- Department of Agriculture and Natural Resources, Delaware State University, Dover, DE, United States
Blackbird Creek is the waterway that empties into the Delaware Bay. The lower 21 km of the creek has been shown to have appreciable salinity measurements, suggesting that this portion is influenced by tidal fluctuations. Fourteen sampling stations were established within this lateral range in order to examine the nutrient dynamics of the creek at various points in time. Our objective was to monitor potential changes in water quality conditions, especially on nutrients, in the creek during the day using the low and high tides as the predominating driver for the change. Temperature, pH, dissolved oxygen, conductivity, and salinity were monitored bi-weekly at each station. Concentrations of dissolved nitrate (NO3), nitrite (NO2), ammonia (NH3), orthophosphate (PO4), alkalinity (Alk), and turbidity (Tbd) were measured at each station over the course of the field season. Average concentrations were generally low for the nitrogen species: NH4 = 0.11 mgL−1, NO3 = 0.30 mgL−1, NO2 = 0.02 mgL−1. Average alkalinity (92 mgL−1 CaCO3) and turbidity (71 FTU) concentrations were appropriate given the nature of the marsh environment. The average PO4 concentration, however, was elevated (= 0.44 mgL−1). The EPA recommends values under 0.1 mgL−1 for this type of waterway. When considered separately, nutrient concentrations on outgoing tides were elevated relative to nutrient concentrations on incoming tides. Overall, the highest concentrations for all parameters occurred at low tide before the shift to the next incoming tide. This suggests that there are greater nutrient concentrations upstream than downstream. Given that land use in the Blackbird Creek watershed is primarily agricultural, it is likely that upstream pore water input from cropland is influencing the nutrient dynamics of the waterway. This information is key to understanding the efficiency of the riparian buffer system that has been established in the watershed and to allow for opportunities for improvement to mitigate nutrient runoff from agricultural fields.
Introduction
Water resources throughout the Mid-Atlantic coastline of the United States are facing constant and increasing threats from growing human population (Postel et al., 1996; Pimentel et al., 2004). Water resource management needs to be a priority as climate changes, sea level rises, and land use is modified to accommodate increase population. Land use, particularly, is an immediate concern because a change in the way land transformed has direct implications on water quality and quantity and aquatic habitat suitability (Forney et al., 2001; Allan, 2004). The change from a native ecosystem to an agricultural landscape has far-reaching implications, including increased soil erosion, fertilizer runoff, removal of critical wildlife habitat, and loss of ecosystem services which help mitigate the effects of climate change (i.e., carbon sequestration; Schempf and Cox, 2007). If, for example, forested wetlands are drained and used instead for agricultural practices to feed an ever-increasing population, the wetland habitat available for use by aquatic biota is reduced due to altered hydrology, affecting the quality of the water directly. Marsh grasses and forested buffers have been shown to decrease nutrient and pollutant runoff into waterways (Bingham et al., 1980; Castelle et al., 1994; Spruill, 2000). If these buffers are removed, there is a direct line of application of such pollutants from surface water and submarine groundwater discharge into streams and estuaries (Bingham et al., 1980).
Blackbird Creek is a small watershed in north-central Delaware that has a measurable concentration of agricultural land practice throughout The Blackbird Creek watershed in New Castle County, Delaware is a model catchment to study the utility of natural riparian buffers on water quality because of a significant concentration of agricultural land practice throughout the creek. The basin drains about 80.3 square kilometers of north-central Delaware into the Delaware Bay. The main waterway is about 44 km long with an additional 2.5 km of tributaries (DNREC, 2006). Approximately, 36% of the watershed is designated as use for agriculture and an additional 13% as urban use. There are no known point sources of pollution throughout the watershed (DNREC, 2006). Therefore, excess nutrient concentrations are either autochthonous or from human-induced runoff.
Blackbird Creek is a tidal system for the lower 21 km of the waterway with bio-directional flow, making it an extremely important ecosystem for many ecologically and economically important species. As a nursery, Blackbird Creek is home to several fish species especially in their juvenile stages, such as weakfish (Cynoscion regalis), channel catfish (Ictalurus punctatus), black drum (Pogonias cromis), white catfish (Ameiurus catus), Atlantic menhaden, alewife (Alosa pseudoharengus), American eel (Anguilla rostrata), white perch (Morone americana), striped bass (Morone saxatilis), and many others. It is also home to the blue crab (Callinectes sapidus).
Of particular importance is habitat change of the ecosystem due to anthropogenic impacts. Land use changes from native ecosystems to farmland can create temperature extremes due to lack of shading along the waterway (Blann et al., 2001), adjust salinity due to altered hydrology (Jayawickreme et al., 2011), and increase nutrient concentrations if a rain event occurs following fertilization of the crop fields in the watersheds (Johnson et al., 1997). Blue crabs, particularly in their post-larval stages, are sensitive to changes in temperature and salinity (Mistiaen et al., 2003; Mazzotti et al., 2006). Costlow (1967) found that the most pronounced post-larval growth was at 30°C in salinities of 10 parts per thousand (ppt) to 40 ppt and that salinity >30 ppt is optimal for growth. He also concluded that water temperatures ranging from 21.5 to 34.5°C are suitable for survival and growth, and that 25°C is optimal (Costlow, 1967).
