- Swiss Centre for Applied Ecotoxicology Eawag, École Polytechnique Fédérale de Lausanne (EPFL), Dübendorf, Switzerland
Freshwater fungi are a diverse group of organisms and fulfill important functions in the food web dynamics of surface water ecosystems. Ascomycetic and basidiomycetic hyphomycetes play key roles in leaf litter breakdown in rivers and creeks, while parasitic chytrids are an important food source for small invertebrates in lakes. Field studies indicate that fungal communities are affected by fungicides at environmentally relevant concentrations. However, despite their ecological importance, freshwater fungi are currently not specifically addressed in the EU regulatory frameworks with respect to the protection of surface waters. Specifically, the prospective risk assessment of fungicides does not evaluate adverse effects on non-target aquatic fungi. This paper aims to describe important functions of freshwater fungi, provides an overview of adverse effect levels of fungicides on this organism group, and proposes to integrate the fungal community of freshwater ecosystems as an additional trophic level in the current fungicide risk assessment frameworks. Results of a literature review on the effects of fungicides on aquatic fungi revealed that information on the toxicity of fungicides to non-target aquatic fungi is limited. This is, in part, due to the lack of standardized bioassays using aquatic fungi as test species. Although there is an encouraging number of bioassays focusing on the degradation of dead organic material by hyphomycetes, studies on fungicide effects on other important ecological functions, like the control of algal blooms in lentic surface waters by parasitic chytrid fungi, or on mutualistic fungi living in the guts of aquatic arthropods are largely missing. Thus, the further development and standardized of different fungi bioassays is recommended.
Background
One of the most important anthropogenic hazards for the ecological health of freshwater ecosystems is the input of pesticides (biocides and plant production products) via point sources such as wastewater treatment plants (mainly biocides) as well as non-point sources, such as spray drift, drainage and run-off from agricultural fields (e.g., Petersen et al., 2013; Moschet et al., 2014). To protect the ecology of water bodies from adverse effects of plant protection products (PPP), a prospective risk assessment is conducted by the European Food Safety Authority (EFSA) prior to authorization of active ingredients and their formulated products. The EFSA guidance document (EFSA, 2013), requires toxicity data for three taxonomic groups: plants (e.g., algae, duckweed), invertebrates (e.g., cladoceran crustacea e.g., Daphnia magna) and a fish species, representing a simplified food chain consisting of primary producers, primary consumers, and secondary consumers. Similar approaches are used for the authorization of biocides (European Chemicals Agency, 2015) as well as for deriving environmental quality standards (EQS) for retrospective risk assessment under the EU Water Framework Directive (WFD, EU 2000).
The most recent version of the EFSA guidance document (EFSA, 2013) acknowledges that studies by Maltby et al. (2009); Bundschuh et al. (2011); Dijksterhuis et al. (2011), and Zubrod et al. (2015a) give reason for concern that the current data requirements for ecological risk assessment does not adequately consider the risk of fungicides for aquatic fungi. In addition, recent studies suggest that aquatic fungi are particularly sensitive to ergosterol-inhibiting fungicides such as triazoles [Dijksterhuis et al. (2011), Dimitrov et al. (2014), Zubrod et al. (2015b) and references therein].
Freshwater fungi are a diverse group of organisms and fulfill important functions in the food web dynamics of surface water ecosystems. They play a key role in the breakdown of allochthonous (foreign to a certain environment) organic material such as twigs, leaves, etc. which provides up to 99% of the total energy input into surface waters (Teal, 1957; Nelson and Scott, 1962; Fisher and Likens, 1973; Bärlocher and Kendrick, 1974). The colonization of organic material by microorganisms and aquatic fungi therefore represents an essential component of the food web of running waters. Due to the large diversity of fungi as well as the scarcity of toxicity data for relevant fungal species EFSA identified the development of standardized ecotoxicity assays as a future research need (EFSA, 2013). Such data are also needed for the derivation of EQS for fungicides under the WFD, which aim at protecting the most sensitive taxonomic groups. Without data on the sensitivity of aquatic fungi, higher assessment factors have to be applied1. An overview on considering aquatic fungi in fungicide risk assessment under different regulatory frameworks can be found in Supplementary Data Sheet 1. It shows that fungal bioassays focussing on ecosystem functioning as well as on community structure are needed.
This paper provides an overview on the current classification and ecology of fungi in freshwater ecosystems, addresses fungicide exposure in surface waters, and reviews current information on the effects of organic fungicides on freshwater fungi. Inorganic fungicides such as copper were not considered. Information on the effect of copper and other heavy metals can be found elsewhere (e.g., Duddridge and Wainwright, 1980; Jaeckel et al., 2005b; Pascoal et al., 2005; Azevedo et al., 2007; Roussel et al., 2008; Solé et al., 2008; Sridhar et al., 2008; Zubrod et al., 2015a). Furthermore, relevant taxonomic groups are recommended for bioassay development or improvement.
Biodiversity of Fungi in Freshwater Ecosystems
Within the domain Eukaryota, fungi represent their own kingdom (Figure 1) and are hence on the same taxonomic level as animals, plants and protists (Woese and Fox, 1977; Woese et al., 1990). Over the last few decades, the taxonomy of fungi has changed considerably as a consequence of genetic analyses (Voigt and Kirk, 2011), and a fungal tree of life was generated by Lutzoni et al. (2004), James et al. (2006), and Hibbett et al. (2007), whose taxonomy is used in this paper.
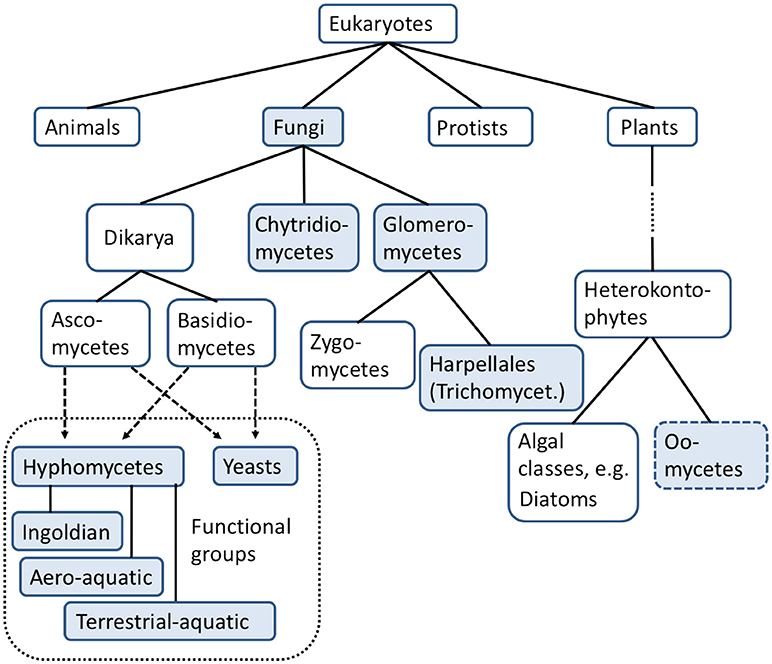
Figure 1. Taxonomic position of aquatic fungi in relation to current standard test organisms (fungal taxonomy based on Hibbett et al., 2007; Wurzbacher et al., 2010; Krauss et al., 2011). Taxonomic groups with filled boxes are subject of this review.
The total number of fungal species is estimated at 1.5 Million (Hawksworth 1991, 2001), while only approximately 7% of these species have been described (Mueller and Schmit, 2007). About 3,000 fungal species and 138 non-fungal oomycetes have been reported to be present in aquatic habitats. The greatest biodiversity of these groups was described for temperate areas (Shearer et al., 2007). Goh and Hyde (1996) reported over 600 freshwater species, consisting of ca. 300 ascomycetes, 300 mitosporic fungi, and a number of chytridiomycetes and non-fungal oomycetes. It can be assumed that just a small fraction of the aquatic fungal community has been described so far and that the number of newly discovered species will increase rapidly (Goh and Hyde, 1996; Shearer et al., 2007; Voigt and Kirk, 2011).
Various classifications of freshwater fungi exist (Goh and Hyde, 1996; Wong et al., 1998; Shearer et al., 2007). Most of the species living in freshwater habitats have been ascribed to the phyla ascomycetes, basidiomycetes, chytridiomycetes, and glomeromycetes (Shearer et al., 2007). The latter includes the zygomycetes, which formerly formed their own phylum (Hibbett et al., 2007). Wurzbacher et al. (2010) and Krauss et al. (2011) used a classification that focuses more on their functional traits in freshwater ecosystems rather than on phylogeny. Since this focus is beneficial for characterizing the effects of fungicides in freshwater ecosystems, their classification is adopted for this review. They proposed the following four main groups: (1) aquatic hyphomycetes (also called freshwater hyphomycetes or Ingoldian fungi), (2) chytridiomycetes (also called chytrids), (3) yeasts, and (4) glomeromycetes. While the majority of these groups can be regarded as being monophyletic, the aquatic hyphomycetes mainly belong to the ascomycetes with a small proportion in the basidiomycetes. Also, yeasts represent a polyphyletic group consisting of ascomycetes and basidiomycetes (Shearer et al., 2007). The differentiation between hyphomycetes and yeasts is hence mainly determined by their different morphology. The oomycetes (5) are treated as an additional but separate group (Shearer et al., 2007), since they are non-fungal from a taxonomical point of view. Their consideration for this review nonetheless is reasonable because they occupy similar niches as aquatic fungi and fulfill fungal-like ecological functions in freshwater ecosystems (Wong et al., 1998). The five functional fungal or fungal-like groups are described in more detail below:
(1) Aquatic hyphomycetes probably represent the most well-studied group and are reported to be part of freshwater ecosystems all over the world (Wong et al., 1998). Traditionally, they are distinguished into two groups based on their biological behavior (Goh and Hyde, 1996): (i) the Ingoldian fungi which are characterized by their ability to sporulate under water, and (ii) the aero-aquatic fungi which do not accomplish their whole life cycle under water, needing air exposure for reproduction (Wurzbacher et al., 2010). Goh and Hyde (1996, and references therein), further discern the (iii) submerged-aquatic hyphomycetes which are regarded as “facultative-aquatic,” since they do not sporulate primarily under water. All these hyphomycete groups are commonly found on submerged plant material (e.g., leaves, twigs, wood, etc.). Finally, there are also terrestrial-aquatic hyphomycetes, (e.g., occurring in rain drops associated with intact terrestrial plant material such as leaf surfaces) but since their habitat is outside aquatic ecosystems they are not considered any further.
