza together in the right root chamber (0-PM), or where the left and right root chambers were separately treated with protists and mycorrhiza (P-M). Means and standard deviation; horizontal lines denote significant contrasts with (*)p < 0.1, *p < 0.05, ***p < 0.001.
Table 1. F- and p-values of GLM contrasts showing treatment effects on total plant biomass, shoot biomass, biomass of the total root system, and shoot-to-root ratio of Triticum aestivum.
Protists mitigated some of the mycorrhiza induced reductions of root systems, resulting in a 2.57-fold increase of root biomass in the mycorrhiza chamber of the P-M treatment as compared to the mycorrhiza chamber of the 0-M treatment [F(1,23) = 5.69; p = 0.026]. This was because root biomass in the mycorrhiza chamber of the P-M treatment was 2-fold higher than that in the neighboring protist chamber, albeit with marginal significance [F(1,8) = 4.42; p = 0.069]. The complementarity of protists in combination with mycorrhiza was further supported by measurements of plant water uptake, which was significantly higher in 0-PM (p < 0.001) and P-M (p = 0.018) treatments compared to the 0-M treatment (Figure S2, Table S2).
Differences in plant nutrient uptake between control (0-0) and microbial treatments reveal either mutualistic, neutral or parasitic relationships in symbioses. Comparing differently inoculated root chambers can reveal competition or synergism between different microorganism groups. Mycorrhiza and protists altered root N mass in opposite directions, with protists increasing and mycorrhiza reducing it; and the negative mycorrhiza effect continued to dominate in 0-PM and P-M treatments. In the 0-P treatment, root N mass of the protist chamber increased by 52% as compared to the control [0-0; F(1, 23) = 4.32; p = 0.049], but this effect was local and increased total root N mass (+30%) and total plant N mass (+22%) only with marginal significance (Figure 2B; Table 2).
Table 2. F- and P-values of GLM contrasts showing treatment effects on total shoot N mass, total root N mass, total plant N mass, and shoot-to-root N mass ratio of Triticum aestivum.
In the mycorrhiza chamber of the 0-M treatment, the root N mass decreased by 66% as compared to the control [0-0; F(1, 23) = 6.05; p = 0.022]. This led to a 58% reduction in total root N mass, while total plant N mass was only reduced by 32% (Figure 2B; Table 2). The nitrogen reduction in the mycorrhiza chamber of the 0-M treatment led to a 2.72-fold increase in the shoot-to-root N mass ratio as compared to the control (0-0) as shoot N was not affected (Table 2). The negative effect of mycorrhiza on plant N mass prevailed in the presence of protists (0-PM, P-M), and was also reflected in root N concentrations (Figure S3, Table S2). Root N concentration in the mycorrhiza + protist chamber of the 0-PM treatment (0.60 mg g−1) was significantly reduced as compared to the protist chamber of the 0-P treatment [1.02 mg g−1; F(1, 23) = 41.3; p < 0.001; Figure S3]. This reduced both, total root N mass and total plant N mass by 56% in 0-P vs. 0-PM treatments (Table 2). In a similar way total root N mass was reduced in the P-M as compared to the 0-P treatment by 77%. These nitrogen reductions in roots led to 2.57 and 2.58-fold enhanced shoot-to-root N mass ratios in 0-PM and P-M treatments as compared to the control, respectively (Figure 2B, Table 2). Interestingly, the root N concentration of the protist + mycorrhiza chamber in the 0-PM treatment was also significantly reduced as compared to the mycorrhiza chamber of the 0-M treatment [from 0.75 to 0.60 mg g−1; F(1, 23) = 4.57; p = 0.043], suggesting N uptake by mycorrhiza in PM (Figure S3). Unexpectedly, total mass of root N in the P-M treatment was almost 2-fold reduced in the protist chamber (10.6 mg N) as compared to the mycorrhiza chamber [19.2 mg N; F(1, 8) = 5.57; p = 0.046; Figure 2B].
Root P mass in the 0-M treatment was reduced by 64% as compared to the control [0-0; F(1, 23) = 6.96; p = 0.015], resulting in a 55% reduction in total root P mass (Figure 3, Table 3), but a 3.7-fold increase in the shoot-to-root P mass ratio. Although root P mass was not affected in the 0-P treatment (Table 3), root P levels were diluted by an increased root biomass, with root P concentration in the protist chamber of the 0-P treatment being only 0.10 mg g−1 as compared to the control (0-0) with 0.14 mg g−1 [F(1, 23) = 4.73; p = 0.041; Figure S4].
Figure 3. Total P mass (mg) of shoots and of roots in both root chambers in control plants (0-0), plants treated with protists in the right root chamber (0-P), or treated with mycorrhiza in the right root chamber (0-M), or treated with protists and mycorrhiza together in the right root chamber (0-PM), or where the left and right root chambers were separately treated with protists and mycorrhiza (P-M). Means and standard deviation; horizontal lines denote significant contrasts with (*)p < 0.1, *p < 0.05.
Table 3. F- and P-values of GLM contrasts showing treatment effects on the total shoot P mass, total root P mass, total plant P mass, and shoot-to-root P mass of Triticum aestivum.
Root P mass in the protist chamber of the P-M treatment was reduced by 54% as compared to the 0-P treatment [Figure 3; F(1, 23) = 5.27; p = 0.031], but in the mycorrhiza chamber of the P-M treatment root P mass was more than 2.5-fold higher than in the 0-M treatment [F(1,23) = 5.04; p = 0.035]. Comparing both split-root chambers in the P-M treatment, root P mass almost doubled (x 1.91) in the mycorrhiza vs. the protist chamber [F(1, 8) = 5.61, p = 0.045], indicating compensation of P deficiency by mycorrhiza since total root P and total plant P mass were not significantly affected (Figure 3; Table 3; Table S3). Only in the 0-PM treatment total root P and whole plant P tended to be reduced by 37 and 16% in 0-PM vs. 0-P, respectively, although with marginal significance (Figure 3; Table 3). This was because root P mass in the mycorrhiza + protist chamber of the 0-PM treatment tended to be 42% lower as compared to the protist chamber in the 0-P treatment [Figure 3; F(1, 23) = 3.17; p = 0.088]. Thus, mycorrhiza appeared to reduce the P gain of plants in direct contact with protists (0-PM), but significantly shifted within-root system allocation of P to the mycorrhiza chamber in P-M.
The C:N ratio reflects a critical balance of carbohydrate gain (by photosynthesis) and/or investment (e.g., in root symbionts) relative to plant nutrient uptake. Mycorrhiza increased the root C:N ratio across treatments (Figure 2C). The root C:N ratio in the mycorrhiza chamber of the 0-M treatment (53) was significantly higher as compared to the control (0-0) at 38 [F(1, 23) = 5.16; p = 0.033]. Interestingly, the effect of mycorrhiza on root nutrient status was systemic, reflected in comparably high root C:N ratios of 61 and 53 in the bacteria only vs. mycorrhiza chambers of the 0-M treatment, respectively [F(1, 6) = 1,74, p = 0.24]. Accordingly, total plant C:N ratio was higher in the 0-M treatment (60) than in the control (46; Table 4). Thus, mycorrhiza strongly reduced root N levels relative to C, but the shoot C:N ratio remained constant.