A pervasive issue with regard to human impact is eutrophication—the introduction of large amounts of nutrients into ecosystems where blue crabs and other nekton exist. In such a situation, as evidenced in the Gulf of Mexico (Kling et al., 2014) and the Chesapeake Bay (Boesch et al., 2001), the nutrient concentrations can become high enough to force blooms of phytoplankton throughout the ecosystem. After some period, these algae blooms die off. When this happens, these plants are no longer producing oxygen as a byproduct of photosynthesis, but rather consuming it via decomposition. This causes a hypoxia episode—the reduction in the dissolved oxygen concentration of the ecosystem (Diaz and Rosenberg, 2008), and could lead to death of sessile organisms and movement of motile organisms from the area.
Blackbird Creek watershed has great potential for eutrophic conditions, with up to 44% of the basic comprised of agriculture land cover (Pomilio, 2015). These lands are fertilized frequently to accommodate the timely growth of crops (Lu et al., 2012). This is a concern for local nekton of commercial and ecological importance. Eutrophication is a common problem for wetlands adjacent to agricultural lands, partially because the nutrient loading tends to favor the growth of aggressive, invasive, often weedy, plant species that displace native wetland plants. Drexler and Bedford (2002), for example, showed that inflow of nutrient rich water into a New York wetland led to growth of monotypic stands of native bluejoint (Calamagrostis canadensis) and broadleaf cattail (Typhalatifolia) in an otherwise diverse ecosystem. In extreme cases, such as the Chesapeake Bay, nitrogen and phosphorous loading causes algae growth so great that the blooms block out sunlight to submerged aquatic vegetation, a crucial habitat for larval and juvenile fish and crabs (Boesch et al., 2001).
Substantial nitrogen is placed on croplands in order to maximize growth and productivity throughout the growing season throughout the Delmarva Peninsula (Bachman et al., 1994). A small, but significant fraction of the total nitrogen used for agriculture is in excess of the plant requirements for appropriate growth. This extra nitrogen can accumulate in the soils, runoff into the waterway, migrate through the soil into groundwater channels, or enter the atmosphere by way of ammonia volatilization or nitrous oxide production. All of these issues are typical in a marsh ecosystem such as Blackbird Creek. Of particular concern is the runoff into the waterway.
Previous studies have shown the detrimental effects of excessive nutrient input, as evidenced by work in the Gulf of Mexico (Rabalais et al., 1996) and the Chesapeake Bay (Boesch et al., 2001) because the land within these watersheds serve as alluvial plains for runoff into the waterway which, in turn, serves as a conduit to a centralized location where the nutrients can accumulate and become a detriment to the system (Carpenter et al., 1998; Valiela et al., 2000; Paul and Meyer, 2001). We hypothesized in this study that due to high levels of agricultural use (Figure 1) within the watershed, there are probably high levels of nutrient runoff from cropland. Because of the bi-directional nature of the waterway, high nutrient levels would be evident in outgoing tides, representative of upstream water. The nutrients would be lower on incoming tides, which represent water from the Delaware Bay. Our objective was to monitor potential changes in water quality conditions in the creek during the day using the low and high tides as the predominating driver for the change.
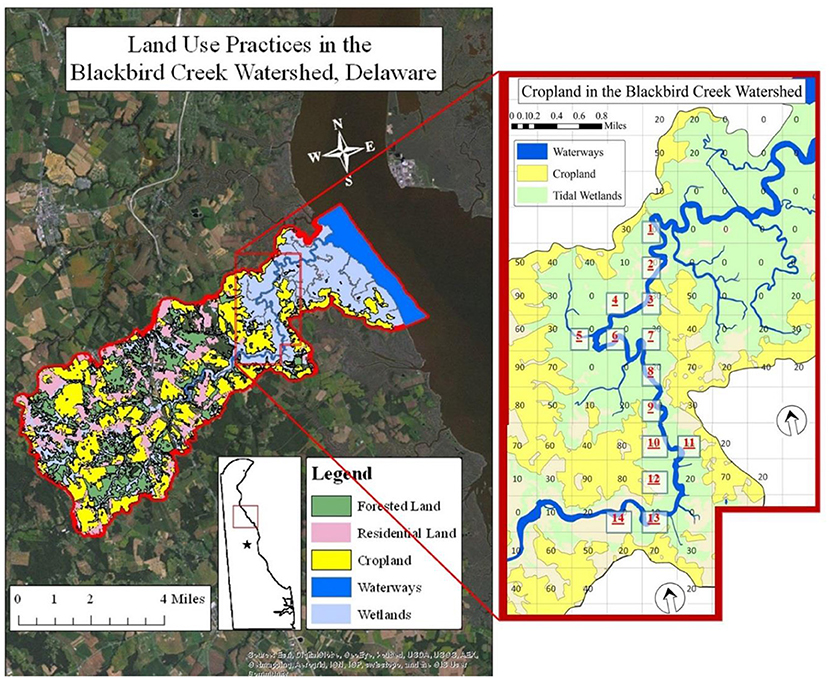
Figure 1. Aerial view of the Blackbird Creek Watershed, Delaware with an inset to show cropland coverage and site selections for sample extraction (Stone, 2016).
