- 1Australian Rivers Institute, Griffith University, Nathan, QLD, Australia
- 2Griffith School of Environment and Science, Griffith University, Nathan, QLD, Australia
- 3Science and Engineering Faculty, Queensland University of Technology, Brisbane, QLD, Australia
- 4Griffith Climate Change Response Program, Griffith University, Gold Coast, QLD, Australia
Environmental water management has become a global imperative in response to environmental degradation and the growing recognition that human well-being and livelihoods are critically dependent on freshwater ecosystems and the ecological functions and services they provide. Although a wide range of techniques and strategies for planning and implementing environmental flows has developed, many remain based on assumptions of hydrologic stationarity, typically focusing on restoring freshwater ecosystems to pre-development or “natural” conditions. Climate change raises major challenges to this conventional approach, in part because of increasing uncertainties in patterns of water supply and demand. In such a rapidly changing world, the implementation of, and capacity of water managers to deliver flow regimes resembling historical hydrological patterns may be both unfeasible and undesirable. Additionally, as emphasis shifts from species-focused water allocation plans toward a greater appreciation of freshwater ecological functions and services, many of which will be influenced by climate change, a thorough re-evaluation of the conventional objectives, planning, delivery and monitoring of environmental water, including its role in the broader context of water and environmental management, is essential. Here, we identify the major challenges posed by climate change to environmental water management and discuss key adaptations and research needed to meet these challenges to achieve environmental and societal benefits and avoid maladaptation.
Introduction
Environmental water management (EWM) has become a global imperative in response to environmental degradation and the growing recognition that human well-being and livelihoods are critically dependent on freshwater ecosystems (Capon et al., 2013; Horne et al., 2017a). Considerable research has underpinned the development of a wide range of approaches and tools to support decision-making regarding the acquisition and delivery of environmental water (Table 1; Arthington, 2012). For the most part, however, EWM remains grounded in assumptions of hydrologic stationarity and typically focuses on restoring freshwater water systems to pre-development or “natural” conditions (Milly et al., 2008; Poff and Matthews, 2013; Poff, 2018). Recent developments in ecological science and natural resources management have prompted a need to expand the spatial and temporal scales of EWM (McCluney et al., 2014) and to broaden consideration of its human context (e.g., Finn and Jackson, 2011; Adams et al., 2017; Capon and Capon, 2017). Climate change in particular necessitates a revision of EWM, especially as it represents, in itself, an important strategy in society's broader adaptation to climate change by promoting the protection and augmentation of increasingly critical ecosystem services (Capon and Bunn, 2015).
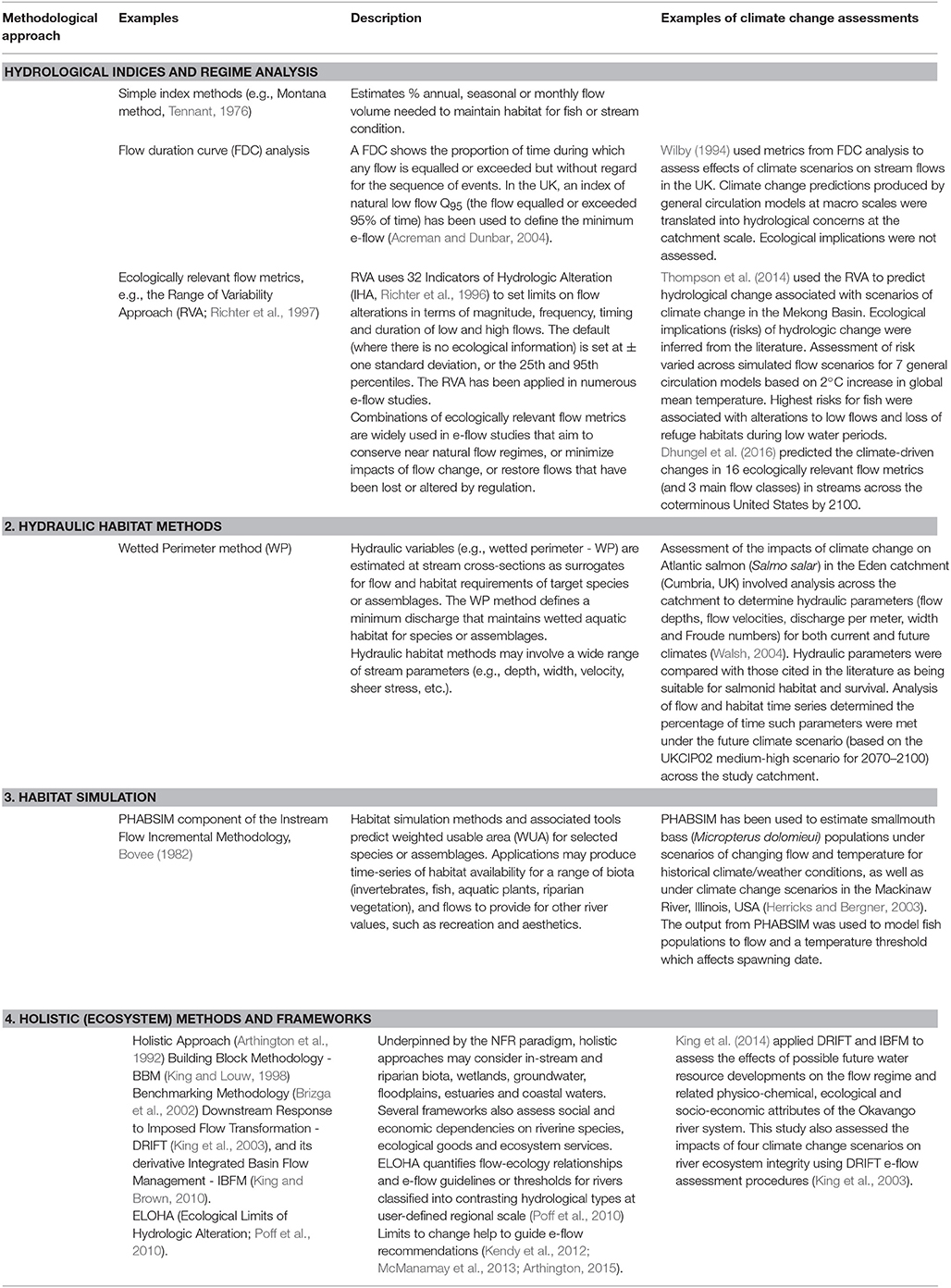
Table 1. Four main methodological approaches used to design environmental flows with examples of relevant climate change assessments (for details of methods and case studies, see Tharme, 2003; Arthington, 2012; Linnansaari et al., 2012).
Here, we discuss major challenges to environmental flows and EWM under a changing climate as well as the adaptations needed to meet these for both environmental and societal benefit. We use the familiar term “environmental flows” to denote the quantity and spatio-temporal distribution of water delivered, or deemed necessary, to support ecological and societal objectives for rivers, wetlands, and groundwater-dependent ecosystems(Dyson et al., 2003; Arthington, 2012), whereas “EWM” conveys the broader context of environmental water research policy, planning and management (Horne et al., 2017a,b). We begin by outlining the main implications of climate change for EWM. We then consider how conventional approaches to setting objectives and targets, planning and prioritization, delivery, monitoring and evaluation of environmental water might be adapted so that such barriers may be overcome and opportunities for transformation capitalized upon. Finally, we identify key knowledge needs required to support such adaptation.
