- INRA, LSE, Université de Lorraine, Nancy, France
Research over the last few decades has shown that the characterization of microaggregates at the micrometer scale using Transmission Electron Microscopy (TEM) provides useful information on the influence of microorganisms on soil functioning. By taking soil heterogeneity into account, TEM provides qualitative information about the state of bacteria and fungi (e.g., intact state of living organisms, spores, residues) at the sampling date within organo-mineral associations, from the soil-root interface to the bulk soil, and in biogenic structures such as casts. The degree of degradation of organic matter can be related to the visualized enzymatic potential of microorganisms that degrade them, thus indicating organic matter dynamics within soil aggregates. In addition, analytical TEM characterization of microaggregates by EELS (Electron Energy Loss Spectroscopy) or EDX (Energy Dispersive X-rays spectroscopy) provides in situ identification of microbial involvement in the biogeochemical cycles of elements. Furthermore, micrometer characterization associated with other methodologies such as Nanoscale Secondary Ion Mass Spectrometry (NanoSIMS) or soil fractionation, enables monitoring both incorporation of biodegraded litter within soil aggregates and impacts of microbial dynamics on soil aggregation, particularly due to production of extracellular polymeric substances. The present focused review suggests that such an approach using micrometer characterization of soil microhabitats provides relevant qualitative and quantitative information when monitoring and modeling microbial processes in dynamics of organo-mineral associations.
Introduction
How a soil functions is closely related to the dynamics of soil microbial communities. Microbial habitats and soil structure are so intimately related that knowledge of these interactions appears essential to understand soil processes and management. While microorganisms influence soil structure as aggregation agents (Tisdall and Oades, 1982), conversely, soil texture and structure form microhabitats (Stotzky, 1986) and thereby strongly influence the dynamics, ecology, and activity of microbial populations. Bacteria are distributed throughout pores of various sizes and within aggregates (Hattori and Hattori, 1976), while simultaneously organic matter provides micro-niches adequate for microorganism growth (Guggenberger et al., 1999). Chenu and Cosentino (2011) described the role of microorganisms in the temporal and spatial dynamics of aggregation. They detailed the formation, stabilization and destruction of aggregates by microorganisms as well as impacts of soil structure evolution on microbial activity. Thus, the visualization of microorganisms within soils has long been, and is especially now, both a real need and a challenge in order to assess “life in inner space” (Ritz, 2011; Baveye et al., 2018).
The in situ observation of soil biota requires that the fabric of the soil be left as undisturbed as possible (Ritz, 2011). In their review of micrometer determinants of bacterial diversity in soils, Vos et al. (2013) presented several methods to describe micrometer-sized soil habitats, based on sieving, dissecting and visualizing individual soil aggregates. They cited advantages and disadvantages of associated techniques, such as Scanning Electron Microscopy (SEM) associated with Energy-Dispersive X-ray spectroscopy (EDX), Infrared Spectroscopy, Nanoscale Secondary Ion Mass Spectrometry (NanoSIMS) and Near Edge X-ray Absorption Fine Structure Spectroscopy. These techniques usually use polished, resin-embedded whole aggregates and thus, from a methodological point of view, require sample preparation similar to that used for Transmission Electron Microscopy (TEM) characterization. Nevertheless, results in the literature characterize and localize organic matter and elements more often than bacterial units. Micro-computed tomography and nuclear magnetic resonance or magnetic resonance imaging are also cited as non-destructive approaches in three-dimensional (3D) analysis of soil structure. Finally, fluorescence in situ hybridization (FISH) techniques combined with micro-pedological methods are promising, allowing specific classes of microorganisms to be localized in soils by using oligonucleotide probes (Eickhorst and Tippkötte, 2008). In addition, micro-cartography of digital-sequential images of soil thin sections combined with fluorescent microscopy improves understanding of the distribution and quantification of hotspots and biofilms in soils regardless of the micrometer scale (Nunan et al., 2001; Castorena et al., 2016). As a precursor, Foster emphasized the use of TEM to visualize in situ soil microstructures, particularly in the rhizosphere, which has been the main site of micrometer studies of soils and soil microorganisms (Foster et al., 1983; Foster, 1988). Within the rhizosphere, many physical, chemical and biological processes take place, stemming from root growth, water and nutrient uptake, respiration and rhizodeposition (Hinsinger et al., 2005). Microbial activity is therefore much greater there than in non-rhizopheric soil due to root activity (Lynch, 1990). TEM makes it possible to identify cellular components such as cellulose, lignin and condensed polyphenolic substances within plant tissues and soil aggregates and to localize bacteriaband fungi, while identifying their intact state at the sampling date, (e.g., alive, sporulated, dead) and their enzymatic activity within cellular components by visualizing areas of lysis around the microorganisms (Kilbertus, 1980; Foster et al., 1983). Roose et al. (2016) reviewed current developments in structural and chemical imaging of the rhizosphere. Although they focus on the influence of pore structure in the rhizosphere on water and nutrients fluxes, the techniques and the main results they mention also concern soil-microorganism interactions. TEM combined with micro-analysis, immuno-labeling or specific staining procedures is extremely well-suited for localizing microorganisms within soil structures at the micrometer scale, whether inside or outside rhizospheres (Hattori and Hattori, 1976; Foster and Martin, 1981; Bartoli et al., 1986; Chotte et al., 1992; Ladd et al., 1993). In addition, image analysis of visualized soil parameters (e.g., pores, roots) can quantify impacts of soil-biota interactions and strengthens use of visualizing techniques. Nevertheless, using TEM requires the physical and chemical fixation of microstructures to avoid any shrinkage, staining procedures to enhance organic matter contrast, and preparation of ultra-thin sections despite the presence of potentially damaging minerals. This is why TEM, despite the large amount of information it can yield, may sometimes seem less approachable to all than other techniques.
In this article, we highlight the use of TEM to elucidate mutual interactions between soils and microorganisms, thereby identifying soil processes and microbial activity. The feasibility of using TEM to localize microorganisms and deduce their influence within soil structures is illustrated by studies involving a variety of soils in different contexts (i.e., five cropped soils, one forest soil and one Technosol). The results presented, most of them already published, are used as examples to highlight microbial contribution to (i) organic matter turnover within microhabitats, assessed through a combination of TEM, Electron Energy Loss Spectroscopy (EELS) or NanoSIMS; (ii) soil aggregation inside and outside rhizospheres and (iii) microaggregate dynamics as indicators of soil health. This focused review of using TEM to assess soil-microorganisms interactions at the micrometer scale is motivated by the need to visualize soil microhabitats efficiently for soil microbiology, providing both qualitative and quantitative data and considering soil heterogeneities. Samples must be representative and soil structure must be preserved to ensure the relevance of results. As hotspots of biological activity, rhizosphere, drilosphere (casts), and 0–20 μm size fractions of soil were sampled and morphologically or analytically characterized by TEM. We discuss relationships among microbial dynamics within these organo-mineral associations and how the soil functioned as a whole, with the aim of identifying data to improve modeling of microbial dynamics in soil processes, in particular soil organic matter evolution and soil aggregation.
Materials and Methods
Soils
We review 5 soils from agrosystems (soils 1–5), one soil from a forest ecosystem (soil 6), and one constructed Technosol (soil 7). Soil names are given according the WRB classification (2014) (see Table S1 of the main physico-chemical parameters):
- soil 1: a calcic Cambisol developed on silt deposits. It was cropped with maize (Zea mays) after moderate tillage and fertilization at an experimental station. Digested sewage sludge was applied according to regulations. Sampling allowed us to monitor any impact of cropping practices on micro-aggregation (Watteau et al., 2012).