Materials and Methods
Study Sites
Blackbird Creek is a tidal waterway located along the central Delaware that drains a watershed of about 80 square kilometers into the Delaware Bay. Approximately, half of its 44 km in length is affected by tidal fluctuations while the upper portion of the waterway is comprised of freshwater only (DNREC, 2006). Several sites were selected in the tidal portion of the creek, representative of the lower 21 km of the waterway nearest to the bay.
Fourteen sites were chosen within the tidal range and measured for physical water quality parameters and nutrient concentrations on incoming and outgoing tides from May through August 2015. Those 14 sites were selected to encompass the portion of the creek upstream of the boat ramp (Figure 1). Each of the sites was labeled based on the percentage of agriculture within a 500 m × 500 m cell, as designated by aerial data collected in 2012. Each cell was labeled based on a range of percent cropland in order to achieve appropriate replicate habitats. Thus, a cell labeled “20” representing a spatial percentage of agricultural land between 20% and 30%. Within each cell, every site was identified as a 300 m stretch of water with 2.44 m PVC poles placed at the upstream and downstream endpoints of the sites. There were four treatments based on cropland percentage: 0–10, 10–20, 20–30, and 30–40%. The 0–10% treatment had seven replicates. The 10–20% treatment had three replicate stations. Each of the remaining categories had two replicates. On any given date, 10 of the 14 sites were chosen using a random number generator in Microsoft Excel® for collection due to time and labor accessing to the sites and commute to the Aquatic Sciences Lab at Delaware State University. Therefore, in some instances, replicate samples may not have been obtained. This was accommodated by repeated sampling within each of three seasons. The crop-planting season was defined as May–June. The growth season was July–August. The crop-harvesting season was defined as September–November. Water quality was monitored and water samples were collected during both high and low tides in this study from those sites.
Water Quality Field Monitoring
In 2015, samples were collected bi-weekly using a 17′ jon boat during the incoming and outgoing tides. Physical data were collected in situ at the time of water collection. Temperature (°C), pH, salinity, conductivity (mS cm−1), and dissolved oxygen (mg L−1) were obtained with a YSI 556 Multiprobe (YSI Incorporated, Yellow Springs, OH). Dissolved oxygen was based on the percent oxygen saturation of the water. The instrument also provided a measurement of dissolved oxygen in mg L−1 via an algorithmic calculation from the percent saturation and a measurement of salinity based on a calculation from the conductivity reading.
Water Quality Laboratory Testing
At each site, water was collected into 1L dark polyethylene bottles (rinsed 3 times with sample water), capped, and then placed on ice for transport to the lab for nutrient analysis. For ex situ analysis, the samples were measured for nitrate + nitrite, ammonia, orthophosphate, turbidity, and alkalinity. Because orthophosphate is reactive in nature, a subsample of water was filtered on site into a separate bottle in order to minimize the potential for such reactions. Nitrate was determined using HACH Method No.8171, the cadmium reduction method to detect levels between 0.1 mg L−1 NO3 and 10.0 mg L−1 NO3. Nitrite concentration was evaluated using HACH Method No.8507, the diazotization method with detection levels up to 0.30 mg L−1. Ammonia was measured using the Ammonia salicylate method, HACH Method No.8155, detecting ammonia concentrations between 0.01 and 0.50 mg L−1 NH3. Orthophosphate concentrations were determined using HACH Method No.8048, the ascorbic acid method. This reaction provided information on soluble reactive phosphate concentrations between 0.02 and 2.50 mg L−1 . Each of these parameters was measured on a HACH DR 3900 spectrophotometer (HACH, Loveland, CO). Palintest methods were used to determine alkalinity and turbidity concentrations in Blackbird Creek and measured on a YSI 9500 Photometer (YSI Incorporated, Yellow Springs, OH). Alkalinity was evaluated using an automatic wavelength selection, measuring between 0 and 500 mg L−1 CaCO3. Turbidity was also determined with an automatic wavelength selection and presented in Formazin Turbidity Units (FTU), which is generally equivalent to Nephelometric Turbidity Units and Jackson Turbidity Units (JTU). This method allowed for measurements between 5 and 400 FTU.
Statistical Analysis
A general linear model (GLM) was conducted for experimental design to determine differences in water quality by the incoming and outgoing tides at 14 study sites. Also within the model were interaction effects, which are dependent upon each other if proven to be significant: study sites × tides interaction term. Mann-Whitney U-Tests were used to determine significant differences between incoming and outgoing tides. With 95% confidence level, p < 0.05 gives the significance.
Results and Discussion
Figure 2 below shows the mean levels of water quality parameters tested during the incoming and outgoing tides during June through November in 2015. Mann-Whitney U-Tests showed significant differences between incoming and outgoing tides for NH3, NO2, , turbidity, salinity, pH, and conductivity (p < 0.05). There was no significant difference in temperature or dissolved oxygen. Raw data shows that the mean values for NH3, NO2, , pH were greater on outgoing tides (Figure 2). Conductivity, turbidity, and salinity were lower.