(2) The chytridiomycetes are also a well-documented group (Wong et al., 1998), but little is known about their ecology (Gleason et al., 2008). They commonly are parasitic or saprotrophic and typically occur in the pelagic zone of stagnant waters (Wurzbacher et al., 2010).
(3) Yeasts are a ubiquitous fungal-group found virtually everywhere in freshwater ecosystems, especially in the pelagic zone of lakes (Wurzbacher et al., 2010). Despite several studies on aquatic yeasts the knowledge about their ecology is generally limited (Ahearn et al., 1968; Wurzbacher et al., 2010), and there exists no comprehensive analysis on yeast ecology and their role in freshwater ecosystems.
(4) The glomeromycetes also represent a group for which little is known regarding their occurrence and ecology in freshwater environments (Goh and Hyde, 1996). Most species of this group are terrestrial (Shearer et al., 2007). An exception are the trichomycetes which live parasitically or mutualistically (mutual advantages for both partners) in the digestive tract of aquatic arthropods (Shearer et al., 2007; Hernández Roa et al., 2009; Jobard et al., 2010). The trichomycetes are considered to be a polyphyletic group (Hibbett et al., 2007), partially belonging to the protists (Benny and O'Donnell, 2000; Cafaro, 2005). The trichomycete order harpellales, for which mutualistic species have been reported (Jobard et al., 2010), is considered to belong to the glomeromycetes (Hibbett et al., 2007).
(5) The non-fungal oomycetes are well-documented (Wong et al., 1998) and among the most ubiquitous aquatic microbes on earth (Shearer et al., 2007). The majority of species in this group lives saprotrophically, whereas some of them are animal parasites (e.g., on fish and crustaceans) or plant pathogens (Shearer et al., 2007). New research suggests that oomycetes are taxonomically related to certain algae such as phaeophytes (brown algae) or bacillariophytes (diatoms), showing their close affiliation with plants (Adl et al., 2005). According to (Voigt and Kirk, 2011) oomycetes are algae without chloroplasts but with cellulose in their cell walls (Figure 1).
Currently, different ways exist to identify aquatic fungi to the species level. For instance, Lin et al. (2012) identified aquatic fungi via the conidial morphology. A more innovative and future-oriented identification method is the determination by means of genetic studies, since results are more reliable and accurate (Krauss et al., 2011). Also, community fingerprinting techniques have proven useful to study the fungal diversity in microcosms [Krauss et al. (2011) and references therein].
Important Roles of Fungi and Oomycetes in Freshwater Ecosystems
Degradation of Dead Organic Material
A key function of aquatic fungi is the degradation of dead plant or other organic material (e.g., chitin, keratin; Figure 2). The decomposition of so called “standing-dead” emergent plants and submerged terrestrial plant litter (primarily leaves) by aquatic hyphomycetes in lentic and lotic waters respectively, plays a substantial role (Gessner et al., 2007) in the nutrient cycle of aquatic systems. While aero-aquatic fungi and yeasts predominantly occur on plant material of stagnant waters, ditches or slow-flowing streams under low to semi-aerobic conditions, the Ingoldian fungi are usually found in great numbers on submerged plant material (primarily leaves and twigs) in fast-flowing tree-lined streams and brooks and well-aerated lakes (Goh and Hyde, 1996; Wurzbacher et al., 2010). The submerged aquatic hyphomycetes prefer similar habitat conditions to the Ingoldian fungi, but are mostly detected on woody material (Goh and Hyde, 1996).
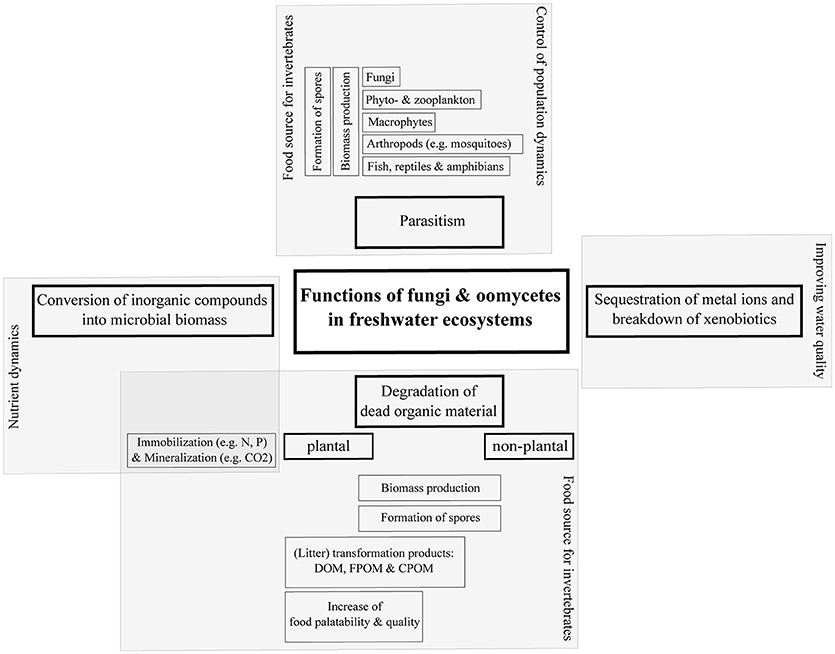
Figure 2. Direct and indirect functions of aquatic fungi and oomycetes in freshwater ecosystems. DOM, dissolved organic matter; FPOM, fine particulate organic matter; CPOM, coarse particulate organic matter.
The degradation of dead plant material results in the production of fungal biomass, the formation of reproductive spores, litter transformation products as dissolved organic matter (DOM) and fine particulate organic matter (FPOM). This process also increases the food quality for shredders (Cummins, 1974; Wong et al., 1998; Gessner et al., 1999, 2007) and food availability for other aquatic invertebrates. Since the input of allochthonous organic material (e.g., leaves, twigs, wood etc.) is considered the main energy source in low order forested streams (Teal, 1957; Nelson and Scott, 1962; Fisher and Likens, 1973; Bärlocher and Kendrick, 1974; Cummins, 1974)–exceeding the primary production in those waters—the degradation of dead plant material by aquatic fungi can be regarded as a critical component in the food web dynamics of these freshwater ecosystems. In addition, pollen and non-plant material is degraded mainly by chytridiomycetes and the non-fungal oomycetes (Goh and Hyde, 1996; Shearer et al., 2007; Gleason et al., 2008; Kagami et al., 2014; Wurzbacher et al., 2014), resulting in biomass and spores that can also be used as a food source by invertebrates.
Parasitism and Mutualism
The role of aquatic fungi (especially chytridiomycetes) and oomycetes as parasites in freshwater ecosystems is currently poorly understood. Fungal parasitism can greatly influence food supply, nutrient transfer and population dynamics in freshwater ecosystems (Kagami, 2008; Miki et al., 2011). Though parasitism is often not clearly distinguishable from mutualism (Jobard et al., 2010), there is evidence that both parasitic and mutualistic fungal species exist (Lichtwardt and Williams, 1999; Shearer et al., 2007; Strongman, 2007; Hernández Roa et al., 2009; Jobard et al., 2010). Examples of such mutualistic and/or parasitic fungi are the trichomycetes. They belong to the glomeromycetes and live in the guts of insects, crustaceans and millipedes (Fisher and Likens, 1973; Lichtwardt and Williams, 1999; Strongman, 2007). The knowledge about trichomycetes is scarce and thus their role and importance in food webs of aquatic ecosystems is still unclear (Jobard et al., 2010).
One of the most significant parasitism-host interactions is the association of parasitic chytridiomycetes with phytoplankton. On the one hand, chytridiomycetes, can serve as an important high-quality food source (polyunsaturated fatty acids, cholesterol) for zooplankton (e.g., daphnids) via biomass production (e.g., formation of zoospores; Müller-Navarra et al., 2000; Kagami et al., 2007; Miki et al., 2011). On the other hand, these fungi can control phytoplankton seasonal succession (Kagami et al., 2007; Miki et al., 2011), thereby preventing algal blooms. This demonstrates the importance of parasitic aquatic fungi in influencing population dynamics. Chytridiomycetes also represent a direct link between sinking, oversized, and hence non-accessible phytoplankton and filter-feeding zooplankton such as daphnids in the pelagic zone (Kagami et al., 2007; Jobard et al., 2010; Wurzbacher et al., 2010; Miki et al., 2011; Rasconi et al., 2014). Based on Kagami et al. (2007) and Kagami et al. (2014), this nutrient transfer between different trophic levels was termed “mycoloop,” and it underlines the significance of parasitic fungi as a crucial factor in food web dynamics of freshwater ecosystems.