- soil 2: a Luvisol developed from aeolian silt on an alluvial terrace in western France and cropped with maize. The impact of cattle manure amendment on soil fertility was studied.
- soil 3: a Cambisol collected from a permanent grassland in northern France. It was used in a mesocosm experiment to study the incorporation of 13C-labeled organic matter [roots or shoots of Italian ryegrass, (Lolium multiflorum)], in the presence or absence of earthworms (Lumbricus terrestris) (Vidal, 2016). It was sieved to 4 mm for homogenization, plant residues and macrofauna > 4 mm were removed, and then the soil was placed into PVC containers (80 cm long × 40 cm in diameter) for 6 months. Results of the treatment with incubation of 13C-labeled ryegrass shoots with earthworms are presented.
- soil 4: a Luvisol developed on old gravelly alluvium sampled from a long-term field experiment in south-eastern France. It was cropped with maize and studied to highlight impacts of root on microaggregation (Watteau et al., 2006).
- soil 5: a Vertisol from south-eastern Martinique. Because of their high exchangeable sodium content, these soils have low structural stability and high susceptibility to erosion, in particular when intensively cropped, which leads to a loss of organic matter. Fallowing these soils, however, rebuilds organic matter content and thus makes them less erodible (Campbell et al., 1999). Results comparing a Vertisol under a former grassland to the same vertisol under former market gardens (Blanchart et al., 2000) are presented.
- soil 6: a Podzol under a beech (Fagus sylvatica) ecosystem in eastern France. This study was designed to identify root aging at the micrometer scale using TEM and EELS (Watteau et al., 2002).
- soil 7: a constructed Technosol. Constructing soil to reclaim derelict land is based on the recycling of secondary by-products. Using pedological engineering concepts, these by-products are combined to construct a new soil (Technosol) over in situ brownfield substrates (Séré et al., 2010). It consisted of three different parent materials deposited in layers (from top to bottom): (i) green-waste compost (10 cm) from urban trees and grass cuttings, (ii) a mixture (1:1 v/v) of paper-mill sludge and thermally treated industrial material extracted from a former coking plant (80 cm), and (iii) pure paper-mill sludge (25 cm). This Technosol was constructed during field tests in 2003 at the GISFI experimental station (http://gisfi.univ-lorraine.fr) in eastern France. The soil was seeded with Italian rye grass and lucerne (Medicago sativa).
Soil Fractionation
Three to five samples of 200 g of soils 1–7 were taken from the topsoil, pooled and kept wet until their conditioning for soil fractionation. The 0–20 μm size fractions were obtained after soil fractionation in water from the soil particles <2-mm obtained by dry sieving fresh soil. Thirty-gram samples were dispersed in 200 ml distilled water and stirred gently for 1 h. The 200 μm−2 mm fractions were obtained by wet sieving. The 0–200 μm fractions were dispersed for 16 h in 500 ml distilled water. The 50–200 μm fractions were then obtained by wet-sieving. The 0–2, 2–20, and 20–50 μm fractions were collected by sedimentation using the Robinson pipette method. All fractions were oven-dried at 60°C and weighed. Weight distributions were adjusted to 100%, and percentages of the fractions were expressed as the means and standard errors of three replicates. From the resulting 0–50 μm residue, 0–2, 2–20, and 20–50 μm fractions were collected separately, according to Stokes' law. To obtain enough material for further analyses, 0–2 μm fractions were mainly collected by five serial elutions of 0–50 μm fractions without the addition of flocculating agents. Parts of these fractions were oven-dried at 110°C and finely ground for elementary analyses, while the remainders were left undried and stored at 8°C until they were processed for TEM.
Morphological and Analytical Characterization by TEM
Roots, casts and 0–20 μm size soil fractions were sampled and morphologically or analytically characterized by TEM. The roots more or less associated with rhizospheric soil were sampled because of the numerous soil-plant-microorganism interactions within the rhizosphere (Waisel et al., 2002). Earthworms are strongly involved in aggregate formation, microbial dynamics in the soil profile, and sequestration/mineralization of organic matter in tropical and temperate environments (Bossuyt et al., 2005; Jouquet et al., 2009). The fine soil fractions, with a particle diameter of 0–20 μm, are considered the most reactive, given their high cation exchange capacity, stable carbon (C) content, microbial (C) content (Anderson et al., 1981; Eliott, 1986; Chotte et al., 1992; Balabane and Plante, 2004), and their recalcitrance to biodegradation (Hassink, 1997). In addition, 2–20 μm micro-aggregates represent very favorable habitats for bacteria in most soils (Ranjart and Richaume, 2001).
Three replicates of a few cubic millimeters each were sampled from roots, casts, and 2–20 μm fractions for TEM examination. These sub-samples were fixed in 2% (w/v) osmium tetroxide in a cacodylate buffer (pH 7.2) for 1 h in order to chemically fix the organo-mineral structure. Osmium-fixed samples were dehydrated in graded acetone solutions, then embedded in epoxy resin (Epon 812) until complete polymerization (16 h at 60°C). Ultra-thin sections (80–100 nm) were cut with a diamond knife on a Leica Ultracut S ultramicrotome. Some sections were filed on nickel grids, stained with uranyl acetate and lead citrate to enhance organic matter contrast, and examined in a JEM 1200 EXII transmission electron microscope operating at 80 kV. A minimum of 15 observation fields at a magnification of 4000 were performed for each of the three replicates. Unstained ultra-thin sections were filed on nickel grids covered with a C film and used for in situ elementary analyses with EDX, EELS or NanoSIMS (Watteau and Villemin, 2001; Watteau et al., 2002; Vidal et al., 2016). EDX is a simple and fast technique but does not give access to light elements (from Z = 3) and is of lower resolution than EELS, whose results analysis is still more complicated (Egerton, 1986; Bauer, 1988).
Results and Discussion
Microbial Activity Within Microstructures
TEM observations of the 2–20 μm fractions of soils 1–3 revealed different states of microorganisms in soil microstructures (Figure 1). Microorganisms can be observed in pores or in aggregates, individually or when gathered into colonies. Bacteria are present within microaggregates, associated either with the organic residues they degraded or with minerals, mainly due to production of extracellular polymeric substances (EPS) (Figures 1.1, 1.2). Typical bacterial aggregate consisting of EPS-producing bacteria, on which minerals are adsorbed, are also frequently observed (Figures 1.3, 1.4). At the time of sampling, bacteria within microaggregates of soil 2 were alive and intact (Figure 1.4), sometimes dividing (Figure 1.5), spores waiting for better conditions (Figure 1.6), or dead in the form of cell wall residues (Figure 1.4). Actinomycetes and fungi are also observed to be associated within soil 3 aggregates (Figures 1.7, 1.8). Certain typical intracellular features (cytoplasmic membranes) allow us to identify nitrogen-cycle bacteria in casts sampled from soil 7 (Figure 1.9). Because sampling and sample processing preserve the initial soil structure, TEM allow localization of the main microorganisms within aggregates.