Table 4. F- and P-values of GLM contrasts showing treatment effects on the C:N ratio of Triticum aestivum shoots, the total root system, and of whole plants.
Mycorrhiza effects also dominated the root C:N ratio in the presence of protists, reflected by root C:N ratios of 74 and 66 in the protist chambers of 0-PM and P-M as compared to the 0-P (37) treatment [Figure 2C; 0-P vs. 0-PM: F(1, 23) = 37.14; p < 0.001; 0-P vs. P-M: F(1, 23) = 23.40; p < 0.001]. At the whole plant level the C:N ratio increased from 46 in the 0-P treatment to 70 and 66 in the 0-PM and P-M treatments, respectively (Table 4).
The N sink effect of the combined treatments with mycorrhiza and protists was also enhanced in comparison to the 0-M treatment. The root C:N ratio was particularly enhanced in the protist + mycorrhiza chamber of the 0-PM treatment (74) as compared to the mycorrhiza chamber of the 0-M treatment [53; F(1, 23) = 10.41; p = 0.004], and also tended to be higher in the mycorrhiza chamber of the P-M treatment [65; F(1, 23) = 3.49; p = 0.075].
Microbial biomass in this experimental set up was almost exclusively bacterial biomass. Its measurements may allow to detect differences in biomass pools (e.g., by protist grazing), C release (basal respiration), and microbial C turnover (specific respiration). Microbial biomass in the protist chamber of the 0-P treatment tended to be reduced by 9% as compared to the control (0-0), although the effect was only marginally significant [F(1, 21) = 3.07; p = 0.09]. There were no treatment effects on microbial basal respiration and specific respiration (data not shown).
Steeper slopes of microbial growth after nutrient addition reflect limitation of microbes and of plant growth by this particular nutrient. Slopes of microbial growth after CN addition were 30-fold higher than slopes of microbial growth after CP addition [F(1, 91) = 692.6, p < 0.001], showing a strong N limitation of microbial growth in our experimental soil. Compared to the 0-P treatment, microbial growth increased by 34% after CN addition in the protist chamber of the 0-PM treatment [F(6, 22) = 5.16, p = 0.034], indicating a much stronger N-limitation in PM as compared to P. Also compared to the 0-M treatment, there was a tendency of increased growth after CN addition (+26%) in the protist + mycorrhiza chamber of the 0-PM treatment [F(6, 22) = 3.88, p = 0.062], suggesting an even stronger N-limitation in PM as compared to M. There were no treatment effects on microbial growth after CP addition (data not shown).
Since PLFA profiles reflect the composition of microbial membrane lipids they are highly sensitive to detect changes in microbial community composition. DFA clearly separated the PLFA profiles of the 0-0, 0-M, and 0-P treatments (Figure 4); mycorrhiza and in particular protists led to strong and contrasting shifts in microbial PLFA patterns as compared to the control. The first axis of the DFA represented 77.1% of the variation and correlated closely with short chain branched fatty acids typical for Gram positive bacteria (i15:0, a15:0, 15:0, i16:0; Table S1). It clearly separated the 0-P treatment from the 0-M and 0-0 treatments, with the PLFA patterns of the combined treatments with mycorrhiza and protists (0-PM and P-M) being more similar to the 0-P treatment. The second axis represented 12.2% of the variation and clearly separated 0-P and 0-M treatments from the control (0-0) and the combined treatments (0-PM, P-M). The second axis correlated with fatty acids typical for Gram negative bacteria (cy19:0; Table S1).
Figure 4. Separation of microbial communities according to PLFA groupings using a discriminant function analysis (DFA). Control plants (0-0, x), plants treated with protists (0-P treated chamber •, non-treated chamber ◦), with mycorrhiza (0-M treated chamber ♦, non-treated chamber ♢), with both protists and mycorrhiza together (0-PM, treated chamber ▴, non-treated chamber Δ), or where the left and right root chambers were separately treated with protists and mycorrhiza (P-M, protists □, mycorrhiza ■). Ellipses represent confidence ranges of 95%. Variation explained by axes 1 and 2 in parentheses.
Notably, as indicated by DFA, bacterial communities between different root chambers within the same treatment did not differ significantly except for the protist and mycorrhiza chambers of the P-M treatment (p < 0.05; Figure 4). The protist chamber contained fewer fatty acids typical for Gram positive bacteria than the chamber without protists. Specifically, these fatty acids were significantly reduced in the 0-P as compared to the control (0-0) treatment, and both combined treatments had significantly lower levels of these fatty acids than the MYC treatment (Figure 5).
Figure 5. Influence of treatments on the levels of fatty acids (% of total) typical of Gram positive bacteria (15:0i, 15:0a, and 16:0i) in the left (l) and right (r) root compartment, respectively. Treatments: control plants (0-0), plants treated with protists in the right root chamber (0-P), or treated with mycorrhiza in the right root chamber (0-M), or treated with protists and mycorrhiza together in the right root chamber (0-PM), or where the left and right root chambers were separately treated with protists and mycorrhiza (P-M). Mean and standard deviation; *p < 0.05, ***p < 0.001.
In the 15 root chambers treated with A. castelanii, the amoebae reached densities of 16,700 (0-P, protist treated chamber), 14,400 (0-PM, protist+mycorrhiza treated chamber) and 10,200 ind. g−1 dw soil (P-M, protist treated chamber; Figure S1). One replicate of the 0-M treatment was discarded from the statistical analyses as it was contaminated by protists.
Unfortunately, the determination of root colonization by mycorrhiza failed because the roots disintegrated after boiling in KOH. However, mycorrhiza in this experiment is a treatment factor and not treated as a continuous variable. The clear treatment effects by mycorrhiza on plant performance leave no doubt that mycorrhization was successful.
We hypothesized that both, mycorrhiza and protists, would show complementary functions for plant growth, comparable to findings from tripartite symbioses between rhizobia, mycorrhiza and their host plants (Kafle et al., 2018). As expected, mycorrhiza and protists, when inoculated separately, had opposite effects on plant N limitation with fundamental consequences for plant stoichiometry and shoot-to-root allometry. High shoot biomass relative to roots is an important plant trait in modern wheat cultivars (Siddique et al., 1990; Slafer and Araus, 2007). Conform to earlier studies (Veresoglou et al., 2012b) and our hypothesis, mycorrhiza increased plant shoot-to-root biomass ratio and shoot-to-root N mass ratio almost 3-fold, and shoot-to-root P mass ratio almost 4-fold. In part this was due to reducing root biomass (−38%), root N mass (−58%), and P mass (−55%), however, shoot biomass was not significantly affected by mycorrhiza. Split root experiments with barley already described R. irregularis as a strong C-sink for its plant hosts (Lerat et al., 2003). The strong reduction of root biomass, despite unchanged shoot biomass of wheat indicates that mycorrrhiza indeed partly replaced root system function as hypothesized. It seems that plants preferently invested in the shoot system even though root biomass and root nutrient levels were strongly reduced by mycorrhiza.