Climate Change Challenges for EWM
In addition to increasing levels of uncertainty and unpredictability, climate change poses four main challenges for EWM, the first two of which concern the supply of environmental water while the latter two affect demand for its application. First, climate change is driving shifts in patterns of water supply globally with increasing water scarcity and risks to water security anticipated in many places (Vörösmarty et al., 2010; Grey et al., 2013). Both surface and ground water hydrology are highly sensitive to the altered precipitation, warming, increased evaporation, sea level rise and altered snow melt projected under many climate change scenarios (Milly et al., 2005; Döll and Schmied, 2012; IPCC, 2012, 2014; Leigh et al., 2015), with small changes in climatic drivers potentially causing large changes in flow regimes (Capon et al., 2013; Acreman et al., 2014a). Concurrent shifts in water quality are also widely expected (e.g., Döll and Schmied, 2012; Ledger and Milner, 2015). Second, human water demands, especially for agriculture, are simultaneously expected to rise including those related to climate change mitigation and adaptation actions in other sectors, e.g., generation of hydroelectricity or plantations for carbon sequestration (Capon and Bunn, 2015), placing further pressure on already limited environmental water allocations.
Third, freshwater ecosystems, their biota, functions and services, are highly vulnerable to climate change due to high levels of exposure and sensitivity to projected changes and extreme events (Capon et al., 2013; Leigh et al., 2015; Peirson et al., 2015). Ecological responses to climate change will be complex, dynamic and variable and are very likely to involve shifts in the composition and structure of freshwater ecosystems which, in turn, will affect the ecological functions, goods and services these provide (Capon et al., 2013; Datry et al., 2017). In particular, significant shifts in the distribution of freshwater taxa can be expected in response to projected climatic changes (James et al., 2017). Ecological responses to hydrology are also likely to change. Warmer temperatures, for instance, may make ecosystems and biota “thirstier” and potentially less tolerant of past drying regimes (Leigh et al., 2015, 2016). Shifts in ecological functions and ecosystem services can be similarly anticipated. The capacity of freshwater ecosystems to retain flood waters, for example, may become more variable in space and time (Capon et al., 2013; Datry et al., 2017). Freshwater ecosystems will furthermore be sensitive to climate change effects in the surrounding landscape which may exacerbate direct impacts (Capon et al., 2013; Hadwen and Capon, 2014). Finally, the demand for and importance of many water ecosystem goods (e.g., fish) and services (e.g., flood mitigation) are likely to increase under a changing climate (Capon and Bunn, 2015), as are the significance of some ecological functions, e.g., the provision of riparian corridors for species' migration and the role of riparian and wetland areas as drought and thermal refuges for terrestrial fauna (Capon et al., 2013).
Collectively, the challenges outlined here have significant implications for most aspects of environmental flows and EWM from setting objectives through to delivery, monitoring and adaptive management. Increasing water scarcity and demand, for instance, will likely create a greater requirement for water managers to justify environmental water allocations and demonstrate their benefits as well as to increase the efficiency of their delivery (Horne et al., 2017a). Overall, climate change can be expected to reduce the availability and quality of environmental water allocations in most places as well as shifting these both spatially and temporally. At the same time, the possibilities of what might be feasibly, and desirably, achieved with environmental water can also be anticipated to shift. Herein lies the opportunity of transformational EWM, whereby targets may be more forward-looking in order to deliver the types of goods and services we will need in a climate-changed world.
Adapting EWM
A wide variety of methodologies and frameworks have been developed to guide environmental flows and EWM, ranging from those which focus on calculating local flow regime requirements associated with specific targets (e.g., the Building Block Methodology) to those which consider the broader EWM arena, i.e., including environmental and societal objective setting etc. (e.g., ELOHA; Table 1). Additionally, some studies have explored the implications of climate change for many of these existing methodologies (Table 1). For the most part, however, such studies have mainly concerned probable hydrologic and, to a far lesser extent, ecological impacts of projected climate change to inform vulnerability or risk assessments. Significant assessments of water security risks posed by climate variability, change, and extreme events from a socio-economic have also been conducted (Grey et al., 2013; Hall et al., 2014). Adapting water resources management to climate change, however, requires integrated assessments of vulnerability across socio-ecological systems (Pahl-Wostl, 2007). Here, we provide a broader discussion of the implications of the climate change challenges previously identified with respect to key stages of adaptive management of environmental water. Throughout, we emphasize three guiding principles which we assert are critical to avoiding perverse outcomes of EWM and approaches to climate change adaptation in this sector (sensu Capon et al., 2013; Peirson et al., 2015; Finlayson et al., 2017a).
First, climate change highlights the need for EWM to extend its scope beyond conventionally narrow ecological objectives, targets and indicators to encompass functional, social, economic and cultural aspects. Second, the scale of, and uncertainties associated with, climate change effects require that EWM adopt both a broader and more nuanced consideration of its spatial and temporal framing, i.e., both in terms of embracing a wider view and recognizing the spatial heterogeneity and temporal variability involved at finer scales. Finally, effective adaptation of EWM, and ultimately its transformation, will depend on its successful alignment and integration, with respect to both water management more broadly and other sectors such as agriculture and energy production. This final guiding principle conforms to the principles of integrated water resources management, which is itself a target within the freshwater-focused Sustainable Development Goal 6 (United Nations, 2016). Broadening EWM to encompass all aspects of water use and management enables a more integrated and holistic approach to deliver the needs of people and environment (Ludwig et al., 2013; Horne et al., 2017a).
Objectives and Targets
Throughout the world, environmental flow studies and EWM has typically been triggered by highly visible signs of environmental degradation (e.g., biodiversity declines, species invasions, toxic algal blooms) and have thus often sought to reactively address specific concerns involving particular taxa (e.g., riparian trees, fish or waterbirds), ecosystems (e.g., iconic wetlands) and/or, to a much lesser extent, human well-being (Arthington and Pusey, 2003; Poff, 2009). Conventional objectives of EWM in many cases have been to deliver flows which support the habitat and life-history requirements of selected taxa with more holistic approaches generally seeking to reinstate historical “natural” flow regimes to restore freshwater ecosystems and their biota to some semblance of “pre-development” conditions (Table 1; Poff et al., 2007; Poff, 2018). In Australia's Murray-Darling Basin, for example, objectives for environmental watering often include the maintenance or restoration of historical extents of key vegetation communities in particular wetland ecosystems (Capon and Capon, 2017). Similarly backwards-looking objectives are also promoted through the management aims of the Ramsar Convention which requires signatory parties to maintain the ecological character of listed wetlands in the condition described at the time of listing (Finlayson et al., 2017a). Such approaches to EWM assume that: (1) past flow regimes are desirable for both present and future conditions (Capon and Capon, 2017); (2) ecological integrity will improve within a system once historic flow attributes are re-instated (sensu the “Field of Dreams hypothesis”; Palmer et al., 1997; Hilderbrand et al., 2005); (3) ecosystems have an optimal state and restoration has a static endpoint (Capon and Capon, 2017); and (4) flow is a master variable, distinct from other ecologically important drivers that may impact water quantity and quality, e.g., land use and sediment dynamics (Karr, 1991; Poff et al., 1997; Poff and Matthews, 2013). These assumptions are difficult to justify, however, in the face of a rapidly changing and increasingly extreme and unpredictable climate (Milly et al., 2008; Poff and Matthews, 2013; Poff et al., 2017) on a human-dominated planet in which many rivers and wetlands exist within catchments drastically modified in terms of their geomorphology, sediment delivery and vegetation (Acreman et al., 2014a; Davies et al., 2014). Furthermore, there is growing recognition that ecosystems are not static but rather dynamic systems that exhibit a wide range of trajectories of socio-ecological change in both space and time (Suding et al., 2004; Capon and Capon, 2017; Poff, 2018).