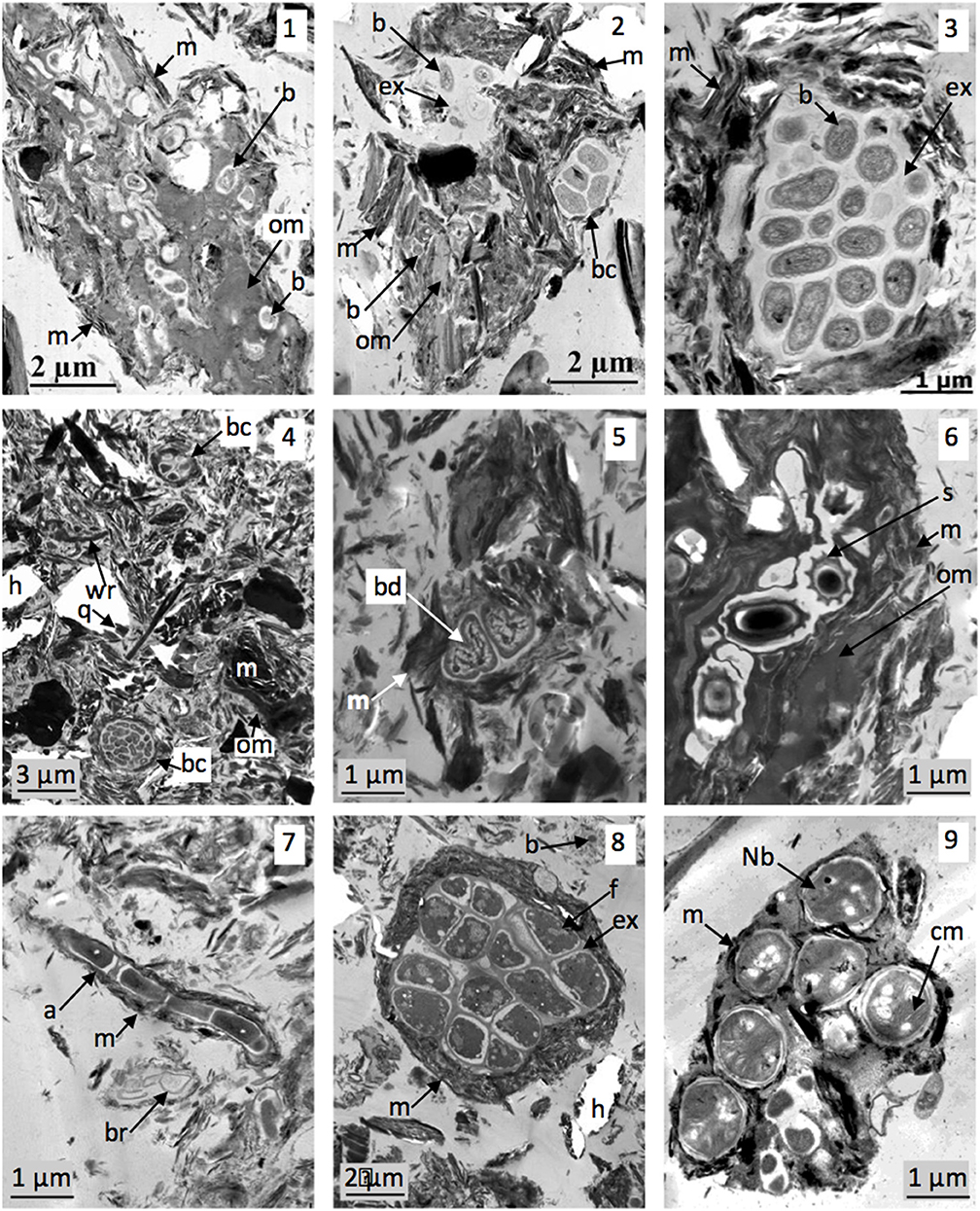
Figure 1. Microorganism localization within soil microstructures. TEM views (1–3): soil 1 microaggregates (already published in Watteau et al., 2012). (1) Organic residue colonized by bacteria and surrounded by with minerals; (2) mainly mineral aggregate containing few organic residues and EPS producing bacteria (individualized or in colony); (3) typical bacterial aggregate constituted by bacteria producing EPS on which are adsorbed minerals. TEM views (4–6): soil 2 aggregates. (4) Global view of 2–20 μm fraction showing mineral or organic particles and bacterial aggregates as bacterial colony associated with minerals. (5) Bacteria in division within aggregate; (6) bacterial spores localized in organic residue associated with minerals. TEM views (7,8): microorganisms in soil 3. (7) Actinomycete associated with minerals; (8) fungal aggregate. TEM view (9) Bacteria of N cycle in soil 4 (already published in Pey et al., 2014). a, actinomycete; b, bacteria; bc, bacterial colony; bd, bacteria in division; br, bacterial residue; cm, cytoplasmic membrane; ex, EPS; f, fungus hypha; h, hole in the resin as an artifact due to mineral presence; Nb, bacteria of nitrogen cycle; om, organic matter; m, mineral; q, microquartz; s, bacterial spore.
These microhabitats visualized by TEM highlight their impact on two main parameters of soil-microorganism interactions: physical protection of microorganisms and nutrient availability. As an illustration of the ability of microorganisms to degrade organic matter, fungi and bacteria attack ligneous residues in soil 3 [Figure S1, (see Datasheet 2)]. Areas of lysis due to fungal activity are visualized in ligneous thickenings, suggesting fungal ligninolytic capacity. Cell intersections already persist, and many bacteria colonize these plant tissue residues until they get completely fragmented. Due to the spatio-temporal sampling, TEM allow us to monitor the successive microbial degradation of organic matter identified within microstructures. TEM microanalysis also identifies microbial enzyme production on beech roots sampled from soil 6 (Watteau et al., 2002). EELS analysis of the contrasting rings surrounding areas of lysis observed in polyphenolic substances contained in cortical cells detect a large amount of nitrogen [Figure S1.2 (see Datasheet 2), EEL spectrum 2]. However, no nitrogen is detected in the polyphenolic substances themselves [Figure S1.2 (see Datasheet 2), EEL spectrum 1]. This supplemental nitrogen came from enzymes produced by hyphae colonizing these cells. Another way to identify organic matter turnover is to combine TEM and NanoSIMS. Vidal et al. (2016) sample earthworm casts as newly formed aggregates in soil 3, to which 13C-labeled shoot residues had been added. From 13C labeling and NanoSIMS imaging of ultra-thin sections, these authors observe incorporation of 13C-labeled carbon in casts and its microflora [Figure S1.3 (see Datasheet 2) and NanoSIMS maps]. NanoSIMS imaging does not always allow them to recognize features of organic matter and microorganisms within aggregates because of the heterogeneity of the aggregates' organo-mineral components and arrangement. Previous TEM examination of the aggregates on the same ultra-thin sections used for NanoSIMS ensured the identification of the features analyzed. Thus, contribution of microorganisms to degradation of freshly introduced litter can be monitored within aggregates at a micrometer scale. Supplementing such information about the C cycle, TEM-EDX and TEM-EELS can also be used to monitor microbial involvement in the biogeochemical cycles of other main elements (e.g., silicon; Watteau and Villemin, 2001).
Biologically promoted soil aggregates are of increasing interest in soil structure dynamics, as hotspots of biological activity influence soil biogeochemical cycles (Tecon and Or, 2017). The contribution of soil organic matter to different aggregate fractions and details of how organic C is organized within aggregates are of critical importance to the self-organization of microbial communities. Besides, only a small percentage of total microbial biomass found in soil is active; most is dormant or inactive (Blagodatskaya and Kuzyakov, 2013; Tecon and Or, 2017). As one way to obtain such data, TEM can identify activity of microflora observed within aggregates, revealing their state and their enzymatic potential. To confirm the nature of organic matter, it can be stained to enhance proteins, lipids or polysaccharides (Foster, 1981; Bisdom, 1983) and differentiate microbial from plant polysaccharrides (Tiessen and Stewart, 1988). Furthermore, microaggregate turnover is high, as the rate of organic matter degradation by microorganisms is related to microhabitats. As shown, combining TEM and NanoSIMS is one promising way to identify this turnover (Vidal et al., 2016) and will add microbial information to the imaging of organic matter dynamics using NanoSIMS and SEM (Mueller et al., 2012). Likewise, combining soil organic matter fractionation and TEM typology of organo-mineral associations will likely become a promising method for confirming the biochemical composition of extracted organic matter regardless of the fractionation method used. It can determine the nature (polyphenolic, ligneous, EPS) of the organic matter observed according to its identified biochemical recalcitrance, in particular the presence of microbial components.