Sequestration and Degradation of Xenobiotics and Nutrient Dynamics
From an ecotoxicological perspective, aquatic fungi can be important for the sequestration of heavy metal ions (e.g., cadmium, copper, zinc, lead) and the breakdown of organic xenobiotic compounds (e.g., nonylphenol, bisphenol A, 1-naphtol) in freshwater ecosystems (Jaeckel et al., 2005a; Augustin et al., 2006; Azevedo et al., 2007; Wurzbacher et al., 2010; Bärlocher et al., 2011; Krauss et al., 2011; Omoike et al., 2013; Lucas et al., 2016; Martínková et al., 2016; Oliveira et al., 2016). For example, aquatic fungi can sequester greater amounts of heavy metals than bacteria (Massaccesi et al., 2002), and outweigh bacteria in biomass (Findlay and Arsuffi, 1989). Recent studies showed that some fungi are able to degrade herbicides, insecticides (Oliveira et al., 2015) and even fungicides (Inoue et al., 2015). The ability to degrade and detoxify organic as well as inorganic pollutants suggests that aquatic fungi could play a role in the improvement of water quality and in biotechnological applications.
Fungi associated with decaying plant material (mainly aquatic hyphomycetes) directly influence the nutrient dynamics of freshwater ecosystems by mineralization of organic carbon to carbon dioxide (CO2) as well as by conversion of inorganic compounds, e.g., nitrogen (N) and phosphorus (P), into microbial biomass. For instance, chytridiomycetes are able to convert inorganic nitrogen, inorganic sulfur and inorganic phosphorus to organic compounds, which then can become available to heterotrophic organisms in ecosystems [Gleason et al. (2008) and references therein].
Exposure and Effects of Fungicides on Freshwater Fungi
Fungicides primarily enter surface waters via non-point sources such as agricultural runoff (e.g., Cruzeiro et al., 2015). Concentrations of fungicides in surface waters therefore fluctuate during the growing season, showing strong temporal and spatial variability (e.g., Rabiet et al., 2010; Bereswil et al., 2012; Moschet et al., 2014; Spycher et al., 2018). Two monitoring studies performed in 2012 in Switzerland and Norway provide insight into fungicide contamination in agriculturally influenced catchments over the course of an entire growing season. Moschet et al. (2014) showed that next to herbicides, fungicides were the second most abundant pesticides detected in medium sized rivers flowing through agricultural areas. Of 13 fungicides that were detected in at least three of five rivers, 7 (azoxystrobin, cyproconazole, carbendazim, tebuconazole, dimethomorph, propamocarb and metalaxyl-M) were detected in >70% of the samples analyzed, with maximum concentrations ranging from 18 ng/l (fenamidone) to 380 ng/l (metalaxyl-M). Petersen et al. (2013) detected at least one fungicide in 57% and >4 fungicides in 9% of 54 water samples. Detection frequency was highest in areas of potato and vegetable production (78%). Maximum fungicide concentrations ranged from 37 ng/l (imazalil) to 680 ng/l (fenamidone). In both studies, herbicides dominated in terms of detected active substances (77 and 58% of samples, respectively), however, the abundance of fungicides can equal or exceeded that of herbicides in catchments with a higher share of orchards and vineyards. This was shown by Kreuger et al. (2010) and Spycher et al. (2018). The latter measured a peak concentration of 6 μg/L fluopyram in a 0.5 day composite sample collected from a stream situated in a wine growing area near the shore of Lake Geneva. There, fungicides accounted for 64% of pesticides detected. Compared to herbicides which tend to be relatively water soluble, fungicides are rather lipophilic. The average octanol-water partition coefficient (logKow) for 45 fungicides detected by Spycher et al. (2018) is 3.4 (5th percentile = 1.8; 95th percentile = 4.7). The frequent occurrence of fungicides in agriculturally influenced streams along with their tendency to bind to organic matter suggest that aquatic fungi, especially leaf litter associated hyphomycetes, are exposed to fungicides both via the water phase and their substrate.
Fungicides detected in the studies described above belong to a wide variety of chemical classes with different modes of action, e.g., anilinopyrimides (inhibition of aminoacid synthesis), azoles (inhibition of sterol synthesis), benzimidazoles (inhibition of beta tubulin synthesis), carbamates (inhibition of phospholipid and fatty acid synthesis), carboxylic acid amides (inhibition of cell wall biosynthesis), pyridine-carboxamides (respiration), phenylamides (nucleic acid synthesis), and strobilurins (inhibition of mitochondrial respiration). They comprise almost all classes listed on the Fungicide Resistance Action Committee website (www.frac.info), which provides a comprehensive overview on fungicidal modes of action. A similar spectrum of fungicide classes was detected in a 2013 study on pesticide exposure in 100 streams in agricultural and urban areas of the midwestern United States (Van Metre et al., 2017; Nowell et al., 2018). The authors analyzed extracts of POCIS passive samplers in addition to water samples. Results of the study show that strobilurins such as azoxystrobin, azoles such as tebuconazole as well as benzimidazoles such as carbendazim are of relevance world-wide, and highlight that fungicide pollution might be of similar or even higher importance in urban catchments.
Although adverse effects of organic fungicides on non-target aquatic fungi might be expected and their widespread application in agriculture (Sungur and Tunur, 2012), little information exists both for active fungicidal substances and formulated products. The literature available on this topic is described below. Some authors studied effects on fungal species abundance (i.e., structural endpoints) as well as functional endpoints (Table 1), whereas others only focused on leaf litter breakdown as a functional endpoint (Table 2).
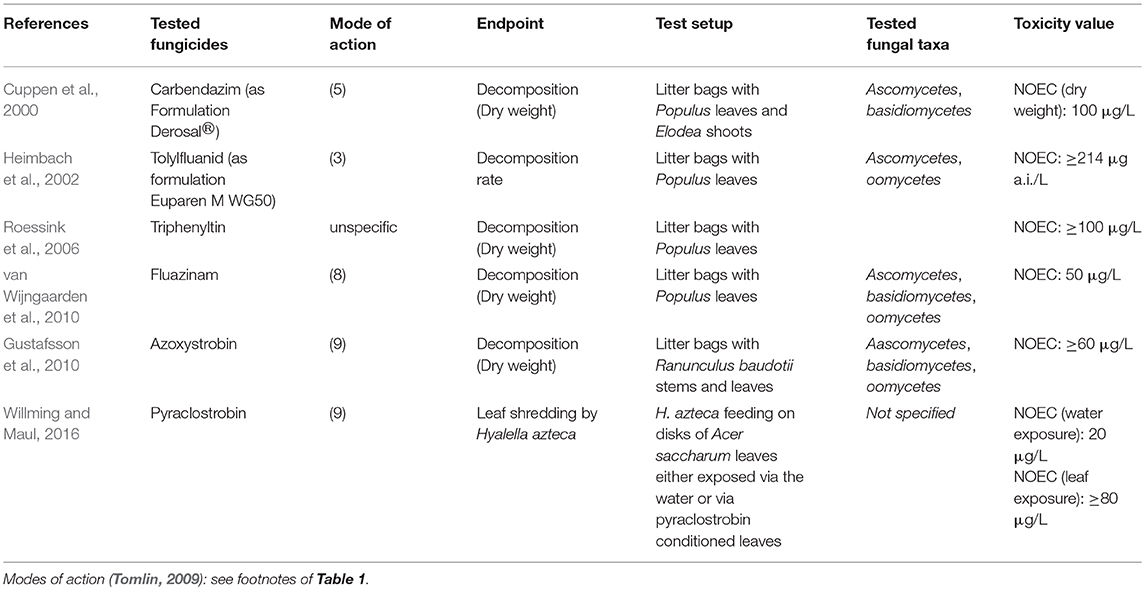
Table 2. Summary of literature on freshwater fungi bioassays focussing on functional effects of fungicides.
Field studies indicate that fungicides affect microbial communities at environmentally relevant concentrations. Wilson et al. (2014) have found that guts of black fly larvae were less infested with mutualistic trychomycetes in agriculturally influenced streams. Fernández et al. (2015) found a correlation between structural changes in microbial communities as well as fungal biomass with increasing predicted fungicide toxicity based on the chemical analysis and toxic unit calculation by combining fungicide monitoring with field studies on fungal communities. Rossi et al. (2017) found differences in fungi community structure between alder leaves exposed in a pristine part of a stream and those exposed at a downstream site where several fungicides were detected by chemical analysis. Gardeström et al. (2016) observed that fungal communities from an agriculturally influenced stream were more tolerant to azoxystrobin than a community without a history of pesticide exposure, indicating a shift in community composition toward tolerant species, also known as pollution induced community tolerance (Molander et al., 1990). The observations from these studies stress the need for considering the hazard to aquatic fungi in fungicide risk assessment, and for new toxicity tests with integral endpoints.