Under climate change, developing environmental flow and EWM objectives based either on historic flow regimes or structural ecological targets associated with particular taxa or local ecosystem attributes is increasingly both unrealistic and undesirable (Poff et al., 2017). Solely with respect to ecological outcomes, for instance, robust objectives must consider the probability of shifts in species' distributions and the appearance of novel ecosystems as well as emerging triggers for EWM beyond restoration or rehabilitation, e.g., protection of refuge habitats or provision of corridors for species migration (Davies, 2010; Acreman et al., 2014a; Moyle, 2014). Growing water scarcity also calls for better integration, and therefore efficiency, of water management objectives for human and environmental purposes. Climate change thus prompts a need to systematically develop multiple integrated objectives for EWM that incorporate socio-economic, cultural and ecological aspects (Dunlop et al., 2013). In particular, adaptive EWM goals might have a greater emphasis on ecosystem functions and services valued by society, e.g., water filtration, bank stability, shading, cultural values etc. (Capon and Capon, 2017). Specific objectives relating to the resilience or adaptive capacity of particular ecological functions or values may also be appropriate, especially in catchments which are characterized by high levels of climate variability and extreme events (Jones et al., 2012). Transformative EWM objectives might even include over-restoration of wetland ecosystems (e.g., Davies, 2010), such that certain ecological functions are enhanced beyond their historical limits, e.g., creation of new aquatic refuges where climate change has negatively impacted historical ones. Such designer EWM objectives may become the norm as natural environments are replaced by novel and/or managed systems that are valued for their particular benefits to ecosystems and people (Acreman et al., 2014a). To be equitable, however, EWM goals may also need to consider the values and maintenance of wild rivers and naturalness (e.g., Ridder, 2007; Arthington, 2012). Indeed, appropriate goals for EWM will vary between highly regulated and developed catchments and those which are less modified and set aside as protected areas (Finlayson et al., 2017b; Finlayson and Pittock, 2018). In less modified catchments, for example, more open-ended ecological goals for unregulated water management might be appropriate (Capon et al., 2013) with a focus on promoting more climate-resilience rather than maintaining past reference states (Finlayson and Pittock, 2018).
To avoid perverse outcomes and maladaptation, adapted EWM objectives and targets also need to be developed with respect to multiple nested spatial and temporal scales and take into account connectivity and spatial heterogeneity (McCluney et al., 2014). Local objectives for particular wetlands, for example, might be designed in relation to those developed for wetlands with which they are hydrologically or otherwise connected as well as those set for larger levels of spatial organization, such as river basins and broader landscape scales (e.g., waterbird flyways). Similarly, different goals will be required for the short-, medium- and long-term, especially in relation to climate change adaptation of EWM, and these also need to be appropriately aligned so that long-term transformation is not prohibited by actions in the short-term (Finlayson et al., 2017a). Finally, because EWM is itself critical to the adaptation of human society to climate change, transformative EWM objectives and targets should additionally be developed in conjunction with broader adaptation strategies and goals of water management more generally, like those associated with the Sustainable Development Goals (SDG) and SDG6 in particular, as well as those of other sectors (Hadwen et al., 2015; United Nations, 2016).
Planning and Prioritization
Systematic spatial and temporal planning and prioritization of environmental watering actions are increasingly critical under climate change (Adams et al., 2017), especially given the need outlined above for more nuanced and aligned environmental flow and EWM objectives and targets over multiple scales. Furthermore, planning under climate change must take into account the many uncertainties involved including multiple plausible trajectories of change over the long term (e.g., Representative Concentration Pathways) as well as the possibility of extreme climatic events (e.g., heat waves, mega-droughts etc.) and other surprises in the short-term (Leigh et al., 2015), all of which generate high levels of uncertainty regarding both the supply of and demand for environmental water. Uncertainties relating to human responses to climate change and planning in other sectors (e.g., agriculture) will also influence environmental water availability and needs in space and time.
Rather than the traditional focus of environmental flows and EWM on reinstating historic flow regimes (Table 1), climate change calls for actively designing flows which address set objectives and are adaptive, resilient and robust across a range of scenarios, especially in regulated and highly modified catchments (Acreman et al., 2014a,b; Rockström et al., 2014). Such designer flow regimes could incorporate a provision to deliver “emergency flows” in response to extreme events or other surprises, e.g., dilution flows in response to pollution events, or flows to support unexpected waterbird breeding events. As per setting climate-ready EWM objectives and targets, planning and prioritizing environmental watering actions and designing flow regimes under climate change should be conducted across multiple spatial and temporal scales. Rivers, for example, require planning at catchment and basin scales while wetlands typically need finer scale priorities (Palmer et al., 2008). Conventional approaches to EMW have often focused on iconic wetlands (Swirepik et al., 2016) rather than whole catchments, with limited regard for the shifting habitat mosaics which comprise freshwater ecosystems and their associated landscapes and which drive dynamic ecosystem processes and biodiversity patterns (Datry et al., 2016). Instead, environmental water delivery needs to be prioritized at basin and broader regional scales (sensu the ELOHA framework: Table 1) to account for landscape connectivity and network structure (McCluney et al., 2014) and to better enable consideration of tradeoffs and synergies between ecological, social, economic and cultural values (Capon and Capon, 2017). Limited information and predictive certainty at local scales also requires ecologists, natural resource managers and policy makers to broaden their spatial scale of actionable influence (Matthews et al., 2011; Poff and Matthews, 2013).
The uncertainties associated with climate change further compel greater flexibility and adaptability in EWM planning. Multiple planning pathways, for instance, might be developed in which objectives, targets, priorities and designer flow regimes vary in relation to antecedent or projected conditions (e.g., prolonged drought). Such plans would require the inclusion of trigger points to dictate when shifts between different management regimes should occur. While conventional EWM plans have typically had limited consideration of temporal context (Rolls et al., 2012), some recent exemplary environmental water plans, e.g., the Murray-Darling Basin Plan, have begun to incorporate multiple time frames (e.g., annual and long-term plans) with decision points shaped by temporal context and conditions (e.g., drought vs. flood years; MDBA, 2014); this approach has been adopted in other jurisdictions (e.g., the state of Victoria).