A combination of TEM and other techniques (e.g., EELS, EDX, NanoSIMS) can observe the contribution within soil of microorganisms to organic matter dynamics and biogeochemical cycles of several elements. TEM data on soil physico-chemical characterization or microbial diversity and abundance will improve knowledge about microbial contribution to soil processes. As part of this approach, combining FISH and immuno-labeling at the micrometer scale appears to be a promising way to carry out future research. Similarly, immunomarking techniques could be developed to detect of viruses within aggregates. Currently, in situ visualization of virus particles within undisturbed soil samples is far from obvious. Thus, direct counting methods using TEM or epifluorescence microscopy have relied on a two-step process of extraction and enumeration (Williamson et al., 2017). TEM allows for visualization of viral morphology and epifluorescence microscopy is more used for viral enumeration. Ashelford et al. (2003) reported that substantial populations of soil bacteriophage exist in soils. Considering the impact that viruses can have on the bacterial cycle and thus on the nutrient cycle, identifying viruses within soil microstructures may prove relevant in specifying carbon dynamics as a function of bacterial population dynamics.
Soil-Microorganism Interactions at Hotspots of Biological Activity
The rhizosphere is a soil hotspot, as microbial interactions impact soil functioning. We studied soil aggregation as a function of increasing distance from roots in soil 4 (Watteau et al., 2006). Coarse and fine roots at the soil interface generate stable 2–20 μm aggregates, whose organization depends on the nature of the plant or microbial organic matter. Distribution of aggregate types varies according to their proximity to roots (Figure 2). Microaggregates near roots contain mainly root cell walls and bacteria, whereas those further from roots contain mainly polyphenolic substances and bacterial aggregate residues. These results agree with the conceptual model of soil organic C dynamics under long-term maize cropping systems, in which C is distributed among four pools with different biodegradation kinetics (Balesdent et al., 2000; Ludwig et al., 2003).
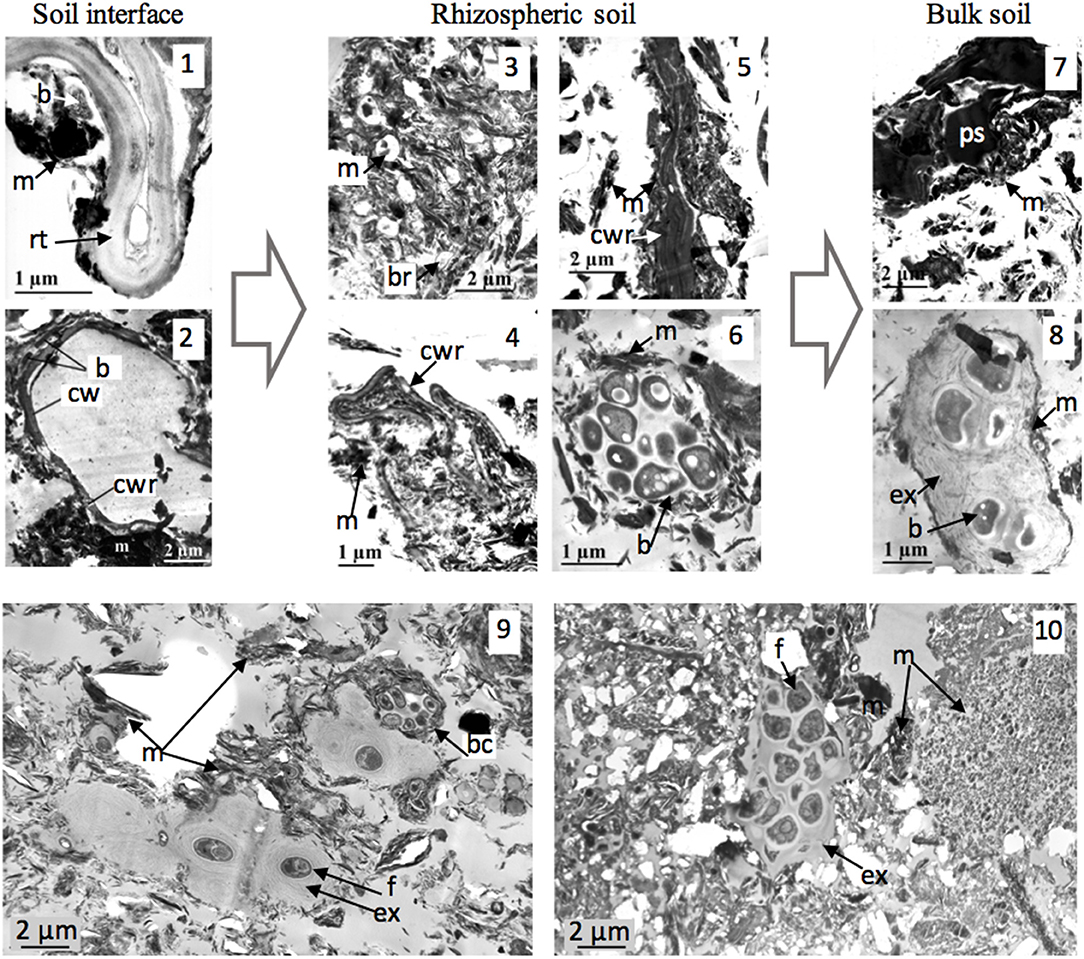
Figure 2. Soil-microorganism interactions at hotspots of biological activity. TEM views (1–8) (soil 4): microaggregation from maize root soil interface (1, 2) to rhizospheric soil (3–6) and to bulk soil (7, 8) (issue from Watteau et al., 2006). (1) Fine root-soil interface; (2) coarse root-soil interface; (3, 4) degradation of root cell walls of fine root forming aggregates; (5) microaggregate with electron-dense root cell wall; (6) bacterial aggregate; (7) microaggegate with electron-dense cell residue; (8) old bacterial aggregate. TEM views (9, 10): EPS as aggregation agents from bacterial and fungal origin in soil 3 (views 9) and in soil 7 (view 10). b, bacteria; bc, bacterial colony; br, bacterial residue; cwr, cell wall residue; ex, EPS; m, mineral; ps, polyphenolic substance; rt, root tissue.
Describing the rhizosphere with TEM enhanced the role of microbial EPS in forming organo-mineral associations in such coarse-textured soil. Bacteria adhered to roots because of EPS, on which minerals were aggregated and EPS are the main components of frequently observed bacterial aggregates (Figures 1, 2). TEM visualization identified the involvement of EPS in microbial aggregation in the main soils, i.e., the cropped soils and the Technosol (Figures 1.2–1.4, 2.9, 2.10). EPS are thus a hotspot of soil-microorganism interactions, especially since they resist degradation. It is well-known that stable EPS influence soil structure dynamics, binding particles together and promoting the formation of a stable structure (Chenu and Guerif, 1993). EPS optimize functions at the microsite scale (Abiven et al., 2007), such as water availability in micropores (Rossi et al., 2012). Bacterial polysaccharride-mediated aggregation has been widely studied, particularly using SEM (Chaney and Swift, 1986; Dorioz et al., 1993). Thus, TEM could identify the contribution of EPS in most soils, even those as coarsely-textured as young Technosols, in which biofilms may predominate during pedogenesis, by analogy with biological crust development (Malam Issa et al., 2007; Weber et al., 2016).