Several studies on structural endpoints analyzed the effects of organic fungicides on fungal communities collected from submerged leaf litter either exposed on leaves, or on agar plates. Bärlocher and Premdas (1988) analyzed the effects of pentachlorophenol (PCP), a non-selective PPP with general biocidal activity (Tomlin, 2009), on aquatic hyphomycetes (Table 1). They found evidence for reduced reproduction (conidia count) and metabolic stress (increased respiration of microbial community on leaf disks) at PCP concentrations of 1 to 1,000 μg/l, with a peak increase at 100 μg/l. Chandrashekar and Kaveriappa (1989) studied the effects of mancozeb and captafol on the growth of aquatic hyphomycetes (Table 1). Both caused no growth inhibition in three fungal species up to a concentration of 5 mg/l, while total inhibition of growth was observed at 500 mg/l to 1000 mg/l. Later Chandrashekar and Kaveriappa (1994) examined the impact of mancozeb, captafol, carbendazim, tridemorph on conidia sporulation and germination in different aquatic hyphomycetes species (Table 1). None of the tested fungicides or other pesticides had inhibitory effects on sporulation or germination at concentrations of ≤5 mg/l and ≤1 mg/l, respectively. Mancozeb, tridemorph, and carbendazim inhibited sporulation of all test species at 500 mg/l and captafol at 2500 mg/l. Conidia germination was inhibited at 1,000 mg/l captafol and 1,000 mg/l mancozeb.
Dijksterhuis et al. (2011) were the first to include species from fungal groups (yeasts, glomeromycetes) and non-fungal groups (oomycetes) other than the aquatic hyphomycetes. They tested the effects of carbendazim, chlorothalonil, fluazinam, imazalil, epoxiconazole, tebuconazole, and azoxystrobin on 6 non-target aquatic fungal species and non-fungal oomycetes isolated from the environment. The authors observed that a comprehensive protection of aquatic fungi and oomycetes in freshwater ecosystems may not be guaranteed through the currently applied standard risk assessment for aquatic organisms for the two tested triazoles, epoxiconazole and tebuconazole, as well as for azoxystrobin. The oomycetes were the most sensitive group for azoxystrobin. Four out of the 6 fungal species (Cryptococcus flavescens, Trichoderma hamatum, Fusarium sporotrichioides, Mucor hiemalis) showed high sensitivity to triazoles, whose mode of action is the inhibition of sterol biosysnthesis. The NOEC for the triazole tebuconazole was lower than an HC5 value derived by Maltby et al. (2009) for these substances with SSDs generated from data on non-fungal species NOECs. A similar finding is reported in Dimitrov et al. (2014), who studied tebuconazole in a lentic water system. The tested concentration of 238 μg/l represents the HC5 of the SSD constructed with acute EC50 values for fish invertebrates and primary producers. While no significant effects were observed for leaf litter decomposition or fungal biomass, a significant reduction in conidia production as well as change in the fungal community composition was observed. Donnadieu et al. (2016) observed a negative effect on fungal biomass after exposure to a single, environmentally relevant concentration of tebuconazole (10.7 μg/l) in indoor streams. Concurrently, bacterial biomass increased. Additionally, the spore number indicated a significant shift in ascomycete composition. The authors concluded that a risk assessment for azole fungicides that is based on vertebrates, invertebrates and primary producers alone may not be protective for the structure and functioning of freshwater ecosystems.
Structural as well as functional endpoints were quantified by Lin et al. (2012) who studied the effects of metiram in outdoor freshwater microcosms on invertebrates, primary producers and microbes. They found no evidence for adverse effects on the biomass and leaf decomposition of aquatic fungi at tested concentrations (0–324 μg/l metiram). No effect on species abundance was observed, however, species identification was limited to only two dominant hyphomycetes species. In the same year, Artigas et al. (2012) conducted a study on the effects of the fungicide tebuconazole on the biomass, community structure, and extracellular enzymatic activities of the microbial community on leaves (Populus nigra, Alnus glutinosa) in indoor stream channels. Tebuconazole applied at 33.1 μg/l reduced leaf litter breakdown rates and biomass development, and modified the fungal community. Moreover, shifts in extracellular enzyme activity were observed, resulting in lower cellulose and hemicellulose decomposition in leaves.
Bundschuh et al. (2011) were the first to study structural (i.e., community composition) as well as ecological (i.e., grazing) endpoints and hence added ecological complexity to their test systems. They investigated the effects of the fungicide tebuconazole (applied as FOLICUR®) on the conditioning process of leaf material by means of food-choice experiments with Gammarus fossarum. Results showed that gammarids preferred leaves conditioned without fungicide over those conditioned in the presence of the fungicide. In addition, fungal biomass (measured as ergosterol concentration) decreased with increasing fungicide concentration and fungal biodiversity was lower in the presence of 50 μg/l and 500 μg/l tebuconazole. The study of Bundschuh et al. (2011) demonstrates the importance of aquatic fungi as food source for invertebrates in the food web of freshwater ecosystems. Also, Zubrod et al. (2015b) observed a significant influence on the feeding rate of G. fossarum when fed leaves preconditioned in the presence of tebuconazole (again applied as FOLICUR®) at a concentration of 500 μg tebuconazole/l. This correlates with shifts in fungal community structure which were observed at 50 μg tebuconazole/l, but were significant only at the next higher test concentration (500 μg/l). Fungal biomass was affected at 5 μg/l whereas the functional endpoint microbial decomposition of leaf material was affected at a concentration of 1 μg/l. The authors also tested formulations of azoxystrobin, carbendazim, cyprodinil, quinoxyfen, and a mixture of all five fungicide formulations (see also Zubrod et al., 2015c) for the same functional and structural endpoints. For four out of five fungicide formulations structural endpoints were more sensitive than functional ones. Similar observations were made by Flores et al. (2014) for the azole fungicide Imazalil and Echinogammarus berilloni, albeit at higher concentrations. The number of fungal species was significantly reduced at 100 μg/l, but there were no significant effects on total sporulation. And also a third amphipod species, Gammarus pulex, showed a significantly reduced feeding rate when fed azole fungicide exposed leaves (again tebuconazole) (Dimitrov et al., 2014). Willming and Maul (2016) observed a reduction in leaf shredding of the amphipod, Hyallela azteca, at 15 μg pyraclostrobin/l. No effect on feeding was observed, however, when only the leaves were exposed to pyraclostrobin, a strobilurin fungicide.
Talk et al. (2016) studied the effects of a mixture of plant protection products, applied in apple orchards. The authors applied the organic fungicides dithianon, dodine, captan, and trifloxystrobin together with copper oxychloride, several insecticides, and herbicides at low concentrations (at or below their regulatory acceptable concentration) in pond mesocosms and studied the fungal community composition by molecular fingerprinting. However, no significant effects were observed due to the pesticide application. Because of the simultaneous presence of insecticides and herbicides this study is not listed in Table 1.
Several studies were conducted on leaf litter decomposition in experimental ponds (Table 2). The fungal community structure was not studied in these experiments. In five of 8 studies no treatment related effects on leaf litter decomposition were observed (Heimbach et al., 2002; Roessink et al., 2006; Gustafsson et al., 2010; Lin et al., 2012): azoxystrobin up to 60 μg/l, metiram up to 324 μg/l, triphenyltin acetate up to 100 μg/l. Transient effects were observed in the remaining studies. Cuppen et al. (2000) detected effects on residual dry weights of Populus leaves after 4 weeks but not after 2 or 8 weeks, indicating a delayed decay at 330 μg/l and 1,000 μg/l carbendazim. In a mixture toxicity study with the fungicide fluazinam, the insecticide lambda-cyhalothrin, and the herbicides asulam and metamitron (van Wijngaarden et al., 2004), delayed leaf decomposition was observed at day 50 for the three highest tested mixture concentrations, but not after 22 or 92 days of exposure. Because of the presence of insecticides this study is not listed in Table 2. For fluazinam applied as a single substance, transient effects for concentrations ≥ 50 μg/l was observed (van Wijngaarden et al., 2010). Pesce et al. (2016) studied the combined effects from fungicide exposure and drought. While a tebuconazole concentration of 20 μg/l did not have a significant effect on leaf litter decomposition when applied alone, it increased the drought effect.
Discussion
Our review clearly demonstrates that fungi are an integral and important part of freshwater ecosystems. Fungicides, which are designed to disrupt fungal cells and their reproduction, have been shown to contaminate surface water bodies in both agricultural and urban areas, and concentrations are high enough to cause concern with regard to negative effects on fungal species and their ecological functions.
Information on the effects of fungicides and fungicide mixtures on fungi is still scarce, primarily because no standardized toxicity tests with fungi species exist. However, non-standard tests have been used in research, including tests with functional (e.g., leaf litter breakdown) and structural (e.g., fungal community composition) endpoints. Results of available studies show that functional test endpoints were generally less sensitive to fungicides than structural endpoints. Mesocosm studies in which leaf litter breakdown was used as an endpoint never showed a long-lasting significant effect in response to fungicide exposure. This is in line with the findings of Cafaro (2005) and Cus et al. (2013), who observed that a decrease in species number did not result in decreased litter breakdown rates. They found, however, that the variability of the litter breakdown rates increased with decreasing species richness. This confirms conclusions made by Bundschuh et al. (2011), who showed that amphipods prefer certain hyphomycete species as food over other fungi, namely that assessing structure is important when aiming at the protection of function.