To minimize the risk of maladaptation, adaptive EWM planning should also occur in conjunction with planning concerning water resources infrastructure, e.g., extension or construction of new water storage or abandonment of infrastructure at high risk of stranding (e.g., Winemiller et al., 2016). Transformative EWM planning would also ideally be aligned with planning in other sectors, e.g., conservation, agriculture, urban planning etc. (Adams et al., 2017) so as to consider, for instance, potential threats to the effective delivery or outcomes of environmental water actions posed by activities in other sectors as well as risks posed by in turn by environmental water to other sectors (e.g., drowning of crops).
Flow Delivery
Delivery of environmental water under climate change is likely to face considerable challenges in relation to water supply, especially in drying catchments where environmental water may be sacrificed to meet human demands. Adaptation approaches will be highly idiosyncratic depending on context, especially levels of river regulation and catchment modification. In regulated rivers, for example, adaptive environmental water delivery may entail dam reoperation (e.g., revised release rules or floodplain management) which takes into account risks and uncertainty associated with climate change (Watts et al., 2011; Poff et al., 2016). Expansion and construction of environmental water delivery works (e.g., pipes and levees to deliver and retain water on floodplains) might also be employed to enable watering of high value assets (e.g., floodplain forests). Such approaches, however, are associated with a high risk of perverse outcomes (Bond et al., 2014; Capon S. J. et al., 2017) and might be considered as either a last resort or a “band-aid” approach until other options become available. Hard engineering adaptation approaches to water delivery further risk stranding and/or mass failure and should be constructed with safety margins and regular reviews (Capon et al., 2013; Capon and Bunn, 2015). In unregulated catchments, environmental water delivery is typically achieved via rules governing water extraction, diversions and storage which might similarly be revised in light of climate change risks (Bond et al., 2008). The effectiveness of such delivery mechanisms, however, will depend on adherence to these rules which, in turn, may depend on both institutional (e.g., monitoring and regulation) and social and cultural factors. Such adaptation approaches might therefore be supported by “soft” strategies aimed at fostering community involvement in the development and enforcement of environmental water rules.
Effective delivery of environmental water under climate change will be particularly promoted through improved integration of EMW with actions in water resources management more broadly as well as those in other sectors. Greater alignment of surface and ground water management, for example, may enhance capacity to deliver appropriate flows to many groundwater influenced freshwater ecosystems (e.g., Arthington, 2012; Gleeson and Richter, 2017). Similarly, flows delivered primarily for human demands (e.g., irrigation) can be designed so that ecological benefits are maximized, e.g., by “piggybacking” irrigation releases with environmental water (Watts et al., 2011). In turn, environmental water could be delivered so that socio-economic and cultural benefits (e.g., religious celebrations, recreational use) are also maximized (e.g., Jackson, 2017). Finally, the quantity and quality of water available for environmental watering actions, as well as ecological responses to these, are very likely to be influenced by pressures in the broader catchment (e.g., vegetation clearing; Davis et al., 2015). Consequently, improved catchment and riparian management is likely to play an important role in adapting environmental water delivery and sustaining ecosystems and livelihoods that depend on EWM (e.g., Stewart-Koster et al., 2010; Sheldon et al., 2012).
Monitoring and Evaluation
Monitoring and evaluation (M&E) of environmental water actions have often been sparse under conventional environmental flow and EWM programs which have therefore generated limited understanding of whether or not interventions have achieved their objectives or, indeed, if objectives are even appropriate (Souchon et al., 2008; King et al., 2015). Climate change compels that considerable effort be directed toward M&E, however, so that ecological and human benefits of EWM can be demonstrated and adaptive management and learning appropriately supported. King et al. (2015) identify three major types of monitoring programs in EWM, all of which will be needed to adequately evaluate and adapt EWM in the face of climate change: (1) condition or program-level monitoring to assess ecological changes over large spatial and temporal scales; (2) compliance or operational monitoring focusing on water delivery targets; and (3) intervention monitoring to assesses responses to specific management interventions that may occur over both short and longer time periods.
To inform adaptive management, M&E must be clearly aligned with management objectives and targets which therefore need to be as specific as possible so that they can be both measured and evaluated while accounting for multiple possible outcomes (McDonald-Madden et al., 2010; King et al., 2015). Consequently, the selection of indicators used to monitor EWM will probably need to be adapted in light of climate change given likely revisions of objectives and targets. In particular, functional ecological indicators (e.g., species traits) which reflect the resilience or adaptive capacity of ecological components and processes as well as socio-economic and cultural indicators describing the human benefits of EWM might be incorporated in addition to traditional structural ecological traits (e.g., species composition; Leigh and Datry, 2017). Holistic environmental flow frameworks (Table 1) facilitate input from diverse stakeholders and increasingly evaluate the social and cultural implications of environmental flows and water management alternatives (e.g., King and Brown, 2010; Finn and Jackson, 2011; Lokgariwar et al., 2014; Conallin et al., 2017). Poff (2018) also calls for a more robust and dynamic predictive science involving time-varying flow characterizations, and more use of process (e.g., demographic) rates and species traits rather than the present reliance on measurement of ecosystem state variables.
M&E related to the conservation of particular species or communities (e.g., threatened taxa, migratory waterbirds) must take into account shifting distributions of species in response to climate change (James et al., 2017). Because such changes are likely to occur both within and beyond the spatial confines of individual catchment planning regions or other jurisdictional boundaries, this emphasizes the need for collaborative M&E and adaptive management of EWM over multiple scales and institutional levels. Transformative M&E especially will require coordinated collection, evaluation and dissemination of monitoring data if responses of target species, ecosystems and landscapes to EWM are to be detected under climate change (Olden and Naiman, 2010; Wilby et al., 2010).
The benefits of monitoring and evaluating environmental flows using an adaptive management approach have long been recognized but unfortunately limited in application, perhaps because adopting such an approach or redesigning existing, non-adaptive programs accordingly can be somewhat daunting for managers and scientists alike (Richter et al., 2006; Pahl-Wostl, 2007; Webb et al., 2018). The challenges that climate change poses for EWM, however, make integrating M&E into broader planning and management frameworks essential to achieving effective outcomes and avoiding maladaptation. Nevertheless, adaptive management processes can take time with some indicators taking months or years to respond to particular flow interventions, delaying decisions on how or even whether to adapt plans for future interventions. A more variable climate means that environmental changes, including changes to river flows, may occur more rapidly and conventional (potentially slow) adaptive approaches may therefore need rethinking. To be transformative, EWM must be proactive and anticipatory rather than reactive (Pahl-Wostl, 2007; Bond et al., 2008; Wiens, 2016). Models that can predict likely outcomes of management interventions under different climate scenarios are therefore likely to become increasingly valuable as an M&E tool (Webb et al., 2018). Anticipating future climate scenarios (e.g., a drier or wetter future) using “signpost” indicators of change within a regular monitoring schedule to trigger pre-emptive action will also allow environmental water management to respond more adaptively to climate change. Additionally, real-time data may also be required to capture rapid changes in environmental conditions so that interventions and management practices can be adapted accordingly in a timely manner (Wilby et al., 2010; Costigan et al., 2017). Technological advances in the collection and analysis of “big data” make such proposals increasingly realistic.