We further hypothesized that mycorrhiza would increase the P content of their plant hosts, but complemented by protist grazers of bacteria (Trap et al., 2016), we expected mycorrhiza to capture and transport N released by protist predation to the plant (Koller et al., 2013b; Trap et al., 2016). Mycorrhiza did not increase plant P uptake, but despite reduced root biomass, mycorrhiza infection kept whole plant P uptake constant. Interestingly, mycorrhiza caused a translocation of P from roots to shoots. This was confirmed by the lower N:P ratio of shoots, the strongly reduced mass of P in roots, but unaltered total mass of plant P in the 0-M treatment. It has been argued that replacement of root system function by mycorrhiza might be more economic to plants than investment into own roots (Fitter, 1991; Hodge, 2004). The reduction of root biomass indeed indicates a replacement of root system function by mycorrhiza, but at the same time mycorrhiza caused a severe reduction of total plant N (−32%). The reduced plant N levels show that a replacement of roots by mycorrhiza was not beneficial to the plants at N-limiting conditions. Rather, the results suggest that the plants partly lost control over root system function—a symbiont strategy to make itself indispensable according to microbial market theory (Werner et al., 2014). Likely the fungus diverted N for its own investment, e.g., into chitin polymers, for the establishment of an extraradical hyphal network (Herdler et al., 2008; Hodge and Fitter, 2010).
In contrast to AMF, protists are known to increase N availability to plants by releasing significant amounts of from consumed microbial biomass, a mechanism known as microbial loop (Clarholm, 1985; Bonkowski and Clarholm, 2012). Such changes in nitrogen form can feed back on mycorrhizal development (Giovannetti et al., 2017; Bukovská et al., 2018; Mollavali et al., 2018). Corresponding to the N-limiting experimental conditions, the increase in total plant N uptake (+22%) in the 0-P treatment corresponded to a 24% increased plant biomass as compared to the control (0-0). However, the protist effect on plant N nutrition was mainly due to a local increase in N contents in the protist chamber of the 0-P treatment (+52%). Further, contrasting the effect of mycorrhiza, protists did not change the shoot-to-root biomass ratio, suggesting that they stimulated above- and belowground growth in a balanced way.
In soils mycorrhiza and protists always co-occur and therefore their individual effect on plants is difficult to study. By manipulating them independently from each other, our split root design not only allowed deeper insight into their individual effect but also their interactions. The positive influence of AMF on plant growth primarily manifests as an improvement of P status against a background of P limitation (Nagy et al., 2009; Smith F. A. and Smith, 2011). Thus, from the plant's perspective, mycorrhiza, and protists seem complementary by respectively improving its P and N status. According to the functional equilibrium model (Mooney, 1972; Johnson et al., 2003), plants should allocate more biomass to those specific structures and rhizosphere symbionts that best garner the limiting resource (Revillini et al., 2016). Assuming that plants are under full control of the mycorrhiza symbiosis (Smith S. E. and Smith, 2011), plants would gain most benefit by investing in the protist rather than the mycorrhizal partner under N limiting conditions, as shown by increased plant biomass in the 0-P treatment. Recent studies indicated that R. irregularis is unable to compete for N mineralized by bacteria, but effectively captures N released from consumed microbial biomass by protist grazers (Koller et al., 2013a,b). Here we showed that AMF, although specialized for efficient P foraging, also gain control over N. It has been widely speculated why AMF hyphae proliferate in organic patches, despite being unable of a direct uptake of organic N (Hodge and Storer, 2014). The significantly stronger microbial N limitation in the mycorrhiza + protist chamber of the 0-PM treatment as compared to the protist chamber of the 0-P treatment indicates that mycorrhiza efficiently captured N mobilized by protists (Herdler et al., 2008; Koller et al., 2013b). This finding positions mycorrhiza as a critical player in the microbial loop (Bukovská et al., 2018).
However, plants did not benefit from the interaction between protists and mycorrhiza as indicated by the absence of plant growth promotion as compared to the 0-P treatment, and the widest C:N ratio of shoots and roots in the 0-PM as compared to all other treatments. Evidently the N released by protists was not delivered to the plant host, since plant N contents in the 0-PM and P-M treatments were as low as in the 0-M treatment. The mycorrhiza effects on plants clearly dominated in the combined treatments with mycorrhiza and protists (0-PM, P-M), suggesting that mycorrhiza, at least in part, took over control of the interactions—a crucial prerequisite for the equitable exchange of resources in mutualisms (Werner et al., 2014).
Remarkably, allocation of resources into roots considerably changed in the P-M treatment where protists and mycorrhiza were spatially separated. According to the 0-P and 0-M treatments one would expect a narrow C:N ratio and increased root growth in presence of protists, and a wide C:N ratio and reduced root growth in presence of mycorrhiza. Strikingly, the opposite pattern was found. Protists generally had a positive effect on root proliferation as observed here in the 0-P treatment and earlier (Bonkowski, 2004; Krome et al., 2010), but the AM fungus is a strong C sink and likely thereby root allocation was translocated to the mycorrhiza chamber in the P-M treatment. In addition, mycorrhiza altered N allocation within roots in a systemic way. Root system architecture is highly sensitive to different forms of nutrients, particularly N (Tian et al., 2014). Mycorrhiza generally reduced root N mass and root concentration of N, but in the 0-M treatment root N mass was also reduced in the non-mycorrhiza chamber of the split-root containers. This was reversed in the P-M treatment, where the doubling of N uptake in the mycorrhiza chamber as compared to the protist compartment suggests that N taken up by roots in the protist chamber was subsequently translocated to the mycorrhiza chamber. Taken together, the provisioning of roots by N through the microbial loop in the protist chamber was coupled with a systemic shift of N allocation to mycorrhiza in P-M. At the same time, also root proliferation shifted to the mycorrhiza chamber, potentially resulting from of a systemic protist effect on root growth. The combination of these effects caused the observed wide C:N ratio of roots in the protist chamber and the shift of root allocation within root systems in the P-M treatment. Importantly, the synergistic effects of protists and mycorrhiza in the P-M treatment could not have been predicted from the individual effects of protists and mycorrhiza in the 0-P and 0-M treatments. Rather, the interactions suggest that mycorrhiza as the root-infecting symbiont at least in part took over control of the tripartite interaction for diverting resources to its own benefit.