Over short time scales, microbial interactions at hotspots (e.g., rhizosphere and aggregates) impact the soil physical environment greatly (Philippot et al., 2013). In this way, composts can also be considered as hotspots. Composts are added as a soil amendment or as parent materials at remediation sites (soil 7). Composted organic matter is expected to be biochemically and microbially stable because of its production process. However, TEM characterization of green-waste composts showed that they also have a microbial potential (Séré et al., 2010; Watteau and Villemin, 2011). Observation of spores or active microflora in identified organic residues indicates both enzymatic potential and dynamics of composted organic matter and associated microorganisms, as these composts will be added to the soil. TEM identification of the microbial potential of organic residues incorporated in the soil is particularly relevant for site remediation. Green-waste composts are currently used as organic parent materials of Technosols (Bacholle et al., 2006), and paper-mill sludge is incorporated in large amounts throughout the entire soil depth (120 cm) (soil 7; Séré et al., 2010). As both residues can also contain bacteria and fungi, it is interesting to note that pedological engineering creates soil microhabitats, even deep in the soil and during soil construction (Watteau et al., 2018). TEM characterization of parent material-microorganism interactions at the micrometer scale can help monitor Technosol functioning. This is of great interest, as Technosols are increasingly used in new ecosystems. By definition, biofeatures (e.g., earthworm casts, burrows, fecal pellets) are also hotspots of microbial activity. TEM characterization of biofeatures indicates the ability of fauna to transform ingested organic matter before it is incorporated in soils and to form new aggregates (Figure 1, Figure S1 (see Datasheet 2); Pey et al., 2014; Vidal et al., 2016]. TEM appears well suited to identify fauna-microorganism interactions in the context of soil functioning. Thus, TEM can identify microbial potential (i.e., presence, state, and enzymatic activity) and also biologically promoted aggregation, at hotspots of biological activity.
Microbial Aggregates as Soil Function Indicators
TEM can be used to characterize 2–20 μm stable soil fractions by comparing Vertisols under either former market gardens or former grassland (soil 5). TEM examination clearly shows that these size fractions consist mainly of microaggregates of three types [Figure S2, (see Datasheet 3)]:
- Type 1: organo-mineral, composed of plant cell residues associated with clays in various proportions. Microorganisms can colonize the organic residues.
- Type 2: bacterial, composed of bacteria producing EPS which were associated with clays.
- Type 3: mainly mineral, composed of clays aggregated by colloidal organic substances. EDX analyses confirmed the presence of organic matter by detecting osmium specifically bound to it [Figure S2 (see Datasheet 3), EDX spectrum 1].
The relative proportions of these three types of microaggregates within the 2–20 μm fractions can be assessed for both Vertisols. Thirty naked-eye counts of 330 μm2 were performed for each, corresponding to 135 and 253 aggregates from the Vertisol under former market gardens and former grassland, respectively. Whereas mineral aggregates predominate under former market gardens, bacterial and organo-mineral aggregates are more numerous under former grassland. These contrasting morphological descriptions may be related to other differing soil parameters, such as the soil stability index (AS500, the percentage of stable aggregates >500 μm after 18 h of stirring in water) and soil C content (Blanchart et al., 2000). AS500 is four times as high under former grassland (16%) compared to Vertisol under former market gardens (4%), and soil C content is almost three times as high under former grassland (39 g.kg−1) than under former market gardens (14 g.kg−1). Biological aggregates (bacterial and organo-mineral) predominate in stable situations (93%), whereas mineral aggregates predominate in erodible situations (67%). Moreover, this is also related to the measured biological activity, as the biomass of roots, the abundance of earthworms and microorganisms in these soils are lower than those in market gardens (Chotte et al., 1992; Blanchart et al., 2000). This clear relationship between microaggregate type and Vertisol stability under various crops was also verified for four other cropping situations [bare soil, sugar cane (Saccharum spp.), meadow, fallow land] of calcic Vertisols in Guadeloupe (Blanchart et al., 2000). Biological aggregates due to soil enrichment by plant debris and increased microbial activity in reclamation situations influence soil structural stability. The relative proportions of these three types of aggregates consequently appear to indicate a sensitivity of these soils to erosion, and the typology of aggregates in the 2–20 μm fractions can be used as an indicator of impacts of cropping practices.
Micrometer characterization of these fractions is thus well suited to identify microhabitats in relation to soil use. Others studies highlighted using the same approach to examine impacts of biological activity (by roots and microorganisms) on the stability and composition of 0–20 μm aggregates in a maize-cropped soil (see section Soil-Microorganism Interactions at Hotspots of Biological Activity), the relationship between soil stability and the nature of aggregated organic matter from plants and microorganisms (Watteau et al., 2012) and the impact of crop rotation on soil structure (Saad et al., 2017). The biochemistry and turnover of organic matter are closely related to the size of organic matter and its associations with mineral soil components, and thus influence aggregate stability (Oades, 1984; Tiessen and Stewart, 1988). We thus confirm that microaggregate type results from, and is an indicator of, soil biofunctioning.
Potential Contribution of TEM to Soil Process Quantification and Modeling
As shown, morphological and analytical characterization by TEM provides much qualitative information about microhabitats in relation to soil processes. It can identify soil-microorganism interactions by visualizing biota in situ while respecting their habitat in the state it has at the time of sampling. Data on the contribution of microorganisms to organic matter dynamics and biogeochemical cycles of main elements are available regardless of the soil compartment (e.g., root interface, undisturbed aggregates, size fractions, faunal dejections). The 0–20 μm soil fractions appear to indicate soil functioning according to soil use or assessment of spatiotemporal heterogeneity. This would provide an opportunity to scale up and use the experimental data obtained at the micrometer scale to take impacts of microorganisms on soil functioning into account at the soil profile or even plot scale. Thus, TEM can provide data for soil process modeling, especially as using or developing image analysis protocols can also yield quantitative results by obtaining representative samples and statistically analyzing the number of counts performed. Future research could focus on the feasibility of using image analysis to quantify certain microbial descriptors indicating the presence or activity of microorganisms in soil fractions (e.g., proportion of biological aggregates, number or size of bacterial aggregates, number of free microorganisms, area of organic matter lysed). Thus, meta-analysis may identify correlations between certain quantified descriptors and other soil characteristics depending on the process studied, then identify the best microhabitat parameters to serve as indicators of soil functioning. Moreover, the entire aggregate could be extrapolated by analyzing many TEM images and mathematically constructing a 3D soil structure from 2D data (e.g., Wu et al., 2006). These descriptors could then be used as input parameters of soil process models, as could the amount and C content of 0–20 μm organo-mineral fractions. Mechanistic representation of small-scale processes is one of the priorities for improving models of soil organic matter dynamics (Manzoni et al., 2009). Microbial degradation and competition are increasingly simulated in models of structured environments and microtomography provides information about soil-fungi interactions (Monga et al., 2014). However, TEM is most relevant for soil-bacteria interactions. Future research combining TEM with other visualization techniques and analyses, may provide information and quantitative parameters relevant for simulating bacterial contributions to soil processes in 2D and 3D models. Characterization of the pore network may become a relevant indicator of soil functioning, while that of aggregation already seems to be one (Rabot et al., 2018). In addition to the micropore-microorganisms interactions already studied (Vos et al., 2013), we recommend using TEM to characterize microaggregates, and particularly microbial interactions within them, as potential soil health indicators. Soil health reflects ecological attributes of the soil for producing a particular crop, encompassing biodiversity, food web structure, soil biotic activity and the range of functions it performs (Pankhurst et al., 1997 in Bünemann et al., 2018). Micron-scale interactions must be considered in macroscale processes (Baveye, 2018). In this way, micrometer-scale soil studies aim to input microhabitat parameters in soil process models, thereby facilitating simulation of microbial dynamics in their organo-mineral environment.