Knowledge Needs
While there remains a paucity of knowledge concerning hydrological processes and flow-ecology linkages in most places (Arthington, 2012; Davies et al., 2014; Olden et al., 2014), effective adaptation and transformation of environmental flows and EWM under climate change is likely to be further hindered by several additional major areas of knowledge deficiency. In particular, relationships between ecosystem structure, function and the provision of ecosystem services, as well as how these respond to changes in flow, tend to be poorly understood in freshwater ecosystems (Dudgeon, 2014). Indeed, human values and benefits derived from freshwater ecosystems in general are not well understood or quantified, particularly with respect to how these are underpinned by flows and ecological responses to these (Arthington, 2015). Greater knowledge regarding likely effects of changes in climatic stimuli and extreme climatic events on all of these relationships, as well as their interactions with other drivers and pressures, is also needed to inform adaptation and transformation of EWM (Capon, S. et al., 2017). Linking human and environmental uses of water, through the lens of integrated water resources management, will require the adoption of connected systems-thinking approaches for EWM. Climate change offers an opportunity to link these oft segregated components of the system and deliver the needs of all in a transformative and proactive way.
Author Contributions
All authors listed have made a substantial, direct and intellectual contribution to the work, and approved it for publication.
Conflict of Interest Statement
The authors declare that the research was conducted in the absence of any commercial or financial relationships that could be construed as a potential conflict of interest.
Acknowledgments
We are grateful to the Australian Rivers Institute for supporting the writing group from which this paper arose.
References
Acreman, M. C., and Dunbar, M. J. (2004). Methods for defining environmental river flow requirements - a review. Hydrol. Earth Syst. Sci. 8, 121–133. doi: 10.5194/hess-8-861-2004
Acreman, M. C., Overton, I. C., King, J., Wood, P. J., Cowx, I. G., Dunbar, M. J., et al. (2014b). The changing role of ecohydrological science in guiding environmental flows. Hydrol. Sci. J. 59, 433–450. doi: 10.1080/02626667.2014.886019
Acreman, M., Arthington, A. H., Colloff, M. J., Couch, C., Crossman, N. D., Dyer, F., et al. (2014a). Environmental flows for natural, hybrid, and novel riverine ecosystems in a changing world. Front. Ecol. Environ. 12, 466–473. doi: 10.1890/130134
Adams, V. M., Álvarez-Romero, J. G., Capon, S. J., Crowley, G. M., Dale, A. P., Kennard, M. J., et al. (2017). Making time for space: the critical role of spatial planning in adapting natural resource management to climate change. Environ. Sci. Policy 74, 57–67. doi: 10.1016/j.envsci.2017.05.003
Arthington, A. H. (2012). Environmental Flows: Saving Rivers in the Third Millennium. Berkeley: University of California Press.
Arthington, A. H. (2015). Environmental flows: a scientific resource and policy tool for river conservation and restoration. Aquat. Conserv. 25, 155–161. doi: 10.1002/aqc.2560
Arthington, A. H., Bunn, S. E., Pusey, B. J., Blühdorn, D. R., King, J. M., Day, J. A., et al. (1992). “Development of an holistic approach for assessing environmental flow requirements of riverine ecosystems,” in Proceedings of an International Seminar and Workshop on Water Allocation for the Environment, eds J. J. Pigram and B. P. Hooper (Armidale, NSW: Centre for Water Policy Research), 69–76.
Arthington, A. H., and Pusey, B. J. (2003). Flow restoration and protection in Australian rivers. River Res. Appl. 19, 377–395. doi: 10.1002/rra.745
Bond, N. R., Lake, P. S., and Arthington, A. H. (2008). The impacts of drought on freshwater ecosystems: an Australian perspective. Hydrobiologia 600, 3–16. doi: 10.1007/s10750-008-9326-z
Bond, N., Costelloe, J., King, A., Warfe, D., Reich, P., and Balcombe, S. (2014). Ecological risks and opportunities from engineered artificial flooding as a means of achieving environmental flow objectives. Front. Ecol. Environ. 12, 386–394. doi: 10.1890/130259
Bovee, K. D. (1982). “A guide to stream habitat analysis using the instream flow incremental methodology,” in Instream Flow Information Paper 12. U.S.D.I. Fish Wildl. Serv., Office of Biol. Serv. FWS/OBS-82/26, 248.
Brizga, S. O., Arthington, A. H., Pusey, B. J., Kennard, M. J., Mackay, S. J., and Werren, G. L. (2002). “Benchmarking, a “top-down” methodology for assessing environmental flows in Australian rivers,” in Environmental Flows for River Systems. An International Working Conference on Assessment and Implementation, incorporating the 4th International Ecohydraulics Symposium. Conference Proceedings. Cape Town: University of Cape Town.
Capon, S. J., Balcombe, S. R., and McBroom, J. (2017). Environmental watering for vegetation diversity outcomes must account for local canopy conditions. Ecohydrology 10:e1859. doi: 10.1002/eco.1859
Capon, S. J., and Bunn, S. E. (2015). Assessing climate change risks and prioritising adaptation options using a water ecosystem services-based approach. Water Ecosyst. Serv. Glob. Perspect. 17, 17–25. doi: 10.1017/CBO9781316178904.004
Capon, S. J., and Capon, T. R. (2017). An impossible prescription: why science cannot determine environmental water requirements for a healthy Murray-darling Basin. Water Econ. Policy 3:1650037. doi: 10.1142/S2382624X16500375
Capon, S. J., Chambers, L. E., Mac Nally, R., Naiman, R. J., Davies, P., Marshall, N., et al. (2013). Riparian ecosystems in the 21st century: hotspots for climate change adaptation?. Ecosystems 16, 359–381. doi: 10.1007/s10021-013-9656-1
Capon, S., Chambers, J., Barmuta, L., Bino, G., Bond, N., Costelloe, J., et al. (2017). National Climate Change Adaptation Research Plan for Freshwater Ecosystems and Biodiversity: Update 2017. Gold Coast, QLD: National Climate Change Adaptation Research Facility.
Conallin, J. C., Dickens, C., Hearne, D., and Allan, C. (2017). “Stakeholder engagement in environmental water management,” in Water for the Environment: From Policy and Science to Implementation and Management, eds A. Horne, A. Webb, M. Stewardson, B. Richter, and M. Acreman (London: Elsevier Press), 129–150.