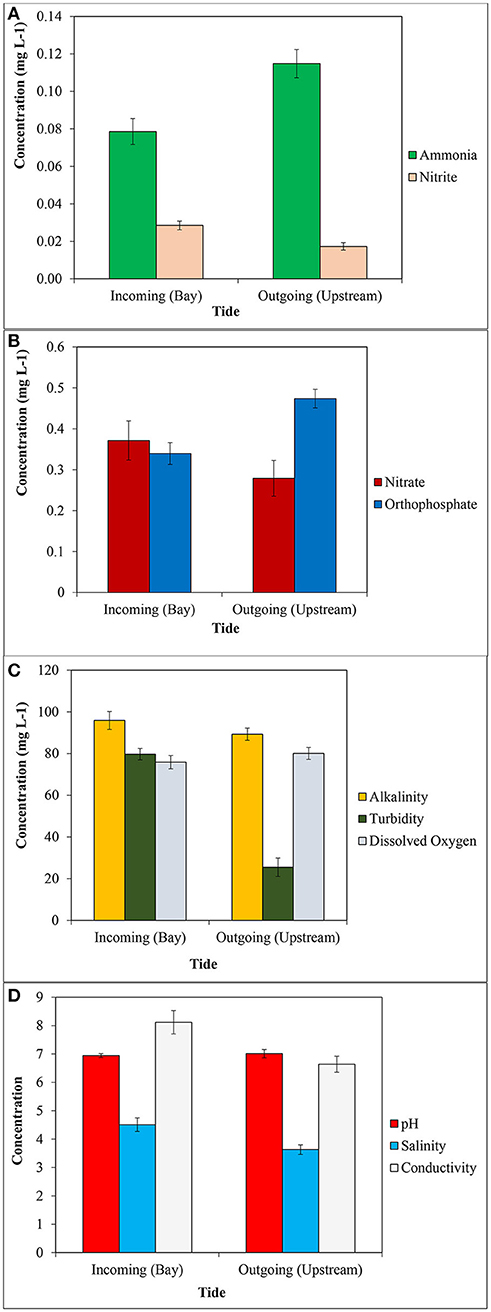
Figure 2. Mean concentrations of: (A) ammonia and nitrite, (B) nitrate and orthophosphate, (C) alkalinity, turbidity and dissolved oxygen, and (D) pH, salinity and conductivity in water in Blackbird Creek on inn coming and outgoing tides. Error bars represent standard error around the mean.
While we monitored differences in majority of water quality parameters (NH3, NO2, , Turbidity, Salinity, pH, and Conductivity) between incoming and outgoing tides, we found no differences in water quality parameters in relation to land uses in Blackbird Creek. Variability in temperature followed predictable seasonal patterns, and was not any different throughout the creek on any given sampling time. This was expected because the portion of Blackbird Creek that was investigated was not in an area with significant amounts of shade from trees or buildings. Thus, any temperature changes were accountable to environmental changes (Figure 3).
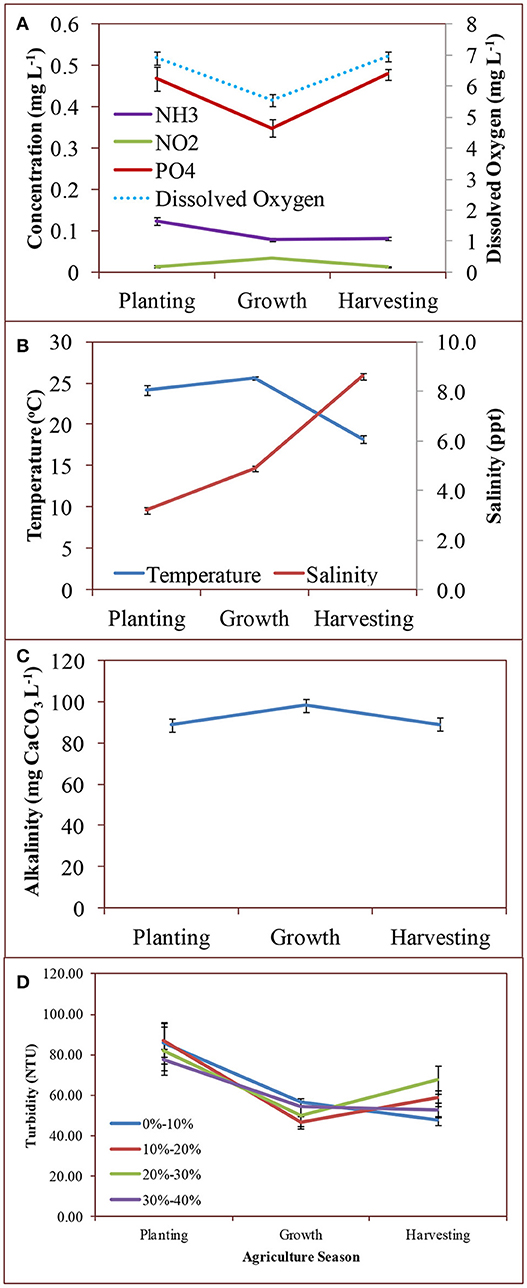
Figure 3. Mean concentration of: (A) ammonia, nitrite, orthophosphate, and dissolved oxygen, (B) temperature and salinity, (C) alkalinity, and (D) turbidity across agriculture seasons (Planting: May-June; Growth: July-August; Harvesting: September-November) in 2015. Error bars represent standard error around the mean.