In recent years much has been learned how plants shape their root-associated soil and its inhabiting microorganisms (Berg and Smalla, 2009; Hartmann et al., 2009). It is assumed that soil type and rhizodeposits act as environmental filters for the enrichment of specific microorganisms (Bais et al., 2006; Bulgarelli et al., 2012; Schreiter et al., 2014), while host genotype–dependent fine tuning at the rhizoplane further selects for specific root-associated microbial consortia (Edwards et al., 2015; van der Heijden and Schlaeppi, 2015). Our results partly challenge this plant-centered view of community assembly, because the unique PLFA groupings in the 0-P and 0-M treatments as compared to the control (0-0) suggest fundamentally different effects of protist grazers and mycorrhiza on bacterial community assembly in the wheat rhizosphere. Also previous studies support this rhizosphere symbiont view of structuring rhizosphere microbial communities by documenting that both protists through top-down control (Kreuzer et al., 2006; Rosenberg et al., 2009) and AMF through bottom-up modifications of resources alter bacterial communities in the rhizosphere (Marschner and Baumann, 2003; Rillig et al., 2006; Bukovská et al., 2016; Rodríguez-Caballero et al., 2017). Since protist grazing is highly selective, preferential grazing together with competitive shifts in bacterial community composition are likely responsible for changes in root-associated bacterial communities (Jousset et al., 2008; Jousset, 2012; Flues et al., 2017). The shifts in PLFA pattern by mycorrhiza and protists support our general hypothesis on differences in bacterial community composition. However, the fact that the non-treated chambers of the split root containers displayed the same PLFA patterns as the treated chambers indicates that the effects of protists and mycorrhiza were communicated by the roots to the chamber not colonized by these symbionts. This supports the view that root symbionts exert systemic effects on rhizosphere community assembly (Marschner and Baumann, 2003; Marschner and Timonen, 2005; Veresoglou et al., 2012a) and that this also applies to the studied protists. Interestingly, microbial communities in the mycorrhiza chamber of the P-M and 0-M treatments were most different, indicating that in addition to mycorrhiza, protists may have altered microbial community assembly in the myco-rhizosphere in a systemic way (Bukovská et al., 2018). Implications of systemic root-responses to protists and mycorrhiza would be far reaching, indicating an adjustment of the root response via changing the quantity and quality of root exudates (Benizri et al., 2002, 2007; Jones et al., 2004). Such responses however, need to be confirmed in subsequent studies by gene expression studies of roots and their metabolites.
The strong effect of protists on the rhizosphere microbial community was unexpected considering the dominance of mycorrhiza on shoot-to-root allometry and plant nutrient uptake. The discovery that the genome of A. castellanii harbors genes for auxin biosynthesis as well as for free-auxin deactivation via formation of IAA conjugates led Clarke et al. (2013) to suggest that A. castellanii directly manipulates the root architecture of plants. Certainly, more research on how protist-root and protist-mycorrhiza interactions alter root allometry, and the assembly and functioning of the plant microbiome is needed. Split-root experiments as used in the present study provide an essential tool to uncover systemic root responses and their modulation by rhizosphere symbionts (Bonkowski, 2018).
Results of the present study provide compelling evidence for a tight functional coupling of the microbial loop and nutrient foraging by AMF. Mycorrhiza markedly altered plant shoot-to-root allometry by reducing root biomass of wheat, and it partly took over root system functioning. Notably, the shoot system responded little to alterations in the root system and rhizosphere community composition, indicating that the plants optimized shoot growth despite varying investment into roots. Protists increased plant growth and N uptake via the microbial loop, but this effect vanished in combined treatments with mycorrhiza, suggesting that mycorrhiza gained control over N provided by protist grazers. Combined effects of protists and mycorrhiza revealed systemic effects on within root system biomass allocation and root N allocation of winter wheat, and on rhizosphere microorganisms. Structuring rhizosphere communities by plants (roots) and root allocation thereby represent emergent properties that could not be predicted from individual treatments with mycorrhiza or protists alone. Notably, protists and mycorrhiza altered rhizosphere microbial communities in contrasting ways and these changes were systemically conveyed to the root side where the symbionts were lacking. The tight plant and microbial feed backs have far reaching implications for understanding the assembly of plant microbiomes, and testify central roles of both protists and mycorrhizas in the assembly process.
MB and SS conceived and designed the experiment. GH and MB designed the split root containers. GH performed the experiment and measurements after harvest, except the PLFA analyses that were carried out by SM and EK. Statistical analyses were performed by GH and MB drafted the manuscript. All co-authors contributed to the interpretation of the data and significantly improved the manuscript by critical revisions.
The authors declare that the research was conducted in the absence of any commercial or financial relationships that could be construed as a potential conflict of interest.
The authors thank Garvin Schulz for his help with the DFA analysis, Heather Hillers for revising an earlier version of the manuscript and two reviewers for their helpful comments. The study received funding from the European Union Marie Curie BIORHIZ Research Network.
The Supplementary Material for this article can be found online at: https://www.frontiersin.org/articles/10.3389/fenvs.2018.00117/full#supplementary-material
Figure S1. Numbers of amoebae in soil (ind. g−1 soil dry wt) of protist-inoculated root-chambers at the end of the experiment. No protists were detected in non-inoculated chambers, except in one replicate of the 0-M treatment, which was excluded from all analyses. Differences between treatments were not statistically significant.
Figure S2. Cumulative average requirements of water (g) of plants in split-root microcosms until 32 days after the set up of the experiment, measured by weighing the water supply bottles attached to each microcosm. Treatments: control plants (0-0), plants treated with protists in one root chamber (0-P), or treated with mycorrhiza in one root chamber (0-M), or treated with protists and mycorrhiza together in one root chamber (0-PM), or where the root chambers were separately treated with protists and mycorrhiza (P-M).
Figure S3. Concentration of N (%) in shoots and in roots of both root chambers in control plants (0-0), plants treated with protists in the right root chamber (0-P), or treated with mycorrhiza in the right root chamber (0-M), or treated with protists and mycorrhiza together in the right root chamber (0-PM), or where the left and right root chambers were separately treated with protists and mycorrhiza (P-M). Means and standard deviation; horizontal lines denote significant contrasts with (*)p < 0.1, *p < 0.05, ***p < 0.001.
Figure S4. Concentration of P (%) in shoots and in roots of both root chambers in control plants (0-0), plants treated with protists in the right root chamber (0-P), or treated with mycorrhiza in the right root chamber (0-M), or treated with protists and mycorrhiza together in the right root chamber (0-PM), or where the left and right root chambers were separately treated with protists and mycorrhiza (P-M). Means and standard deviation; horizontal lines denote significant contrasts with (*)p < 0.1, *p < 0.05.
Table S1. Correlation of single PLFAs with the major axes of the DFA (Figure 4). Significant correlations (p < 0.05) are shown in bold.
Table S2. F- and P-values of GLM contrasts showing treatment effects on N concentration (%) of shoots, N (%) of the treated root chambers inoculated with protists or/and mycorrhiza, N (%) of the whole root system, N (%) of the whole plants, and treatment effects on the total use of water of Triticum aestivum. Treatments: control plants (0-0), root halves treated with protists (0-P), with mycorrhiza (0-M), with protists and mycorrhiza together (0-PM), or where the left and right root compartments were separately treated with protists and mycorrhiza (P-M). Nominator and denominator degrees of freedom in brackets. Significant effects p < 0.05 in bold; p < 0.1 in italics.