For several fungicidal modes of action information on fungal toxicity is completely missing so far, e.g., inhibition of nucleic acid synthesis (e.g., metalaxyl-M), inhibition of lipid synthesis (e.g., propamocarb) or cell wall biosynthesis (e.g., dimethomorph). On the other hand, inhibition of sterol biosynthesis (e.g., tebuconazole), inhibition of mitochondrial respiration (e.g., azoxystrobin) and inhibition of beta tubulin synthesis (e.g., carbendazim) are comparatively well studied (Tables 1, 2). It would be desirable to expand the spectrum of test substances and modes of action in future studies.
Currently, the rather qualitative nature of many of the published fungal toxicity studies as well as the limited substance spectrum precludes the performance of a risk assessment, i.e., the comparison of environmental concentrations to effect concentrations. Similarly, there is not enough data to compare sensitivities of aquatic fungi and standard test organisms to fungicides. So far, few assays were able to establish concentration-response curves. Studies aiming at detecting significant differences relative to a control often resulted in unbound (i.e., “<” or “≥”) NOECs. In other cases, NOECs were of limited regulatory value because a spacing factor of 10 was used between test concentrations. The fact that formulation additives, which may increase the aquatic toxicity of pesticides (e.g., Coors and Frische, 2011), are usually neither disclosed by the producer nor included in environmental monitoring campaigns, further complicates risk assessments for aquatic fungi. Nevertheless, when the lowest NOEC values (Tables 1, 2) are compared to the highest concentrations detected by Petersen et al. (2013), and Moschet et al. (2014), the resulting toxicity exposure ratios (TER) are 12 and 25 for the triazole, tebuconazole, and the strobilurin, azoxystrobin, respectively. Using the highly resolved exposure data from the study of Spycher et al. (2018) the lowest TER for azoxystrobin is 0.67, indicating a NOEC exceedance. This confirms the conclusions of previous studies, i.e., that effects of fungicides on aquatic fungi may be of regulatory concern (e.g., Bundschuh et al., 2011; Dijksterhuis et al., 2011; Dimitrov et al., 2014; Donnadieu et al., 2016; Feckler et al., 2016).
The need for new methods has been identified in the aquatic risk assessment guidance document for authorization of plant protection products (EFSA, 2013). Based on the protection goals, tests with functional endpoints were encouraged as a possible way forward. Currently, either leaf discs or whole leaves with naturally occurring or previously inoculated fungal communities are exposed in the lab or as so-called “litter bags” in mesocosm studies. Besides the questions regarding their sensitivity, a principal issue with such bioassays is that they are conducted under conditions that hardly possess similarities to those occurring in freshwater ecosystems. For example, studies using litter bags are often performed in ponds rather than streams, where leaf litter breakdown is ecologically more important. For this reason, guidelines should be developed which consider the ecological relevance of the test system with regard to endpoints and application scenarios. For instance, aquatic hyphomycetes, typical species found on submerged leaf litter, should be tested in mesocosms under flow-through conditions, where oxygen concentrations are representative of their preferred habitat (e.g., Donnadieu et al., 2016; Pesce et al., 2016). For stagnant waters, aquatic fungi colonizing standing-dead emergent plants such as cane (predominantly aero-aquatic fungi and yeasts), would be ecologically relevant test organisms. To date, no such bioassay exists, but some methods are described in the literature on aquatic fungi ecology.
While the degradation of dead plant material represents a key function in food webs of freshwater ecosystems, it is known that aquatic fungi fulfill additional important functions which may be at risk due to fungicide exposure, in particular mutualism (Wilson et al., 2014), the control of phytoplankton population dynamics and the degradation of non-plant dead material. Other interactions such as the relationship between enzyme producing microbes and those that profit from these enzymes and may even outgrow the enzyme producing microbes, so called “cheaters” (Allison, 2005), may also be affected. However, this would require relatively complex testing conditions, and no suitable bioassays currently exist to test toxic effects on these functions. A bioassay using chytridiomycetes, a group known to be crucial for the control of phytoplankton populations, and important for nutrient transfer across different trophic levels, would need to simulate the pelagic zone of a lake with a simple food web. Promising methods as a basis for bioassay development for chytridiomycetes can be found in the literature on aquatic fungi ecology. For example, Kagami et al. (2007) tested the control of algal growth by chytridiomycetes, which have already been used to build a population dynamics model for the control of algal blooms by chytridiomycetes.
According to their protection goals, the WFD (EU 2000) and the biocidal products regulation (European Chemicals Agency, 2015) not only aim at protecting functions, but also the structure (biodiversity and abundance) of organisms in freshwater ecosystems (c.f. SI). From an ecological point of view protecting structural diversity is likely to concomitantly protect ecosystem function. Less structurally diverse communities tend to be more vulnerable to chemical and non-chemical stressors (e.g., Vinebrooke et al., 2004; Morin et al., 2015; Pesce et al., 2016). However, identifying fungal diversity means being able to identify species and reliable identification is often difficult (Krauss et al., 2011). Surveys using DNA barcoding and next generation sequencing techniques are promising approaches to depict fungal species structure in freshwater ecosystems and mesocosms.
Conclusions and Outlook
Freshwater ecosystems comprise complex food webs in which each species plays an essential role as primary producer (e.g., algae) consumer (e.g., Daphnia, fish) or decomposer (e.g., bacteria, fungi). Although largely understudied, aquatic fungi fulfill important and unique functions in freshwater ecosystems, especially in the degradation of allochthonous dead plant litter and the resulting energy transfer to higher trophic levels. In addition, recent studies demonstrate their importance in population dynamics of phytoplankton. Other ecological roles of freshwater fungi may yet be discovered.
The biodiversity and abundance of fungal communitieg9s in freshwater ecosystems is not explicitly protected by current EU regulation. Due to their important ecosystem functions, it is obvious that aquatic fungi should be considered when assessing the risk of pesticides—especially fungicides, of which they are the target organisms. There is evidence that triazoles, in particular, can adversely affect the fungal community of freshwater ecosystems (Bundschuh et al., 2011; Artigas et al., 2012) at environmentally relevant concentrations (Donnadieu et al., 2016). We therefore recommend to extend fungicide risk assessment for aquatic organisms to the trophic level of decomposers using selected fungal species as test organisms. Sufficiently developed methods are available for leaf litter decomposing hyphomycetes. They are of high relevance and should be used in current fungicide risk assessments. In parallel, new fungal bioassays should be developed to account for the structural and functional diversity of aquatic fungi, e.g., interactions of chytridiomycetes with algae and their effect on algal population growth, and fungicide effects on trichomycetes living in the guts of aquatic arthropods.
Author Contributions
LI and MJ conducted the review and would therefore like to share first authorship. IW supervised the work and was involved in the design und preparation of the review.
Funding
The study was solely funded by the Swiss Center for Applied Ecotoxicology Eawag-EPFL.
Conflict of Interest Statement
The authors declare that the research was conducted in the absence of any commercial or financial relationships that could be construed as a potential conflict of interest.
Supplementary Material
The Supplementary Material for this article can be found online at: https://www.frontiersin.org/articles/10.3389/fenvs.2018.00105/full#supplementary-material
Footnotes
1. ^For deriving the environmental quality standard accounting for acute ecotoxicity, the maximum acceptable concentration environmental quality standard (MAC-EQS) for fungicides, the availability of fungi EC50 values is needed to lower the standard AF from 100 to 10 (European Commission, 2011): “For substances with a specific mode of action the most sensitive taxa can be predicted with confidence. Where representatives of the most sensitive taxa are present in the acute dataset, an AF < 100 may again be justified”.