Salinity throughout Blackbird Creek showed predictable trends over time. While differences in Salinity was expected between the incoming and outgoing tides, there was no difference between sites, but there were seasonal changes (Figure 3). Summer months have characteristically low rain and high temperatures in the Mid-Atlantic States. The tidal regime was a key player for salinity change daily. If the tide were on its way in from the Delaware Bay, salinity was greater due to the source of the water, especially if the tide had been incoming for several hours.
Turbidity throughout the waterway was extremely high, with extremes in 2015 on November 4th (32 NTU) and 14 May (222 NTU) in 2015. While there are no standards set forth by regulatory agencies to maintain the turbidity levels, studies have shown that values such as these can be detrimental to the ecosystem. Of primary concern is the problem that fish rely on sight and speed for prey capture may flee highly turbid water in order to find a food source. In addition, high turbidity can reduce light penetration into the water to the point that aquatic vegetation cannot photosynthesize for lack of light energy. These high turbidity values were likely a function of the nature of a tidal creek and not reflective of adjacent agricultural land. Modeling the turbidity in these ecosystems is challenging due to the complicated hydrodynamics and rheological behavior of the soft mud, which can dissociate easily under varying conditions (Dyer et al., 2004; Uncles et al., 2006). High rainfall, for example, can cause mudflats to break up and move into the waterway, especially during low tide. The changing environmental parameters of air temperature, rainfall, and wind may account for the significant differences in turbidity between seasons. However, it cannot be ignored that the turbidity was higher during the planting season (May and June)—that in which there may have been considerable tilling of agricultural fields, which loosened soil particles and increased the likelihood of the soil to runoff into the waterway (University of Minnesota Extension, 2018).
Generally, the dissolved oxygen in the creek was at appropriate levels according to the minimum acceptable standard to minimize growth effects of fish based on 5.0 mg L−1 established by the state of Delaware (DNREC, 2006) or 4.8 mg L−1 by the Environmental Protection Agency (USEPA, 2000). There were a few instances when the levels were indeed too low based on this standard. It is likely that this was due primarily based on the tidal structure at the time of collection. Nearly all of the measurements of lower dissolved oxygen were recorded at or near low slack tide, when movement of water was minimal. The extreme lows were recorded when readings were taken at low tide and mid-day during the peak summer months (Figure 3). Higher temperature water is not capable of holding as much dissolved oxygen as cooler water and standing water does not afford the opportunity of direct injection of oxygen from the air-water interface via wave action (Falkowski and Raven, 2007). However, most readings were taken when the tide was in flux, thus providing for appropriate ecological DO levels.
The buffering capacity of Blackbird Creek was high. The pH fluctuated little throughout the entire study period, and was an appropriate brackish water level, with a mean of 7.18 ± 0.3 SD. The high alkalinity values throughout the watershed were responsible for this, whereby the mean was 87.76 mg L−1 CaCO3 ± 23.71 SD, suitable to sustain aquatic life and retain the pH levels between agricultural seasons and treatments (Figure 3).
While it was likely that most of the contribution to alkalinity levels is from the carbonate and bicarbonate levels, some of the contribution may be from phosphates (Millero, 1996). Blackbird Creek had elevated levels of orthophosphate, according to the DNREC protocol outlined in 2006 (DNREC, 2006), where the maximum total phosphorous concentration in the system should not exceed 0.2 mg L−1. The data collected in this project revealed orthophosphate levels between 0.16 and 1.02 mg L−1 (Figure 3).Orthophosphate (or simply phosphate) represents the form that is most readily available for plant uptakes and typically enters the waterway by way of runoff of eroded soil from land (Pagliari et al., 2017). While the values were generally elevated, there was no suggestion in the data products that phosphate concentrations were influenced by agricultural practices. There were only significant seasonal differences, which seems to represent typical phosphorous cycling, with a reduction during the growth season due to plant uptake followed by an increase in aquatic phosphate in the harvesting season when plants die and phosphate is re-mineralized into the soil (Correll, 1998). The elevated concentrations were possibly evident due to the nature of tidal systems in general, whereby incoming and outgoing tides continually erode the creek's channel, which could be responsible for both increased turbidity and, by extension, increased phosphorous from upstream shorelines.
The surface water nitrogen parameters that were included in this project were nitrate, nitrite, and ammonia. Nitrite concentrations proved to be extremely low for most of the sampling season and between the sites in regards to land uses never measured >0.08 mg L−1 (Figure 3). Given that the acceptable level from the EPA is 1.0 mg NO2 L−1, there is no concern with the nitrite concentrations in Blackbird Creek at present time.
Nitrate concentrations were also well below the EPA-designated maximum contaminant level of 10 m L−1 (USEPA, 2013). The highest recorded level in Blackbird Creek was recorded once where there was no riparian buffer in place to prevent runoff of contaminants—the only known part of Blackbird Creek. Previous research at BBC by Roeske (2013) suggested that a crop field near the location where this sample was extracted grew corn in 2013. In 2014, the field ran fallow. It may be suggested that legacy nutrients, which were not used by crops in 2013, were then able to run into the waterway early in the season. Never after this sampling date were nitrate values >1.41 mg L−1 (Stone, 2016). Nevertheless, it cannot be ignored that there were increased nitrate levels, which proved to be significant between cropland and forested wetland.