Table S3. F- and P-values of GLM contrasts showing treatment effects on P concentration (%) of shoots, P (%) of the treated root chambers inoculated with protists or/and mycorrhiza, P (%) of the whole root system, P (%) of the whole Triticum aestivum plants. Treatments: control plants (0-0), root halves treated with protists (0-P), with mycorrhiza (0-M), with protists and mycorrhiza together (0-PM), or where the left and right root compartments were separately treated with protists and mycorrhiza (P-M). Nominator and denominator degrees of freedom in brackets. Significant effects p < 0.05 in bold; p < 0.1 in italics.
Agazzi, A., and Pirola, C. (2000). Fundamentals, methods and future trends of environmental microwave sample preparation. Microchem. J. 67, 337–341. doi: 10.1016/S0026-265X(00)00085-0
Anderson, J. P. E., and Domsch, K. H. (1978). A physiological method for the quantitative measurement of microbial biomass in soils. Soil Biol. Biochem. 10, 215–221. doi: 10.1016/0038-0717(78)90099-8
Anderson, J. P. E., and Domsch, K. H. (1980). Quantities of plant nutrients in the microbial biomass of selected soils. Soil Sci. 130, 211–216. doi: 10.1097/00010694-198010000-00008
Bais, H., Weir, T., Perry, L., Gilroy, S., and Vivanco, J. (2006). The role of root exudates in rhizosphere interactions with plants and other organisms. Annu. Rev. Plant Biol. 57, 233–266. doi: 10.1146/annurev.arplant.57.032905.105159
Beck, T., Joergensen, R. G., Kandeler, E., Makeschin, F., Nuss, E., Oberholzer, H. R., et al. (1997). An inter-laboratory comparison of ten different ways of measuring soil microbial biomass C. Soil Biol. Biochem. 29, 1023–1032. doi: 10.1016/S0038-0717(97)00030-8
Benizri, E., Dedourge, O., Dibattista-Leboeuf, C., Piutti, S., Nguyen, C., and Guckert, A. (2002). Effect of maize rhizodeposits on soil microbial community structure. Appl. Soil Ecol. 21, 261–265. doi: 10.1016/S0929-1393(02)00094-X
Benizri, E., Nguyen, C., Piutti, S., Slezack-Deschaumes, S., and Philippot, L. (2007). Additions of maize root mucilage to soil changed the structure of the bacterial community. Soil Biol. Biochem. 39, 1230–1233. doi: 10.1016/j.soilbio.2006.12.026
Berg, G., and Smalla, K. (2009). Plant species and soil type cooperatively shape the structure and function of microbial communities in the rhizosphere. FEMS Microbiol. Ecol. 68, 1–13. doi: 10.1111/j.1574-6941.2009.00654.x
Bloom, A. J., Chapin, F. S. III., and Mooney, H. A (1985). Resource limitation in plants-an economic analogy. Annu. Rev. Ecol. Syst. 16, 363–392. doi: 10.1146/annurev.es.16.110185.002051
Bonfante, P., and Genre, A. (2010). Mechanisms underlying beneficial plant-fungus interactions in mycorrhizal symbiosis. Nat. Commun. 1:48. doi: 10.1038/ncomms1046
Bonkowski, M. (2004). Protozoa and plant growth: the microbial loop in soil revisited. N. Phytol. 162, 617–631. doi: 10.1111/j.1469-8137.2004.01066.x
Bonkowski, M. (2018). “Microcosm approaches to investigate multitrophic interactions between bacteria, protists, symbiotic fungi and their host plants,” in Methods in Rhizosphere Biology Research, eds D. Reinhard and A. K. Sharma (Singapore: Springer), 80–100.
Bonkowski, M., and Brandt, F. (2002). Do soil protozoa enhance plant growth by hormonal effects? Soil Biol. Biochem. 34, 1709–1715. doi: 10.1016/S0038-0717(02)00157-8
Bonkowski, M., and Clarholm, M. (2012). Stimulation of plant growth through interactions of bacteria and protozoa: testing the auxillary microbial loop hypothesis. Acta Protozool. 51, 237–247. doi: 10.4467/16890027AP.12.019.0765
Bukovská, P., Bonkowski, M., Konvalinková, T., Beskid, O., Hujslová, M., Püschel, D., et al. (2018). Utilization of organic nitrogen by arbuscular mycorrhizal fungi—is there a specific role for protists and ammonia oxidizers? Mycorrhiza 28, 269–283. doi: 10.1007/s00572-018-0825-0
Bukovská, P., Gryndler, M., Gryndlerová, H., Püschel, D., and Jansa, J. (2016). Organic nitrogen-driven stimulation of arbuscular mycorrhizal fungal hyphae correlates with abundance of ammonia oxidizers. Front. Microbiol. 7:711. doi: 10.3389/fmicb.2016.00711
Bulgarelli, D., Rott, M., Schlaeppi, K., Ver Loren van Themaat, E., Ahmadinejad, N., Assenza, F., et al. (2012). Revealing structure and assembly cues for Arabidopsis root-inhabiting bacterial microbiota. Nature 488, 91–95. doi: 10.1038/nature11336
Clarholm, M. (1985). Interactions of bacteria, protozoa and plants leading to mineralization of soil nitrogen. Soil Biol. Biochem. 17, 181–187. doi: 10.1016/0038-0717(85)90113-0
Clarke, M., Lohan, A., Liu, B., Lagkouvardos, I., Roy, S., Zafar, N., et al. (2013). Genome of Acanthamoeba castellanii highlights extensive lateral gene transfer and early evolution of tyrosine kinase signaling. Genome Biol. 14:R11. doi: 10.1186/gb-2013-14-2-r11
Darbyshire, J. F., Wheatley, R. E., Greaves, M. P., and Inkson, R. H. E. (1974). A rapid micromethod for estimating bacterial and protozoan populations in soil. Reviev d'Ecologie et Biologie du Sol 11, 465–475.