References
Abelho, M., Martins, T. F., Shinn, C., Moreira-Santos, M., and Ribeiro R. (2016). Effects of the fungicide pyrimethanil on biofilm and organic matter processing in outdoor lentic mesocosms. Ecotoxicology 25, 121–131. doi: 10.1007/s10646-015-1574-x
Adl, S. M., Simpson, A. G., Farmer, M. A., Anderson, R. A., Anderson, O. R., Barta, J. R., et al. (2005). The new higher level classification of eukaryotes with emphasis on the taxonomy of protists. J. Eukary Microbiol. 52, 399–451. doi: 10.1111/j.1550-7408.2005.00053.x
Ahearn, D. G., Roth, F. J., and Meyers, S. P. (1968). Ecology and characterization of yeasts from aquatic regions of South Florida. Mar. Biol. 1, 291–308. doi: 10.1007/BF00360780
Allison, S. D. (2005). Cheaters, diffusion and nutrients constrain decomposition by microbial enzymes in spatially structured environments. Ecol. Lett. 8, 626–635. doi: 10.1111/j.1461-0248.2005.00756.x
Artigas, J., Majerholc, J., Foulquier, A., Margoum, C., Volat, B., Neyra, M., et al. (2012). Effects of the fungicide tebuconazole on microbial capacities for litter breakdown in streams. Aquat. Toxicol. 122–123, 197–205. doi: 10.1016/j.aquatox.2012.06.011
Augustin, T., Schlosser, D., Baumbach, R., Schmidt, J., Grancharov, K., Krauss, G., et al. (2006). Biotransformation of 1-naphtol by a strictly aquatic fungus. Curr. Microbiol. 52, 216–220. doi: 10.1007/s00284-005-0239-z
Azevedo, M. M., Carvalho, A., Pascoal, C., Rodrigues, F., and Cássio, F. (2007). Responses of antioxidant defenses to Cu and Zn stress in two aquatic fungi. Sci. Total Environ. 377, 233–243. doi: 10.1016/j.scitotenv.2007.02.027
Bärlocher, F., Guenzel, K., Sridhar, K. R., and Duffy, S. J. (2011). Effects of 4-n-nonylphenol on aquatic hyphomycetes. Sci. Total Environ. 409, 1651–1657. doi: 10.1016/j.scitotenv.2011.01.043
Bärlocher, F., and Kendrick, B. (1974). Dynamics of the fungal populations on leaves in a stream. J. Ecol. 62, 761–791. doi: 10.2307/2258954
Bärlocher, F., and Premdas, P. D. (1988). Effects of pentachlorophenol on aquatic hyphomycetes. Mycologia 80, 135–137. doi: 10.1080/00275514.1988.12025513
Benny, G. L., and O'Donnell, K. (2000). Amoebidium parasiticum is a protozoan, not a Trichomycete. Mycologia 92, 1133–1137. doi: 10.2307/3761480
Bereswil, R., Golla, B., Streloke, M., and Schulz, R. (2012). Entry and toxicity of organic pesticides and copper in vineyard streams: erosion rills jeopardise the efficiency of riparian buffer strips. Agric. Ecosyst. Environ. 146 81–92 doi: 10.1016/j.agee.2011.10.010
Bundschuh, M., Zubrod, J. P., Kosol, S., Maltby, L., Stang, C., Duester, L., et al. (2011). Fungal composition on leaves explains pollutant-mediated indirect effects on amphipod feeding. Aquatic Toxicol. 104, 32–37 doi: 10.1016/j.aquatox.2011.03.010
Cafaro, M. J. (2005). Eccrinales (Trichomycetes) are not fungi, but a clade of protists at the early divergence of animals and fungi. Mol. Phylogenet. Evol. 35, 21–34. doi: 10.1016/j.ympev.2004.12.019
Chandrashekar, K. R., and Kaveriappa, K. M. (1989). Effect of pesticides on the growth of aquatic hyphomycetes. Toxicol. Lett. 48, 311–315. doi: 10.1016/0378-4274(89)90058-1
Chandrashekar, K. R., and Kaveriappa, K. M. (1994). Effect of pesticides on sporulation and germination of conidia of aquatic hyphomycetes. J. Environ. Biol. 15, 315–324.
Coors, A., and Frische, T. (2011). Predicting the aquatic toxicity of commercial pesticide mixtures. Environ. Sci Europe 23:22 doi: 10.1186/2190-4715-23-22
Cruzeiro, C., Rocha, E., Pardal, M. Â., and Rocha, M. J. (2015). Uncovering seasonal patterns of 56 pesticides in surface coastal waters of the Ria Formosa lagoon (Portugal), using a GC-MS method. Int. J. Environ. Anal. Chem. 95, 1370–1384. doi: 10.1080/03067319.2015.1100724
Cummins, K. W. (1974). Structure and functions of stream ecosystems. Bio. Sci. 24, 631–641. doi: 10.2307/1296676
Cuppen, J. G., van den Brink, P. J., Camps, E., Uil, K. F., and Brock, T. C. M. (2000). Impact of the fungicide carbendazim in freshwater microcosms. I. Water quality, breakdown of particulate organic matter and responses of macroinvertebrates. Aquatic Toxicol. 48, 233–250. doi: 10.1016/S0166-445X(99)00036-3
Cus, F., Bach, B., Barnavon, L., and Pongrac, V. Z. (2013). Analytical determination of Dolenjska region wines quality. Food Control 33, 274–280. doi: 10.1016/j.foodcont.2013.03.017
Dijksterhuis, J., van Doorn, T., Samson, R., and Postma, J. (2011). Effects of seven fungicides on non-target aquatic fungi. Water Air Soil Pollution 222, 421–425. doi: 10.1007/s11270-011-0836-3
Dimitrov, M. R., Kosol, S., Smidt, H., Buijse, L., Van den Brink, P. J., Van Wijngaarden, R. P., et al. (2014). Assessing effects of the fungicide tebuconazole to heterotrophic microbes in aquatic microcosms. Sci. Total Environ. 490, 1002–1011. doi: 10.1016/j.scitotenv.2014.05.073
Donnadieu, F., Besse-Hoggan, P., Forestier, C., and Artigas, J. (2016). Influence of streambed substratum composition on stream microbial communities exposed to the fungicide tebuconazole. Freshw. Biol. 61, 2026–2036. doi: 10.1111/fwb.12679
Duddridge, J. E., and Wainwright, M. (1980). Heavy metal accumulation by aquatic fungi and reduction in viability of Gammarus pulexi fed Cd2+ contaminated mycelium. Water Res. 14, 1605–1611. doi: 10.1016/0043-1354(80)90065-2
EFSA (2013). Outcome of the Public Consultation on the Draft PPR Panel Guidance Document on Tiered Risk Assessment for Plant Protection Products for Aquatic Organisms in Edge-Of-Field Surface Waters. Technical report EFSA supporting publication 2013: EN−460.
European Chemicals Agency (2015). Guidance on Biocidal Products Regulation: Volume IV Environment Part B Risk Assessment (active substances). Helsinki: European Chemicals Agency.
European Commission (2011). Common Implementation Strategy for the Water Framework Directive. Technical Guidance for Deriving Environmantal Quality Standards
Feckler, A., Goedkoop, W., Zubrod, J. P., Schulz, R., and Bundschuh, M. (2016). Exposure pathway-dependent effects of the fungicide epoxiconazole on a decomposer-detritivore system. Sci. Total Environ. 571, 992–1000. doi: 10.1016/j.scitotenv.2016.07.088
Fernández, D., Voss, K., Bundschuh, M., Zubrod, J. P., and Schäfer, R. B. (2015). Effects of fungicides on decomposer communities and litter decomposition in vineyard streams. Sci. Total Environ. 533, 40–48 doi: 10.1016/j.scitotenv.2015.06.090
Findlay, S., and Arsuffi, T. L. (1989). Microbial growth and detritus transformations during decomposition of leaf litter in a stream. Freshwater Biol 21, 261–269. doi: 10.1111/j.1365-2427.1989.tb01364.x
Fisher, S., and Likens, G. (1973). Energy flow in Bear Brook, New Hamphshire: an intergrative approach to stream ecosystem metabolism. Ecol. Monogr. 43, 421–439. doi: 10.2307/1942301
Flores, L., Banjac, Z., Farré, M., Larrañaga, A., Mas-Martí, E., Muñoz, I., et al. (2014). Effects of a fungicide (imazalil) and an insecticide (diazinon) on stream fungi and invertebrates associated with litter breakdown. Sci. Total Environ. 476–477, 532–541. doi: 10.1016/j.scitotenv.2014.01.059
Gardeström, J., Ermold, M., Goedkoop, W., and McKie, B. G. (2016). Disturbance history influences stressor impacts: effects of a fungicide and nutrients on microbial diversity and litter decomposition. Freshw. Biol. 61, 2171–2184. doi: 10.1111/fwb.12698
Gessner, M. O., Chauvet, E., and Dobson, M. (1999). A perspective on leaf litter breakdown in streams. Oikos 85, 377–384. doi: 10.2307/3546505
Gessner, M. O., Gulis, V., Kuehn, K. A., Chauvet, E., and Suberkropp, K. (2007). “Fungal Decomposers of Plant Litter in Aquatic Ecosystems,” in The Mycota, ed K. Esser (Berlin: Springer), 301–324.
Gleason, F. H., Maiko, K., Lefevre, E., and Sime-Ngando, T. (2008). The ecolocy of chytrids in aquatic ecosystems: roles in food web dynamics. Fung. Biol. Rev. 22, 17–25. doi: 10.1016/j.fbr.2008.02.001
Goh, T. K., and Hyde, K. D. (1996). Biodiversity of freshwater fungi. J. Ind. Microbiol. Biot. 17, 328–345. doi: 10.1007/BF01574764
Gustafsson, K., Blidberg, E., Elfgren, I. K., Hellström, A., Kylin, H., and Gorokhova, E. (2010). Direct and indirect effects of the fungicide azoxystrobin in outdoor brackish water microcosms. Ecotoxicology 19, 431–444. doi: 10.1007/s10646-009-0428-9
Hawksworth, D. L. (1991). The fungal dimension of biodiversity: magnitude, significance, and conservation. Mycol. Res. 95, 641–655. doi: 10.1016/S0953-7562(09)80810-1
Hawksworth, D. L. (2001). The magnitude of fungal diversity: the 1.5 million species estimate revisited. Mycol. Res. 105, 1422–1432. doi: 10.1017/S0953756201004725
Heimbach, F., Brock, T., Arts, G., and Deneer, J. (2002). Effects of multiple applications of Tolylfluanid WG50 on the aquatic community in outdoor microcosm enclosures. Bayer, A. G., unpublished report No.:HBF/Mt 13 cited in the EU Draft Assessment Report (DAR). for Tolylfluanid Volume 3, Annex, B., B.9 Ecotoxicology, 480–482.