The final parameter of interest was ammonia. The levels were low (0.09 mg L−1 ± 0.043 SD). There is no EPA-designated standard contaminant level for ammonia, but it was recommended by EPA in 2013 that 1.9 mg L−1 should be the maximum contaminant level to sustain aquatic life (USEPA, 2013). There was a significant difference in ammonia levels between seasons for both data sets, suggesting that there may be some impact on the parameter by agricultural use (Figure 3). However, because there is no difference between sites in relation to % agriculture uses, this is unlikely.
Flyover aerial images of the Blackbird Creek Watershed obtained by Roeske (2013) showed some agricultural land cover in close proximity to the creek with limited vegetative buffers to the creek banks. Department of Natural Resource and Environmental Control (DNREC Delaware National Estuarine Research Reserve (DNERR), 2007) identified restoration needs and opportunities for forests as the riparian buffer restoration to augment poorly buffered corridors in terms of width and composition. As they stated in their report, both water quality and habitat connectivity are affected by their current condition.
The seasonal variability of the nitrogen parameters in general may be more likely attributable to tidal creek geo-hydrodynamics and microbial nitrification and denitrification processes. Concomitant research in Blackbird Creek identified several nitrogen-fixing bacteria that influence nitrogen species concentrations, including Nitrosomas and Rhizobium (Ozbay et al., 2014). Several studies have shown that microbial concentrations and associated nitrogen-fixation processes fluctuate seasonally (i.e., Biesboer, 1984; Ravikumar et al., 2012). Bacteria tend to be greatest in number at the height of the growing season. Because the population is increased, there is greater potential for mediating the nitrification and denitrification processes and reducing concentrations of ammonia and nitrate in surface waters, especially at the soil-water interface. Thus, even if there were some excess runoff from cropland (which was not proven in statistical analysis), much of that would be taken up by these bacteria for their consumptive and regulatory processes, a phenomenon would might explain the seasonal variation in nitrogen variables in Blackbird Creek without any significance between sites in relation to % agriculture uses.
Based on our study, Blackbird Creek does not appear to be influenced by the agriculture land cover. Orthophosphate values were greater than the EPA-recommended maximum, but it is unlikely that this is due to agricultural land use because there were no differences between the sites in relation to percentage agriculture uses. It is more likely that the phosphate was high due to tidal scouring, a natural component of a tidal creek.
Turbidity values were elevated in the system, but that was to be expected for a Mid-Atlantic tidal creek and was probably not attributable to erosion of soil from modified land. Indeed, this reflects the results from the National Estuarine Research Reserve data synthesis report, where it was shown that in areas of intermediate salinity, turbidity was generally elevated (Brush et al., 2007).
Conclusion
In a unidirectional waterway, elevated nutrients may be compared only to another entire watershed in order to ascertain whether there is excessive runoff from agricultural fields. In this instance, a second waterway is not needed, as it exists within itself if a researcher were to wait 6 h or so for the tide to shift. The elevated values for these several nutrients on outgoing tides suggest that there is runoff from the upstream croplands, especially given that those values are lower on incoming tides, which represent water from the Delaware Bay. While there may be higher values on outgoing tides, the flushing rate of the system is quite fast, so there is no evidence to suggest that the waterway is being “polluted.” With the intermittent exception of orthophosphate, all values on both tides fall below the requirements set forth by the EPA.
Per our assessment based on our research findings, the riparian buffer system that was in place in Blackbird Creek appeared to be working efficiently as an appropriate nutrient management practice by disallowing runoff of contaminants into the waterway.
Author Contributions
GO is the principal investigator of the project discussed in this manuscript. She led the project efforts and activities and provided training and support students needed. She helped with data interpretation, discussion and manuscript preparation. MS was involved in the initial planning and overall execution of this project. He prepared a report and was involved in field sampling, data collection and analyses. He reported the program outcomes and presented during the statewide meeting.
Funding
This study was supported primarily by the NSF EPSCoR Program Grant Award# EPS-1301765, and partially by USDA-NIFA CBG Grant Award# 2014-38821-22430 and USDA-NIFA CBG Grant Award# 2013-38821-21246.
Conflict of Interest Statement
The authors declare that the research was conducted in the absence of any commercial or financial relationships that could be construed as a potential conflict of interest.
Acknowledgments
We would like to thank DSU-CARS, Delaware EPSCoR Program and specifically Dr. Venu Kalavacharla for the support. Special thanks go out to our research lab and all of the assistants who helped collect and analyze the data, especially our technician, Laurieann Phalen, and valuable discussion by Dr. Lathadevi Karuna. Thanks to fellow students in GO lab who assisted in this project including Katherine Ommanney, Benjamin Jenkins, Brittney Chesser, and Nivette Perez-Perez.
References
Allan, J. D. (2004). Landscapes and riverscapes: the Influence of land use on stream ecosystems. Ann. Rev. Ecol. Evol. Syst. 35, 257–284. doi: 10.1146/annurev.ecolsys.35.120202.110122
Bachman, L. J., Phillips, P. J., and Denver, J. M. (1994). Quality of Baseflow in Non-tidal Tributaries to the Chesapeake Bay, Delmarva Peninsula, Delaware and Maryland. EOS, Transactions of the American Geophysical Union. Abstract HB32–3.
Biesboer, D. (1984). Seasonal variation in nitrogen fixation, associated microbial populations, and carbohydrates in roots and rhizomes of Typha latifolia (Typhaceae). Can. J. Bot. 62, 1965–1967.