Edwards, J., Johnson, C., Santos-Medellin, C., Lurie, E., Podishetty, N. K., Bhatnagar, S., et al. (2015). Structure, variation, and assembly of the root-associated microbiomes of rice. Proc. Natl. Acad. Sci. U.S.A. 112, 911–920. doi: 10.1073/pnas.1414592112
Ericsson, T. (1995). Growth and shoot-root ratio of seedlings in relation to nutrient availability. Plant Soil 168, 205–214. doi: 10.1007/BF00029330
Fiore-Donno, A. M., Weinert, J., Wubet, T., and Bonkowski, M. (2016). Metacommunity analysis of amoeboid protists in grassland soils. Sci. Rep. 6:19068. doi: 10.1038/srep19068
Fitter, A. (1991). Costs and benefits of mycorrhizas: implications for functioning under natural conditions. Cell. Molecul. Life Sci. 47, 350–355. doi: 10.1007/BF01972076
Flues, S., Bass, D., and Bonkowski, M. (2017). Grazing of Cercomonads (Protists: Rhizaria: Cercozoa) structures bacterial phyllosphere communities and function. Environ. Microbiol. 19, 3297–3309. doi: 10.1111/1462-2920.13824
Friesen, M. L., Porter, S. S., Stark, S. C., von Wettberg, E. J., Sachs, J. L., and Martinez-Romero, E. (2011). Microbially mediated plant functional traits. Annu. Rev. Ecol. Evol. Syst. 42, 23–46. doi: 10.1146/annurev-ecolsys-102710-145039
Frostegård, Å., and Bååth, E. (1996). The use of phospholipid fatty acid analysis to estimate bacterial and fungal biomass in soil. Biol. Fertil. Soils 22, 59–65. doi: 10.1007/BF00384433
Frostegård, Å., Tunlid, A., and Bååth, E. (1991). Microbial biomass measured as total lipid phosphate in soils of different organic content. J. Microbiol. Methods 14, 151–163. doi: 10.1016/0167-7012(91)90018-L
Gange, A., and Ayres, R. (1999). On the relation between arbuscular mycorrhizal colonization and plant ‘benefit’. Oikos 87, 615–621. doi: 10.2307/3546829
Giovannetti, M., Volpe, V., Salvioli, A., and Bonfante, P. (2017). “Fungal and plant tools for the uptake of nutrients in arbuscular mycorrhizas: a molecular view,” in Mycorrhizal Mediation of Soil, eds N. C. Johnson, C. Gehring, and J. Jansa (Amsterdam: Elsevier), 107–128.
Graham, J. H., Leonard, R. T., and Menge, J. A. (1981). Membrane-mediated decrease in root exudation responsible for phorphorus inhibition of vesicular-arbuscular mycorrhiza formation. Plant Physiol. 68, 548–552. doi: 10.1104/pp.68.3.548
Griffiths, B. S., Bonkowski, M., Roy, J., and Ritz, K. (2001). Functional stability, substrate utilisation and biological indicators of soils following environmental impacts. Appl. Soil Ecol. 16, 49–61. doi: 10.1016/S0929-1393(00)00081-0
Hartmann, A., Schmid, M., van Tuinen, D., and Berg, G. (2009). Plant-driven selection of microbes. Plant Soil 321, 235–257. doi: 10.1007/s11104-008-9814-y
Hensel, M., Bieleit, G., Meyer, R., and Jagnow, G. (1990). A reliable method for the selection of axenic seedlings. Biol. Fertil. Soils 9, 281–282. doi: 10.1007/BF00336240
Herdler, S., Kreuzer, K., Scheu, S., and Bonkowski, M. (2008). Interactions between arbuscular mycorrhizal fungi (Glomus intraradices, Glomeromycota) and amoebae (Acanthamoeba castellanii, Protozoa) in the rhizosphere of rice (Oryza sativa). Soil Biol. Biochem. 40, 660–668. doi: 10.1016/j.soilbio.2007.09.026
Herman, D. J., Firestone, M. K., Nuccio, E., and Hodge, A. (2012). Interactions between an arbuscular mycorrhizal fungus and a soil microbial community mediating litter decomposition. FEMS Microbiol. Ecol. 80, 236–247. doi: 10.1111/j.1574-6941.2011.01292.x
Hoad, S., Russell, G., Lucas, M., and Bingham, I. (2001). The management of wheat, barley, and oat root systems. Adv. Agron. 74, 193–246. doi: 10.1016/S0065-2113(01)74034-5
Hodge, A. (2004). The plastic plant: root responses to heterogeneous supplies of nutrients. N. Phytol. 162, 9–24. doi: 10.1111/j.1469-8137.2004.01015.x
Hodge, A., Campbell, C., and Fitter, A. (2001). An arbuscular mycorrhizal fungus accelerates decomposition and acquires nitrogen directly from organic material. Nature 413, 297–299. doi: 10.1038/35095041
Hodge, A., and Fitter, A. H. (2010). Substantial nitrogen acquisition by arbuscular mycorrhizal fungi from organic material has implications for N cycling. Proc. Natl. Acad. Sci. U.S.A. 107, 13754–13759. doi: 10.1073/pnas.1005874107
Hodge, A., and Storer, K. (2014). Arbuscular mycorrhiza and nitrogen: implications for individual plants through to ecosystems. Plant Soil 386, 1–19. doi: 10.1007/s11104-014-2162-1
Johnson, N. C. (2010). Resource stoichiometry elucidates the structure and function of arbuscular mycorrhizas across scales. N. Phytol. 185, 631–647. doi: 10.1111/j.1469-8137.2009.03110.x
Johnson, N. C., Rowland, D. L., Corkidi, L., Egerton-Warburton, L. M., and Allen, E. B. (2003). Nitrogen enrichment alters mycorrhizal allocation at five mesic to semiarid grasslands. Ecology 84, 1895–1908. doi: 10.1890/0012-9658(2003)084[1895:NEAMAA]2.0.CO;2
Jones, D., Hodge, A., and Kuzyakov, Y. (2004). Plant and mycorrhizal regulation of rhizodeposition. N. Phytol. 163, 459–480. doi: 10.1111/j.1469-8137.2004.01130.x
Jousset, A. (2012). Ecological and evolutive implications of bacterial defences against predators. Environ. Microbiol. 14, 1830–1843. doi: 10.1111/j.1462-2920.2011.02627.x
Jousset, A., Rochat, L., Péchy-Tarr, M., Keel, C., Scheu, S., and Bonkowski, M. (2009). Predators promote defence of rhizosphere bacterial populations by selective feeding on non-toxic cheaters. ISME J. 3, 666–674. doi: 10.1038/ismej.2009.26
Jousset, A., Scheu, S., and Bonkowski, M. (2008). Secondary metabolite production facilitates establishment of rhizobacteria by reducing both protozoan predation and the competitive effects of indigenous bacteria. Funct. Ecol. 22, 714–719. doi: 10.1111/j.1365-2435.2008.01411.x
Kafle, A., Garcia, K., Wang, X., Pfeffer, P. E., Strahan, G. D., and Bücking, H. (2018). Nutrient demand and fungal access to resources control the carbon allocation to the symbiotic partners in tripartite interactions of Medicago truncatula. Plant Cell Environ. doi: 10.1111/pce.13359. [Epub ahead of print].