Costigan, K. H., Kennard, M. J., Leigh, C., Sauquet, E., Datry, T., and Boulton, A. J. (2017). “Flow regimes in intermittent rivers and ephemeral streams,” in Intermittent Rivers Ephemeral Streams, eds T. Datry, N. Bonada, and A. Boulton (London: Academic Press), 51–78. doi: 10.1016/B978-0-12-803835-2.00003-6
Datry, T., Boulton, A. J., Bonada, N., Fritz, K., Leigh, C., Sauquet, E., et al. (2017). Flow intermittence and ecosystem services in rivers of the Anthropocene. J. Appl. Ecol. 55, 1–12. doi: 10.1111/1365-2664.12941
Datry, T., Pella, H., Leigh, C., Bonada, N., and Hugueny, B. (2016). A landscape approach to advance intermittent river ecology. Freshw. Biol. 61, 1200–1213. doi: 10.1111/fwb.12645
Davies, P. M. (2010). Climate change implications for river restoration in global biodiversity hotspots. Restor. Ecol. 18, 261–268. doi: 10.1111/j.1526-100X.2009.00648.x
Davies, P. M., Naiman, R. J., Warfe, D. M., and Bunn, S. E. (2014). Flow–ecology relationships: closing the loop on effective environmental flows. Mar. Freshw. Res. 65, 133–141. doi: 10.1071/MF13110
Davis, J., O'Grady, A. P., Dale, A., Arthington, A. H., Gell, P. A., Driver, P. D., et al. (2015). When trends intersect: the challenge of protecting freshwater ecosystems under multiple land use and hydrological intensification scenarios. Sci. Total Environ. 534, 65–78. doi: 10.1016/j.scitotenv.2015.03.127
Dhungel, S., Tarboton, D. G., Jin, J., and Hawkins, C. P. (2016). Potential effects of climate change on ecologically relevant streamflow regimes. River Res. Appl. 32, 1827–1840. doi: 10.1002/rra.3029
Döll, P., and Schmied, H. M. (2012). How is the impact of climate change on river flow regimes related to the impact on mean annual runoff ? A global-scale analysis. Environ. Res. Lett. 7, 14–37. doi: 10.1088/1748-9326/7/1/014037
Dudgeon, D. (2014). “Threats to freshwater biodiversity in a changing world,” in Global Environmental Change, ed B. Freedman (Dordrecht: Springer), 243–253.
Dunlop, M., Parris, H., and Ryan, P. (2013). Climate-ready Conservation Objectives: a Scoping Study. Gold Coast, QLD: National Climate Change Adaptation Research Facility.
Dyson, M., Bergkamp, G., and Scanlon, J. (2003). Flow: The Essentials of Environmental Flows. Gland; Cambridge: IUCN.
Finlayson, C. M., Athington, A. H., and Pittock, J. (2017b). Freshwater Ecosystems in Protected Areas: Conservation and Management. Oxford: Taylor and Francis.
Finlayson, C. M., Capon, S. J., Rissik, D., Pittock, J., Fisk, G., Davidson, N. C., et al. (2017a). Policy considerations for managing wetlands under a changing climate. Mar. Freshw. Res. 68, 1803–1815. doi: 10.1071/MF16244
Finlayson, C. M., and Pittock, J. (2018). “Climate change and the management of freshwater protected areas,” in Freshwater Ecosystems in Protected Areas: Conservation and Management, eds C. M. Finlayson, A. H. Arthington, and J. Pittock (Oxford: Taylor and Francis), 242–255.
Finn, M., and Jackson, S. (2011). Protecting indigenous values in water management: a challenge to conventional environmental flow assessments. Ecosystems 14, 1232–1248. doi: 10.1007/s10021-011-9476-0
Gleeson, T., and Richter, B. (2017). How much groundwater can we pump and protect environmental flows through time? Presumptive standards for conjunctive management of aquifers and rivers. River Res. Appl. 34, 1–10.
Grey, D., Garrick, D., Blackmore, D., Kelman, J., Muller, M., and Sadoff, C. (2013). Water security in one blue planet: twenty-first century policy challenges for science. Philos. Trans. R. Soc. A 371:20120406. doi: 10.1098/rsta.2012.0406
Hadwen, W. L., and Capon, S. J. (2014). Navigating from climate change impacts to adaptation actions in coastal ecosystems. Appl. Stud. Clim. Adapt. 190, 190–199. doi: 10.1002/9781118845028.ch21
Hadwen, W. L., Powell, B., MacDonald, M. C., Elliott, M., Chan, T., Gernjak, W., et al. (2015). Putting WASH in the water cycle: Climate change, water resources and the future of water, sanitation and hygiene challenges in Pacific Island Countries. J. Water Sanitat. Hyg. Dev. 5, 183–191. doi: 10.2166/washdev.2015.133
Hall, J. W., Grey, D., Garrick, D., Fung, F., Brown, C., Dadson, S. J., et al. (2014). Coping with the curse of freshwater variability. Science 346, 429–430. doi: 10.1126/science.1257890
Herricks, E. E., and Bergner, E. R. (2003). Prediction of climate change effects of fish communities in the Mackinaw River watershed, Illinois, USA. Water Sci. Tech. 48, 199–207. doi: 10.2166/wst.2003.0575
Hilderbrand, R. H., Watts, A. C., and Randle, A. M. (2005). The myths of restoration ecology. Ecol. Soc. 10:19. doi: 10.5751/ES-01277-100119
Horne, A. C., O'Donnell, E. L., Webb, J. A., Stewardson, M. J., Acreman, M., and Richter, B. (2017b). “The environmental water management cycle,” in Water for the Environment: From Policy and Science to Implementation and Management, eds A. Horne, J. Webb, M. Stewardson, M. Acreman, and B. D. Richter (Cambridge, MA: Elsevier) 3–16.
Horne, A. C., Webb, J. A., Stewardson, M. J., Richter, B. D., and Acreman, M. (2017a). Water for the Environment: From Policy and Science to Implementation and Management. Cambridge, MA: Elsevier.
IPCC (2012). “Managing the risks of extreme events and disasters to advance climate change adaptation,” in A Special Report of Working Groups I and II of the Intergovernmental Panel on Climate Change (New York, NY; Cambridge: Cambridge University Press).
IPCC (2014). Climate Change 2014: Synthesis Report. Contribution of Working Groups I, II and III to the Fifth Assessment Report of the Intergovernmental Panel on Climate Change, eds Core Writing Team, R. K. Pachauri, and L. A. Meyer (Geneva: IPCC), 151.
Jackson, S. (2017). “How much water does a culture need? Environmental water management's cultural challenge and indigenous responses,” in Water for the Environment: From Policy and Science to Implementation and Management, eds A. Horne, J. Webb, M. Stewardson, M. Acreman, and B. D. Richter (Cambridge, MA: Elsevier), 173–188.
James, C. S., Reside, A. E., VanDerWal, J., Pearson, R. G., Burrows, D., Capon, S. J., et al. (2017). Sink or swim? Potential for high faunal turnover in Australian rivers under climate change. J. Biogeogr. 44, 489–501. doi: 10.1111/jbi.12926
Jones, H. P., Hole, D. G., and Zavaleta, E. S. (2012). Harnessing nature to help people adapt to climate change. Nat. Clim. Change 2, 504–509. doi: 10.1038/nclimate1463
Karr, J. R. (1991). Biological integrity: a long-neglected aspect of water resource management. Ecol. Appl. 1, 66–84. doi: 10.2307/1941848
Kendy, E., Apse, C., and Blann, K. (2012). A Practical Guide to Environmental Flows for Policy and Planning. Arlington, VA: The Nature Conservancy.