Bingham, S. C., Westerman, P. E., and Overcash, M. R. (1980). Effect of grass buffer zone length in reducing the pollution from land application areas. Trans. Am. Soc. Agri. Eng. 23, 330–342.
Blann, K., Nerbonne, J. F., and Vondracek, B. (2001). Relationship of riparian buffer type to water temperature in the driftless area of ecoregion of minnesota. N. Am. J. Fish. Manag. 22, 441–451. doi: 10.1577/1548-8675(2002)022 < 0441:RORBTT>2.0.CO;2
Boesch, D. F., Brinsfield, R. B., and Magnien, R. E. (2001). Chesapeake bay eutrophication: scientific understanding, ecosystem restoration, and challenges for agriculture. J. Environ. Qual. 30, 303–320. doi: 10.2134/jeq2001.302303x
Brush, M. J., Moore, K. A., and Condon, E. D. (2007). Synthesis of Data from the National Estuarine Research Reserve System-Wide Monitoring Program for the Mid-Atlantic Region. Report: The NOAA/UNH Cooperative Institute for Coastal and Estuarine Environmental Technology (CICEET), 120.
Carpenter, S. R., Caraco, N. F., Correll, D. L., Howarth, R. W., Sharpley, A. N., and Smith, V. H. (1998). Nonpoint pollution of surface waters with phosphorous and nitrogen. Ecol. Appl. 8, 559–568.
Castelle, A. J., Conolly, C., and Johnson, A. W. (1994). Wetland and stream buffer size requirements–A review. J. Environ. Qual. 23, 878–882.
Correll, D. (1998). The role of phosphorous in the eutrophication of receiving waters: a review. J. Environ. Qual. 27:261–267.
Costlow, J. D. Jr. (1967). The effect of salinity and temperatures on survival and metamorphosis of megalops of the blue crab, Callinectes sapidus rathbun. Helgoland Mar. Res. 15, 84–87.
Diaz, R. J., and Rosenberg, R. (2008). Spreading dead zones and consequences for marine ecosystems. Science 321:926–929.
DNREC (2006). Blackbird Creek Watershed Proposed TMDLs. Report DNRE0080, Dover, DE, 54. Available online at: http://www.dnrec.delaware.gov/swc/wa/Documents/TMDL_TechnicalAnalysisDocuments/5_BlackbirdTMDLAnalysis.pdf
DNREC Delaware National Estuarine Research Reserve (DNERR) (2007). Blackbird Creek Reserve Ecological Restoration Master Plan March 2007. Dover, DE. Available online at: http://www.dnrec.delaware.gov/coastal/DNERR/Documents/Blackbird%20EMP%20Final%20Print%208x11.pdf
Drexler, J. Z., and Bedford, B. (2002). Pathways of nutrient loading and impacts on plant diversity in a New York Peatland. Wetlands 22, 263–281. doi: 10.1672/0277-5212(2002)022[0263:PONLAI]2.0.CO;2
Dyer, K. R., Christie, M. C., and Manning, A. J. (2004). The effects of suspended sediment on turbulence within an estuarine turbidity maximum. Estuar. Coast. Shelf Sci. 59, 237–248. doi: 10.1016/j.ecss.2003.09.002
Falkowski, P. G., and Raven, J. (2007). Aquatic Photosynthsis, 2nd Edn. Princeton, NJ: Princeton University Press.
Forney, W., Raumann, C. G., Minor, T. B., Smith, J. L., Vogel, J., and Vitales, R. (2001). Land Use Change and Effects on Water Quality and Ecosystems Health on the Lake Tahoe Basin, Nevada and California. USGS Report 01-418 (Menlo Park, CA: United States Geological Survey), 29.
Jayawickreme, D. H., Santoni, C. S., Kim, J. H., Jobbágy, E. G., and Jackson, R. B. (2011). Changes in hydrology and salinity accompanying a century of agricultural conversion in Argentina. Ecol. Appl. 21, 2367–2379. doi: 10.1890/10-2086.1
Johnson, L. B., Richards, C., Host, G. E., and Arthur, J. W. (1997). Landscape influences on water chemistry in midwestern stream ecosystems. Freshw. Biol. 37, 193–208.
Kling, C. L., Panagopoulos, Y., Rabotyogov, S. S., Valcu, A. M., Gassman, P. W., Campbell, T., et al. (2014). LUMINATE: linking agricultural land use, local water quality and Gulf of Mexico Hypoxia. Eur. Rev. Agri. Econ. 41, 431–459. doi: 10.1093/erae/jbu009
Lu, Q., He, Z. L., and Stoffella, P. J. (2012). Land application of biosolids in the USA: a review. Appl. Environ. Soil Sci. 1–11. 2012:201462. doi: 10.1155/2012/201462
Mazzotti, F. J., Pearlstine, L. G., Barnes, T., Volety, A., Chartier, K., Weinstein, A., et al. (2006). Stressor Response Models for the Blue Crab, Callinectes sapids. JEM Technical Report. Final report to the South Florida Water Management District and the U.S.G.S. Univ. Florida, FL, 12.