Hernández Roa, J. J., Virella, C. R., and Cafaro, M. J. (2009). First survey of arthropod gut fungi and associates from Vieques, Puerto Rico. Mycologia 101, 896–903. doi: 10.3852/08-187
Hibbett, D. S., Binder, M., Bischoff, J. F., Blackwell, M., Cannon, P. F., Eriksson, O. E., et al. (2007). A higher-level phylogenetic classification of the Fungi. Mycol. Res. 111, 509–547. doi: 10.1016/j.mycres.2007.03.004
Inoue, S., Igarashi, Y., Yoneda, Y., Kawai, S., Okamura, H., and Nishida, T. (2015). Elimination and detoxification of fungicide miconazole and antidepressant sertraline by manganese peroxidase-dependent lipid peroxidation system. Int. Biodeteriorat. Biodegradat. 100, 79–84. doi: 10.1016/j.ibiod.2015.02.017
Jaeckel, P., Krauss, G., Menge, S., Schierhorn, A., Rücknagel, P., and Krauss, G. J. (2005a). Cadmium induces a noval metallothionein and phytochelatin 2 in an aquatic fungus. Biochem. Bioph. Res. Co. 333, 150–155. doi: 10.1016/j.bbrc.2005.05.083
Jaeckel, P., Krauss, G.-J., and Krauss, G. (2005b). Cadmium and zinc response of the Heliscus lugdenensis and Verticillium cf. alboatrum isolated from highly polluted water. Sci. Total Environ. 346, 274–279. doi: 10.1016/j.scitotenv.2004.12.082
James, T. Y., Kauff, F., Schoch, C. L., Matheny, P. B., Hofstetter, V., Cox, C. J., et al. (2006). Reconstructing the early evolution of fungi using a six-gene phylogeny. Nature 443, 818–822. doi: 10.1038/nature05110
Jobard, M., Rasconi, S., and Sime-Ngando, T. (2010). Diversity and functions of microsporic fungi: a missing component in pelagic food webs. Aquat. Sci. 72, 255–268. doi: 10.1007/s00027-010-0133-z
Kagami, M. (2008). The roles of parasitic chytrids in the aquatic food web. Jpn. J. Ecol. 58, 71–80.
Kagami, M., de Bruin, A., Ibelings, B. W., and Van Donk, E. (2007). Parasitic chytrids: their effects on phytoplankton communities and food-web dynamics. Hydrobiologia 578, 113–129. doi: 10.1007/s10750-006-0438-z
Kagami, M., Miki, T., and Takimoto, G. (2014). Mycoloop: Chytrids in aquatic food webs. Front. Microbiol. 5:166. doi: 10.3389/fmicb.2014.00166
Krauss, G.-J., Solé, M., Krauss, G., Schlosser, D., Wesenberg, D., and Bärlocher, F. (2011). Fungi in freshwaters: ecology, physiology and biochemical potential. FEMS Microbiol. Rev. 35, 620–651. doi: 10.1111/j.1574-6976.2011.00266.x
Kreuger, J., Graaf, S., Patring, J., and Adielsson, S. (2010). Pesticides in surface water in areas with open ground and greenhouse horticultural crops in Sweden 2008. Ekohydrologi 117, 1–47.
Lichtwardt, R. W., and Williams, M. C. (1999). Three Harpellales that live in one species of aquatic chironomid larva. Mycologia 91, 396–399. doi: 10.2307/3761385
Lin, R., Buijse, L., Dimitrov, M. R., Dohmen, P., Kosol, S., Maltby, L., et al. (2012). Effects of the fungicide metiram in outdoor freshwater microcosms: responses of invertebrates, primary producers and microbes. Ecotoxicology 21, 1550–1569. doi: 10.1007/s10646-012-0909-0
Lucas, D., Badia-Fabregat, M., Vicent, T., Caminal, G., Rodríguez-Mozaz, S., Balcázar, J. L., et al. (2016). Fungal treatment for the removal of antibiotics and antibiotic resistance genes in veterinary hospital wastewater. Chemosphere 152, 301–308. doi: 10.1016/j.chemosphere.2016.02.113
Lutzoni, F., Kauff, F., Cox, C. J., McLaughlin, D., Celio, G., Dentinger, B., et al. (2004). Assembling the fungal tree of life: progress, classification, and evolution of subcellular traits. Am. J. Bot. 91, 1446–1480. doi: 10.3732/ajb.91.10.1446
Maltby, L., Brock, T. C., and van den Brink, P. J. (2009). Fungicide risk assessment for aquatic ecosystems: importance of interspecific variation, toxic mode of action, and exposure regime. Environ. Sci. Technol. 43, 7556–7563. doi: 10.1021/es901461c
Martínková, L., Kotik, M., Marková, E., and Homolka, L. (2016). Biodegradation of phenolic compounds by Basidiomycota and its phenol oxidases: a review. Chemosphere 149, 373–382. doi: 10.1016/j.chemosphere.2016.01.022
Massaccesi, G., Romero, M. C., Cazau, M. C., and Bucsinszky, A. M. (2002). Cadmium removal capacities of filamentous soil fungi isolated from industrially polluted sediments, in La Plata (Argentina). World J. Microb. Biot. 18, 817–820. doi: 10.1023/A:1021282718440
Miki, T., Takimoto, G., and Kagami, M. (2011). Roles of parasitic fungi in aquatic food webs: a theoretical approach. Freshwater Biol. 56, 1173–1183. doi: 10.1111/j.1365-2427.2010.02562.x
Molander, S., Blanck, H., and Söderström, M. (1990). Toxicity assessment by pollution-induced community tolerance (PICT), and identification of metabolites in periphyton communities after exposure to 4,5,6-trichloroguaiacol. Aquatic Toxicol. 18, 115–136. doi: 10.1016/0166-445X(90)90022-H
Morin, S., Bonet, B., Corcoll, N., Guasch, H., Bottin, M., and Coste, M. (2015). Cumulative stressors trigger increased vulnerability of diatom communities to additional disturbances. Microbiol. Aquat. Syst. 70, 585–595. doi: 10.1007/s00248-015-0602-y
Moschet, C., Wittmer, I., Simovic, J., Junghans, M., Piazzoli, A., Singer, H., et al. (2014). How a complete pesticide screening changes the assessment of surface water quality. Environ. Sci. Technol. 48, 5423–5432. doi: 10.1021/es500371t
Mueller, G. M., and Schmit, J. P. (2007). Fungal biodiversity: what do we know? What can we predict? Biodivers. Conserv. 16, 1–5. doi: 10.1007/s10531-006-9117-7
Müller-Navarra, D. C., Brett, M. T., Liston, A. M., and Goldman, C. R. (2000). A highly unsaturated fatty acid predicts carbon transfer between primary producers and consumers. Nature 403, 74–77. doi: 10.1038/47469
Nelson, D. J., and Scott, D. C. (1962). Role of detritus in the productivity of a rock-outcrop community in a Piedmont stream. Limnol. Oceanogr. 7, 396–413. doi: 10.4319/lo.1962.7.3.0396
Nowell, L. H., Moran, P. W., Schmidt, T. S., Norman, J. E., Nakagaki, N., Shoda, M. E., et al. (2018). Complex mixtures of dissolved pesticides show potential aquatic toxicity in a synoptic study of Midwestern U.S. streams. Sci. Total Environ. 613–614, 1469–1488. doi: 10.1016/j.scitotenv.2017.06.156
Oliveira, B. R., Penetra, A., Cardoso, V. V., Benoliel, M. J., Barreto Crespo, M. T., Samson, R. A., et al. (2015). Biodegradation of pesticides using fungi species found in the aquatic environment. Environ. Sci. Pollut. Res. 22, 11781–11791. doi: 10.1007/s11356-015-4472-0
Oliveira, M. V., Vidal, B. T., Melo, C. M., de Miranda Rde, C., Soares, C. M., Coutinho, J. A., et al. (2016). (Eco)toxicity and biodegradability of protic ionic liquids. Chemosphere 147, 460–466. doi: 10.1016/j.chemosphere.2015.11.016
Omoike, A., Wacker, T., and Navidonski, M. (2013). Biodegradaion of bisphenol A by Heliscus lugdunensis, a naturally occurring hyphomycete in freshwater environments. Chemosphere 91, 1643–1647. doi: 10.1016/j.chemosphere.2012.12.045
Pascoal, C., Cássio, F., and Marvanov,á, L. (2005). Anthropogenic stress may affect aquatic hyphomycete diversity more than leaf decomposition in a low-order stream. Archiv für Hydrobiologie 162, 481–496. doi: 10.1127/0003-9136/2005/0162-0481
Pesce, S., Zoghlami, O., Margoum, C., Artigas, J., Chaumot, A., and Foulquier, A. (2016). Combined effects of drought and the fungicide tebuconazole on aquatic leaf litter decomposition. Aquat. Toxicol. 173, 120–131. doi: 10.1016/j.aquatox.2016.01.012
Petersen, K., Stenrod, M., and Tollefsen, K. E. (2013). Initial Environmental Risk Assessment of Combined Effects of Palnt Protection Products in Six Different Areas in Norway. Oslo: NIVA - Norwegian Institute for Water Research.