Author Contributions
All authors listed have made a substantial, direct and intellectual contribution to the work, and approved it for publication.
Conflict of Interest Statement
The authors declare that the research was conducted in the absence of any commercial or financial relationships that could be construed as a potential conflict of interest.
Acknowledgments
The authors thank A. Vidal (UPMC, Paris) and T. Morvan (SAS, Rennes) for allowing us to use unpublished photos obtained as part of collaborative research efforts (Figure 1).
Supplementary Material
The Supplementary Material for this article can be found online at: https://www.frontiersin.org/articles/10.3389/fenvs.2018.00106/full#supplementary-material
Table S1. Main physico-chemical characteristics of the 2 mm sieved topsoils.
Figure S1. Microbial degradation of organic matter within soil microstructures. TEM view 1: degradation of ligneous residue issue from cattle manure added to soil 2, showing lysis area due to microbial enzymatic activity. TEM view 2 (soil 6): weathered features of polyphenolic substances contained in mycorrhizal beech root, showing area of lysis due to fungal activity, emphasized by N enrichment detected using EELS spectroscopy: EEL detection of C in the less-electron dense area of the polyphenolic substances (spectrum 1) and EEL detection of C and N in the contrasting ring around the less-electron dense area of the polyphenolic substances (spectrum 2) (already published in Watteau et al., 2002).
TEM view 3 (soil 3): cast microaggregates of Lumbricus terrestris and NanoSIMS maps of 12C14N and δ13C highlighting the incorporation of labeled elements in fungi and bacteria (already published in Vidal et al., 2016). b, bacteria; bc, bacterial colony; ci, cell intersection; cw, cell wall; cwr, cell wall residue; f, fungi; fr, fungal residue; h, hypha; la, lysis area; lt, ligneous thickening; L, pure silica layer surrounding polyphenolic substances; m, mineral; ps, polyphenolic substance; q, microquartz; r, contrasted ring; s, spore; *1 and *2, localization of EEL analyses corresponding to EEL spectra 1 and 2.
Figure S2. Microaggregates of Vertisol. TEM view 1: 2–20 μm fraction presenting the 3 types of microaggregates; TEM view 2: “organo-mineral” aggregate; TEM view 3: bacterial aggregate; TEM view 4: “mineral” aggregate and corresponding EDX spectrum 1 and 2 for respectively fine organic matter (presence of C and Os) and clay.
b, bacteria; ba, bacterial aggregate; cw, plant cell wall; ex, exopolymer; ma, mineral aggregate; m, mineral; om, organic matter; oma, organo-mineral aggregate; pr, plant residue; *1 and *2, localization of EDX analysis 1 and 2.
References
Abiven, S., Menaserri, S., Angers, D. A., and Leterme, P. (2007). Dynamics of aggregate stability and biological binding agents during decomposition of organic materials. Eur. J. Soil Sci. 58, 239–247. doi: 10.1111/j.1365-2389.2006.00833.x
Anderson, D. W., Saggar, S., Bettany, J. R., and Stewart, J. W. B. (1981). Particle size fractionation and their use in studies soil organic matter: I. the nature and distribution of forms of carbon, nitrogen and sulfur. Soil Sci. Soc. Am. J. 45, 767–772. doi: 10.2136/sssaj1981.03615995004500040018x
Ashelford, K. E., Day, M. J., and Fry, C. (2003). Elevated abundance of bacteriophage infecting bacteria in soil. Appl. Environ. Microbiol. 69, 285–289. doi: 10.1128/AEM.69.1.285-289.2003
Bacholle, C., Leclerc, B., and Coppin, Y. (2006). Utilisation des produits organiques en reconstitution de sol – Inventaire des pratiques en France; Etat de l'art des connaissances liées aux impacts de ces pratiques. Rapport ADEME. 1–135.
Balabane, M., and Plante, A. F. (2004). Aggregation and carbon storage in silty soil using physical fractionation techniques. Eur. J. Soil Sci. 55, 415–427. doi: 10.1111/j.1351-0754.2004.0608.x
Balesdent, J., Chenu, C., and Balabane, M. (2000). Relationship of soil organic matter dynamics to physical protection and tillage. Soil Tillage Res. 53, 215–230. Available online at: http://citeseerx.ist.psu.edu/viewdoc/download?doi=10.1.1.458.7739&rep=rep1&type=pdf
Bartoli, F., Demai, J. J., Philippy, R., Gueniot, B., Hatira, A., Villemin, G., et al. (1986). Structure de matières organiques de sols en microscopie électronique à transmission. Soil Biol. Bioch. 18, 497–505. doi: 10.1016/0038-0717(86)90007-6
Bauer, R. (1988). Electron spectroscopic imaging: an advanced technique for imaging and analysis in transmission electron microscopy. Methods Microbiol. 20, 113–146. doi: 10.1016/S0580-9517(08)70050-3
Baveye, C. P. (2018). Book review: shifting paradigms on soil microbial biomass. Front. Environ. Sci. 6:10. doi: 10.3389/fenvs.2018.00010
Baveye, P. C., Otten, W., Kravchenko, A., Balseiro Romero, M., Beckers, É, Chalhoub, M., et al. (2018). Emergent properties of microbial activity in heterogeneous soil microenvironments: different research approaches are slowly converging, yet major challenges remain. Front. Microbiol. 8:1364. doi: 10.3389/fmicb.2018.01929
Bisdom, E. B. A. (1983). Submicroscopic examination of soils. Adv. Agron 36, 55–96. doi: 10.1016/S0065-2113(08)60352-1
Blagodatskaya, E., and Kuzyakov, Y. (2013). Active microorganisms in soil: critical review of estimation criteria and approaches. Soil Biol. Biochem. 67, 192–211. doi: 10.1016/j.soilbio.2013.08.024
Blanchart, E., Achouak, W., Albrecht, A., Barakat, M., Bellier, G., Cabidoche, Y. M., et al. (2000). Déterminants biologiques de l'agrégation dans les vertisols des Petites Antilles. Conséquences sur l'érodibilité des sols. Etude et Gestion des Sols 7, 309–328.
Bossuyt, H., Six, J., and Hendrix, P. F. (2005). Protection of soil carbon by microaggregates within earthworm casts. Soil Biol. Bioch. 37, 251–258. doi: 10.1016/j.soilbio.2004.07.035
Bünemann, E. K., Bongiorno, G., Bai, Z., Creamer, R. E., De Deyn, G., De Goede, R., et al. (2018). Soil quality - A critical review. Soil Biol. Biochem. 120, 105–125. doi: 10.1016/j.soilbio.2018.01.030
Campbell, C. A., Bierderbeck, V. O., McConkey, B. G., Curtin, D., and Zentner, R. P. (1999). Soil quality - Effect of tillage and fallow frequency. Soil organic matter quality as influenced by tillage and fallow frequency in a silt loam in southwestern Saskatchewan. Soil Biol. Biochem. 31, 1–7. doi: 10.1016/S0038-0717(97)00212-5
Castorena, E. V. G., Gutierrez-Castorena, M. C., Vargas, T. G., Bontemps, L. C., Delgadillo Martinez, J., Suastegui Mendez, E., et al. (2016). Micromapping of microbial hotspots and biofilms from different crops using digital image mosaics of soil thin sections. Geoderma 279, 11–21. doi: 10.1016/j.geoderma.2016.05.017
Chaney, K., and Swift, R. S. (1986). Studies on aggregate stability. I. Re-formation of soil aggregates. J. Soil Sci. 37, 329–335. doi: 10.1111/j.1365-2389.1986.tb00035.x
Chenu, C., and Cosentino, D. (2011). “Microbial regulation of soil structural dynamics,” in The Architecture and Biology of Soils: Life in Inner Space, ed K. Ritz and I. Young (Croydon: CPI Group), 37–70.