King, A. J., Gawne, B., Beesley, L., Koehn, J. D., Nielsen, D. L., Price, A., et al. (2015). Improving ecological response monitoring of environmental flows. Environ. Manage. 55, 991–1005. doi: 10.1007/s00267-015-0456-6
King, J. M., and Brown, C. A. (2010). Integrated basin flow assessments: concepts and method development in Africa and south-east Asia. Freshw. Biol. 55, 127–146. doi: 10.1111/j.1365-2427.2009.02316.x
King, J. M., and Louw, M. D. (1998). Instream flow assessments for regulated rivers in South Africa using the Building Block Methodology. Aquat. Ecosyst. Health Manag. 1, 109–124. doi: 10.1016/S1463-4988(98)00018-9
King, J., Beuster, H., Brown, C., and Joubert, A. (2014). Pro-active management: the role of environmental flows in transboundary cooperative planning for the Okavango River system. Hydrol. Sci. J. 59, 786–800. doi: 10.1080/02626667.2014.888069
King, J. M., Brown, C. A., and Sabet, H. (2003). A scenario-based holistic approach for environmental flow assessments. River Res. Appl. 19: 619–639. doi: 10.1002/rra.709
Ledger, M. E., and Milner, A. M. (2015). Extreme events in running waters. Freshw. Biol. 60, 2455–2460. doi: 10.1111/fwb.12673
Leigh, C., Boulton, A. J., Courtwright, J. L., Fritz, K., May, C. L., Walker, R. H., et al. (2016). Ecological research and management of intermittent rivers: an historical review and future directions. Freshw. Biol. 61, 1181–1199. doi: 10.1111/fwb.12646
Leigh, C., Bush, A., Harrison, E. T., Ho, S. S., Luke, L., Rolls, R. J., et al. (2015). Ecological effects of extreme climatic events on riverine ecosystems: insights from Australia. Freshw. Biol. 60, 2620–2638. doi: 10.1111/fwb.12515
Leigh, C., and Datry, T. (2017). Drying as a primary hydrological determinant of biodiversity in river systems: a broad-scale analysis. Ecogr. 40, 487–499. doi: 10.1111/ecog.02230
Linnansaari, T., Monk, W. A., Baird, D. J., and Curry, R. A. (2012). Review of approaches and methods to assess Environmental Flows across Canada and internationally. DFO Can. Sci. Adv. Secretar. Res. Document 39, 1–74.
Lokgariwar, C., Chopra, V., Smakhtin, V., Bharati, L., and O'Keeffe, J. (2014). Including cultural water requirements in environmental flow assessment: an example from the upper Ganga River, India. Water Int. 39, 81–96. doi: 10.1080/02508060.2013.863684
Ludwig, F., van Slobbe, E., and Cofine, W. (2013). Climate change adaptation and integrated water resource management in the water sector. J. Hydrol. 518, 235–242. doi: 10.1016/j.jhydrol.2013.08.010
Matthews, J. H., Wickel, B. A. J., and Freeman, S. (2011). Converging currents in climate-relevant conservation: water, infrastructure, and institutions. PLoS Biol. 9:e1001159. doi: 10.1371/journal.pbio.1001159
McCluney, K. E., Poff, N. L., Palmer, M. A., Thorp, J. H., Poole, G. C., Williams, B. S., et al. (2014). Riverine macrosystems ecology: sensitivity, resistance, and resilience of whole river basins with human alterations. Front. Ecol. Environ. 12, 48–58. doi: 10.1890/120367
McDonald-Madden, E., Baxter, P. W., Fuller, R. A., Martin, T. G., Game, E. T., Montambault, J., et al. (2010). Monitoring does not always count. Trends Ecol. Evol. 25, 547–550. doi: 10.1016/j.tree.2010.07.002
McManamay, R. A., Orth, D. J., Dolloff, C. A., and Mathews, D. C. (2013). Application of the ELOHA framework to regulated rivers in the Upper Tennessee River Basin: a case study. Environ. Manage. 51, 1210–1235. doi: 10.1007/s00267-013-0055-3
MDBA (2014). Basin-wide Environmental Watering Strategy. Canberra, ACT: Murray-Darling Basin Authority.
Milly, P. C. D., Betancourt, J., Falkenmark, M., Hirsch, R. M., Kundzewicz, Z. W., Lettenmaier, D. P., et al. (2008). Stationarity is dead: whither water management? Science 319, 573–574. doi: 10.1126/science.1151915
Milly, P. C., Dunne, K. A., and Vecchia, A. V. (2005). Global pattern of trends in streamflow and water availability in a changing climate. Nature 438, 347–350. doi: 10.1038/nature04312
Moyle, P. B. (2014). Novel aquatic ecosystems: the new reality for streams in California and other Mediterranean climate regions. River Res. Appl. 30, 1335–1344. doi: 10.1002/rra.2709
Olden, J. D., Konrad, C. P., Melis, T. S., Kennard, M. J., Freeman, M. C., and Williams, M. C. (2014). Are large-scale flow experiments informing the science and management of freshwater ecosystems? Front. Ecol. Environ. 12, 176–185. doi: 10.1890/130076
Olden, J. D., and Naiman, R. J. (2010). Incorporating thermal regimes into environmental flows assessments: modifying dam operations to restore freshwater ecosystem integrity. Freshw. Biol. 55, 86–107. doi: 10.1111/j.1365-2427.2009.02179.x
Pahl-Wostl, C. (2007). Transitions towards adaptive management of water facing climate and global change. Water Resour. Manage. 21, 49–62. doi: 10.1007/s11269-006-9040-4
Palmer, M. A., Ambrose, R. F., and Poff, N. L. (1997). Ecological theory and community restoration ecology. Restoration Ecol. 5, 291–300. doi: 10.1046/j.1526-100X.1997.00543.x
Palmer, M. A., Reidy Liermann, C. A., Nilsson, C., Flörke, M., Alcamo, J., Lake, P. S., et al. (2008). Climate change and the world's river basins: anticipating management options. Front. Ecol. Environ. 6, 81–89. doi: 10.1890/060148
Peirson, W., Davey, E., Jones, A., Hadwen, W., Bishop, K., Beger, M., et al. (2015). Opportunistic management of estuaries under climate change: a new adaptive decision-making framework and its practical application. J. Environ. Manage. 163, 214–223. doi: 10.1016/j.jenvman.2015.08.021
Poff, N. L. (2009). Managing for variability to sustain freshwater ecosystems. J. Water Resour. Plann. Manage. 135, 1–4. doi: 10.1061/(ASCE)0733-9496(2009)135:1(1)
Poff, N. L. (2018). Beyond the natural flow regime? Broadening the hydro-ecological foundation to meet environmental flows challenges in a non-stationary world. Freshw. Biol. 63, 1011–1021. doi: 10.1111/fwb.13038
Poff, N. L., Allan, J. D., Bain, M. B., Karr, J. R., Prestegaard, K. L., Richter, B. D., et al. (1997). The natural flow regime. Bioscience 47, 769–784. doi: 10.2307/1313099
Poff, N. L., Brown, C. M., Grantham, T. E., Matthews, J. H., Palmer, M. A., and Baeza, C. M. (2016). Sustainable water management under future uncertainty with eco-engineering decision scaling. Nat. Clim. Chang. 6, 25–34. doi: 10.1038/nclimate2765
Poff, N. L., and Matthews, J. H. (2013). Environmental flows in the Anthropocence: past progress and future prospects. Curr. Opin. Environ. Sustain. 5, 667–675. doi: 10.1016/j.cosust.2013.11.006
Poff, N. L., Olden, J. D., Merritt, D. M., and Pepin, D. M. (2007). Homogenization of regional river dynamics by dams and global biodiversity implications. Proc. Natl. Acad. Sci. U.S.A. 104, 5732–5737. doi: 10.1073/pnas.0609812104
Poff, N. L., Richter, B. D., Arthington, A. H., Bunn, S. E., Naiman, R. J., Kendy, E., et al. (2010). The ecological limits of hydrologic alteration (ELOHA): a new framework for developing regional environmental flow standards. Freshw. Biol. 55, 147–170. doi: 10.1111/j.1365-2427.2009.02204.x
Poff, N. L., Tharme, R. E., and Arthington, A. H. (2017). “Evolution of environmental flows assessment science, principles, and methodologies,” in Water for the Environment: from Policy and Science to Implementation and Management (Cambridge, MA: Elsevier).