Rabiet, M., Margoum, C., Gouy, V., Carluer, N., and Coquery, M. (2010). Assessing pesticide concentrations and fluxes in the stream of a small vineyard catchment - Effect of sampling frequency. Environ. Pollut. 158, 737–748 doi: 10.1016/j.envpol.2009.10.014
Rasconi, S., Grami, B., Niquil, N., Jobard, M., and Sime-Ngando, T. (2014). Parasitic chytrids sustain zooplankton growth during inedible algal bloom. Front. Microbiol. 5:229. doi: 10.3389/fmicb.2014.00229
Roessink, I., Crum, S. J. H., Bransen, F., van Leeuwen, E., Van Kerkum, F., Koelmans, A. A., et al. (2006). Impact of triphenyltin acetate in microcosms simulating floodplain lakes. I. Influence of sediment quality. Ecotoxicology 15, 267–293. doi: 10.1007/s10646-006-0058-4
Rossi, F., Artigas, J., and Mallet, C. (2017). Structural and functional responses of leaf-associated fungal communities to chemical pollution in streams. Freshw. Biol. 62, 1207–1219. doi: 10.1111/fwb.12937
Roussel, H., Chauvet, E., and Bonzom, J. M. (2008). Alteration of leaf decomposition in copper-contaminated freshwater mesocosms. Environ. Toxicol. Chem. 27, 637–644. doi: 10.1897/07-168.1
Shearer, C. A., Descals, E., Kohlmeyer, B., Kohlmeyer, J., Marvanov,á, L., Padgett, D., et al. (2007). Fungal biodiversity in aquatic habitats. Biodivers. Conserv. 16, 49–67. doi: 10.1007/s10531-006-9120-z
Solé, M., Fetzer, I., Wennrich, R., Sridhar, K. R., Harms, H., and Krauss, G. (2008). Aquatic hyphomycete communities as potential bioindicators for assessing anthropogenic stress. Sci. Total Environ. 389, 557–565. doi: 10.1016/j.scitotenv.2007.09.010
Spycher, S., Mangold, S., Doppler, T., Junghans, M., Wittmer, I., Stamm, C., et al. (2018). Pesticide risks in small streams-how to get as close as possible to the stress imposed on aquatic organisms. Environ. Sci. Technol. 52, 4526–4535. doi: 10.1021/acs.est.8b00077
Sridhar, K. R., Bärlocher, F., Wennrich, R., Krauss, G.-J., and Krauss, G. (2008). Fungal biomass and diversity in sediments and on leaf litter in heavy metal contaminated waters of Central Germany. Fund. Appl. Limnol. 171, 63–74. doi: 10.1127/1863-9135/2008/0171-0063
Strongman, D. B. (2007). Trichomycetes in aquatic insects from Prince Edward Island, Canada. Can. J. Bot. 85, 949–963. doi: 10.1139/B07-095
Sungur, S., and Tunur, C. (2012). Investigation of pesticide residues in vegetables and fruits grown in various regions of Hatay, Turkey. Food Addit. Cont. Part B-Surveill. 5, 265–267. doi: 10.1080/19393210.2012.704597
Talk, A., Kublik, S., Uksa, M., Engel, M., Berghahn, R., Welzl, G., et al. (2016). Effects of multiple but low pesticide loads on aquatic fungal communities colonizing leaf litter. J. Environ. Sci. 46, 116–125. doi: 10.1016/j.jes.2015.11.028
Teal, J. M. (1957). Community metabolism in a temperate cold spring. Ecol. Monogr. 27, 283–302. doi: 10.2307/1942187
Van Metre, P. C., Alvarez, D. A., Mahler, B. J., Nowell, L., Sandstrom, M., and Moran, P. (2017). Complex mixtures of pesticides in Midwest U.S. streams indicated by POCIS time-integrating samplers. Environ. Pollut. 220, 431–440. doi: 10.1016/j.envpol.2016.09.085
van Wijngaarden, R. P., Arts, G. H., Belgers, J. D., Boonstra, H., Roessink, I., Schroer, A. F., et al. (2010). The species sensitivity distribution approach compared to a microcosm study: a case study with the fungicide fluazinam. Ecotoxicol. Environ. Saf. 73, 109–122. doi: 10.1016/j.ecoenv.2009.09.019
van Wijngaarden, R. P., Cuppen, J. G., Arts, G. H., Crum, S. J., van den Hoorn, M. W., Van Den Brink, P. J., et al. (2004). Aquatic risk assessment of a realistic exposure to pesticides used in bulb crops: a microcosm study. Environ. Toxicol. Chem. 23, 1479–1498. doi: 10.1897/03-80
Vinebrooke, R. D., Cottingham, K. L., Norberg, J., Scheffer, M., Dodson, S. I., Maberly, S. C., et al. (2004). Impacts of multiple stressors on biodiversity and ecosystem functioning: the role of species co-tolerance. Oikos 104, 451–457. doi: 10.1111/j.0030-1299.2004.13255.x
Voigt, K., and Kirk, P. M. (2011). Recent developments in the taxonomic affiliation and phylogenetic positioning of fungi: impact in applied microbiology and environmental biotechnology. Appl. Microbiol. Biot. 90, 41–57. doi: 10.1007/s00253-011-3143-4
Willming, M. M., and Maul, J. D. (2016). Direct and indirect toxicity of the fungicide pyraclostrobin to Hyalella azteca and effects on leaf processing under realistic daily temperature regimes. Environ. Pollut. 211, 435–442. doi: 10.1016/j.envpol.2015.11.029
Wilson, E. R., Smalling, K. L., Reilly, T. J., Gray, E., Bond, L., Steele, L., et al. (2014). Assessing the potential effects of fungicides on nontarget gut fungi (trichomycetes). and their associated larval black fly hosts. J. Am. Water Resour. Assoc. 50, 420–433. doi: 10.1111/jawr.12166
Woese, C. R., and Fox, G. E. (1977). Phylogenetic structure of the prokaryotic domain: The primary kingdoms. Proc. Natl. Acad. Sci. U.S.A. 74, 5088–5090. doi: 10.1073/pnas.74.11.5088
Woese, C. R., Kandler, O., and Wheelis, M. L. (1990). Towards a natural system of organisms: Proposal for the domains Archaea, Bacteria and Eucarya. Proc. Natl. Acad. Sci. U.S.A. 87, 4576–4579. doi: 10.1073/pnas.87.12.4576
Wong, M. K. M., Goh, T.-K., Hodgkiss, I. J., Hyde, K. D., Ranghoo, V. M., Tsui, C. K. M., et al. (1998). Role of fungi in freshwater ecosystems. Biodivers. Conservat. 7, 1187–1206. doi: 10.1023/A:1008883716975
Wurzbacher, C., Rösel, S., Rychła, A., and Grossart, H. P. (2014). Importance of saprotrophic freshwater fungi for pollen degradation. PLoS ONE 9:e94643. doi: 10.1371/journal.pone.0094643
Wurzbacher, C. M., Bärlocher, F., and Grossart, H.-P. (2010). Fungi in lake ecosystems. Aquat. Microb. Ecol. 59, 125–149. doi: 10.3354/ame01385
Zubrod, J. P., Englert, D., Feckler, A., Koksharova, N., Konschak, M., Bundschuh, R., et al. (2015b). Does the current fungicide risk assessment provide sufficient protection for key drivers in aquatic ecosystem functioning? Environ. Sci. Technol. 49, 1173–1181. doi: 10.1021/es5050453
Zubrod, J. P., Englert, D., Wolfram, J., Wallace, D., Schnetzer, N., Baudy, P., et al. (2015c). Waterborne toxicity and diet-related effects of fungicides in the key leaf shredder Gammarus fossarum (Crustacea: Amphipoda). Aquat. Toxicol. 169, 105–112. doi: 10.1016/j.aquatox.2015.10.008
Zubrod, J. P., Feckler, A., Englert, D., Koksharova, N., Rosenfeldt, R. R., Seitz, F., et al. (2015a). Inorganic fungicides as routinely applied in organic and conventional agriculture can increase palatability but reduce microbial decomposition of leaf litter. J. Appl. Ecol. 52, 310–322. doi: 10.1111/1365-2664.12393
Keywords: fungal ecology, fungal diversity, plant protection products, biocides, water framework directive, policy analysis
Citation: Ittner LD, Junghans M and Werner I (2018) Aquatic Fungi: A Disregarded Trophic Level in Ecological Risk Assessment of Organic Fungicides. Front. Environ. Sci. 6:105. doi: 10.3389/fenvs.2018.00105
Received: 31 March 2017; Accepted: 27 August 2018;
Published: 25 September 2018.
Edited by:
Carsten A. Brühl, Universität Koblenz Landau, GermanyReviewed by:
Yong Liu, Hunan Academy of Agricultural Sciences (CAAS), ChinaFernando José Cebola Lidon, Universidade Nova de Lisboa, Portugal
Joan Artigas, UMR6023 Laboratoire Microorganismes Génome Et Environnement (LMGE), France
Copyright © 2018 Ittner, Junghans and Werner. This is an open-access article distributed under the terms of the Creative Commons Attribution License (CC BY). The use, distribution or reproduction in other forums is permitted, provided the original author(s) and the copyright owner(s) are credited and that the original publication in this journal is cited, in accordance with accepted academic practice. No use, distribution or reproduction is permitted which does not comply with these terms.
*Correspondence: Marion Junghans, TWFyaW9uLkp1bmdoYW5zQG9la290b3h6ZW50cnVtLmNo
†These authors share first authorship