Klironomos, J. N. (2003). Variation in plant response to native and exotic arbuscular mycorrhizal fungi. Ecology 84, 2292–2301. doi: 10.1890/02-0413
Koller, R., Rodriguez, A., Robin, C., Scheu, S., and Bonkowski, M. (2013a). Protozoa enhance foraging efficiency of arbuscular mycorrhizal fungi for mineral nitrogen from organic matter in soil to the benefit of host plants. N. Phytol. 199, 203–211. doi: 10.1111/nph.12249
Koller, R., Scheu, S., Bonkowski, M., and Robin, C. (2013b). Protozoa stimulate N uptake and growth of arbuscular mycorrhizal plants. Soil Biol. Biochem. 65, 204–210. doi: 10.1016/j.soilbio.2013.05.020
Kramer, S., Marhan, S., Haslwimmer, H., Ruess, L., and Kandeler, E. (2013). Temporal variation in surface and subsoil abundance and function of the soil microbial community in an arable soil. Soil Biol. Biochem. 61, 76–85. doi: 10.1016/j.soilbio.2013.02.006
Kreuzer, K., Adamczyk, J., Iijima, M., Wagner, M., Scheu, S., and Bonkowski, M. (2006). Grazing of a common species of soil protozoa (Acanthamoeba castellanii) affects rhizosphere bacterial community composition and root architecture of rice (Oryza sativa L.). Soil Biol. Biochem. 38, 1665–1672. doi: 10.1016/j.soilbio.2005.11.027
Krome, K., Rosenberg, K., Bonkowski, M., and Scheu, S. (2009). Grazing of protozoa on rhizosphere bacteria alters growth and reproduction of Arabidopsis thaliana. Soil Biol. Biochem. 41, 1866–1873. doi: 10.1016/j.soilbio.2009.06.008
Krome, K., Rosenberg, K., Dickler, C., Kreuzer, K., Ludwig-Müller, J., Ullrich-Eberius, C., et al. (2010). Soil bacteria and protozoa affect root branching via effects on the auxin and cytokinin balance in plants. Plant Soil 328, 191–201. doi: 10.1007/s11104-009-0101-3
Kuikman, P. J., Jansen, A. G., and van Veen, J. A. (1991). 15N-nitrogen mineralization from bacteria by protozoan grazing at different soil moisture regimes. Soil Biol. Biochem. 23, 193–200. doi: 10.1016/0038-0717(91)90134-6
Lau, J. A., and Lennon, J. T. (2012). Rapid responses of soil microorganisms improve plant fitness in novel environments. Proc. Natl. Acad. Sci. U.S.A. 109, 14058–14062. doi: 10.1073/pnas.1202319109
Leigh, J., Fitter, A. H., and Hodge, A. (2011). Growth and symbiotic effectiveness of an arbuscular mycorrhizal fungus in organic matter in competition with soil bacteria. FEMS Microbiol. Ecol. 76, 428–438. doi: 10.1111/j.1574-6941.2011.01066.x
Lerat, S., Lapointe, L., Gutjahr, S. Y. P, and Vierheilig, H. (2003). Carbon partitioning in a split-root system of arbuscular mycorrhizal plants is fungal and plant species dependent. N. Phytol. 157, 589–595. doi: 10.1046/j.1469-8137.2003.00691.x
López-Bucio, J., Cruz-Ramírez, A., and Herrera-Estrella, L. (2003). The role of nutrient availability in regulating root architecture. Curr. Opin. Plant Biol. 6, 280–287. doi: 10.1016/S1369-5266(03)00035-9
Marschner, P., and Baumann, K. (2003). Changes in bacterial community structure induced by mycorrhizal colonisation in split-root maize. Plant Soil 251, 279–289. doi: 10.1023/A:1023034825871
Marschner, P., and Timonen, S. (2005). Interactions between plant species and mycorrhizal colonization on the bacterial community composition in the rhizosphere. Appl. Soil Ecol. 28, 23–36. doi: 10.1016/j.apsoil.2004.06.007
Mollavali, M., Perner, H., Rohn, S., Riehle, P., Hanschen, F. S., and Schwarz, D. (2018). Nitrogen form and mycorrhizal inoculation amount and timing affect flavonol biosynthesis in onion (Allium cepa L.). Mycorrhiza 28, 59–70. doi: 10.1007/s00572-017-0799-3
Mooney, H. A. (1972). The carbon balance of plants. Annu. Rev. Ecol. Syst. 3, 315–346. doi: 10.1146/annurev.es.03.110172.001531
Nagy, R., Drissner, D., Amrhein, N., Jakobsen, I., and Bucher, M. (2009). Mycorrhizal phosphate uptake pathway in tomato is phosphorus-repressible and transcriptionally regulated. N. Phytol. 181, 950–959. doi: 10.1111/j.1469-8137.2008.02721.x
Ngosong, C., Gabriel, E., and Ruess, L. (2012). Use of the signature fatty acid 16:1ω5 as a tool to determine the distribution of arbuscular mycorrhizal fungi in soil. J. Lipids 2012:236807. doi: 10.1155/2012/236807
Nuccio, E. E., Hodge, A., Pett-Ridge, J., Herman, D. J., Weber, P. K., and Firestone, M. K. (2013). An arbuscular mycorrhizal fungus significantly modifies the soil bacterial community and nitrogen cycling during litter decomposition. Environ. Microbiol. 15, 1870–1881. doi: 10.1111/1462-2920.12081
Olsson, P. A., Rahm, J., and Aliasgharzad, N. (2010). Carbon dynamics in mycorrhizal symbioses is linked to carbon costs and phosphorus benefits. FEMS Microbiol. Ecol. 72, 123–131. doi: 10.1111/j.1574-6941.2009.00833.x
Page, F. C. (1976). An Illustrated Key to Freshwater and Soil Amoebae. Vol. 34. Ambleside: Freshwater Biological Association, 11.