Chenu, C., and Guerif, J. (1993). Mechanical strength of clay minerals as influenced by an adsorbed polysaccharide. Soil Sci. Soc. Am. J. 55, 1076–1080. doi: 10.2136/sssaj1991.03615995005500040030x
Chotte, J. L., Jocteur-Monrozier, L., Villemin, G., and Toutain, F. (1992). Effet du mode de dispersion du sol sur la localisation de sa biomasse microbienne; cas d'un vertisol. Cahier de l'Orstom, Pédologie 27, 81–95.
Dorioz, J. M., Robert, M., and Chenu, C. (1993). The role of roots, fungi and bacteria on clay particle organization. An experimental approach. Geoderma 56, 179–194. doi: 10.1016/0016-7061(93)90109-X
Egerton, R. F. (1986). Electron Energy Loss Spectroscopy in the Electron Microscope. New York, NY: Plenum Press.
Eickhorst, T., and Tippkötte, R. (2008). Detection of microorganisms in undisturbed soil by combining fluorescence in situ hybridization (FISH) and micropedological methods. Soil Biol. Biochem. 40, 1284–1293. doi: 10.1016/j.soilbio.2007.06.019
Eliott, E. T. (1986). Aggregate structure and carbon, nitrogen and phosphorus in native and cultivated soils. Soil Sci. Soc. Am. J. 50, 627–633. doi: 10.2136/sssaj1986.03615995005000030017x
Foster, R. (1981). Polysaccharides in soil fabrics. Science 214, 665–667. doi: 10.1126/science.214.4521.665
Foster, R. C. (1988). Microenvironments of soil microorganisms. Biol. Fert. Soils 6, 189–203. doi: 10.1007/BF00260816
Foster, R. C., and Martin, J. K. (1981). “In situ analysis of soil components of biological origin,” in Soil Biochemistry, Vol. 5, eds E. A. Paul and J. N. Ladds (New York, NY: Marcel Dekker), 75–112.
Foster, R. C., Rovira, A. D., and Cock, T. W. (1983). Ultrastructure of the Root-Soil Interface. St Paul, MN: American Phytophathological Society.
Guggenberger, G., Elliott, E. T., Frey, S. D., Six, J., and Paustian, K. (1999). Microbial contributions to the aggregation of a cultivated grassland soil amended with starch. Soil Biol. Biochem. 31, 407–419. doi: 10.1016/S0038-0717(98)00143-6
Hassink, J. (1997). The capacity of soils to preserve organic C and N by their association with clay and silt particles. Plant Soil 191, 77–87. doi: 10.1023/A:1004213929699
Hattori, T., and Hattori, R. (1976). The physical environment in soil microbiology: an attempt to extend principles of microbiology to soil microorganisms. Crit. Rev. Microbiol. 4, 423–461. doi: 10.3109/10408417609102305
Hinsinger, P., Gobran, G. R., Gregory, P. J., and Wenzel, W. W. (2005). Rhizosphere geometry and heterogeneity arising from root-mediated physical and chemical processes. New Phytol. 168, 293–303. doi: 10.1111/j.1469-8137.2005.01512.x
Jouquet, P., Zangerle, A., Rumpel, C., Brunet, D., Bottinelli, N., and Tran Duc, T. (2009). Relevance and limitations of biogenic and physicogenic classification: a comparison of approaches for differentiating the origin of soil aggregates. Eur. J. Soil Sci. 60, 1117–1125. doi: 10.1111/j.1365-2389.2009.01168.x
Kilbertus, G. (1980). Etude des microhabitats contenus dans les agrégats du sol, leur relation avec la biomasse bactérienne et la taille des procaryotes présents. Rev. Ecol. Biol. Sols 17, 543–557.
Ladd, J. N., Foster, R. C., and Skjemstad, J. O. (1993). Soil structure: carbon and nitrogen metabolism. Geoderma 56, 401–434. doi: 10.1016/0016-7061(93)90124-4
Ludwig, B., John, B., Ellerbrock, R., Kaiser, M., and Flessa, H. (2003). Stabilization of carbon from maize in a sandy soil in a long-term experiment. Eu. J. Soil Sci. 54, 117–126. doi: 10.1046/j.1365-2389.2003.00496.x
Lynch, J. M. (1990). “Introduction: some consequences of microbial rhizosphere competence for plant and soil,” in The Rhizosphere, ed J. M. Lynch (Chichester: John Whiley & Sons), 1–10.
Malam Issa, O., Défarge, C., Le Bissonnais, Y., Marin, B., Duval, O., Bruand, A., et al. (2007). Effects of the inoculation of cyanobacteria on the microstructure and the structural stability of a tropical soil. Plant Soil 290, 209–219. doi: 10.1007/s11104-006-9153-9
Manzoni, S., Schimel, J. P., and Porporato, A. (2009). Responses of soil microbial communities to water stress: results from a meta-analysis. Ecology 93, 930–938. doi: 10.1890/11-0026.1
Monga, O., Garnier, P., Pot, V., Coucheney, E., Nunan, N., Otten, W., et al. (2014). Simulating microbial degradation of organic matter in a simple porous system using the 3-D diffusion-based model MOSAIC. Biogeosciences 11, 2201–2209. doi: 10.5194/bg-11-2201-2014
Mueller, C. W., Kölbl, A., Hoeschen, C., Hillon, F., Heister, K., Herrmann, A. M., et al. (2012). Submicron scale imaging of soilorganic mater dynamics using NanoSIMS – From single particles to intact aggregates. Org. Geochem. 42, 1476–1488. doi: 10.1016/j.orggeochem.2011.06.003
Nunan, N., Ritz, K., Crabb, D., Harris, K., Wu, K., Crawford, J. W., et al. (2001). Quantification of the in situ distribution of soil bacteria by large scale imaging of thin sections of undisturbed soil. FEMS Micro. Ecol. 37, 67–77. doi: 10.1111/j.1574-6941.2001.tb00854.x
Oades, J. M. (1984). Soil organic matter and structural stability: mechanisms and implications for management. Plant Soil 76, 319–337. doi: 10.1007/BF02205590
Pankhurst, C. E., Doube, B. M., and Gupta, V. V. S. R. (eds.). (1997). “Biological indicators of soil health: synthesis,” Biological Indicators of Soil Health (Wallingford: CAB International), 419–435.