Mistiaen, J. A., Strand, I. E., and Lipton, D. (2003). Effects of environmental stress on blue crab (Callinectes sapidus) harvests in Chesapeake Bay Tributaries. Estuaries 26, 316–322. doi: 10.1007/BF02695970
Ozbay, G., Roeske, K., Chintapenta, L. K., Kalavacharla, V., Stone, M., and Phalen, L. (2014). Land use impacts: the effects of non-native grasses on marsh and aquatic ecosystem. Ecosyst. Ecogr. 4, 1–5. doi: 10.4172/2157-7625.1000151
Pagliari, P. H., Kaiser, D. E., Rosen, C. J., and Lamb, J. A. (2017). The Nature of Phosphorus in Soils. Nutrient Management. University of Minnesota Extension FO-6795-C (REVISED 2017). Available online at: https://extension.umn.edu/nutrient-management/phosphorus-and-potassium (Accessed April 20, 2018).
Paul, M. J., and Meyer, J. L. (2001). Streams in the urban landscape. Ann. Rev. Ecol. Syst. 32, 333–365. doi: 10.1146/annurev.ecolsys.32.081501.114040
Pimentel, D., Berger, B., Filiberto, D., Newton, M., Wolfe, B., Karabinakis, E., et al. (2004). Water Resources, Agriculture, and the Environment. Ithaca. Report 04–1NY. New York State College of Agriculture and Life Sciences, Cornell University.
Pomilio, M. (2015). DE_LandUse_LandCover_12. Geospatial Data Presentation Form: Vector Digital Data. Office of State Planning Coordination, State GIS Coordinator.
Postel, S. L., Daily, G. C., and Ehrlich, P. R. (1996). Human appropriation of renewable fresh water. Science 271, 785–787.
Rabalais, N. N., Turner, R. E., Justic, E., Dortch, Q., Wiseman, W. J. B., and Sen Gupta, K. (1996). Nutrient changes in the Mississippi River and system responses on the adjacent continental shelf. Estuaries 19, 386–407.
Ravikumar, S., Gnanadesigan, M., Ignatiammal, S. T., and Sumaya, S. (2012). Population dynamics of free living, nitrogen fixing bacteria Azospirillum in Manakkudi Mangrove Ecosystem, India. J. Environ. Biol. 33, 597–602.
Roeske, K. P. (2013). Assessing the Impact of Phragmites australis subspecies australis on Blue Crab (Callinectes sapidus) and Fish Population Dynamics in Blackbird Creek, Delaware. Masters' thesis, Department of Agriculture and Natural Resources, Delaware State University, Dover, DE.
Schempf, M., and Cox, C. A. (2007). Managing Environmental Landscapes for Environmental Quality: Strengthening the Science Base. Ankeny, IA: Soil and Water Conservation Society.
Spruill, T. B. (2000). Statistical evaluation of effects of riparian buffers on nitrate and ground water quality. J. Environ. Qual. 29, 1523–1538. Available online at: https://nc.water.usgs.gov/reports/abstracts/JEQriparian.html
Stone, M. (2016). Land Use Practice and Water Quality, Blue Crab Population Dynamics and Fish Biodiversity in Blackbird Creek, Delaware. Masters' thesis in Natural Resources, Department of Agriculture and Natural Resources, Delaware State University, Dover, DE 19901, 116.
Uncles, R. J., Stephens, J. A., and Law, D. J. (2006). Turbidity maximum in the macrotidal, highly turbid humber estuary, UK: Flocs, fluid mud, stationary suspensions and tidal bores. Estuar. Coast. Shelf Sci. 67, 30–52. doi: 10.1016/j.ecss.2005.10.013
University of Minnesota Extension (2018). Introduction to Soil Management. Available online at: https://extension.umn.edu/soil-and-water/soil-management-and-health (Accessed April 20, 2018).
USEPA (2000). Ambient Aquatic Life Water Quality Criteria for Dissolved Oxygen (Saltwater): Cape Cod to Cape Hatteras. Report EPA-822-R-00-012, 140.
USEPA (2013). Aquatic Life Ambient Water Quality Criteria for Ammonia – Freshwater – 2013. Report: EPA 822-R-13-001. Springfield, VA, 142.
Keywords: blackbird creek, outgoing tides, incoming tides, water pollutants, chemical, bi-directional water, nutrients
Citation: Ozbay G and Stone M (2018) Bi-Directional Waterway Reveals Nutrient Runoff From Cropland. Front. Environ. Sci. 6:114. doi: 10.3389/fenvs.2018.00114
Received: 06 May 2018; Accepted: 18 September 2018;
Published: 16 October 2018.
Edited by:
Mohiuddin Md. Taimur Khan, Washington State University, United StatesReviewed by:
M. Jahangir Alam, University of Houston, United StatesKeith Dana Thomsen, National Aeronautics and Space Administration (NASA), United States
Copyright © 2018 Ozbay and Stone. This is an open-access article distributed under the terms of the Creative Commons Attribution License (CC BY). The use, distribution or reproduction in other forums is permitted, provided the original author(s) and the copyright owner(s) are credited and that the original publication in this journal is cited, in accordance with accepted academic practice. No use, distribution or reproduction is permitted which does not comply with these terms.
*Correspondence: Gulnihal Ozbay, gozbay@desu.edu
† Present Address: Matthew Stone, Center for Aquatic Biology & Aquaculture (CABA), University of California, Davis, Davis, CA, United States