Revillini, D., Gehring, C. A., Johnson, N. C., and Bailey, J. (2016). The role of locally adapted mycorrhizas and rhizobacteria in plant-soil feedback systems. Funct. Ecol. 30, 1086–1098. doi: 10.1111/1365-2435.12668
Rillig, M. C., Mummey, D. L., Ramsey, P. W., Klironomos, J. N., and Gannon, J. E. (2006). Phylogeny of arbuscular mycorrhizal fungi predicts community composition of symbiosis-associated bacteria. FEMS Microbiol. Ecol. 57, 389–395. doi: 10.1111/j.1574-6941.2006.00129.x
Rodríguez-Caballero, G., Caravaca, F., Fernández-González, A. J., Alguacil, M. M., Fernández-López, M., and Roldán, A. (2017). Arbuscular mycorrhizal fungi inoculation mediated changes in rhizosphere bacterial community structure while promoting revegetation in a semiarid ecosystem. Sci. Total Environ. 584–585, 838–848. doi: 10.1016/j.scitotenv.2017.01.128
Rosenberg, K., Bertaux, J., Krome, K., Hartmann, A., Scheu, S., and Bonkowski, M. (2009). Soil amoebae rapidly change bacterial community composition in the rhizosphere of Arabidopsis thaliana. ISME J. 3, 675–684. doi: 10.1038/ismej.2009.11
Ruess, L., and Chamberlain, P. M. (2010). The fat that matters: soil food web analysis using fatty acids and their carbon stable isotope signature. Soil Biol. Biochem. 42, 1898–1910. doi: 10.1016/j.soilbio.2010.07.020
Ryan, M. H., Tibbett, M., Edmonds-Tibbett, T., Suriyagoda, L. D., Lambers, H., Cawthray, G. R., et al. (2012). Carbon trading for phosphorus gain: the balance between rhizosphere carboxylates and arbuscular mycorrhizal symbiosis in plant phosphorus acquisition. Plant Cell Environ. 35, 2170–2180. doi: 10.1111/j.1365-3040.2012.02547.x
Sanders, F. E., and Tinker, P. B. (1971). Mechanism of absorption of phosphate from soil by Endogone mycorrhizas. Nature 233, 278–279. doi: 10.1038/233278c0
Scheu, S. (1992). Automated measurement of the respiratory response of soil microcompartments: active microbial biomass in earthworm faeces. Soil Biol. Biochem. 24, 1113–1118. doi: 10.1016/0038-0717(92)90061-2
Scheublin, T. R., Sanders, I. R., Keel, C., and van der Meer, J. R. (2010). Characterisation of microbial communities colonising the hyphal surfaces of arbuscular mycorrhizal fungi. ISME J. 4, 752–763. doi: 10.1038/ismej.2010.5
Schreiter, S., Sandmann, M., Smalla, K., and Grosch, R. (2014). Soil type dependent rhizosphere competence and biocontrol of two bacterial inoculant strains and their effects on the rhizosphere microbial community of field-grown lettuce. PLoS ONE 9:e103726. doi: 10.1371/2Fjournal.pone.0103726
Siddique, K., Belford, R., and Tennant, D. (1990). Root: shoot ratios of old and modern, tall and semi-dwarf wheats in a Mediterranean environment. Plant Soil 121, 89–98. doi: 10.1007/BF00013101
Slafer, G., and Araus, J. (2007). “Physiological traits for improving wheat yield under a wide range of conditions,” in Scale and Complexity in Plant Systems Research: Gene-Plant-Crop Relations, eds J. H. J. Spiertz, P. C. Struik, H. H. van Laar (Heidelberg: Springer), 145–154.
Smith, F. A., and Smith, S. E. (2011). What is the significance of the arbuscular mycorrhizal colonisation of many economically important crop plants? Plant Soil 348, 63–79. doi: 10.1007/s11104-011-0865-0
Smith, S. E., and Read, D. (2008). The Symbionts Forming Arbuscular Mycorrhizas. Mycorrhizal Symbiosis 3rd Edn. London: Academic Press, 13–41.
Smith, S. E., and Smith, F. A. (2011). Roles of arbuscular mycorrhizas in plant nutrition and growth: new paradigms from cellular to ecosystem scales. Annu. Rev. Plant Biol. 62, 227–250. doi: 10.1146/annurev-arplant-042110-103846
Stockinger, H., Walker, C., and Schüßler, A. (2009). 'Glomus intraradices DAOM197198', a model fungus in arbuscular mycorrhiza research, is not Glomus intraradices. N. Phytol. 183, 1176–1187. doi: 10.1111/j.1469-8137.2009.02874.x
Tian, H., De Smet, I., and Ding, Z. (2014). Shaping a root system: regulating lateral versus primary root growth. Trends Plant Sci. 19, 426–431. doi: 10.1016/j.tplants.2014.01.007
Trap, J., Bonkowski, M., Plassard, C., Villenave, C., and Blanchart, E. (2016). Ecological importance of soil bacterivores for ecosystem functions. Plant Soil 398, 1–24. doi: 10.1007/s11104-015-2671-6
van der Heijden, M. G., and Schlaeppi, K. (2015). Root surface as a frontier for plant microbiome research. Proc. Natl. Acad. Sci. U.S.A. 112, 2299–2300. doi: 10.1073/pnas.1500709112
Van Noordwijk, M., and De Willigen, P. (1987). Agricultural concepts of roots: from morphogenetic to functional equilibrium between root and shoot growth. Neth. J. Agricul. Sci. 35, 487–496.
Venables, W. N., and Ripley, B. D. (2002). Modern Applied Statistics with S. New York, NY: Springer. doi: 10.1007/978-0-387-21706-2
Veresoglou, S. D., Chen, B., and Rillig, M. C. (2012a). Arbuscular mycorrhiza and soil nitrogen cycling. Soil Biol. Biochem. 46, 53–62. doi: 10.1016/j.soilbio.2011.11.018
Veresoglou, S. D., Menexes, G., and Rillig, M. C. (2012b). Do arbuscular mycorrhizal fungi affect the allometric partition of host plant biomass to shoots and roots? A meta-analysis of studies from 1990 to 2010. Mycorrhiza 22, 227–235. doi: 10.1007/s00572-011-0398-7
Wasson, A. P., Richards, R. A., Chatrath, R., Misra, S. C., Prasad, S. V., Rebetzke, G. J., et al. (2012). Traits and selection strategies to improve root systems and water uptake in water-limited wheat crops. J. Exp. Bot. 63, 3485–3498. doi: 10.1093/jxb/ers111
Werner, G. D., Strassmann, J. E., Ivens, A. B., Engelmoer, D. J., Verbruggen, E., Queller, D. C., et al. (2014). Evolution of microbial markets. Proc. Natl. Acad. Sci. U.S.A. 111, 1237–1244. doi: 10.1073/pnas.1315980111
Keywords: arbuscular mycorrhiza, wheat, nitrogen, amoebae, protists, microbial loop, shoot-to-root allocation, spit-root experiment
Citation: Henkes GJ, Kandeler E, Marhan S, Scheu S and Bonkowski M (2018) Interactions of Mycorrhiza and Protists in the Rhizosphere Systemically Alter Microbial Community Composition, Plant Shoot-to-Root Ratio and Within-Root System Nitrogen Allocation. Front. Environ. Sci. 6:117. doi: 10.3389/fenvs.2018.00117
Received: 31 May 2018; Accepted: 20 September 2018;
Published: 16 October 2018.
Edited by:
Caroline Gutjahr, Technische Universität München, GermanyReviewed by:
Karin E. Groten, Max-Planck-Institut für chemische Ökologie, GermanyCopyright © 2018 Henkes, Kandeler, Marhan, Scheu and Bonkowski. This is an open-access article distributed under the terms of the Creative Commons Attribution License (CC BY). The use, distribution or reproduction in other forums is permitted, provided the original author(s) and the copyright owner(s) are credited and that the original publication in this journal is cited, in accordance with accepted academic practice. No use, distribution or reproduction is permitted which does not comply with these terms.
*Correspondence: Michael Bonkowski, bS5ib25rb3dza2lAdW5pLWtvZWxuLmRl
Disclaimer: All claims expressed in this article are solely those of the authors and do not necessarily represent those of their affiliated organizations, or those of the publisher, the editors and the reviewers. Any product that may be evaluated in this article or claim that may be made by its manufacturer is not guaranteed or endorsed by the publisher.
Research integrity at Frontiers
Learn more about the work of our research integrity team to safeguard the quality of each article we publish.