Richter, B. D., Baumgartner, J. V., Powell, J., and Braun, D. P. (1996). A method for assessing hydrologic alteration within ecosystems. Conserv. Biol. 10, 1163–1174. doi: 10.1046/j.1523-1739.1996.10041163.x
Richter, B. D., Baumgartner, J. V., Wigington, R., and Braun, D. P. (1997). How much water does a river need? Freshw. Biol. 37, 231–249. doi: 10.1046/j.1365-2427.1997.00153.x
Richter, B. D., Warner, A. T., Meyer, J. L., and Lutz, K. (2006). A collaborative and adaptive process for developing environmental flow recommendations. River Res. Applic. 22, 297–318. doi: 10.1002/rra.892
Ridder, B. (2007). An exploration of the value of naturalness and wild nature. J. Agri. Environ. Ethics 20, 195–213. doi: 10.1007/s10806-006-9025-6
Rockström, J., Falkenmark, M., Allan, T., Folke, C., Gordon, L., Jägerskog, A., et al. (2014). The unfolding water drama in the Anthropocene: towards a resilience-based perspective on water for global sustainability. Ecohydrology 7, 1249–1261. doi: 10.1002/eco.1562
Rolls, R. J., Leigh, C., and Sheldon, F. (2012). Mechanistic effects of low-flow hydrology on riverine ecosystems: ecological principles and consequences of alteration. Freshw. Sci. 31, 1163–1186. doi: 10.1899/12-002.1
Sheldon, F., Peterson, E. E., Boone, E. L., Sippel, S., Bunn, S. E., and Harch, B. D. (2012). Identifying the spatial scale of land use that most strongly influences overall River Ecosystem Health Score. Ecol. Appl. 22, 2188–2203. doi: 10.1890/11-1792.1
Souchon, Y., Sabaton, C., Diebel, R., Reiser, D., Kershner, J. L., Gard, M., et al. (2008). Detecting biological responses to flow management: missed opportunities; future directions. River Res. Appl. 24, 506–518. doi: 10.1890/11-1792.1
Stewart-Koster, B., Bunn, S. E., Mackay, S. J., Poff, N. L., Naiman, P. J., and Lake, P. S. (2010). The use of Bayesian networks to guide investments in flow and catchment restoration for impaired river ecosystems. Freshw. Biol. 55, 243–226. doi: 10.1111/j.1365-2427.2009.02219.x
Suding, K. N., Gross, K. L., and Houseman, G. R. (2004). Alternative states and positive feedbacks in restoration ecology. Trends Ecol. Evol. 19, 46–53.doi: 10.1016/j.tree.2003.10.005
Swirepik, J. L., Burns, I. C., Dyer, F. J., Neave, I. A., O'Brien, M. G., Pryde, G. M., et al. (2016). Establishing environmental water requirements for the Murray–Darling Basin, Australia's largest developed river system. River Res. Appl. 32, 1153–1165. doi: 10.1002/rra.2975
Tennant, D. L. (1976). Instream flow regimens for fish, wildlife, recreation and related environmental resources. Fisheries 1, 6–10.
Tharme, R. E. (2003). A global perspective on environmental flow assessment: emerging trends in the development and application of environmental flow methodologies for rivers. River Res. Appl. 19, 397–441. doi: 10.1002/rra.736
Thompson, J. R., Laizé, C. L. R., Green, A. J., Acreman, M. C., and Kingston, D. G. (2014). Climate change uncertainty in environmental flows for the Mekong River. Hydrol. Sci. J. 59, 935–954. doi: 10.1080/02626667.2013.842074
Vörösmarty, C. J., McIntyre, P. B., Gessner, M. O., Dudgeon, D., Prusevich, A., Green, P., et al. (2010). Global threats to human water security and river biodiversity. Nature 467:555. doi: 10.1038/nature09440
Walsh, C. L. (2004). Simulation and Analysis of River Flow Regimes. Implications for Sustainable Management of Atlantic Salmon (Salmo salar) Under Climate Change. Unpublished Ph.D. Thesis, School of Civil Engineering and Geosciences, University of Newcastle upon Tyne, UK.
Watts, R. J., Richter, B. D., Opperman, J. J., and Bowmer, K. H. (2011). Dam reoperation in an era of climate change. Mar. Freshw. Res. 62, 321–327. doi: 10.1071/MF10047
Webb, J. A., Watts, R. J., Allan, C., and Conallin, J. C. (2018). Adaptive management of environmental flows. Environ. Manage. 61, 339–346. doi: 10.1007/s00267-017-0981-6
Wiens, J. A. (2016). Ecological Challenges and Conservation Conundrums: Essays and Reflections for a Changing World. Chichester: John Wiley & Sons.
Wilby, R. L. (1994). Stochastic weather type simulation for regional climate change impact assessment. Water Resourc. Res. 30, 3395–3403. doi: 10.1029/94WR01840
Wilby, R. L., Orr, H., Watts, G., Battarbee, R. W., Berry, P. M., Chadd, R., et al. (2010). Evidence needed to manage freshwater ecosystems in a changing climate: turning adaptation principles into practice. Sci. Total Environ. 408, 4150–4164. doi: 10.1016/j.scitotenv.2010.05.014
Keywords: adaptation, climate change, environmental flows, hydrology, water resources, wetlands
Citation: Capon SJ, Leigh C, Hadwen WL, George A, McMahon JM, Linke S, Reis V, Gould L and Arthington AH (2018) Transforming Environmental Water Management to Adapt to a Changing Climate. Front. Environ. Sci. 6:80. doi: 10.3389/fenvs.2018.00080
Received: 01 March 2018; Accepted: 29 June 2018;
Published: 09 August 2018.
Edited by:
David Tickner, World Wide Fund for Nature, United KingdomReviewed by:
Lucia De Stefano, Complutense University of Madrid, SpainRenato Tavares Martins, Universidade Federal de Goiás, Brazil
Copyright © 2018 Capon, Leigh, Hadwen, George, McMahon, Linke, Reis, Gould and Arthington. This is an open-access article distributed under the terms of the Creative Commons Attribution License (CC BY). The use, distribution or reproduction in other forums is permitted, provided the original author(s) and the copyright owner(s) are credited and that the original publication in this journal is cited, in accordance with accepted academic practice. No use, distribution or reproduction is permitted which does not comply with these terms.
*Correspondence: Samantha J. Capon, cy5jYXBvbkBncmlmZml0aC5lZHUuYXU=