Pey, B., Cortet, J., Capowiez, Y., Nahmani, J., Watteau, F., and Schwartz, C. (2014). Technosol composition affects Lumbricus terrestris surface cast composition and production. Ecol. Eng. 67, 238–247. doi: 10.1016/j.ecoleng.2014.03.039
Philippot, L., Raaijmakers, J. M., Lemanceau, P., and van der Putten, W. H. (2013). Going back to the roots: the microbial ecology of the rhizosphere. Nat. Rev. Microbiol. 11, 789–799. doi: 10.1038/nrmicro3109
Rabot, E., Wiesmeier, M., Schlüter, S., and Vogel, H. J. (2018). Soil structure as an indicator of soil functions: a review. Geoderma 314, 123–137. doi: 10.1016/j.geoderma.2017.11.009
Ranjart, L., and Richaume, A. (2001). Mini-review: Quantitative and qualitative microscale distribution of bacteria in soil. Res. Microbiol. 152, 707–716. doi: 10.1016/S0923-2508(01)01251-7
Ritz, K. (2011). “In situ visualization of soil biota,” in The Architecture and Biology of Soils: Life in Inner Space, eds K. Ritz and I. Young (Croydon: CPI Group), 1–12.
Roose, T., Keyes, S. D., Daly, K. R., Carminati, A., Otten, W., Vetterlein, D., et al. (2016). Challenges in imaging and predictive modeling of rhizosphere processes. Plant Soil 407, 9–38. doi: 10.1007/s11104-016-2872-7
Rossi, F., Potrafka, R. M., Pichel, F. G., and De Philippis, R. (2012). The role of exopolysaccharides in enhancing hydraulic conductivity of biological soil crusts. Soil Biol. Biochem. 46, 33–40. doi: 10.1016/j.soilbio.2011.10.016
Saad, R. F., Kobaissi, A., Machinet, G., Villemin, G., Echevarria, G., and Benizri, E. (2017). Crop rotation associating a legume and the nicckel hyperaccumulator Alyssum murale improves the structure and biofunctioning of an ultramafic soil. Ecol. Res. 645, 380–392. doi: 10.1007/s11284-017-1526-4
Séré, G., Schwartz, C., Ouvrard, S., Renat, J. C., Watteau, F., Villemin, G., et al. (2010). Early pedogenic evolution of constructed Technosols. J. Soils Sediments 10, 1246–1254. doi: 10.1007/s11368-010-0206-6
Stotzky, G. (1986). “Influence of soil mineral colloids on metabolic processes, growth, adhesion and ecology of microbes and viruses,” in Interactions of Soils Minerals With Natural Organics and Microbes, ed P. M. Huang and M. Schnitzer (Madison, WI), 305–428.
Tecon, R., and Or, D. (2017). Biophysical processes supporting the diversity of microbial life in soil. FEMS Microbiol. Rev. 39, 599–623. doi: 10.1093/femsre/fux039
Tiessen, H., and Stewart, B. (1988). Light and electron microscopy of stained microaggregates: the role of organic matter and microbes in soil aggregation. Biogeochemistry 5, 312–322. doi: 10.1007/BF02180070
Tisdall, J. M., and Oades, J. M. (1982). Organic matter and water-stable aggregates in soils. J. Soil Sci. 33, 141–163. doi: 10.1111/j.1365-2389.1982.tb01755.x
Vidal, A. (2016). The fate of 13C labelled root and shoot litter in soil and earthworm casts: a multidisciplinary approach based on mesocosm experiment. Thesis, Pierre et Marie Curie University. Available online at: http://www.theses.fr/2016PA066264
Vidal, A., Remusat, L., Watteau, F., Derenne, S., and Quenea, K. (2016). Incorporation of 13C labelled shoot residues in Lumbricus terrestris casts: a combination of transmission microscopy and nanoscale secondary ion mass spectrometry. Soil Biol. Biochem. 93, 8–16. doi: 10.1016/j.soilbio.2015.10.018
Vos, M., Wolf, A. B., Jennings, S. J., and Kowalchuk, G. A. (2013). Micro-scale determinants of bacterial diversity in soil. FEMS Microbiol. Rev. 37, 936–954. doi: 10.1111/1574-6976.12023
Waisel, Y., Eshel, A., and Kafkafi, U. (2002). Plant Roots – The Hidden Half New York, NY; Basel: Marcel Dekker Inc., 1136.
Watteau, F., Huot, H., Séré, G., Begin, J. C., Rees, F., Schwartz, C., et al. (2018). Micropedology to reveal pedogenetic processes in technosols. SJSS. 8, 148–163. doi: 10.3232/SJSS.2018.V8.N2.02
Watteau, F., and Villemin, G. (2001). Ultrastructural study of the biogeochemical cycle of silicon in the soil and litter of a temperate forest. Eur. J. Soil Sci. 52, 385–396. doi: 10.1046/j.1365-2389.2001.00391.x
Watteau, F., and Villemin, G. (2011). Characterization of organic matter microstructure dynamics during co-composting of sewage sludge, barks and green waste. Bioresource Technol. 102, 9313–9317. doi: 10.1016/j.biortech.2011.07.022
Watteau, F., Villemin, G., Bartoli, F., Schwartz, C., and Morel, J. L. (2012). 0-20 μm aggregate typology based on the nature of aggregative organic materials in a cultivated silty topsoil. Soil Biol. Biochem. 4, 103–114. doi: 10.1016/j.soilbio.2011.11.021
Watteau, F., Villemin, G., Burtin, G., and Jocteur-Monrozier, L. (2006). Root impact on the stability and constitution of the fine organo-mineral associations in a maize cultivated soil. Eur. J. Soil Sci. 57, 247–257. doi: 10.1111/j.1365-2389.2005.00734.x
Watteau, F., Villemin, G., Ghanbaja, J., Genet, P., and Pargney, J. C. (2002). In situ ageing of fine beech roots (Fagus sylvatica) assessed by transmission electron microscopy and electron energy loss spectroscopy: description of microsites and evolution of polyphenolic substances. Biol. Cell 94, 55–63. doi: 10.1016/S0248-4900(02)01182-6
Weber, B., Büdel, B., and Belnap, J. (eds.). (2016). “Biological soil crusts: an organizing principle in drylands,” in Ecological Studies (Springer), 3–541.
Williamson, K. E., Furhrmann, J. J., Wommack, K. E., and Radosevich, M. (2017). Viruses in soil ecosystems: an unknown quantity within an unexplored territory. Ann. Rev. Vir. 4, 201–219. doi: 10.1146/annurev-virology-101416-041639
WRB, J. (2014). World Reference Base for Soil Ressource. World Soil Resources Reports No. 106. Rome: FAO.
Keywords: bacteria, EPS, microhabitats, in situ localization, soil fractionation, micro-analyses, hotspots of biological activity
Citation: Watteau F and Villemin G (2018) Soil Microstructures Examined Through Transmission Electron Microscopy Reveal Soil-Microorganisms Interactions. Front. Environ. Sci. 6:106. doi: 10.3389/fenvs.2018.00106
Received: 28 February 2018; Accepted: 30 August 2018;
Published: 09 October 2018.
Edited by:
Philippe C. Baveye, AgroParisTech Institut des Sciences et Industries du Vivant et de L'Environnement, FranceReviewed by:
Kai Uwe Totsche, Friedrich-Schiller-Universität Jena, GermanyHannes Schmidt, Universität Wien, Austria
Copyright © 2018 Watteau and Villemin. This is an open-access article distributed under the terms of the Creative Commons Attribution License (CC BY). The use, distribution or reproduction in other forums is permitted, provided the original author(s) and the copyright owner(s) are credited and that the original publication in this journal is cited, in accordance with accepted academic practice. No use, distribution or reproduction is permitted which does not comply with these terms.
*Correspondence: Françoise Watteau, ZnJhbmNvaXNlLndhdHRlYXVAdW5pdi1sb3JyYWluZS5mcg==